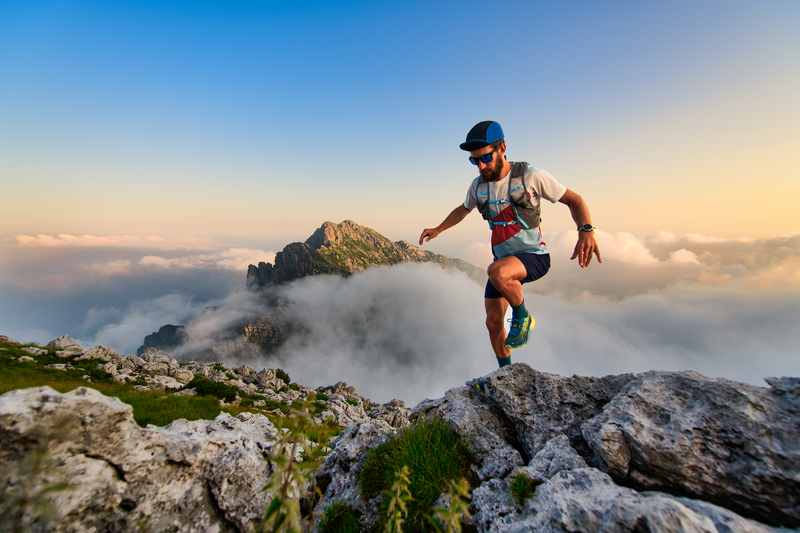
95% of researchers rate our articles as excellent or good
Learn more about the work of our research integrity team to safeguard the quality of each article we publish.
Find out more
ORIGINAL RESEARCH article
Front. Neurosci. , 08 March 2018
Sec. Perception Science
Volume 12 - 2018 | https://doi.org/10.3389/fnins.2018.00142
This article is part of the Research Topic From Perception to Action: The Role of Auditory and Visual Information in Perceiving and Performing Complex Movements View all 19 articles
The purpose of the study was to assess the influence of real-time auditory feedback on knee proprioception. Thirty healthy participants were randomly allocated to control (n = 15), and experimental group I (15). The participants performed an active knee-repositioning task using their dominant leg, with/without additional real-time auditory feedback where the frequency was mapped in a convergent manner to two different target angles (40 and 75°). Statistical analysis revealed significant enhancement in knee re-positioning accuracy for the constant and absolute error with real-time auditory feedback, within and across the groups. Besides this convergent condition, we established a second divergent condition. Here, a step-wise transposition of frequency was performed to explore whether a systematic tuning between auditory-proprioceptive repositioning exists. No significant effects were identified in this divergent auditory feedback condition. An additional experimental group II (n = 20) was further included. Here, we investigated the influence of a larger magnitude and directional change of step-wise transposition of the frequency. In a first step, results confirm the findings of experiment I. Moreover, significant effects on knee auditory-proprioception repositioning were evident when divergent auditory feedback was applied. During the step-wise transposition participants showed systematic modulation of knee movements in the opposite direction of transposition. We confirm that knee re-positioning accuracy can be enhanced with concurrent application of real-time auditory feedback and that knee re-positioning can modulated in a goal-directed manner with step-wise transposition of frequency. Clinical implications are discussed with respect to joint position sense in rehabilitation settings.
Real-time kinematic auditory feedback can be effective in enhancing motor perception, control, and learning (Effenberg, 2005, 2014; Sigrist et al., 2015; Effenberg et al., 2016; Dyer J. et al., 2017). The perception of additional real-time acoustic feedback driven by dynamic or kinematic movement parameters obviously supports sensory/perceptual-motor representations (Effenberg, 2005; Schmitz et al., 2013) by enhancing cross-modal stimulation (Scholz et al., 2015; Ghez et al., 2017), multisensory integration (Schmitz et al., 2013; Effenberg et al., 2016), internal motor simulation (Schmitz and Effenberg, 2017), and neural plasticity (Altenmüller et al., 2009; Ghai et al., 2017c). Literature indicates strong associations between auditory and motor areas for enhancing the performance in music (Lahav et al., 2013), breathing (Murgia et al., 2016), writing (Effenberg et al., 2015; Danna and Velay, 2017), sports (Sigrist et al., 2013, 2015; Effenberg et al., 2016), and rehabilitation (Altenmüller et al., 2009; Murgia et al., 2015; Pau et al., 2016; Scholz et al., 2016; Ghai et al., 2017c; Mezzarobba et al., 2017). Strong auditory motor couplings have also been confirmed in neuroimaging studies, where enhanced activation in cortical and sub-cortical structures associated with biological motion perception were reported (Scheef et al., 2009; Schmitz et al., 2013). Several underlying theories have been suggested to ascertain the beneficial effects of concurrent auditory feedback on motor performance. For instance, the concurrent auditory feedback is thought to amplify the brain's ability to integrate multiple congruent perceptual streams, leading to formation of stable internal feed-forward models (Wolpert and Miall, 1996; Calvert et al., 2000; Shams and Seitz, 2008; Van Vugt, 2013). Moreover the real-time availability of feedback can serve as an external guidance for motor execution (Dyer J. F. et al., 2017) as well as an error feedback (Altenmüller et al., 2009; van Beers, 2009; Sigrist et al., 2015; van Vugt and Tillmann, 2015), and can enhance motor imagery (Sigrist et al., 2013), cognitive-emotional functioning (Eschrich et al., 2008; Sihvonen et al., 2017; see also Sigrist et al., 2013).
A strong influence of real-time auditory feedback on motor performance (Eriksson and Bresin, 2010; Schmitz et al., 2014; Scholz et al., 2015; Sigrist et al., 2015; Danna and Velay, 2017; Dyer J. F. et al., 2017), indicates a proportional influence of auditory domain over proprioception (Pantev et al., 2001; Scholz et al., 2015; Effenberg et al., 2016; Danna and Velay, 2017; Sihvonen et al., 2017), and it becomes effective as an integral component of motor control and coordination process (Proske, 2005; Ghai et al., 2017a). Scholz et al. (2015) mentioned that spatio-temporal associations generated by real-time kinematic auditory feedback during motor execution might allow substitution of proprioceptive deficits, possibly by closing the sensorimotor loop (Altenmüller et al., 2009; Särkämö et al., 2016; Scholz et al., 2016). Dyer J. et al. (2017) and van Vugt and Tillmann (2015) further added that the concurrent auditory feedback might supplement the low temporal-perceptual resolution of the proprioceptive domain (Tinazzi et al., 2002). Danna and Velay (2017) in their recent study proposed auditory-proprioceptive substitution for the enhancements the authors reported in handwriting performance for deafferented subjects receiving concurrent auditory feedback. These findings draw inferences from literature pertaining to cross-modal stimuli processing (Stein and Meredith, 1993; Calvert, 2001; Bavelier and Neville, 2002). For instance, sensory convergence from different sensory modalities have been reported to provoke cross-modal interactions (Macaluso et al., 2000; Macaluso and Driver, 2001). Furthermore, these claims are supported by neuroanatomical studies, reporting the presence of long range cortico-cortical connections in between sensory cortices (Falchier et al., 2001; Foxe, 2009; Keniston et al., 2010; Butler et al., 2012), and multisensory integration sites (Chabrol et al., 2015; for a detailed review see Calvert, 2001). This might suggest the possibility of a level of interdependency that the sensory modalities might share with each other to generate an integrated multimodal percept (Macaluso et al., 2000; Macaluso and Driver, 2001; Bavelier and Neville, 2002; Butler et al., 2012). In addition, several psychophysical studies have reported strong associations between the auditory and motor areas (Jokiniemi et al., 2008; Chen et al., 2009; Yau et al., 2009; Wilson et al., 2010b; Butler et al., 2012). These findings are further supplemented by the neuroimaging studies, reporting shorter pathways between the auditory and motor cortices, especially for multisensory integration (Lang et al., 1990; Zatorre et al., 2007; Foxe, 2009; Keniston et al., 2010; Butler et al., 2012; Chauvigné et al., 2014; Ishikawa et al., 2015). This might explain the strong influence of such audio-tactile cross-modal stimuli in terms of processing temporal (Fujisaki and Nishida, 2009), and certain impact on spatial information (Belardinelli et al., 2009; Jimenez and Jimenez, 2017; for a review see Lu et al., 2013). Nevertheless, despite the vast amount of literature indicating a strong influence of the audio-motor coupling for sensorimotor processing (Ghai et al., 2017c,d,e, 2018), a gap in literature persists concerning its applications in rehabilitation (Danna and Velay, 2017; Ghez et al., 2017), and/or sports (Ghai et al., 2017c).
As mentioned before, proprioception is an integral component of the coordination processes of the body (Gentilucci et al., 1994; Laskowski et al., 2000; Smith et al., 2012; Aman et al., 2014; Ghai et al., 2016, 2017a). Deficits in proprioceptive perception are directly linked with poor sensorimotor and somatosensory functioning (Aman et al., 2014; Ghai et al., 2016), characterized by a wide range of musculoskeletal and neuromuscular disorders (Sacco et al., 1987; Jensen et al., 2002; Ribeiro and Oliveira, 2007; Gay et al., 2010; Konczak et al., 2012; Ghai et al., 2017a). Its predominant role in rehabilitation has been emphasized in several studies (Lephart et al., 1997; Laskowski et al., 2000; Ribeiro and Oliveira, 2007; Rosenkranz et al., 2009; Gay et al., 2010; Aman et al., 2014). Therefore, exploring the possible influences of concurrent auditory feedback on proprioception might provide multifaceted benefits. First and foremost, the outcomes might provide a better understanding of intervention designs in rehabilitation, and sport settings with auditory feedback. Moreover, the evaluation of audio-proprioceptive coupling during an arbitrary action (knee-joint proprioception) might allow a better understanding of trans-modal activity of auditory and motor domains beyond music and language (Altenmüller et al., 2009). Finally, a better comprehensive understanding might be developed to support the psychophysical (Butler et al., 2012), neurophysiological (Ishikawa et al., 2015), studies analyzing the multisensory and cross modal integration between auditory and proprioceptive domains. Till this date, only a handful of researchers have attempted to answer the possible effects of real-time auditory feedback on proprioception (Van Vugt, 2013; Scholz et al., 2016; Danna and Velay, 2017; Dyer J. et al., 2017; Ghez et al., 2017). However, their interpretations on proprioceptive-auditory substitution are mostly speculative. For instance, none of the performed studies excluded vision during the performance of the motor task. As a result, possible influences from the visual modality during multisensory or cross modal integration processes can be expected (Plooy et al., 1998; Verschueren et al., 1998; Lönn et al., 2000). Research indicates the importance of isolating inputs from specific sensorimotor structures to provide a better understanding of direct influence over proprioception (Gay et al., 2010).
In a first attempt we tried to analyse the effects of real-time auditory feedback on clinical aspects of knee joint proprioception in a joint position sense test (Sherrington, 1907; Dover and Powers, 2003; Van Vugt, 2013). Based on interpretations drawn from state feedback control theory (Wolpert and Miall, 1996; Shadmehr and Krakauer, 2008), we expected real-time auditory feedback to cause enhancements in knee-joint proprioception or. Moreover, in a second step, we tried to analyze the effects of subliminal transposition of real-time auditory feedback's frequency on auditory-proprioceptive perceptions. The motivation of this part of study was derived from psychophysical studies revealing strong evidence of convergence between auditory and motor systems for computing frequency (Pantev et al., 2009; Wilson et al., 2009, 2010a), especially within well matched stimuli reflecting a similar event (Foxe, 2009). We expected that if auditory feedback could influence proprioception, understanding the role of frequency in this attained effect could allow a better understanding of the results. We therefore, evaluated influence of any divergent step-wise transposition of frequency with real-time auditory feedback would allow directed modulation of proprioceptive perceptions in terms of knee position.
In this article two experiments are mentioned. The second experiment is an extension of the first study, which was conducted after the analysis of results. The experiment II follows the same design and protocol but differs in terms of the magnitude and direction of step-wise transposition of the frequency of the feedback. se experiments differ based on magnitude and direction of step-wise transposition. We expect the outcomes from this study to provide novel practical implications in rehabilitation and sports settings.
This whole CCT was carried out between August 2016 and February 2017. Participants were randomly allocated to experimental or control group. In each group, participants carried out the active (knee-joint) repositioning task with their dominant legs. The experimental group concurrently received real-time and transposed (0.25°/repetition) auditory feedback while performing the active knee re-positioning tasks. The control group received white noise. The experiment consisted of five treatment blocks. Re-positioning tasks without any auditory feedback were performed on the odd numbered blocks. Auditory feedback (real-time, modulated, white noise) was provided in the even treatment blocks. The participants performed 15 repetitions per angle in a block i.e., 30 repetitions per block. The target angle for the repositioning task was 40 and 75°.
Thirty participants, randomly divided in control [8 males/7 females; mean ± SD (age): 23.5 ± 2.5 years], and experimental group I (7 male/8 female; 24.2 ± 3.7 years) volunteered to participate in the study. All participants self-reported as healthy with no history of significant hip, knee, or back injury. Written informed consent was obtained from each participant, and ethical approval was obtained from the Ethics Committee of the Leibniz University Hannover. All participants underwent a baseline test for auditory capabilities (HTTS Audiometry) and were asked to fill a self-reported questionnaire post the experiment. All participants received eight Euros for their participation.
Participants were comfortably seated with their feet on the floor, their back resting against a wall, and their pelvis stabilized (Tiggelen et al., 2008; Ghai et al., 2016). During the sitting position, the knee joint was maintained at the right angle. This position of the knee joint was considered as 0° and further extension from this position onwards was referred as positive angles from this value (Supplementary File 1). Participants wore wireless headphones (Sennheiser, Wedemark, Germany), and were blindfolded to eliminate visual cues. The experimenter passively moved the dominant leg to a previously identified target position (40 or 75°) in an open kinetic chain and held at the target angle to allow the participant to memorize the position (Selfe et al., 2006; Ghai et al., 2016). The experimenter, a physiotherapist, checked and rechecked the angle while using a handheld goniometer, and motion capture reading to confirm the target angle. The leg was then returned to the initial position, and following a 5 s interval, the participant attempted to reposition the leg at the same joint angle. The participant was instructed to repeatedly re-position the leg to the instructed angle with an instruction “please re-position your leg to the performed angle hold the angle for 2 s and then return it to the starting position.” The experimenter counted 15 repetitions and asked the participants to stop. This protocol was repeated for both the target angles (40 and 75°), across 5 treatment blocks. During the first, third, and fifth treatment blocks no auditory feedback was provided to the participants. However, during the second treatment block the same protocol was followed with real-time auditory feedback i.e., the experimenter initially took the dominant leg to the target angles with real-time auditory feedback. Thereafter, the participants performed the same target angles with real-time auditory feedback. During the fourth block, the experimenter initially positioned the dominant leg passively with real time auditory feedback, after which participants re-positioned their knee unaware of the modulation in frequency of auditory feedback (Supplementary File 2). Dynamic repositioning accuracy was computed to determine discrepancies while consecutively repositioning the knee joint. For instance, the repositioning performance of 40, 38, 43, 37°… the computation of repositioning error was performed by subtracting the performed angle with the previous angle i.e., 38°–40°, 43°–38°, 37°–43°…and so on. After the experiment was concluded, the participants were asked to fill a four-point questionnaire. The questionnaire enquired about the perceived duration of the experiment, the fatigue level, the excerptions perceived if any in the quality of the auditory feedback (for identifying whether participants were consciously able to detect changes in the frequency of the real-time auditory feedback), and subjective rating for compliance with auditory feedback on a 10-point Likert scale. The experimental protocol lasted approximately for 45 min.
Real-time auditory feedback was generated using Python (version 2.7) and Csound version 6.0. Sound synthesis was based on a band-limited oscillator bank with lowpass filtering. Knee joint angle and angular velocity are mapped onto pitch and amplitude of the auditory feedback, respectively. During sitting the right angle at the knee joint is regarded 0°, and any extension from this point onwards is referred in positive values from this angle. The changes in angles from 0 to 90° of full extension is configured from 120 to 300 Hz of frequency change, respectively. Here, amplitude is a function of square of knee angular velocity which is relevant to kinematic energy. For the amplitude function, exaggerated representation of the angular position was added because, as the frequency increases, human ear gets less sensitive in identifying the same pitch differences. The exaggeration in amplitude can therefore complement the lack of sensitivity, which properly stimulates the human ears. These mapping functions are also provided as a mathematical equation for clarity.
In the equations, Pit is pitch (audio frequency), θknee.joint is the knee joint angle, Amplitude is Amp, ωknee.joint is joint angular velocity. The equation also includes coefficients α, β as well as a constant value, k.
Modulation of real-time auditory feedback was subtle and provided in an under-transposition manner. Here, the mapping information between audio frequency and knee angle was manipulated during repetitions. For example, 15 repetitions in a step-down transposition by −0.25° (−0.5 Hz/rep) at the target angle. Frequency was changed per repetition, for instance from 180 to 193 Hz which would be is equivalent to a change of the knee angle from 40 to 36.5° in the constant original mapping (Supplementary Files 3, 6) for 15 repetitions. A sample for both the real-time auditory feedback (Supplementary File 5) and modulated auditory feedback (Supplementary File 6) have been provided.
Repositioning error (RE) was assessed in each trial using XSENS MVN Biomech (XSENS Technologies B.V, Netherlands), in a configuration mode limited to the lower body. High reliability and validity of this inertial sensor based motion analysis device has been previously reported (Cooper et al., 2009; Zhang et al., 2013). Seven pre-identified inertial measurement units (IMUs) were placed by a physiotherapist on sacrum, lateral side of femoral shaft, medial surface of tibia, and tarus using velcro straps (Supplementary File 1; Zhao et al., 2016). The angular repositioning data, expressed in sensor coordinate frame was wirelessly recorded with a sampling frequency of 60 Hz in a laptop (Lenovo INC, Hongkong) and saved in MVN file format. Thereafter, the saved file was converted to XML format (MVNX) and imported in a Microsoft Excel spreadsheet. This format incorporates information concerning sensor data, segment kinematics and joint angles. Marked data points (highlighted in MVN file during recording) were matched with MVN recording graphs and the data was manually extracted by two researchers for further calculations. Absolute and constant error were then computed for characterizing the repositioning error in both the magnitude and direction of error, by considering the target angle as the previous consecutive angle to the current performance by the participant.
Statistical analyses were performed using Statistical Package for Social Science (V. 23.0, SPSS Inc., Chicago, IL). In 2 separate analysis for absolute and constant errors. We analyzed Repositioning Error (the dependent measure), by conducting a Group (Experimental/control) × block (1–5) × Angles (40/75°) RM-ANOVA with repeated measures on the last two factors. Effect sizes of the independent variables were expressed using partial eta squared (ηp2), with effect sizes <0.01 considered to be small, effect sizes between 0.01 and 0.06 considered to be medium and effect sizes >0.14 considered to be large (Sedlmeier and Renkewitz, 2008). Post-hoc comparisons were performed using stepwise Bonferroni holm corrections. The overall significance level was set to 5%.
Figure 1 illustrates the absolute repositioning accuracy in both groups. The experimental group I, with real-time auditory feedback performed significantly better than the control group without auditory feedback as confirmed by the significant main effect of group [F(1, 28) = 6.92, p = 0.014, ηp2 = 0.20]. Furthermore, repositioning accuracy depended on block [F(4, 112) = 10.16, p < 0.001, ηp2 = 0.27]. Differences between block were mainly caused by the auditory feedback in the experimental group I as shown by the interaction block*group [F(4, 112) = 8.34, p < 0.001, ηp2 = 0.23]. A post-hoc test confirmed significant differences between the first and second block in the experimental group I (p < 0.001), but not in the control group (p > 0.999). Furthermore, the second (p < 0.001), but not the first (p > 0.999) block differed significantly between groups. After the removal of feedback this effect diminished. Accordingly, both groups performed in block 3 not significantly different than in block 1 (experimental group I: p > 0.999; control group: p > 0.999). Differences between angles were not significant [angles: F(1, 28) = 3.39, p = 0.076, ηp2 = 0.11; angle*group; F(1, 28) = 3.65, p = 0.066, ηp2 = 0.12; angle*block: F(4, 112) = 0.46, p = 0.714, ηp2 = 0.02; angle*block*group: F(4, 112) = 0.49, p = 0.690, ηp2 = 0.02].
Figure 1. Absolute mean and standard error of repositioning error (°) for the control, experimental group I (Dotted line represents control group. Darkened black line represents experimental group I, T: Proprioceptive test without auditory feedback, RT: Real-time auditory feedback, MAP: Acoustic mapping, CT: Control group, EXP: Experimental group). *Represents significant differences.
Figure 2 illustrates the constant repositioning error in both groups. The experimental group I with real-time auditory feedback performed significantly better than the control group without auditory feedback, as confirmed by the significant main effect of group [F(1, 28) = 6.150, p = 0.019, ηp2 = 0.18]. Furthermore, a main effect was observed for block [F(4, 112) = 4.320, p = 0.030, ηp2 = 0.13]. Differences between blocks were mainly caused by the auditory feedback in the experimental group I as shown by the interaction block*group [F(4, 112) = 4.560, p = 0.002, ηp2 = 0.140]. A post-hoc test confirmed significant differences between the first and second block in the experimental group I (p < 0.001), but not in the control group (p = 0.360). Furthermore, the second (p < 0.001), but not the first (p = 0.810) block differed significantly between groups. After the removal of feedback this effect diminished. Accordingly, both groups performed in block 3 not significantly different than in block 1 (experimental group I: p > 0.999; control group: p > 0.999).
Figure 2. Constant mean and standard error of repositioning error (°) for the control, experimental group I (Dotted line represents control group. Darkened black line represents experimental group I, T: Proprioceptive test without auditory feedback, RT: Real-time auditory feedback, MAP: Acoustic mapping, CT: Control group, EXP: Experimental group). *Represents significant differences.
In the 4th block, modulation in frequency of real-time feedback were introduced. We observed significant differences between the 3rd and 4th block of experimental group I (p = 0.001), and as compared to the 4th block control group (p < 0.001). No such differences were observed between 3rd and 4th block in control group (p = 0.660). Likewise, in 5th block both groups performed not significantly different than in 1st and 3rd block (all p's > 0.05). Significant differences were also not evident when the 4th block was compared with the 2nd block (p > 0.999) i.e., modulated feedback with un-modulated feedback. Constant error was significantly larger for angle 40° as compared to 75° [F(1, 28) = 21.80, p < 0.001, ηp2 = 0.44]. However, none of the interactions with the effects of the angles were significant, but not for angle*group; [F(1, 28) = 0.40, p = 0.532, ηp2 = 0.01]; angle*block [F(4, 112) = 0.36, p = 0.838, ηp2 = 0.01] angle*block*group [F(4, 112) = 0.20, p = 0.941, ηp2 = 0.01].
This whole trial was carried out between March 2017 and September 2017. Participants were allocated to experimental group II. Due to the identical experimental design as experiment I data from the same control group was utilized for comparison and the data from control group of first experiment was utilized. Here, the participants carried out the active (knee-joint) repositioning task with their dominant legs. The experimental group concurrently received real-time, modulated (±1.3°/repetition) auditory feedback while performing the re-positioning tasks. The control group received white noise. The experiment consisted of five treatment blocks. Re-positioning tasks without any auditory feedback were performed on the odd numbered blocks. Auditory feedback (real-time, modulated, white noise) was provided in the even treatment blocks. The participants performed 15 repetitions per angle in a block i.e., 30 repetitions per block. The target angle for the repositioning task was 40 and 75°.
Twenty healthy participants were included in experimental group II [10 females/10 males; mean ± SD (age): 26.8 ± 3.5 years]. All participants underwent a baseline test for auditory capabilities (HTTS Audiometry). All participants received eight Euros for their participation.
Same as experiment I.
Real-time auditory feedback was generated using Python (version 2.7) and Csound version 6.0. Sound synthesis was based on a band-limited oscillator bank with lowpass filtering. Knee joint angle and angular velocity are mapped onto pitch and amplitude of the auditory feedback, respectively. During sitting the right angle at the knee joint is regarded 0°, and any extension from this point onwards is referred in positive values from this angle. The changes in angles from 0 to 90° of full extension is configured from 120 to 300 Hz of frequency change, respectively. Here, amplitude is a function of square of knee angular velocity which is relevant to kinematic energy.
The modulation of real-time auditory feedback was subtle and provided in an over/under-transposition manner. Here as well, the frequency of the auditory feedback was manipulated per repetition, for 15 repetitions. However, the gradient of change was larger i.e., ±2.6 Hz (equivalent to ±1.3° change). Here during step down-up the change in frequency was equivalent as a change from 180 Hz (40°) to 167 Hz (34.8°) in the 5th repetition, and then to 182.6 Hz (41.7°) for the 10th repetition, and finally to 167 Hz (34.8°) for the 15th repetition. For instance, in step up-down manner 15 repetitions were accounted in three continuous steps: first five repetitions i.e., 1–5 transposition were performed in step-up manner i.e., 40, 41.3, 42.6, 43.9, 45.2°. Thereafter, for repetitions 6–10 continuously the direction of transposition was changed in step-down manner i.e., 43.9, 42.6, 41.3, 40, 38.7°. Lastly, for the final 11–15 repetitions the transposition was again changed to step-up manner i.e., 40, 41.3, 42.6, 43.9, 45.2°. This transposition change was randomized with step down-up approach during the study. For better clarity see Supplementary Files 4, 7.
The application of transposition was counterbalanced across four sub-groups i.e., sub-group I (40°: under-over-under, 75°: over-under-over), sub-group II (40°: over-under-over, 75°: over-under-over), sub-group III (40°: over-under-over, 75°: under-over-under), and sub-group IV (40°: under-over-under, 75°: under-over-under). Therefore, the number of participants was balanced across the conditions and increased to 20 i.e., 5 in each sub-group. A sample for both the real-time and modulated auditory feedback (Supplementary Files 6, 7) have been provided.
Same as experiment I.
Like experiment I, in 2 separate analysis absolute and constant errors were compared with control group. Here, the control group from experiment I was utilized. We analyzed Repositioning Error (the dependent measure), by conducting a Group (Experimental/control) × blocks (1-5) × Angles (40/75°) RM-ANOVA with repeated measures on the last two factors. Additionally, data were decomposed for the 4th block, where the frequency was modulated, across four different sub-groups. Here, the data were normalized on an individual level to the real-time non-modulated auditory feedback by subtraction. The four sub-groups differed in performance of episodes of transposition i.e., sub-group I (40°: under-over-under, 75°: over-under-over), sub-group II (40°: over-under-over, 75°: over-under-over), sub-group III (40°: over-under-over, 75°: under-over-under), and sub-group IV (40°: under-over-under, 75°: under-over-under). Here, each episode represented the mean of five subsequent movements. For the analysis the values for the over-transposition were inverted. Here, analysis of variance was performed on normalized repositioning errors as dependent variable and between subject factor sub-groups (I, II, III, IV) and within subject factor episodes (1–3) and angles (40/75°). Here, each episode represented the mean of five subsequent movements. Post-hoc comparisons were performed using step wise Bonferroni holm corrections.
Figure 3 illustrates the absolute repositioning error in both groups. Significant differences were observed in between blocks [F(4, 132) = 38.3, p < 0.001, ηp2 = 0.54] and interaction was evident for block*group [F(4, 132) = 4.4, p < 0.01, ηp2 = 0.12]. A post-hoc test confirmed significant differences between the first and second block in the experimental group I (p < 0.001), but not in the control group (p = 0.940). Furthermore, the second (p < 0.001), but not the first (p = 0.30) block differed significantly between groups. After the removal of feedback this effect diminished. Accordingly, both groups performed in block 3 not significantly different than in block 1 (experimental group I: p > 0.999; control group: p > 0.999). None of the other results were significant group [F(1, 33) = 2.0, p = 0.15, ηp2 = 0.06], angles [F(1, 33) > 0.01, p = 0.970, ηp2 < 0.001], angle*group [F(1, 33) = 0.01, p = 0.920, ηp2 < 0.001], angle*block [F(4, 132) = 0.3, p = 0.780, ηp2 = 0.01], angle*block*group [F(4, 132) = 0.77, p = 0.490, ηp2 = 0.02].
Figure 3. Absolute mean and standard error of repositioning error (°) for the control and experimental group II (Dotted line represents control group. Darkened black line represents experimental group II, T: Proprioceptive test without auditory feedback, RT: Real-time auditory feedback, MAP: Acoustic mapping, CT: Control group, EXP: Experimental group). *Represents significant differences.
Figure 4 illustrates the constant repositioning accuracy in both groups. The repositioning accuracy depended on block [F(4, 132) = 14.2, p < 0.001, ηp2 = 0.3]. Differences between conditions were mainly caused by the auditory feedback in the experimental group I as shown by the interaction block*group [F(4, 112) = 4.56, p = 0.002, ηp2 = 0.14]. A post-hoc test confirmed significant differences between the first and second block in the experimental group I (p = 0.003), but not in the control group (p = 0.730). Furthermore, the second (p = 0.001), but not the first (p > 0.999) block differed significantly between groups. After the removal of feedback this effect diminished. Accordingly, both groups performed in block 3 not significantly different than in block 1 (experimental group I: p > 0.999; control group: p > 0.999). In the fourth block, subliminal modulation in frequency of real-time feedback were introduced. We observed no significant differences in the 4th block of experimental group II (p = 0.220), control group (p = 0.770) as compared to the 3rd block. This difference was however, significant when compared to the control group (p = 0.010). Likewise, both groups performance in 5th block did not significantly different than in block 1, and 3 (experimental group II: p > 0.999; control group: p > 0.999). Significant differences were not evident when modulated feedback in 4th block was compared with un-modulated feedback in the 2nd block (p > 0.999). Differences were significant in between the angles [F(1, 33) = 19.6, p < 0.01, ηp2 = 0.37] i.e., constant errors were larger for 40° as compared to 75° and for angle*group; [F(1, 33) = 14.5, p = 0.001, ηp2 = 0.31], but not for group [F(1, 33) < 0.01, p = 0.990, ηp2 < 0.01], angle*block [F(4, 132) = 0.6, p = 0.650, ηp2 = 0.02], angle*block*group [F(4, 132) = 0.89, p = 0.470, ηp2 = 0.03].
Figure 4. Constant mean and standard error of repositioning error (°) for the control, and experimental group II. (Dotted line represents control group. Darkened black line represents experimental group I, gray line represents experimental group II, T: Proprioceptive test without auditory feedback, RT: Real-time auditory feedback MAP: Acoustic mapping step-down 0.25/rep for exp I, 1.3/rep for exp II, CT: Control group, EXP: Experimental group). *Represents significant differences.
For specifying the effect of transposition, we decomposed the data from the 4th block. We computed constant errors separately for every five repetitions with transposition in the same directions. Each episode began with either over-under-over or under-over-under transposition. Figure 5 shows the constant errors separately for participants with different episodes. Here, four sub-groups were distinguished with five participants each i.e., sub-group I performed for (40°: under-over-under, 75°: over-under-over), sub-group II (40°: over-under-over, 75°: over-under-over), sub-group III (40°: over-under-over, 75°: under-over-under), and sub-group IV (40°: under-over-under, 75°: under-over-under). Figure 5 indicates that the re-positioning performance tended to compensate in the opposite direction in which the auditory feedback was manipulatively directed i.e., the participants knee flexion when the feedback was over transposed and vice versa for the under transposition. For the analysis, the over transposition repositioning errors were multiplied with −1.
Figure 5. Constant mean and standard error of repositioning error (°) for the experimental II, 2nd and 4th block, also for episodes (1–3). Difference in proprioceptive perceptions in between decomposed mapping conditions have been described for 5 sub-groups i.e., RT: real-time auditory feedback, sub-group I (G I: 40°: under-over-under, 75°: over-under-over), sub-group II (G II: 40°: over-under-over, 75°: over-under-over), sub-group III (G III:40°: over-under-over, 75°: under-over-under), and sub-group IV (G IV: 40°: under-over-under, 75°: under-over-under), and across 3 treatment blocks. The values on left represent 40°, and right 75° (RT: Real-time kinematic auditory feedback). *Represents significant differences.
The data were normalized for the analysis according to individual real-time auditory feedback performance of each participants. Further, step-up transposition findings were multiplied with −1 to allow the direction of transposition to be similar for all episodes (1–3). The statistical analysis revealed that episodes had no significant effect [Episode: F(3.16) = 1.51, p = 0.414, ηp2 = 0.16; angle*episode: F(3.16) = 0.72, p = 0.556, ηp2 = 0.12; block*episode: F(6.32) = 1.43, p = 0.233, ηp2 = 0.22; angle*episode*group: F(6.32) = 1.04, p = 0.420, ηp2 = 0.16] indicating that over- and under-transpositions did not differ in their impact. However, the transpositions were more effective in the second compared to the first episode (p = 0.002) as confirmed by post-hoc comparisons to the main effect of episode [F(2, 32) = 7.39, p = 0.002, ηp2 = 0.32]. Differences between the first and the third (p = 0.267) or the second and the third episode (p = 0.090) were not significant.
To scrutinize whether the altered mapping between auditory feedback and angle changed the repositioning error we performed t-tests against zero separately for episodes (1–3). The results confirmed significant differences to zero in episode 2 (p < 0.001) and episode 3 (p = 0.029) but not block 1 (p = 0.208).
Results from the current experiment demonstrate beneficial effects of real-time auditory feedback on knee re-positioning accuracy. Significant enhancement in re-positioning accuracy was observed for both absolute (p < 0.001) and constant error (p < 0.01) and both within and across the experimental I and II (For clarity see Figures 1–4), with real-time auditory feedback. These findings agree with previous literature indicating strong associations between the auditory and motor domains (Foxe, 2009; Butler et al., 2012; Schmitz et al., 2013; Ishikawa et al., 2015), and support the possibility of the auditory-proprioceptive substitution hypothesis raised by Altenmüller et al. (2009), Danna and Velay (2017), and Scholz et al. (2015). In this experiment, the enhancement in re-positioning accuracy with real-time auditory feedback could possibly be associated with the “guidance hypothesis” (Schmidt, 1991; Park et al., 2000). The auditory feedback could have made it easier for the participant to identify the target angles, reduce errors, and re-produce the instructed target angles more precisely. This enhancement in re-producibility of target angles could also be due to high spatio-temporal precision of combined audio-motor domains (Hancock et al., 2013; van Vugt and Tillmann, 2015; Dyer J. et al., 2017), which also might have lowered the somatosensory mismatch negativity (Butler et al., 2012). These changes were also affirmed by Fujioka et al. (2012a). The authors reported modulations in the functional reorganization of spatio-temporal patterns of neuromagnetic β activity (between auditory and sensorimotor modalities; Fujioka et al., 2012a,b). Moreover, the enhanced activation in multisensory integration sites (such as neocortex, superior colliculi, striatum, and cerebellum) and action observation system (Superior temporal sulcus, BA 44, 45) might have aided in enhancing the saliency of executed movement patterns (Schmitz et al., 2013; Stein et al., 2014; Chabrol et al., 2015).
These enhancements in re-positioning accuracy however, were not as stable. Once the auditory feedback was removed in the third treatment block, the re-positioning errors returned to their initial levels. This lack of retention in re-positioning accuracy might be linked with over dependency of the participants with the concurrent feedback (Schmidt, 1991). Park et al. (2000) reported that the concurrent feedback can make the learners dependent on the feedback for maintaining their performances, possibly by bypassing the important internal correction and/or error detecting mechanisms (Schmidt, 1991). Moreover, the concurrent feedback might also limit a performer's initial movement error's (Winstein and Schmidt, 1990), which are thought to represent internal variability of the motor system and are considered as essential for the learning process (see dynamic system theory; Clark and Phillips, 1993). Similarly, the rapid change in knee re-positioning accuracy with substitution of auditory feedback could be affirmed with changes in attentional resources. Recently, Ghai et al. (2016) demonstrated that proprioception is adversely impacted under the influence of higher information processing constrains. However, Hopkins et al. (2017) suggested that cross modal cueing can avoid information overload in the native sensory modality by directing task-irrelevant information toward the underused sensory modality (Hameed et al., 2009). Here as well, the introduction of auditory feedback could have possibly allowed enhancements in re-positioning accuracy by transferring excess information in the sister domain (Lohnes and Earhart, 2011; Ghai et al., 2017b).
Furthermore, we analyzed modulations in knee repositioning performance with modulations in frequency of the auditory feedback. We confirmed with a self-reported questionnaire that participants were not able to consciously perceive any differences introduced in the frequency of the auditory feedback in both group I and II. However, our results demonstrate that these modulations were dependent on the magnitude of modulation introduced in the frequency. In experiment group I, the step-wise modulations were produced in a step-down transposition by 0.5 Hz/repetition (0.25° or 0.2%/rep). Although a trend toward step-wise modulation was observed for some individual participants, possibly due to their different inherent auditory perceptual capabilities (Kagerer et al., 2014), these differences could not be proven statistically (p > 0.05), when compared with real-time auditory feedback condition. Thereafter, upon deliberate examination in multiple pilot trials, a step-wise modulation by 2.6 Hz/repetition (1.3° or 1.1%/rep) was identified and included. The step-wise modulation was performed in three steps, across both the directions i.e., under, over, under transposition across 15 repetitions and vice versa. The direction was changed after five repetitions to avoid conscious perceptions i.e., five repetitions accounted for 6.5° change in one direction, and 19.5° overall change 15 repetitions. On the contrary, in experiment I only 3.5° change was evitable across 15 repetitions. During the initial analysis, no significant differences in knee repositioning accuracy were observed, possibly due to the negation of directional errors in perceptions across the blocks by step-up/down transposition. Therefore, upon factorial re-analysis of decomposed data for directional changes for knee repositioning, we observed significant effect of modulated auditory feedback as compared to real-time auditory feedback. The participants tried to compensate their knee re-positioning by tending to either extend or flex their knee's more with step-down and step-up transposition in frequency (Figure 5), respectively. In our analysis we observed a significant effect of transposition as compared to real-time auditory feedback and demonstrated a combined effect of the transposition to manipulate knee repositioning. As demonstrated in Figure 6, the participants could have taken time to adjust their re-positioning according to the dynamically transposed auditory feedback, or the significance in the next two episodes might be due to practice effect. Previously, published literature has demonstrated the effectiveness of audio-motor coupling due to subliminal changes in rhythmic auditory feedback (Repp, 2000, 2001; Tecchio et al., 2000; Kagerer et al., 2014). These findings also build up on psychophysical studies demonstrating the cross-sensory impacts of frequency modulation between auditory and motor domains (Foxe, 2009; Butler et al., 2012). We demonstrate that subliminal modulation of frequency can lead to goal-directed changes in knee repositioning. To the best of our knowledge, this study for the first time demonstrates modulation in knee repositioning due to subliminal changes in frequency of real-time auditory feedback. Previously, published literature has only demonstrated this association of audio-motor coupling with subliminal changes in inter stimulus interval for rhythmic auditory feedback (Repp, 2000, 2001; Tecchio et al., 2000; Kagerer et al., 2014).
Figure 6. Constant mean and standard error of the repositioning error (°) for the experimental II, 4th block, the values of transposition are normalized, and step-up transpositions have been multiplied with −1 to allow the direction of transposition to be similar for all three blocks. Mean values across the 2 angles for episodes (1–3). *Represents significant differences.
Finally, building upon the strong correlation suggested for proprioceptive, re-positioning tasks (Vidoni and Boyd, 2009; Van Vugt, 2013), and similar open kinetic chain training regimes in rehabilitation (Tagesson et al., 2008; Fukuda et al., 2013; see review Glass et al., 2010), we believe enhancements observed in this experiment can have a range of practical implications in both rehabilitation and sports settings. Fukuda et al. (2013), for instance reported considerable enhancement in quadriceps, hamstrings strength recovery in patients with ACL reconstruction while performing similar non-weight bearing open kinetic chain movements at the knee joint. Moreover, changes in movement patterns associated with subliminal changes in frequency can also have practical implications. For instance, enhancement in breathing (Murgia et al., 2016), music learning (Hol, 2011; Lahav et al., 2013), arm reaching (Maulucci and Eckhouse, 2001; Schmitz et al., 2014; Scholz et al., 2016), gait (Maulucci and Eckhouse, 2011; Zhang et al., 2013; Mezzarobba et al., 2017), sports (Eriksson and Bresin, 2010; Sigrist et al., 2013), performance with real-time auditory feedback has been demonstrated in a few studies. Here, subliminal modulation in frequency during training can be introduced to enhance variability in movement patterns, which further can lead to a dynamic learning pattern (Stein et al., 2014). Moreover, introduction of subliminal changes can be used to prompt the patient or sports person to exceed their performance parameters without consciously perceiving them i.e., possibly reducing movement re-investment (see Masters and Maxwell, 2008). Future studies can evaluate these aspects of modulation in training paradigms in both sports and rehabilitation settings. Finally, the subjective rating of the compliance of auditory feedback in the experiment revealed higher rating for the auditory feedback (6.1 ± 1.0) as compared to the control condition (3.5 ± 1.5). A higher compliance with auditory feedback in past has been associated with enhanced motivation, attention and arousal (Menon and Levitin, 2005; Cha et al., 2014). Thereby, possibly supporting the applications of such type of concurrent auditory feedback in rehabilitation settings.
AE, GS, and SG developed the research question; SG, AE, and GS developed the research paradigm; SG conducted the experiment, collected the data, and wrote main parts of the paper; GS performed the statistical analysis supported by AE; SG contributed to the results section; T-HH was responsible for technical implementing and customization of the sonification system; AE supervised the project. All authors critically revised the paper.
The work was supported by EC H2020-FETPROACT-2014 No. 641321.
The authors declare that the research was conducted in the absence of any commercial or financial relationships that could be construed as a potential conflict of interest.
The publication of this article was funded by the Open Access funds of Leibniz Universität Hannover. The co-author would like to thank student assistant Mr. Pascal Moszczynski, Christian Speckelmeyer for their assistance during the experimental procedure.
The Supplementary Material for this article can be found online at: https://www.frontiersin.org/articles/10.3389/fnins.2018.00142/full#supplementary-material
Altenmüller, E., Marco-Pallares, J., Münte, T. F., and Schneider, S. (2009). Neural reorganization underlies improvement in stroke-induced motor dysfunction by music-supported therapy. Ann. N.Y. Acad. Sci. 1169, 395–405. doi: 10.1111/j.1749-6632.2009.04580.x
Aman, J. E., Elangovan, N., Yeh, I. L., and Konczak, J. (2014). The effectiveness of proprioceptive training for improving motor function: a systematic review. Front. Hum. Neurosci. 8:1075. doi: 10.3389/fnhum.2014.01075
Bavelier, D., and Neville, H. J. (2002). Cross-modal plasticity: where and how? Nat. Rev. Neurosci. 3, 443–452. doi: 10.1038/nrn848
Belardinelli, M. O., Federici, S., Delogu, F., and Palmiero, M. (2009). “Sonification of spatial information: audio-tactile exploration strategies by normal and blind subjects,” in International Conference on Universal Access in Human-Computer Interaction (Berlin; Heidelberg: Springer), 557–563.
Butler, J. S., Foxe, J. J., Fiebelkorn, I. C., Mercier, M. R., and Molholm, S. (2012). Multisensory representation of frequency across audition and touch: high density electrical mapping reveals early sensory-perceptual coupling. J. Neurosci. 32, 15338–15344. doi: 10.1523/JNEUROSCI.1796-12.2012
Calvert, G. A. (2001). Crossmodal processing in the human brain: insights from functional neuroimaging studies. Cereb. Cortex 11, 1110–1123. doi: 10.1093/cercor/11.12.1110
Calvert, G. A., Campbell, R., and Brammer, M. J. (2000). Evidence from functional magnetic resonance imaging of crossmodal binding in the human heteromodal cortex. Curr. Biol. 10, 649–657. doi: 10.1016/S0960-9822(00)00513-3
Cha, Y., Kim, Y., Hwang, S., and Chung, Y. (2014). Intensive gait training with rhythmic auditory stimulation in individuals with chronic hemiparetic stroke: a pilot randomized controlled study. NeuroRehabilitation 35, 681–688. doi: 10.3233/NRE-141182
Chabrol, F. P., Arenz, A., Wiechert, M. T., Margrie, T. W., and DiGregorio, D. A. (2015). Synaptic diversity enables temporal coding of coincident multisensory inputs in single neurons. Nat. Neurosci. 18, 718–727. doi: 10.1038/nn.3974
Chauvigné, L. A., Gitau, K. M., and Brown, S. (2014). The neural basis of audiomotor entrainment: an ALE meta-analysis. Front. Hum. Neurosci. 8:776. doi: 10.3389/fnhum.2014.00776
Chen, J. L., Penhune, V. B., and Zatorre, R. J. (2009). The role of auditory and premotor cortex in sensorimotor transformations. Ann. N.Y. Acad. Sci. 1169, 15–34. doi: 10.1111/j.1749-6632.2009.04556.x
Clark, J. E., and Phillips, S. J. (1993). A longitudinal study of intralimb coordination in the first year of independent walking: a dynamical systems analysis. Child Dev. 64, 1143–1157. doi: 10.2307/1131331
Cooper, G., Sheret, I., McMillian, L., Siliverdis, K., Sha, N., Hodgins, D., et al. (2009). Inertial sensor-based knee flexion/extension angle estimation. J. Biomech. 42, 2678–2685. doi: 10.1016/j.jbiomech.2009.08.004
Danna, J., and Velay, J. L. (2017). On the auditory-proprioception substitution hypothesis: movement sonification in two deafferented subjects learning to write new characters. Front. Neurosci. 11:137. doi: 10.3389/fnins.2017.00137
Dover, G., and Powers, M. E. (2003). Reliability of joint position sense and force-reproduction measures during internal and external rotation of the shoulder. J. Athlet. Train. 38, 304–310.
Dyer, J., Stapleton, P., and Rodger, M. (2017). Transposing musical skill: sonification of movement as concurrent augmented feedback enhances learning in a bimanual task. Psychol. Res. 81, 850–862. doi: 10.1007/s00426-016-0775-0
Dyer, J. F., Stapleton, P., and Rodger, M. W. M. (2017). Advantages of melodic over rhythmic movement sonification in bimanual motor skill learning. Exp. Brain Res. 235, 3129–3140. doi: 10.1007/s00221-017-5047-8
Effenberg, A. O. (2005). Movement sonification: effects on perception and action. IEEE Multimedia 12, 53–59. doi: 10.1109/MMUL.2005.31
Effenberg, A. O. (2014). “Sensory systems: auditory, tactile, proprioceptive,” in Encyclopedia of Sport and Exercise Psychology, eds R. C. Eklund and G. Tenenbaum (Los Angeles, CA: SAGE Publications), 663–667.
Effenberg, A. O., Fehse, U., Schmitz, G., Krueger, B., and Mechling, H. (2016). Movement sonification: effects on motor learning beyond rhythmic adjustments. Front. Neurosci. 10:219. doi: 10.3389/fnins.2016.00219
Effenberg, A. O., Schmitz, G., Baumann, F., Rosenhahn, B., and Kroeger, D. (2015). SoundScript-Supporting the acquisition of character writing by multisensory integration. Open Psychol. J. 8, 230–237. doi: 10.2174/1874350101508010230
Eriksson, M., and Bresin, R. (2010). “Improving running mechanics by use of interactive sonification,” in Proceedings of ISon (Stockholm), 95–98.
Eschrich, S., Munte, T. F., and Altenmuller, E. O. (2008). Unforgettable film music: the role of emotion in episodic long-term memory for music. BMC Neurosci. 9:48. doi: 10.1186/1471-2202-9-48
Falchier, A., Renaud, L., Barone, P., and Kennedy, H. (2001). Extensive projections from the primary auditory cortex and polysensory area STP to peripheral area V1 in the macaque. Abstr. Soc. Neurosci. 27.
Foxe, J. J. (2009). Multisensory integration: frequency tuning of audio-tactile integration. Curr. Biol. 19, R373–R375. doi: 10.1016/j.cub.2009.03.029
Fujioka, T., Trainor, L. J., Large, E. W., and Ross, B. (2012a). Internalized timing of isochronous sounds is represented in neuromagnetic beta oscillations. J. Neurosci. 32, 1791–1802. doi: 10.1523/JNEUROSCI.4107-11.2012
Fujioka, T., Ween, J. E., Jamali, S., Stuss, D. T., and Ross, B. (2012b). Changes in neuromagnetic beta-band oscillation after music-supported stroke rehabilitation. Ann. N.Y. Acad. Sci. 1252, 294–304. doi: 10.1111/j.1749-6632.2011.06436.x
Fujisaki, W., and Nishida, S. (2009). Audio–tactile superiority over visuo–tactile and audio–visual combinations in the temporal resolution of synchrony perception. Exp. Brain Res. 198, 245–259. doi: 10.1007/s00221-009-1870-x
Fukuda, T. Y., Fingerhut, D., Moreira, V. C., Camarini, P. M., Scodeller, N. F., Duarte, A. Jr., et al. (2013). Open kinetic chain exercises in a restricted range of motion after anterior cruciate ligament reconstruction: a randomized controlled clinical trial. Am. J. Sports Med. 41, 788–794. doi: 10.1177/0363546513476482
Gay, A., Harbst, K., Kaufman, K. R., Hansen, D. K., Laskowski, E. R., and Berger, R. A. (2010). New method of measuring wrist joint position sense avoiding cutaneous and visual inputs. J. Neuroeng. Rehabil. 7:5. doi: 10.1186/1743-0003-7-5
Gentilucci, M., Toni, I., Chieffi, S., and Pavesi, G. (1994). The role of proprioception in the control of prehension movements: a kinematic study in a peripherally deafferented patient and in normal subjects. Exp. Brain Res. 99, 483–500. doi: 10.1007/BF00228985
Ghai, S., Driller, M., and Ghai, I. (2017a). Effects of joint stabilizers on proprioception and stability: a systematic review and meta-analysis. Phys. Ther. Sport 25, 65–75. doi: 10.1016/j.ptsp.2016.05.006
Ghai, S., Driller, M. W., and Masters, R. S. (2016). The influence of below-knee compression garments on knee-joint proprioception. Gait Posture 60, 258–261. doi: 10.1016/j.gaitpost.2016.08.008
Ghai, S., Ghai, I., and Effenberg, A. (2017b). Effects of dual-task training and dual-tasks on postural stability: a systematic review and meta-analysis. Clin. Interv. Aging 12, 557–577. doi: 10.2147/CIA.S125201
Ghai, S., Ghai, I., and Effenberg, A. O. (2017c). Effect of rhythmic auditory cueing on aging gait: a systematic review and meta-analysis. Aging Dis. 131–200.
Ghai, S., Ghai, I., and Effenberg, A. O. (2017d). Effect of rhythmic auditory cueing on gait in cerebral palsy: a systematic review and meta-analysis. Neuropsychiatr. Dis. Treat. 14, 43–59. doi: 10.2147/NDT.S148053
Ghai, S., Ghai, I., Schmitz, G., and Effenberg, A. O. (2017e). Effect of rhythmic auditory cueing on Parkinsonian gait: A systematic review and meta-analysis. Sci. Rep. 8:506. doi: 10.1038/s41598-017-16232-5
Ghai, S., Ghai, I., and Effenberg, A. O. (2018). “Low road” to rehabilitation: a perspective on subliminal sensory neuroprosthetics. Neuropsychiatr. Dis. Treat. 14:301. doi: 10.2147/NDT.S153392
Ghez, C., Dubois, R. L., Rikakis, T., and Cook, P. R. (2017). An Auditory Display System for Aiding Interjoint Coordination. Georgia Institute of Technology, 2000. Available online at: http://hdl.handle.net/1853/50663
Glass, R., Waddell, J., and Hoogenboom, B. (2010). The effects of open versus closed kinetic chain exercises on patients with ACL deficient or reconstructed knees: a systematic review. North Am. J. Sports Phys. Ther. 5, 74–84.
Hameed, S., Ferris, T., Jayaraman, S., and Sarter, N. (2009). Using informative peripheral visual and tactile cues to support task and interruption management. Hum. Factors 51, 126–135. doi: 10.1177/0018720809336434
Hancock, P. A., Mercado, J. E., Merlo, J., and Van Erp, J. B. (2013). Improving target detection in visual search through the augmenting multi-sensory cues. Ergonomics 56, 729–738. doi: 10.1080/00140139.2013.771219
Hol, J. D. (2011). Sensor Fusion and Calibration of Inertial Sensors, Vision, Ultra-Wideband and GPS. Linköping: Linköping University Electronic Press.
Hopkins, K., Kass, S. J., Blalock, L. D., and Brill, J. C. (2017). Effectiveness of auditory and tactile crossmodal cues in a dual-task visual and auditory scenario. Ergonomics 60, 692–700. doi: 10.1080/00140139.2016.1198495
Ishikawa, T., Shimuta, M., and Häusser, M. (2015). Multimodal sensory integration in single cerebellar granule cells in vivo. Elife 4:e12916. doi: 10.7554/eLife.12916
Jensen, T. O., Fischer-Rasmussen, T., Kjaer, M., and Magnusson, S. P. (2002). Proprioception in poor- and well-functioning anterior cruciate ligament deficient patients. J. Rehabil. Med. 34, 141–149. doi: 10.1080/165019702753714174
Jimenez, R., and Jimenez, A. M. (2017). “Blind waypoint navigation using a computer controlled vibrotactile belt,” in Advances in Human Factors and System Interactions: Proceedings of the AHFE 2016 International Conference on Human Factors and System Interactions July 27-31, 2016, Walt Disney World®, Florida, USA, ed I. L. Nunes (Cham: Springer International Publishing), 3–13.
Jokiniemi, M., Raisamo, R., Lylykangas, J., and Surakka, V. (2008). “Crossmodal rhythm perception,” in Haptic and Audio Interaction Design: Third International Workshop, HAID Jyväskylä, Finland, September 15-16, 2008 Proceedings, eds A. Pirhonen and S. Brewster (Berlin; Heidelberg: Springer), 111–119.
Kagerer, F. A., Viswanathan, P., Contreras-Vidal, J. L., and Whitall, J. (2014). Auditory-motor integration of subliminal phase shifts in tapping: better than auditory discrimination would predict. Exp. Brain Res. 232, 1207–1218. doi: 10.1007/s00221-014-3837-9
Keniston, L. P., Henderson, S. C., and Meredith, M. A. (2010). Neuroanatomical identification of crossmodal auditory inputs to interneurons in somatosensory cortex. Experimental brain research. Experimentelle Hirnforschung. Exp. Cerebr. 202, 725–731. doi: 10.1007/s00221-010-2163-0
Konczak, J., Sciutti, A., Avanzino, L., Squeri, V., Gori, M., Masia, L., et al. (2012). Parkinson's disease accelerates age-related decline in haptic perception by altering somatosensory integration. Brain 135, 3371–3379. doi: 10.1093/brain/aws265
Lahav, A., Katz, T., Chess, R., and Saltzman, E. (2013). Improved motor sequence retention by motionless listening. Psychol. Res. 77, 310–319. doi: 10.1007/s00426-012-0433-0
Lang, W., Obrig, H., Lindinger, G., Cheyne, D., and Deecke, L. (1990). Supplementary motor area activation while tapping bimanually different rhythms in musicians. Exp. Brain Res. 79, 504–514. doi: 10.1007/BF00229320
Laskowski, E. R., Newcomer-Aney, K., and Smith, J. (2000). Proprioception. Phys. Med. Rehabil. Clin. N. Am. 11, 323–340.
Lephart, S. M., Pincivero, D. M., Giraldo, J. L., and Fu, F. H. (1997). The role of proprioception in the management and rehabilitation of athletic injuries. Am. J. Sports Med. 25, 130–137. doi: 10.1177/036354659702500126
Lohnes, C. A., and Earhart, G. M. (2011). The impact of attentional, auditory, and combined cues on walking during single and cognitive dual tasks in Parkinson disease. Gait Posture 33, 478–483. doi: 10.1016/j.gaitpost.2010.12.029
Lönn, J., Crenshaw, A. G., Djupsjöbacka, M., Pedersen, J., and Johansson, H. (2000). Position sense testing: influence of starting position and type of displacement. Arch. Phys. Med. Rehabil. 81, 592–597. doi: 10.1016/S0003-9993(00)90040-6
Lu, S. A., Wickens, C. D., Prinet, J. C., Hutchins, S. D., Sarter, N., and Sebok, A. (2013). Supporting interruption management and multimodal interface design: three meta-analyses of task performance as a function of interrupting task modality. Hum. Factors 55, 697–724. doi: 10.1177/0018720813476298
Macaluso, E., and Driver, J. (2001). Spatial attention and crossmodal interactions between vision and touch. Neuropsychologia 39, 1304–1316. doi: 10.1016/S0028-3932(01)00119-1
Macaluso, E., Frith, C. D., and Driver, J. (2000). Modulation of human visual cortex by crossmodal spatial attention. Science 289, 1206–1208. doi: 10.1126/science.289.5482.1206
Masters, R. S. W., and Maxwell, J. (2008). The theory of reinvestment. Int. Rev. Sport Exerc. Psychol. 1, 160–183. doi: 10.1080/17509840802287218
Maulucci, R. A., and Eckhouse, R. H. (2001). Retraining reaching in chronic stroke with real-time auditory feedback. NeuroRehabilitation 16, 171–182.
Maulucci, R. A., and Eckhouse, R. H. (2011). “A real-time auditory feedback system for retraining gait,” in Annual International Conference of the IEEE Engineering in Medicine and Biology Society, IEEE Engineering in Medicine and Biology Society, 5199–5202. doi: 10.1109/IEMBS.2011.6091286
Menon, V., and Levitin, D. J. (2005). The rewards of music listening: response and physiological connectivity of the mesolimbic system. Neuroimage 28, 175–184. doi: 10.1016/j.neuroimage.2005.05.053
Mezzarobba, S., Grassi, M., Pellegrini, L., Catalan, M., Kruger, B., Furlanis, G., et al. (2017). Action observation plus sonification. A novel therapeutic protocol for parkinson's patient with freezing of gait. Front. Neurol. 8:723. doi: 10.3389/fneur.2017.00723
Murgia, M., Corona, F., Pili, R., Sors, F., Agostini, T., Casula, C., et al. (2015). Rhythmic auditory stimulation (RAS) and motor rehabilitation in Parkinson's disease: new frontiers in assessment and intervention protocols. Open Psychol. J. 8, 220–229. doi: 10.2174/1874350101508010220
Murgia, M., Santoro, I., Tamburini, G., Prpic, V., Sors, F., Galmonte, A., et al. (2016). Ecological sounds affect breath duration more than artificial sounds. Psychol. Res. 80, 76–81. doi: 10.1007/s00426-015-0647-z
Pantev, C., Engelien, A., Candia, V., and Elbert, T. (2001). Representational cortex in musicians. Plastic alterations in response to musical practice. Ann. N.Y. Acad. Sci. 930, 300–314. doi: 10.1111/j.1749-6632.2001.tb05740.x
Pantev, C., Lappe, C., Herholz, S. C., and Trainor, L. (2009). Auditory-somatosensory integration and cortical plasticity in musical training. Ann. N.Y. Acad. Sci. 1169, 143–150. doi: 10.1111/j.1749-6632.2009.04588.x
Park, J. H., Shea, C. H., and Wright, D. L. (2000). Reduced-frequency concurrent and terminal feedback: a test of the guidance hypothesis. J. Mot. Behav. 32, 287–296. doi: 10.1080/00222890009601379
Pau, M., Corona, F., Pili, R., Casula, C., Sors, F., Agostini, T., et al. (2016). Effects of physical rehabilitation integrated with rhythmic auditory stimulation on spatio-temporal and kinematic parameters of gait in Parkinson's disease. Front. Neurol. 7:126. doi: 10.3389/fneur.2016.00126
Plooy, A., Tresilian, J. R., Mon-Williams, M., and Wann, J. P. (1998). The contribution of vision and proprioception to judgements of finger proximity. Exp. Brain Res. 118, 415–420. doi: 10.1007/s002210050295
Proske, U. (2005). What is the role of muscle receptors in proprioception? Muscle Nerve 31, 780–787. doi: 10.1002/mus.20330
Repp, B. H. (2000). Compensation for subliminal timing perturbations in perceptual-motor synchronization. Psychol. Res. 63, 106–128. doi: 10.1007/PL00008170
Repp, B. H. (2001). Phase correction, phase resetting, and phase shifts after subliminal timing perturbations in sensorimotor synchronization. J. Exp. Psychol. Hum. Percept. Perf. 27, 600–621. doi: 10.1037/0096-1523.27.3.600
Ribeiro, F., and Oliveira, J. (2007). Aging effects on joint proprioception: the role of physical activity in proprioception preservation. Eur. Rev. Aging Phys. Activity 4, 71–76. doi: 10.1007/s11556-007-0026-x
Rosenkranz, K., Butler, K., Williamon, A., and Rothwell, J. C. (2009). Regaining motor control in musician's dystonia by restoring sensorimotor organization. J. Neurosci. 29, 14627–14636. doi: 10.1523/JNEUROSCI.2094-09.2009
Sacco, R. L., Bello, J. A., Traub, R., and Brust, J. C. (1987). Selective proprioceptive loss from a thalamic lacunar stroke. Stroke 18, 1160–1163. doi: 10.1161/01.STR.18.6.1160
Särkämö, T., Altenmüller, E., Rodríguez-Fornells, A., and Peretz, I. (2016). Music, brain, and rehabilitation: emerging therapeutic applications and potential neural mechanisms. Front. Hum. Neurosci. 10:103. doi: 10.3389/fnhum.2016.00103
Scheef, L., Boecker, H., Daamen, M., Fehse, U., Landsberg, M. W., Granath, D. O., et al. (2009). Multimodal motion processing in area V5/MT: evidence from an artificial class of audio-visual events. Brain Res. 1252, 94–104. doi: 10.1016/j.brainres.2008.10.067
Schmidt, R. A. (1991). “Frequent augmented feedback can degrade learning: evidence and interpretations,” in Tutorials in Motor Neuroscience, eds J. Requin and G. E. Stelmach (Dordrecht: Kluwer), 59–75.
Schmitz, G., and Effenberg, A. O. (2017). Schlagmann 2.0–bewegungsakustische dimensionen interpersonaler koordination im mannschaftssport. German J. Exer. Sport Res. 47, 232–245.
Schmitz, G., Kroeger, D., and Effenberg, A. O. (2014). A Mobile Sonification System for Stroke Rehabilitation. New York, NY: Georgia Institute of Technology.
Schmitz, G., Mohammadi, B., Hammer, A., Heldmann, M., Samii, A., Münte, T. F., et al. (2013). Observation of sonified movements engages a basal ganglia frontocortical network. BMC Neurosci. 14:32. doi: 10.1186/1471-2202-14-32
Scholz, D. S., Rhode, S., Großbach, M., Rollnik, J., and Altenmüller, E. (2015). Moving with music for stroke rehabilitation: a sonification feasibility study. Ann. N.Y. Acad. Sci. 1337, 69–76. doi: 10.1111/nyas.12691
Scholz, D. S., Rohde, S., Nikmaram, N., Brückner, P. H., Großbach, M., Rollnik, J. D., et al. (2016). Sonification of arm movements in stroke rehabilitation – a novel approach in neurologic music therapy. Front. Neurol. 7:106. doi: 10.3389/fneur.2016.00106
Sedlmeier, P., and Renkewitz, F. (2008). Forschungsmethoden und Statistik in der Psychologie. München: Pearson Studium.
Selfe, J., Callaghan, M., McHenry, A., Richards, J., and Oldham, J. (2006). An investigation into the effect of number of trials during proprioceptive testing in patients with patellofemoral pain syndrome. J. Orthopaed. Res. 24, 1218–1224. doi: 10.1002/jor.20127
Shadmehr, R., and Krakauer, J. W. (2008). A computational neuroanatomy for motor control. Exp. Brain Res. 185, 359–381. doi: 10.1007/s00221-008-1280-5
Shams, L., and Seitz, A. R. (2008). Benefits of multisensory learning. Trends Cogn. Sci. 12, 411–417. doi: 10.1016/j.tics.2008.07.006
Sherrington, C. S. (1907). On the proprio-ceptive system, especially in its reflex aspect. Brain 29, 467–482. doi: 10.1093/brain/29.4.467
Sigrist, R., Rauter, G., Marchal-Crespo, L., Riener, R., and Wolf, P. (2015). Sonification and haptic feedback in addition to visual feedback enhances complex motor task learning. Exp. Brain Res. 233, 909–925. doi: 10.1007/s00221-014-4167-7
Sigrist, R., Rauter, G., Riener, R., and Wolf, P. (2013). Augmented visual, auditory, haptic, and multimodal feedback in motor learning: a review. Psychon. Bull. Rev. 20, 21–53. doi: 10.3758/s13423-012-0333-8
Sihvonen, A. J., Särkämö, T., Leo, V., Tervaniemi, M., Altenmüller, E., and Soinila, S. (2017). Music-based interventions in neurological rehabilitation. Lancet Neurol. 16, 648–660. doi: 10.1016/S1474-4422(17)30168-0
Smith, T. O., King, J. J., and Hing, C. B. (2012). The effectiveness of proprioceptive-based exercise for osteoarthritis of the knee: a systematic review and meta-analysis. Rheumatol. Int. 32, 3339–3351. doi: 10.1007/s00296-012-2480-7
Stein, B. E., Stanford, T. R., and Rowland, B. A. (2014). Development of multisensory integration from the perspective of the individual neuron. Nat. Rev. Neurosci. 15, 520–535. doi: 10.1038/nrn3742
Tagesson, S., Oberg, B., Good, L., and Kvist, J. (2008). A comprehensive rehabilitation program with quadriceps strengthening in closed versus open kinetic chain exercise in patients with anterior cruciate ligament deficiency: a randomized clinical trial evaluating dynamic tibial translation and muscle function. Am. J. Sports Med. 36, 298–307. doi: 10.1177/0363546507307867
Tecchio, F., Salustri, C., Thaut, M. H., Pasqualetti, P., and Rossini, P. M. (2000). Conscious and preconscious adaptation to rhythmic auditory stimuli: a magnetoencephalographic study of human brain responses. Exp. Brain Res. 135, 222–230. doi: 10.1007/s002210000507
Tiggelen, D. V., Coorevits, P., and Witvrouw, E. (2008). The effects of a neoprene knee sleeve on subjects with a poor versus good joint position sense subjected to an isokinetic fatigue protocol. Clin. J. Sport Med. 18, 259–265. doi: 10.1097/JSM.0b013e31816d78c1
Tinazzi, M., Fiaschi, A., Frasson, E., Fiorio, M., Cortese, F., and Aglioti, S. M. (2002). Deficits of temporal discrimination in dystonia are independent from the spatial distance between the loci of tactile stimulation. Mov. Disord. 17, 333–338. doi: 10.1002/mds.10019
van Beers, R. J. (2009). Motor learning is optimally tuned to the properties of motor noise. Neuron 63, 406–417. doi: 10.1016/j.neuron.2009.06.025
Van Vugt, F. (2013). Sounds on Time: Auditory Feedback In Motor Learning, Re-Learning and Over-Learning of Timing Regularity. Lyon: Claude Bernard Université Lyon I.
van Vugt, F. T., and Tillmann, B. (2015). Auditory feedback in error-based learning of motor regularity. Brain Res. 1606, 54–67. doi: 10.1016/j.brainres.2015.02.026
Verschueren, S. M., Cordo, P. J., and Swinnen, S. P. (1998). Representation of wrist joint kinematics by the ensemble of muscle spindles from synergistic muscles. J. Neurophysiol. 79, 2265–2276. doi: 10.1152/jn.1998.79.5.2265
Vidoni, E. D., and Boyd, L. A. (2009). Preserved motor learning after stroke is related to the degree of proprioceptive deficit. Beh. Brain Funct. 5:36. doi: 10.1186/1744-9081-5-36
Wilson, E. C., Braida, L. D., and Reed, C. M. (2010a). Perceptual interactions in the loudness of combined auditory and vibrotactile stimuli. J. Acoust. Soc. Am. 127, 3038–3043. doi: 10.1121/1.3377116
Wilson, E. C., Reed, C. M., and Braida, L. D. (2009). Integration of auditory and vibrotactile stimuli: effects of phase and stimulus-onset asynchrony. J. Acoust. Soc. Am. 126, 1960–1974. doi: 10.1121/1.3204305
Wilson, E. C., Reed, C. M., and Braida, L. D. (2010b). Integration of auditory and vibrotactile stimuli: effects of frequency. J. Acoust. Soc. Am. 127, 3044–3059. doi: 10.1121/1.3365318
Winstein, C. J., and Schmidt, R. A. (1990). Reduced frequency of knowledge of results enhances motor skill learning. J. Exp. Psychol. Learn. Memory Cogn. 16:677.
Wolpert, D. M., and Miall, R. C. (1996). Forward models for physiological motor control. Neural Netw. 9, 1265–1279. doi: 10.1016/S0893-6080(96)00035-4
Yau, J. M., Olenczak, J. B., Dammann, J. F., and Bensmaia, S. J. (2009). Temporal frequency channels are linked across audition and touch. Curr. Biol. 19, 561–566. doi: 10.1016/j.cub.2009.02.013
Zatorre, R. J., Chen, J. L., and Penhune, V. B. (2007). When the brain plays music: auditory-motor interactions in music perception and production. Nat. Rev. Neurosci. 8, 547–558. doi: 10.1038/nrn2152
Zhang, J. T., Novak, A. C., Brouwer, B., and Li, Q. (2013). Concurrent validation of Xsens MVN measurement of lower limb joint angular kinematics. Physiol. Meas. 34:N63. doi: 10.1088/0967-3334/34/8/N63
Keywords: perception, rehabilitation, sonification, coordination, joint position sense
Citation: Ghai S, Schmitz G, Hwang T-H and Effenberg AO (2018) Auditory Proprioceptive Integration: Effects of Real-Time Kinematic Auditory Feedback on Knee Proprioception. Front. Neurosci. 12:142. doi: 10.3389/fnins.2018.00142
Received: 15 December 2017; Accepted: 22 February 2018;
Published: 08 March 2018.
Edited by:
Mauro Murgia, University of Trieste, ItalyReviewed by:
Federica Corona, Università degli Studi di Cagliari, ItalyCopyright © 2018 Ghai, Schmitz, Hwang and Effenberg. This is an open-access article distributed under the terms of the Creative Commons Attribution License (CC BY). The use, distribution or reproduction in other forums is permitted, provided the original author(s) and the copyright owner are credited and that the original publication in this journal is cited, in accordance with accepted academic practice. No use, distribution or reproduction is permitted which does not comply with these terms.
*Correspondence: Shashank Ghai, c2hhc2hhbmsuZ2hhaUBzcG9ydHdpc3MudW5pLWhhbm5vdmVyLmRl
Disclaimer: All claims expressed in this article are solely those of the authors and do not necessarily represent those of their affiliated organizations, or those of the publisher, the editors and the reviewers. Any product that may be evaluated in this article or claim that may be made by its manufacturer is not guaranteed or endorsed by the publisher.
Research integrity at Frontiers
Learn more about the work of our research integrity team to safeguard the quality of each article we publish.