- 1LCEN3, Department of Neurology, Institute of Neuroscience, Ghent University, Ghent, Belgium
- 2Neuroscience Research Flanders, Leuven, Belgium
- 3Life Science and Imaging, Imec, Leuven, Belgium
- 4Environment, Health and Safety, Imec, Leuven, Belgium
Deep Brain Stimulation (DBS) has evolved into a well-accepted add-on treatment for patients with severe Parkinsons disease as well as for other chronic neurological conditions. The focal action of electrical stimulation can yield better responses and it exposes the patient to fewer side effects compared to pharmaceuticals distributed throughout the body toward the brain. On the other hand, the current practice of DBS is hampered by the relatively coarse level of neuromodulation achieved. Optogenetics, in contrast, offers the perspective of much more selective actions on the various physiological structures, provided that the stimulated cells are rendered sensitive to the action of light. Optogenetics has experienced tremendous progress since its first in vivo applications about 10 years ago. Recent advancements of viral vector technology for gene transfer substantially reduce vector-associated cytotoxicity and immune responses. This brings about the possibility to transfer this technology into the clinic as a possible alternative to DBS and neuromodulation. New paths could be opened toward a rich panel of clinical applications. Some technical issues still limit the long term use in humans but realistic perspectives quickly emerge. Despite a rapid accumulation of observations about patho-physiological mechanisms, it is still mostly serendipity and empiric adjustments that dictate clinical practice while more efficient logically designed interventions remain rather exceptional. Interestingly, it is also very much the neuro technology developed around optogenetics that offers the most promising tools to fill in the existing knowledge gaps about brain function in health and disease. The present review examines Parkinson's disease and refractory epilepsy as use cases for possible optogenetic stimulation therapies.
1. Introduction
Therapeutic use of electricity dates back to antiquity. Deep Brain Stimulation (DBS) originates from the advancement of the sterotactic surgical techniques, which allowed the transition from lesional to stimulating technique of the deep nuclei of the brain for therapeutic purposes. Readers are directed to the historical survey by Sironi (2011) for further information. At present, DBS is an established therapeutic option for a variety of neurological diseases, such as Parkinson's disease (Beitz, 2014), essential tremor (Børretzen et al., 2014), dystonia (Lumsden et al., 2013) and obsessive-compulsive disorder (Greenberg et al., 2010). Closely related to DBS is another electrically-based therapy called neuromodulation. Applied to peripheral (Bhadra and Peckham, 1997) or cranial nerves (Ben Menachem and French, 2005), the spinal cord (Francois et al., 2017), the cochlea (Rajguru et al., 2010), the retina (Nirenberg and Pandarinath, 2012) as well as to the brain (Rossi et al., 2016). This technique is used to treat many conditions and for theses indications it can already be classified as a well–accepted clinical treatment.
Using light to control neural activity holds promises for much improvement since genetics has developed the tools to make specific structures light sensitive. Under appropriate conditions, the action potential, an all-or-nothing phenomenon seen as the neural information carrier, can be triggered by light1 as well as by electrical stimulation. The use of light for brain stimulation is a disruptive advancement for both neuromodulation and DBS. This entirely different stimulation modality is brought about by a critical mass of innovations in molecular genetics and virology. Towne and Thompson (2016) define optogenetics as “a method that uses light to control cells in living tissue, typically neurons, that have been modified to express light-sensitive ion channels and pumps.” The foundation of optogenetics is a combination of genetic manipulations, which renders identified populations of neurons sensitive to the action of light. Development of optogentics would not have been possible without the research conducted on light sensitive algae by Nagel et al. (2002). The optogenetic approach was further championed by Deisseroth, Boyden, Miesenböck and Haegemann and has led to an explosive proliferation of different variants of light-sensitive ion channels, G protein-coupled receptors and ion pumps (Boyden et al., 2005; Lima and Miesenböck, 2005). In the last 8 years, optogenetics has become an established research tool for studying brain function. In fact, results obtained so far indicate that optogenetics provides unprecedented control and granularity of stimulation (Boyden et al., 2005).
So far optogenetics has been used predominantly as a research tool in animals, however applications in humans are not deemed impossible. There are patents in this direction filed by Boyden et al. (2012) and Deisseroth et al. (2015, 2016) to name but a few. Orphan status was recently granted to a viral-vector-based optogenetic therapy (from the company RetroSense Therapeutics) for retinitis pigmentosa, and initial clinical trials to evaluate safety are underway (Yun and Kwok, 2017). Microbial opsins do not possess toxic properties per se, therefore, applications in humans appear feasible from this perspective. Safety of the resulting therapy is, therefore, expected to depend mostly on the long-term properties of the genetic vector together with the safety of the implant. As such, safety aspects of the implants can be optimized to a sufficient extent based on the abundant experience with various types of DBS and other electrodes applied to neuromodulation and neuroprosthetics.
In this review, we will focus on Parkinsons disease with its local degenerative changes inducing a profound motor control disorder and refractory epilepsy as an example of a more distributed network disease, although often triggered by a focal lesion. Parkinson's disease and epilepsy can be taken ins some sense as extreme cases, since the acceptance criteria for eventual optogentics therapies can be very different. Both diseases get increasing attention in the literature as potential applications (see Figure 1). In Parkinson's disease, a loss of dopaminergic neurons leads to the loss of inhibitory gamma aminobutyric acid-sensitive input to the subthalamic nucleus. While there is no generally-accepted definition of refractory epilepsy (French, 2006), this term generally designates a spectrum of pathologies characterized by recurrent seizures, which respond poorly or not at all to conventional medicines. Clinical evidence indicates that some of these patients will actually benefit to some extent from add-on treatments while maintaining the antiepileptic drugs unchanged. At present, the main treatment options for refractory epilepsy are brain surgery (i.e., temporal lobe localized neocortical resection) and vagus nerve stimulation, which is a variety of neuromodulation (review in Cox et al., 2014). More recently, researchers started to explore possible optogenetic approaches as well (Wykes et al., 2016).
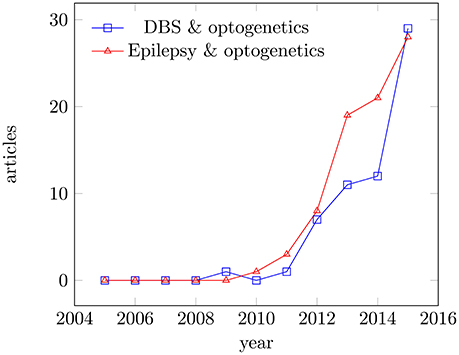
Figure 1. Articles published for the period 2005–2015. Articles published in Medline for the period 2005–2015; keywords: deep brain stimulation and optogenetics, epilepsy and optogenetics. Data were analyzed using the Medline trends tool (Corlan, 2004).
Neuromodulation, defined as as “the alteration—or modulation—of nerve activity by delivering electrical or pharmaceutical agents directly to a target area2,” will be considered in some alternative realizations. At present, clinically accepted neuromodulation and DBS therapies are still open-loop. However, the importance of simultaneous stimulation and recording from the neural tissue should not be overlooked. There are several reasons for this, including the possibility to better titrate the therapy and also to ensure appropriate timing of stimuli according to the state of the neural target. The sensing branches can control the stimulation regimen, adapt it to the state and needs of the neural system itself (Krook-Magnuson et al., 2013) or lock the activation under a given safety limit. In “controlled therapy,” sensing is essential to use physiological signals for triggering the therapeutic device (Zrenner et al., 2016). This is particularly important for epilepsy (Sorokin et al., 2017). Contrarily to electrical stimulation, optogenetics has the great advantage not to interfere with the recording of the tiny neural electrical signals. Already existing systems combine optogenetic activation with electric feedback loops (Emiliani et al., 2015; Yang et al., 2016).
The present review will not consider issues related to the optimizations and efficiency of light-sensitive ion channels and ion pumps, where there is a steady progress. Interested readers are directed to recent overviews by Wietek and Prigge (2016) and Deisseroth (2015) on opsin technology. Instead, we will address clearly unresolved issues, among which the biosafety of viral vectors.
2. A Note on the Mechanisms of DBS
The clinical use of DBS is mostly empirical, based on decades of experience with surgical ablative therapies. It must be emphasized that historically the major findings in both DBS and neuromodulation fields rest on serendipity and empirical observations because fundamental knowledge about the brain is still far from allowing complete understanding of patho-physiology as would be required to design optimal therapies. There are numerous historical examples ranging from the Renaissance era observations of Galvani to mid twentieth (Sironi, 2011) discovery of positive reinforcement by electrical stimulation (Olds and Milner, 1954).
It is an accepted clinical observation that both DBS (expected to stimulate the immediate target) and a lesion (silencing the same target) do alleviate symptoms of motor disorders despite their apparently opposite actions. In contrast, local stimulation leads to neuronal excitation and DBS affects symptoms on varying timescales and involving structures at different levels of organization.
To the present date, the mechanisms underlying the therapeutic effects of DBS remain insufficiently understood to predict applications in new therapeutic domain (recent reviews in Chiken and Nambu, 2016; McIntyre and Anderson, 2016). Even after 25 years of continuous application and research the circuit-level mechanisms of DBS remain elusive. While early hypotheses focused on the analogy between effects of ablation and those of stimulation, recent hypotheses on the mechanisms of DBS have shifted toward network-based theories, forgoing a direct link between lesioning and stimulation, and focusing instead on stimulation-induced disruption of pathological network oscillations (Johnson et al., 2008; McIntyre and Hahn, 2010; McIntyre and Anderson, 2016). It can even be conceived that DBS interferes with certain communication channels of the brain and thus disrupts pathological patterns of activity. The simplistic view of an imbalance between excitatory and inhibitory pathways must be complemented to take into account phenomena, such as retrograde activation, synchronicity in specific cells, interference with spontaneous (rhythmic) activity (Tass et al., 2012) and cell type activated (Witt et al., 2013). The role of astrocytes is well recognized but still difficult to integrate in our models (Kovacs and Pal, 2017). More and more, it is the limitations in physiological knowledge that impedes an optimal exploitation of techniques, such as optogenetics in the clinical world (Karas et al., 2013). On the other hand, optogenetics offers the new tools needed to further explore the brain and that is indeed what most application papers are about today (Gradinaru et al., 2009).
3. A Multidisciplinary Context
In this review, we argue that a number of independent technologies must converge to implement optogenetics as an effective therapy. We discern six major steps, which will be discussed in more details in subsequent sections:
1. The recombinant genetic technology required to develop a gene encoding the desired photosensitive ion-channel or any other desired protein.
2. The transfection technology required to introduce the new gene into target cells.
3. The genomic know-how needed to assure that the newly inserted gene gets activated.
4. The stereotaxic surgery which can play an essential role in the transfection by injecting agents into the appropriate target and by implanting the light stimulation device as required.
5. The engineering know-how of designing and producing an implantable optical stimulator is also significant and it represents a clearly distinct field of expertise, itself subdivided in several disciplines.
6. The clinical application of the optogenetic system involves the clinical and physiological knowledge, which is necessary to personalize parameter settings and optimize the clinical protocol.
Steps 1–3 are specific to optogenetics, while 4–6 are more generic.
It should be stressed that each technological domain listed above can also develop some forms of treatment on its own. Gene therapy (Collins and Thrasher, 2015) for example has been attempted long before optogenetics was even conceived. Direct insertion of functional proteins (Lin et al., 2017) or optic control of these proteins (Brechun et al., 2016) have also been suggested. The observation of Parkinson symptoms improvement after a localized brain lesion (Dubois et al., 1986) inspired the use of stereotactic brain surgery in patients. This old technique of stereotactic surgery (Lehman and Augustine, 2013) is facing a recent revival through the availability of new technological improvements (Starr et al., 1998). The effect of electrical stimulation used for anatomical guidance during ablative surgery finally resulted in the replacement of the brain lesion by a reversible DBS system (Benabid et al., 1996) and brain stimulators for human clinical use are now common. Hitherto, history of therapeutic progresses has very much resulted from serendipity and empirical findings rather than physiological knowledge based design. In addition to the need for more basic science, multi-disciplinarity characterizes modern brain therapies (Rossi et al., 2016). Genetics, pharmacology, photo-chemistry, cellular biology, surgery, neuroscience, optics, electronics, material science and various medical clinical specialties, to name but the most evident domains will have to collaborate. Unfortunately, there is often a significant tension between the required scientific freedom to transgress discipline boundaries on one hand and the institutional organization for teaching and employment of academics on the other hand (Osborne, 2015). Today, this constraint still represents a major challenge in a field, such as optogenetics, creating an urgent need for experts with overlapping skills and knowledge and, perhaps further thoughts about transdisciplinarity (Mittelstrass, 2011).
4. Light vs. Electrical Current
4.1. Electrical Stimulation for DBS
Electrical activation of voltage sensitive channels is controlled by the voltage difference across the cell membrane, which drives ion currents. Sodium transient channel opening is activated by a membrane depolarization, thus by an inward current through the neural membrane. Other voltage sensitive channels have different responses to voltage and are more or less specific to other ions. The seven nanometer-thin lipid cell membranes form a relatively large electrical capacitance, requiring current to flow for some time before the activation threshold voltage can be reached. This explains the main part of the strength-duration relationship between activation threshold and stimulation pulse duration (Bostock, 1983). Assuming that the membrane conductivity is negligible compared to the extracellular space, Kirchoff's laws indicate that the current through the membrane is roughly proportional to the Laplacian (second spatial derivative) of the voltage along the membrane and a constant resistive factor. In peripheral nerves, the differential equations can be approximated by difference equations spanning over anatomically-defined distances determined by the nodes of Ranvier. These are considered as the only active spots along myelinated axons (Stephanova and Bostock, 1995). More complex volume conductor models have been developed for complex dendritic trees of the neurons in the central nervous system (Ranck, 1975). Not all identical neural structures are activated simultaneously but their recruitment depends on the anatomical position of the stimulation electrodes, the electrical properties (conductivity) of the intervening tissue and the anatomy of the neural structure itself. Variation of the waveform's shape can be used to improve the selectivity of stimulation in the peripheral nervous system by recruiting different groups of fibers. Readers are referred to the recent review of Grill et al. (2009) for update on the topic. Recent modeling studies point out some possibilities in the central nervous system in terms of neuromodulation by AC currents (Mahmud and Vassanelli, 2016).
Magnetic stimulation can be compared to electrical stimulation in the sense that the extracellular current generated by a pair of electrodes is now replaced by an induced current by a varying magnetic flux (Silva et al., 2007). In both cases, the extracellular current generates an extracellular potential field which induces the trans-membranous current. When that current has loaded the membrane capacitance up to a threshold potential, the voltage sensitive channels set up an all-or-nothing action potential.
Variables determining DBS outcome are the stimulation parameters and the positioning of the electrode. The stimulation parameters include the amplitude (current of voltage), frequency, and pulse width. These parameters play a role defining the volume of tissue activated (VTA) and in the therapeutic effectiveness of DBS (Butson et al., 2007). Typical clinically effective parameters for monopolar DBS are presented in Table 1.
VTA in human subjects has been estimated in computational models (Maks et al., 2009). The more complete versions use patient specific imaging data that enrich the models with anatomical information. They also incorporate electrical characteristics, such as impedance and anisotropy, of the different brain parts surrounding the electrode. The study by Maks et al. (2009), found volumes varying from 30 to 116 mm3 with an average of 71 mm3 (i.e., 5 mm diameter). The results from this study further suggest that smaller stimulation volumes in the subthalamic nucleus (STN) are better at alleviating Parkinson's symptoms. The model was experimentally verified indirectly by estimating the stimulation volume spreading into the capsula interna for different voltages and comparing this to muscle twitching of the arm or leg by patient stimulation of the corticospinal tract (Butson et al., 2007). Other studies from this laboratory measured the voltage spread in the brain of a rhesus monkey and compared it to the one predicted by the model (Miocinovic et al., 2009). The study found that the model accurately predicted the voltage distribution observed in vivo.
4.2. Light Stimulation and Opsins
Within the tissue, photons interact with biological matter via various processes, which can be broadly categorized into scattering and absorption. An accessible overview on current photonics medical applications is given in Yun and Kwok (2017). Light works in a different way upstream of the activation of the membrane ion channels. Here, it is the optical properties of light across the tissues that will determine the threshold and target selectivity. In the case of the well-studied ion channel ChR2, the blue light will cause all-trans-retinal to isomerize to 13-cis-retinal, and so a conformational change in the protein will open the ChR2 channel to allow cations to enter and depolarize the cell. Switching the light off again will cause the 13-cis-retinal to revert to its original state closing the channel and thereby repolarizing the cell. These ion channels do not close spontaneously (as the voltage sensitive sodium channel does) and the resulting response dynamics is in the first place dependent on the opto-chemical properties and density of the photosensitive channels. The strength-duration relationship or the significance of the stimulus duration is now entirely different despite still linked to electrical charges on the membrane capacitance. When open, these charges depolarize the membrane by providing a leakage current. Only then the membrane capacitance starts loading and the density of the available channels is thus much more a limiting factor. The strength-duration relationship still corresponds to a membrane capacitance being loaded, but no longer through the same current.
It should be noted that even if the stimulus does not produce an action potential, a membrane potential shift occurs, such that very long stimulus pulses will modulate spontaneous activity. New phenomena can thus be expected to occur (Grossman et al., 2011). For example, on striatal brain slices, dopamine release (which is an effect pursued in treating Parkinson's disease) appears to be stable when electrically stimulated while a rundown effect is observed with optical stimulation (O'Neill et al., 2017). Once the membrane depolarization voltage has reached the threshold value, however, the same voltage-controlled mechanism as described for electrical stimulation will launch a propagated action potential. Activation selectivity still depends on the geometry and intervening tissues. However, whereas electrical stimulation is controlled by the electrode position, the membrane ion channel distribution and roughly constant resistivity, here, source orientation, source optic features (color, polarization), optic characteristics of intervening tissues, channel density and opto-sensitivity, are the key parameters defining the threshold, selectivity and recruitment.
Selectivity also arises from the use of cell-specific promoters, which drives the expression of the protein in the cells. The opsin is placed downstream of a strong promoter such as synapsin, CMV or CAG. This will lead to strong expression of the opsin in almost all of the cells where the construct is present. Alternatively, one can opt to target cell-specific promoters such as alpha-calcium/calmodulin-dependent kinase II (α-CamKII), which is expressed in forebrain pyramidal neurons. Where electrical stimuli can in some conditions block the propagation of action potentials, similar effects are now obtained with infra-red pulses (Walsh et al., 2016). Some opto-sensitive channels can work as cell silencers, which is not the same as the selective activation of inhibitory pathways, which both techniques could in principle achieve (Malyshev et al., 2017). Through color selectivity, a single optic device could now selectively inhibit or activate the same cells.
Geometric or anatomical selectivity is in principle available to optic stimulation as well as to electrical micro-electrodes (McCreery et al., 2006). Activating light oriented toward a specific points is a realistic goal in examples, such as the retinal prosthesis (Soltan et al., 2017). Optical techniques are being developed that could achieve similar or better selectivity and parallelism than the electrical equivalent (McAlinden et al., 2015; Conti et al., 2016). It should be emphasized that the placement of electrodes is entirely dependent on the surgery. Electrode placement is also a selectivity factor in the frame of optogenetics but selective transfection is another possibility so that only the targets are made photo-sensitive. Selective activation of specific cells, as can be achieved in the frame of optogenetics, could become a key to a successful therapy (Yekhlef et al., 2017). However, the impact on the therapeutic effect cannot yet be established on the basis of the rather preliminary information available today.
4.3. Functionality
The architecture of the brain nuclei poses a problem for purely electrical stimulation because electrodes are relatively indiscriminate with regards to the underlying physiology of the neurons that they stimulate. Typically, physical proximity of the stimulating electrode contact to the neuron is often the determining factor as to which neurons will be stimulated (Deisseroth et al., 2014). Accordingly, it is not considered feasible to restrict stimulation to a single class of neurons with electrical stimulation while optogenetics promises to do just that and even to activate specific channels within the same neurons.
In optogenetics, the volume of tissue that gets activated is also determined by the interaction of several processes. Neuronal activation depends on the absolute amount of light that reaches the neurons, the efficiency of the transfection and the sensitivity of the opsin. Besides these, neuronal physiological properties and the network in which the neuron is embedded also influence how effectively light can control the activity. The propagation of light in the brain is determined by the light absorption and scattering (Vo-Dinh, 2003). How far light reaches inside the brain can be estimated using Monte Carlo simulations. Different studies have calculated that the light intensity drops to 1% at a distance 1 mm away from the emission point (Bernstein et al., 2008; Chow et al., 2010; Stujenske et al., 2015). Additionally, these simulations show that the light distribution inside the brain has an ellipsoidal shape depending on the wavelength, the size and type of light source and the emission aperture angle (i.e., numerical aperture). The simulations also show that a portion of the light back scatters and illuminates the tissue behind the optical fiber. This effect gets exacerbated at higher emission powers to the point that, in some cases, the tip of the fiber is at the center of the illuminated volume. Regarding the sensitivity, studies in acute slices and in vitro show that maximal channel activation for different channelrhodopsin 2 (ChR2) variants is achieved at a power density of 10 mW/mm2, with only 50% of the channels getting activated at 1 mW/mm2 (Wang et al., 2007; Lin et al., 2009). If an optgenetic DBS would deliver light through an optical fiber with a core diameter of 200 μm and a numerical aperture of 0.22, the data obtained by (Stujenske et al., 2015) can be used to estimate the power required to achieve an activation volume similar to electrical DBS (i.e., 5 mm sphere). For maximal neuronal acativation, a total of 1.5 W would be required to obtain a power density larger than 10 mW/mm2 within the illumination volume. Assuming that the tip is indeed at the center. The required power gets exponentially higher if it is not. If the criteria are relaxed and only a power density of 1 mW/mm2 is provided at the end of the volume only 150 mW should be delivered. The first value is prohibitively high and would probably create some direct damage in the brain. The second is also quite high, although the brain might be able to withstand it. However, a portable device that has to deliver that amount of power would probably not be practical. Tapered fibers have been tested recently by Pisanello et al. (2017) who showed that varying tapering angle allows for obtaining selectivity of stimulation in vertical direction. Authors claim that achieving uniform effective illumination of large brain structures with minimal invasiveness and light power. In addition, tapering permits smooth insertion into the brain, which also minimizes tissue reaction.
So-stated arguments indicate that stimulation paradigm with ChR2 analogous to DBS might not be the best approach with common ChR2 variants. An alternative could be to use recent variants like ChR2-XXL, which is 10,000 times more sensitive with the disadvantage that it is slower (Dawydow et al., 2014). However, this approach does not take advantage of the capabilities of the optogenetical tools for specific cell type stimulation. Selectivity of stimulation has been tested in rodent models with some positive results (Gradinaru et al., 2009). As an alternative, the inhibition modality of optogenetics could be used. A widely accepted hypothesis of DBS for Parkinson's disease is that excitatory neurons in the STN are inhibited (Shin et al., 2007; Sutton et al., 2013). This hypothesis has been tested by two different groups in rodent models of Parksinosn's disease (Gradinaru et al., 2009; Yoon et al., 2014, 2016). Unfortunately, the results obtained by each group contradict the other, although Yoon et al. (2014) attribute the difference to the animal model and the test used to evaluate Parksinosn's disease improvement by the other group. Tables 2, 3 show the efficacy of applying optogenetic stimulation or inhibition to rodent models in different studies. The tables have a line-to-line correspondence to facilitate comparison. Although, there is yet no definitive conclusion regarding the use of optogenetics of the treatment of Parkinson's disease, these studies demonstrate that optogenetics would be an indispensable tool to better understand mechanisms of Parksinosn's disease and DBS.
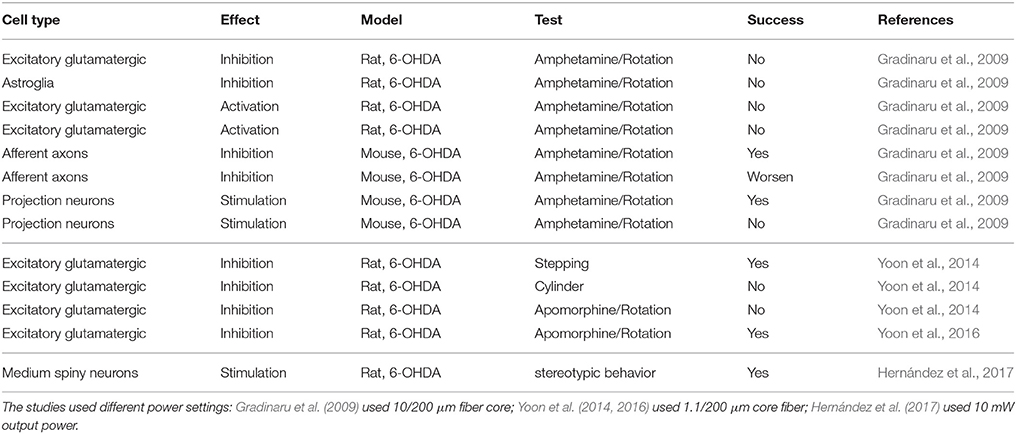
Table 3. Summary of the efficacy of applying optogenetics in Parksinosn's disease rodent models, II.
We have seen that electrical currents and light do not necessarily activate the same structures. In addition, photosensitive channels have been developed for specific ions and superposition of different light wavelengths allows for activation/inhibition combinations that are not possible with electrical stimuli. However, once action potentials are launched, they can no longer be distinguished on the basis of the triggering event. Parameters, such as pulse frequency, train duration, train rate, treatment session duration, duration of the therapy, event-triggering of the therapy and so on are expected to yield the same effects if the same structures were activated and, this is where a serious word of caution is warranted. In addition, the neural system is an adaptive network. Plasticity, as a general phenomenon can completely modify long-term effects.
Long-term consideration is thus not only important to check the lifetime of implanted device or to evaluate unwanted side effects but also to ensure effectiveness of the treatment strategy (Jarvis and Schultz, 2015). As “accelerated aging” methods do not work here, chronic studies and high sensitivity detection seem to be the only alternative.
5. Optogenetics
At present, optogenetic ion channels are designed routinely on the basis of the family of the bacterial opsins. In hindsight is seems surprising that applications in the neural system took as long as 40 years after the initial isolation of the halobacterial rhodopsin. Only, by 2010, the major classes of ion-conducting microbial opsins have been demonstrated to be able to excite neurons (Yizhar et al., 2011). Optogenetic proteins can be classified into several overlapping groups by the mode of their actions (i) fast-inhibiting; (ii) fast exciting; (iii) step function opsins (i.e., bi-stable); (iv) modulated biochemically (review in Yizhar et al., 2011). A large variety of channels with different absorption spectra and kinetics have been designed. The temporal precision based on the use of custom opsin designs offers unprecedented opportunity for modulation of defined neuronal populations. Such perspective is in a sharp contrast with the relatively “broad–band” electrical stimulation traditionally employed in DBS.
5.1. Variants of Optogenetic Approaches
5.1.1. Overwhelming Possibilities
In addition to forms sensitive to different wavelengths, the classical opsins such as DNA/channelrhodopsin 2 (ChR2) have been complemented with Halorhodopsin (NpHR) that hyperpolarizes the Cl- pump (Klapper et al., 2016) and Archaerhodopsin-3 (Arch) that forms a light sensitive proton pump (Mantoan Ritter et al., 2014). As already mentioned, combining these makes it possible to control different effects using different light colors (Stark et al., 2012). More recently, several cellular control methods have been developed around the possibility to modulate G-proteins (Kleinlogel, 2016) thus opening a new branch of optogenetics with new applications in perspective. Alternatively, genes for endo-cellular molecules (for example genes expressing fluorescent proteins for bio-sensing Enterina et al., 2015) as well as various ion channels can be integrated in the cell genome. For example, intracellular calcium can be specifically controlled (Mager et al., 2017). Key chemicals controlling cellular biology (precursors, enzymes, neurotransmitters and their agonists, sensors or signaling molecules (Smedemark-Margulies and Trapani, 2013), can be delivered to the brain in an inactive form (drug-encapsulating liposomes, caged molecules or photosensitive inactivating bounds) and activated under light control (Nakano et al., 2016). In other applications, optochemistry uses photosensitive uncaging (glutamate release in acute experiments) (Venkataramani et al., 2007). There are also examples of photoreceptors that can be used as “optogenetic switches” (Salinas et al., 2017). Astrocytes can be selectively activated by light-activated Gq protein-coupled opsins (Mantoan Ritter et al., 2014). Among the non-neuronal cells that can be activated, oligodendrocytes might also become important therapeutic targets (Lee et al., 2016). The new possibility to modify synapses (Sinnen et al., 2017) could become a major tool in correcting or adjusting neural pathway balances. Even unexpected possibilities, such as controlling cell mechanical interactions, might become possible (Valon et al., 2017).
5.1.2. Limitations
As a rule, the introduced foreign proteins trigger a foreign body immune reaction. In addition, proteins tend to be catabolized so that only gene modifications can enable a chronic effect. Direct introduction of functional proteins seems limited to acute experiments.
5.1.3. Possible Applications
All monogenic diseases are in principle candidates for gene therapy. Many clinical trials have already taken place in various diseases outside the neural system: myopathies, haemophilia, retinitis pigmentosa are just a few examples (Collins and Thrasher, 2015). Genetically encoded sensors have become essential research tools and might later offer perspectives for “closed loop systems” (Emiliani et al., 2015; Yun and Kwok, 2017). Bioluminescent indicators for voltage and various ion sensors are commercially available (Inagaki et al., 2017).
6. Viral Vectors
From the point of view of biotechnology, viruses are almost perfectly evolved nano-machines for gene delivery. Viruses can infect host cells and completely overtake their metabolism reprogramming it to serve only for their replication. Outside the host cell, the nucleic acid (i.e., DNA or RNA) forming the genome is encapsulated in a protected shell called capsid forming a particle with dimensions in the range of 20–200 nm. The particle itself is called virion. The proteins on the surface of the capsid are responsible for the target specificity and incorporation in the cell.
Rapid development of viral vector biotechnologies allows for selective modification of the wild type virus properties. Viral genomes can be edited and the genes, encoding pathogenic functions, can be removed and replaced by different genes allowing for engineering of the target cell's function. Such engineered construct is called a viral vector and it has important differences by design compared to the native prototype (i.e., the wild type virus).
• The most important difference is that by design, viral vectors do not replicate. This feature is achieved by deliberately removing all replication-specific genes from the prototype viral genome. This has impact on the production of the vector. Often the viral genome is segregated into 2–4 different plasmids.
• Viral vectors retain their invasiveness. They can infect host cells and inject their genetic material in the cytoplasm and in some cases integrate in the eukaryotic genome.
• Viral vectors are selected for their specificity.
Many viruses have been used as prototypes of viral vectors, however only few types are considered suitable for human application. Readers are directed to the recent reviews of Gray et al. (2010b), Lentz et al. (2012), and Kantor et al. (2014) for specific gene-therapy and production aspects of viral vectors. Since the envisioned genetic manipulation making the brain susceptible for optogenetics aims to treat specific diseases one has to consider it as a type of gene therapy. Therefore, all safety and ethical properties of such therapies should apply in further analysis. We give an initial consideration of the issues in the subsequent sections.
6.1. Safety of Gene Therapy and Viral Vectors
Gene transfer experiments in humans started in 1990s but were hampered by initial failures and serious adverse effects, which occurred in the early trials. By present, viral vector technology advanced substantially because of the substantial improvement of our understanding of how different viruses interact with the organism and how their genomes can be designed to reduce biohazard and improve efficiency.
6.2. Adenovirus Vectors
Historically, the first applications of gene therapy used adenoviruses and retroviruses. An adenovirus is a non-enveloped particle of size ranging between 70 and 100 nm. Adenoviruses (AV) have been isolated from a large number of species and tissue types, and in humans, they cause mild respiratory illnesses and gastroenteritis. The virus genome consists of linear double stranded DNA of approximately 36 kbp. An AV particle enters a cell via receptor-mediated endocytosis. The virus escapes the endosome and translocates to the nucleus where it delivers its DNA, which stays episomal. AV can efficiently infect a wide variety of cell types independent of the phase of the cell cycle (Harui et al., 1999).
While adenovirus vectors are very efficient at delivering genes, adenovirus vectors can cause toxic effects that limit their efficiency and safety. AV are highly immunogenic. Upon contact with the virus, the human immune system mounts a full-scale assault, including CD4+ T-helper cells, CD8+ cytotoxic-T cells, and NK cells, in order to clear the virus (Xu et al., 2010). Intravenous AV vector delivery for gene transfer purposes, especially at high doses, stimulates strong innate and adaptive immune responses and can be fatal for the host as notoriously found out in the first clinical trials. Further deletions of the adenoviral genes have yielded helper-dependent adenovirus (HD-Ads) or “gut-less” vectors (Kochanek et al., 2001), which are completely devoid of viral protein coding sequences. This has decreased immunogenicity and also prolonged transgene expression.
In systemic application AV are sequestrated by the liver Kupffer cells and cause toxic effects there. AV vectors also activate the complement system (Manickan et al., 2006; Tian et al., 2009). In addition, high doses of adenovirus vectors rapidly induce a burst of platelet activating factor in vivo that can lead to shock.
From this overview it can be concluded that AV vectors are sub-optimal for gene delivery in the brain due to their immunogenicity (i.e., possibility ot trigger gliosis and neurodegeneration) and low specificity.
6.3. Adeno Associated Virus (AAV) Based Vectors
AAV forms small, non-enveloped virions with icosahedral symmetry. AAV belongs to the Parvoviridae family and has single-stranded DNA (see Table 5). Multiple serotypes of AAV have been described (AAV1–AAV9) with distinct tissue tropism and transduction efficiency (see Table 4). AV can not replicate by itself and is not related to any known human pathology. AAV can replicate only in co-infection with helper viruses, such as AV, HSV or papiloma virus (HPV) (Weitzman and Linden, 2011). For example, the adenovirus acts as a helper virus by supplying the E1a, E1b, E2a, E4orf6 and viral-associated RNA genes.
The AAV genome consists of two 145 base-pair inverted terminal repeats (ITR), rep and cap genes. The ITRs form loop structures and are the only cis-acting elements that are necessary for genome replication, integration and packaging in the capsid (Kantor et al., 2014). The wild type AAV genome encodes four rep proteins—Rep 78, 68, 52, and 40; three viral structure proteins forming the capsid—VP1, 2 and 3; assembly activating protein, which is involved in the translocation to the nucleolus—AAP (review in Smith, 2008).
The capsid determines the tissue specificity or tropism of a given virus by regulating the immediate cellular response to the virus, mediating pathways for internalization into the cell, and functions in the uncoating process within the nucleus. Specific regions of the capsid proteins interact with receptors and co-receptors on the host cellular surface to mediate the viral infection process and serotypes can differ with respect to the receptors that they bind to. AAV infects a host cell through receptor-mediated endocytosis.
Approximately 80% of the population is seropositive for anti-AAV antibodies (review in Weitzman and Linden, 2011). Also approximately 60% of the population has neutralizing antibodies at age 10, which persists into adulthood. Nevertheless, surprisingly little is known about the life cycle of AAV in humans.
The AAV vectors can stably transfect tissues in different species, including humans. Reports demonstrate durations of such tranfections of at least 6 years in primates (Rivera et al., 2005), 8 years in dogs (Niemeyer et al., 2009) and more that 10 years in the human brain (Leone et al., 2012).
AAV is unique among mammalian viruses in that it integrates into a distinct region of the human chromosome 19, the so called AAV integration site AAVS1 (review in Smith, 2008). The site-specific integration of AAV requires the presence of two viral elements: the inverted terminal repeats (ITRs) and nonstructural proteins Rep78/68. Accordingly, all current AAV vectors lacking the rep gene lack the capacity for site-specific integration. Wild-type AAV sequences are integrated into the host cell genome as tandem, head-to-tail repeats linked to genomic DNA sequences by the viral inverted terminal repeat elements (or ITRs). Specificity is achieved through the interaction of a glycine-rich loop that binds the major groove and an α-helix that interacts with a downstream minor groove on the same face of the DNA. In contrast, the integration of recombinant AAV genomic sequences in the absence of the AAV Rep proteins is inefficient and is not limited to chromosome 19. It is estimated that only about 10% of recombinant AAV sequences integrate into the host cell genome, thus indicating that the majority of vector genomes persist in an extrachromosomal form in vivo (Smith, 2008).
6.4. Herpes Simplex Virus (HSV) Derived Vectors
HSV is a member of the Herpesviridae family. There are two main viral types—HSV-1 and HSV-2. While HSV-1 causes orolabial lesions and resides in the trigeminal ganglion, HSV-2 causes genital lesions and resides in the sacral ganglia. About 40% of the adult population is seropositive for HSV-1 in the developed countries (review in Kantor et al., 2014). The HSV particles have icosahedral capsid covered by a lipid bilayer envelope. The envelope includes glycoproteins, which are essential for the viral entry into the cell. The viral genome consists of dsDNA of 152 kb (see Table 5). The life cycle of HSV is particular: the cycle has two alternative phases—lytic and latent. The lytic pathway leads to viral proliferation, emission and imminent cell death, while the latent pathway causes the virus to form an episomal particle within the nucleus in a dormant state. The latent viral particle is capable of lytic transformation upon the action of physical factors (i.e., cold, heat shock etc.). Viral replication is a multistage process, controlled by many genes, which can be removed from the genetic backbone of the vector. This ensures large packaging capacity of up to 125 kbp. What makes HSV suitable for CNS and PNS applications are the following properties: (i) the wild type virus propagates trans-synaptically in both anterograde and retrograde directions; (ii) the wild type virus is neurotropic and (iii) stability of the latent phase.
Infection with HSV typically occurs at the cutaneous or mucosal epithelium where replication of the virus is initially lytic. The virus can also invade axons of sensory neurons in the affected area and undergo retrograde transport to the dorsal root ganglia where it can switch to a latent phase.
Once the virus has reached the nucleus of a cell, the linear genome circularizes and is maintained as an episome with minimal integration (Lentz et al., 2012). HSV vectors demonstrate extensive host cell range, high efficiency gene transfer, and enhanced safety, as persistence of the genome as an episome decreases the likelihood of insertional mutagenesis (Shayakhmetov et al., 2010). Due to cytotoxicity associated with viral gene expression non-replicating and amplicon vectors have been developed. HSV amplicons are eukaryotic expression vectors that harbor the HSV origin of replication and cleavage/packaging signals. HSVs do not require pseudotyping to increase neurotropism, as these viruses are inherently neurotropic. HSV-mediated gene expression has a rapid onset (< 1 day) (Penrod et al., 2015). Transgene expression mediated by HSV vectors has been demonstrated to persist for up to 7 months in the rat brain, but may not be stable (Zhang et al., 2000; Sun et al., 2003).
Replication-attenuated and replication-deficient HSV vectors have the transgene of interest inserted into the viral genome, with targeted deletion of specific immediate early (IE) genes to disable the lytic cycle and render these vectors non-toxic (Wu et al., 1996; Krisky et al., 1998).
6.5. Lentriviral Vectors
Lentivirial vectors are derived from the HIV genome and there is abundant literature about their properties and safety. Interested readers can consult the recent review of Kantor et al. (2014). Lentriviral vectors lead to permanent genetic modification, which may not be desirable in all cases. Since neurons in the majority of brain areas do not divide, there is a little justification, from medical ethics perspective, why a permanent genetic modification of the patient's brain tissue is desirable.
6.6. Gene Therapy in CNS Related to the Applications
The CNS has proven quite permissive to viral vector gene transfer and expression for many of the conventional delivery vectors. AAV, lentiviral, and to a lesser extent HSV vectors, are the most frequently utilized agents for brain and spinal cord gene delivery. At present, AAV vectors are the leading platform for gene delivery in CNS. Beyond the large number of preclinical and basic mammalian studies involving AAV delivery to the brain and/or spinal cord, the large majority (approximately 75%) of clinical trials that have been initiated for CNS gene therapy have utilized AAV, as contrasted to trials utilizing adenovirus (6%), lentivirus (12%) or retrovirus (6%) (Gray et al., 2010a).
In a recent clinical trial, 12 patients with advanced Parkinson's disease had an application of an AAV vector carrying a transgene encoding glutamic acid decarboxylase (GAD) (Kaplitt et al., 2007). The therapy was well tolerated, with no adverse effects attributable to gene therapy noted for any of the patients. Observed improvement in motor activity lasted for at least 1 year. The trial using AAV-GAD did not establish adverse effects for up to 12 months (Feigin et al., 2007). Another trial for Parkinson's disease using AAV-hAADC vector for dopamine replacement established favorable safety profile (n = 5 patients) but low efficacy (Christine et al., 2009). Similar safety profiles have been established in another trial (Muramatsu, 2010). A phase 2 study using 45 patients and AAV-GAD did not establish serious adverse affects attributed to the treatment (LeWitt et al., 2011). So-reported results demonstrate that AAV is a sufficiently safe vector for applications in the brain. In summary, the recombinant AAV vectors have emerged as a viable delivery method for human gene therapy as they can be designed to meet the precise treatment needs of a given disease by delivering a gene to specific cell types within the affected tissues with a minimal immune response.
6.7. Assessing the Risks of the Genetic Modification
Genetic modification has some inherent risks. A comprehensive treatment of the principles of risk assessment will require a dedicated publication. Interested readers can consult Baldo et al. (2013) for treatment of gene therapy cases. Briefly, the methodology of risk assessment consists of the following steps:
• hazard identification;
• hazard characterization;
• risk estimation, i.e., risk band estimation;
• evaluation of risk management options based on the assigned risk band.
Considering viral vector applications the following principal hazards can be identified:
• Immune system reaction
• Pleiotropic effects due to low specificity
• Recombination of the vector (older generation vectors)
• Insertional mutagenesis and carcinogenesis (older generation retroviral vectors)
Various candidate viral vectors are compared in Table 5, based on the studies of Doherty et al. (2011) and Howarth et al. (2010).
Transfection-related properties of different vectors are summarized in Table 6.
We can identify some desirable properties of the ideal vector, considering the specific application:
• very low insertional mutagenesis potential—due to necessity of long-term action;
• very low immunogenic potential—for the same reasons and considering the importance of chronic neuroinflammation in the pathogenesis of neurodegenerative diseases;
• neurotropism;
• low recombination potential.
The payload capacity is not a differentiating factor for optogenetics applications as the channelrhodopsins are in the range 1.7 kbp (i.e., hChR2-GFP). Based on these criteria, a preference table can be assembled (Table 7). From the table it appears that, given the present state of development of the viral vector technology, the best choice for optogenetic application in human is AAV followed by HSV.
6.8. Alternatives for Gene Delivery
Non-viral alternatives for gene delivery have been developed in parallel to viral vector technology. These are for example, transfection by electroporation (Nomura et al., 2016) or focused ultrasounds (Wang et al., 2017). Another potential alternative is laser photoporation, currently developed in vitro (Antkowiak et al., 2013). For various reasons however, it is the viral vector-based gene delivery that stands out as the most mature transfection technology.
7. Implanted Optical Brain Stimulator
Research on implantable hybrid optoelectronic probes has progressed rapidly in the last couple of years. Optical stimulation can be applied in several ways. Available techniques include fiber optics, on-probe μLED-s and waveguide-based approaches. A recent overview on the topic can be found in Iseri and Kuzum (2017). Focal optic stimulation can be achieved with proper optical design, for example using independently controllable GaN light emitting diodes (McAlinden et al., 2015). Optical brain stimulators can work with several wavelengths to activate selectively differently transfected cells within the same region (Emiliani et al., 2015); or they can be designed to provide complex illumination patterns (Segev et al., 2017).
7.1. Alternative Realization
Micro and perhaps nanotechnologies are at stake here when considering the development of the appropriate stimulation system (Pisanello et al., 2016). Multi-functional devices can combine photo-stimulation and electrical recording (Wang et al., 2012) including micro-electrode arrays placed in the vicinity of the target (Buzsaki et al., 2015; Naughton et al., 2016).
7.2. Limitations
Designing probes that can be safely inserted in the brain is a significant challenge. Generic tissue reactions to the implant device have been discussed elsewhere (Prodanov and Delbeke, 2016a). For any implanted device, power dissipation and thus local temperature must be kept at an acceptable level (Arias-Gil et al., 2016; Shin et al., 2016). The strong light power used in photogenetic could transform photochemically some cell molecules into toxic agents. Chronic aspects are important to consider: a good example is the mitochondria-mediated apoptosis induced by prolonged ChR2 activation (Perny et al., 2016). Whether electric, microfluidic or optical, any application must take into account the diffusion and depth of penetration. Although only mentioned in animals, light leaks might influence an implant (Eckmier et al., 2016), considering that near infra-red spectroscopy is a technique based on light transmission through scalp, skull and brain (Benaron et al., 2000). Optic implants are new and require a specific encapsulation still lacking chronic evaluation (Rossi et al., 2015). On the other hand, it is much easier to make optic devices Magnetic Resonance Imaging (MRI) compatible than their electrical counterparts. This is an important factor for the modern clinical practice.
7.3. Possible Applications
DBS is presently used in Parkinson's disease, epilepsy, pain, dyskinesia, tremor, dystonia, major depression and obsessive compulsive disorder (OCD). The exact mechanism of action is unknown and it is assumed that all neurons within a given volume are being activated. Because the cell type activated can be very different and sometimes non-neuronal (Nam et al., 2016), optical stimulation of the same volume might not perform an equivalent job. Epilepsy is presently the object of many attempts to apply neuro-modulation. This is justified by the large number of therapy resistant cases. However the pathophysiology of this condition remains obscure. The selectivity of optogenic stimulation might help sort out the pathophysiology and arrive at an efficient clinical treatment (Xu et al., 2016; Yekhlef et al., 2017). Also for conditions, such as retinal diseases, light stimulation would probably make the generation of meaningful visual perceptions easier in a retinal prosthesis (Nirenberg and Pandarinath, 2012; Al Atabany et al., 2013). Stimulation and sensing can be implemented exclusively with optical elements (Inagaki et al., 2017). A combination of techniques, such as electrophysiology and optogenetics (Chen et al., 2017) or micro-fluidics (Rubehn et al., 2013; McCall et al., 2017), can offer additional perspectives in feedback controlled systems.
8. Biocompatibility Issues
8.1. Gene Delivery
In a standard optogenetics protocol the channelrhodopsin gene is delivered by viral vector injection. However, as already mentioned, innate immunity and antigen-specific adaptive immune responses against vector-derived antigens could reduce the efficacy and stability of the gene transfer when this technique is translated to the clinic (for an overview, see Bessis et al., 2004; Nayak and Herzog, 2009). In the case of human gene therapy, AAV vectors are frequently put forward as optimal solutions due to their good safety profiles (Carter, 2005). Innate immune response are limited, but have been observed at high doses or with specific serotypes (Lowenstein et al., 2007; Hadaczek et al., 2009). Adaptive immune responses, on the other hand, are more common. Anti-AAV antibodies are predominantly directed at the vector's capsid protein (Mingozzi and High, 2013). Neutralizing antibodies constitute one of the main challenges of successful gene delivery, as they impact transfection efficiency in animal (Arruda et al., 2010; Haurigot et al., 2010; Jiang et al., 2013) and clinical studies (Manno et al., 2006), even at low titers (Scallan et al., 2006). Especially persons with pre-existing anti-AAV antibodies would be at a disadvantage, though there is a difference in neutralizing effect depending on the serotype (Xiao et al., 1999). Other studies in the brain (Lo et al., 1999; Mastakov et al., 2002), muscle (Kay et al., 2000) and retina (Anand et al., 2002) revealed, however, no relation between the presence of the anti-capsid antibodies and the amount of transgene expression.
At present, it is seems too early to choose between systemic vs. local viral vector application. Systemic application of viral vectors could be favorable in the case mechanisms of DBS turn out to be more delocalized. On the other hand, in this case the volume of illumination can turn out to be the performance limiting factor. Localized application by the device itself may pose conflicting engineering requirements (e.g., microfluidics), which could decrease reliability.
8.2. Expression of the Transgene
Besides vector-specific antibodies, the body might also mount an immune response against the transgene itself. Transgene-specific immune responses are dependent on the target organ, the administration route and dosage (Toromanoff et al., 2010; Mingozzi and High, 2013). Target tissue such as the liver display a high level of tolerance to the transgene product (LoDuca et al., 2009), while muscle (Ross et al., 2006) is more prone to activation of the immune system. In the case of neural tissue, opsins do not seem toxic to human neurons in the brain or retina (Busskamp et al., 2010; Valtcheva et al., 2016). However, high levels of opsin expression might still induce cell death (Klein et al., 2006), alteration in electrical membrane properties or form aggregates in neurons (Gradinaru et al., 2008; Zimmermann and Dours-Zimmermann, 2008; Diester et al., 2011), while no observable damage has been reported (Han et al., 2009). The diversity in responses points to the difficulty of determining the correct levels of expression (Allen et al., 2015; Jarvis and Schultz, 2015). Therefore, to counter potential toxicity, different modification strategies have been developed, leading to safe expression even at high titers (Gradinaru et al., 2008). Finally, as in any gene therapy case, there is a risk that the opsin might insert randomly into the genome and thereby result in oncogenesis (Hacein-bey abina et al., 2008).
8.3. Bio-Mechanical Device Compliance
The light-delivering device can either be implanted into or remain external to the brain. The necessity of opening a pathway in the first case bears the risk of tissue damage due to the insertion or chronic presence in the body as with any implantable device (for a review, see Prodanov and Delbeke, 2016a). Acute vascular damage can be controlled to some extent by the shape of the implant and the surgical trajectory; while the amount of micromotion is a function of the implant inertial properties and the mode of attachment to the skull. Both parameters are difficult to modify, once an application has been chosen. To prevent mechanical damage, the device should be as small as possible, while providing sufficient light to the target deep brain structures. For this purpose, multiple solutions have been proposed: for example, novel probe designs or adaptations of high-density fiber arrays (Zorzos et al., 2010; Han, 2012; Ozden et al., 2013; Hoffman et al., 2015).
8.4. Optical Stimulation
Light absorbed by brain tissue can produce non-specific effects due to heating or photodamage. Heating may alter the activity of neurons or even behavior (Moser and Mathiesen, 1996; Long and Fee, 2008). The risk of overheating constraints the power that can be used by the device and hence limits the illumination volume. A possible solution is to place the device further from the neurons of interest or even outside the brain and use red light in combination with red-shifted opsins (Lin et al., 2013).
Whereas most of the optogenetic protocols do not induce excessive tissue damage (Gysbrechts et al., 2016), heating effects were also observed at standard light powers (Christie et al., 2013; Stujenske et al., 2015) and even in non-transduced cells (Han, 2012). On the other hand, heat itself has been used as well to perform optical stimulation of wild-type cells, using infrared pulses (Shapiro et al., 2012; Carvalho-de Souza et al., 2015). The light might also have non-thermal effects on neural tissue. Light-sensitive pathways naturally present in cells could produce unwanted effects to both targeted and surrounding cells (Koyanagi et al., 2013; Cheng et al., 2016).
8.5. Long-Term Effects
Finally, a favorable acute response does not necessarily predict the chronic stimulation effects on neuronal tissue. Changes at single-cell and network level need to be considered when translating optogenetics to the clinic. Long-term exposure to light pulses has been known to plastically modify the behavior of transfected neurons, changing their response to stimulation (Schultheis et al., 2011; Lignani et al., 2013), or induce long-term potentiation (Zhang and Oertner, 2007). Some of these chronic effects may prove to be beneficial in a clinical context, leading for example to a reduction of the stimulation periods. On a single-cell level, changes in neuron morphology have been observed, due to the prolonged expression (Miyashita et al., 2013) or stimulation (Goold and Nicoll, 2010; Grubb and Burrone, 2010).
Four main aspects contributing to compromised long-term compliance can be outlined:
• acute vasuclar damage including hemorrhage;
• micromotion of the implant;
• localized blood-brain barrier (BBB) breakdown;
• formation of reactive oxygen species (ROS, i.e., oxidative stress).
The disruption of BBB leads to the deposition of plasma proteins foreign to the CNS, such as albumin, globulins, fibrin/fibrinogen, thrombin, plasmin, complement (Kozai et al., 2015). The vascular damage is accompanied by fluid displacement, dragging of the blood vessels and eventual hemorrhage observed after implantation (Bjornsson et al., 2006). Hemorrhages have been shown to be particularly detrimental for long term recording (Stensaas and Stensaas, 1976; Turner et al., 1999; Grand et al., 2010). The cerebral vasculature is particularly susceptible to the action of ROS, which is of great importance since cerebral endothelial cells play a major role in the creation and maintenance of BBB (review in Obermeier et al., 2013). Such BBB dysfunction can result in an imbalance of ions, transmitters and metabolic products in the interstitial fluid, causing abnormal neuronal activity. In the implantation setting ROS can be linked to the catalytic function of Fe3+ present in the blood clot, which can lead to formation of stationary diffusion-limited concentration gradients around the implant (Prodanov and Delbeke, 2016b).
9. Neuromodulation
The clinical application of optogenetic systems (i.e., optical brain stimulators) will require much more attention for the target selection, stimulus characteristics (Shiri et al., 2017; Weible et al., 2017) and therapy regimen than has been the case hitherto. Within the framework of DBS, several different neural targets are still being proposed for electrical neurostimulation. Cortical stimulation is being considered for pain (Liu and Tao, 2016) and epilepsy as well as for totally different applications such as providing sense of touch to prosthetic hands (Nghiem et al., 2015).
9.1. Critera for Clinical Acceptance
Refractory epilepsy and Parkinson's disease present different cases for an eventual application of optogenetics. While the Parkinson's disease is an established target for DBS since 1997, long-term efficacy of electrical stimulation for refractory epilepsy is still an ongoing investigation. Despite maximal antiepileptic drug therapy, more than 30% of patients with epilepsy suffer from persistent seizures, while up to 40% of patients are not candidates for surgical resection (Halpern et al., 2008). The SANTE trial3 fond out that the median percent seizure reduction from baseline at 1 year was 41%, and 69% at 5 years. The long-term follow-up of anterior thalamic nucelus (ANT) DBS showed sustained efficacy and safety in a treatment-resistant population (Salanova et al., 2015). The readers are directed to the recent review of Laxpati et al. (2014) for a summary of the clinical trials in epilepsy.
While for Parkinson's disease an optogenetic stimulator must demonstrate superiority to eventually become an accepted therapy, in epilepsy the target can be somehow lowered to non-inferiority. This indicates that optogenetic modulation of refractory epilepsy could in fact be achieved first.
9.2. Alternative Realization
Optogenetics also offers the possibility to work with cells other than neurons (Cho et al., 2016). Systems combining optic simulation and electrical recording have been developed (Wang et al., 2012; Rubehn et al., 2013; Hoffman et al., 2015, 2016; Naughton et al., 2016; Segev et al., 2017). Targets have not been limited to the brain and include the spinal cord and peripheral nerves (VNS for epilepsy and depression, motor nerves for various palsies, hypoglossal nerve for sleep apnoea and many more). For some peripheral nerves, optic stimulation could be realized without implant or transfection (Maimon et al., 2017). Alternatively, non invasive techniques are being developed with Transcranial Direct Current Stimulation (tDCS), transcutaneous VNS (tVNS), Repetitive Transcranial Magnetic Stimulation (rTMS) and others.
9.3. Limitations
Despite its acceptance, there is still much room for progress in the practice of DBS—for example, by providing bi-directional (i.e., including sensing) interfaces, which would open the path for closed-loop approaches and titration of stimulation. Neural tissue does not necessarily provide a stable response to chronic stimuli (McCreery et al., 1997). Poorly controllable brain plasticity can turn out to be an ally or an enemy in chronic applications. Along the same line, cellular biology homeostasis (Sinnen et al., 2017) should be considered. It is known that long term potentiation or depression (LTP, LTD) (Nabavi et al., 2014; Correia et al., 2017) can be induced by some stimulation conditions. Specific strategies might be essential in the control of epileptic activity (Ching and Ritt, 2013). Cell-type and even sub-cellular specificity hold the promise for real progress (Dvorzhak et al., in press) but we lack the knowledge to benefit from such developments. Also stimulus phase timing (Witt et al., 2013) can be important. Appropriate stimulation can shift the excitability of the cortex (Heitmann et al., 2017). Stimulation regimens are important (Shiri et al., 2017) but mostly established on empirical basis because of a lack of neurophysiological and pathophysiological understanding (Gradinaru et al., 2009; Dvorzhak et al., in press). Safe limits for the various stimulation parameters have not been firmly established. Despite the brain being essentially a control system, most rational explanations are still in terms of a simple balance between excitatory and inhibitory influences. Closed loop system are being presented (Grosenick et al., 2015) but they still represent “event-triggered” stimulation rather than network integrated devices.
9.4. Possible Applications
DBS is a recognized therapy in Parkinson's disease and it has also benefited some cases of major depression. An optogenetics equivalent could do equally well or perhaps even better but there is no clinical evidence yet. Present findings point to a number of pathways along which optogenetics is likely to play a role in the near future, namely in the treatment of epilepsy (Mantoan Ritter et al., 2014). With the assumption that various issues related to genetic manipulations of adult patients can be resolved, optogenetics could soon become an alternative to DBS (Karas et al., 2013) in the treatment of Parkinson's disease (Beitz, 2014) and epilepsy (see for example Boon et al., 2007; Cox et al., 2014). Many more conditions might soon enter the therapeutic perspective including Alzheimer's disease (Kastanenka et al., 2017), the cochlear prosthesis (Richardson et al., 2017), visual pathologies (Scholl et al., 2016; Sengupta et al., 2016) and perhaps cardiac (Entcheva and Bub, 2016) or other non-neural conditions.
10. Conclusions and Outlook
Presented brief overview of the perspectives of optogenetics for DBS and neuromodulation leads to the following conclusions:
On the first place, the term “optogenetics” cannot be reduced to a single method or technique but can be broken down into a number of components that could be used independently of assembled in various combinations leading to a very broad spectrum of exciting therapeutic perspectives. A bright future for such applications can be foreseen. Optogenetics thus appears as a challenge well worth the substantial research effort that it still requires.
Secondly, safety of viral vectors is not a roadblock toward human application of optogenetics. There is already sufficient experience with clinical trials and level of maturity of viral vector technology. Despite the major input of technology in the field, it also appears that a lack in fundamental knowledge remains a major obstacle to progress (Jarvis and Schultz, 2015). Investing massively in applied trials while neglecting basic science might not be the most cost-effective way on the long run. Safety, optimization and chronic applicability would surely benefit from such a basic knowledge-first based approach.
Thirdly, attempts to apply optogenetics immediately confront researchers with the large gaps in our knowledge of the brain. Clearly, trials based only on partial understanding will lead to inefficiency and increasing risks as already shown by the early failures in gene therapy. The shortest path toward successful optogenetics applications appears to be to focus on fundamental research on mechanisms of DBS.
Optogenetics itself seems to offer the necessary tools to perform the experimental brain studies that are so badly needed. Progress in the field precisely identifies the function of specific populations of neurons in a complex anatomical substrate. Chronic clinical evaluation is necessary before it can be stated that initial results will be maintained in time. Long term evolution of the therapeutic effects, however can turn out either way. It may be either favorable or leading to inefficiency and side effects.
Optogenetics can be considered as a case demonstrating the value of exploratory research. This is clearly recognized by Deisseroth (2015), who further admits that pioneering optogenetic experiments were not suitable to typical grant programs focusing on a disease state, on a translational question, or even on solidly justified basic science.
Finally, optogenetics provides a large variety of tools, which enable further fundamental research. On the other hand it also raises challenges because of its transdisciplinary character. The extraordinary therapeutic breakthroughs that can be foreseen, the sheer number of conditions for which an application seems ultimately possible and the accumulating evidence balances the hurdles described in the preceding sections.
Author Contributions
All authors listed have made a substantial, direct and intellectual contribution to the work, and approved it for publication.
Conflict of Interest Statement
The authors declare that the research was conducted in the absence of any commercial or financial relationships that could be construed as a potential conflict of interest.
The reviewer WW declared a past co-authorship with one of the authors JD to the handling Editor.
Acknowledgments
The work has been supported in part by a project grant from Research Fund—Flanders (FWO), Belgium, contract number G.0C75.13N and an individual PhD FWO fellowship to KM.
Footnotes
1. ^Conditioned on prior gene transfection by viral vectors or other means.
2. ^International Neuromodulation Society: http://www.neuromodulation.com/about-neuromodulation.
3. ^Stimulation of the Anterior Nucleus of the Thalamus for Epilepsy trial (SANTE; NCT00101933, Medtronic, Minneapolis, MN, USA).
References
Akache, B., Grimm, D., Pandey, K., Yant, S. R., Xu, H., and Kay, M. A. (2006). The 37/67-kilodalton laminin receptor is a receptor for adeno-associated virus serotypes 8, 2, 3, and 9. J. Virol. 80, 9831–9836. doi: 10.1128/JVI.00878-06
Al-Atabany, W., McGovern, B., Mehran, K., Berlinguer-Palmini, R., and Degenaar, P. (2013). A processing platform for optoelectronic/optogenetic retinal prosthesis. IEEE Trans. Biomed. Eng. 60, 781–791. doi: 10.1109/TBME.2011.2177498
Allen, B. D., Singer, A. C., and Boyden, E. S. (2015). Principles of designing interpretable optogenetic behavior experiments. Learn. Mem. 22, 232–238. doi: 10.1101/lm.038026.114
Anand, V., Duffy, B., Yang, Z., Dejneka, N. S., Maguire, A. M., and Bennett, J. (2002). A deviant immune response to viral proteins and transgene product is generated on subretinal administration of adenovirus and adeno-associated virus. Mol. Ther. 5, 125–132. doi: 10.1006/mthe.2002.0525
Antkowiak, M., Torres-Mapa, M. L., Witts, E. C., Miles, G. B., Dholakia, K., and Gunn-Moore, F. J. (2013). Fast targeted gene transfection and optogenetic modification of single neurons using femtosecond laser irradiation. Sci. Rep. 3:3281. doi: 10.1038/srep03281
Arias-Gil, G., Ohl, F. W., Takagaki, K., and Lippert, M. T. (2016). Measurement, modeling, and prediction of temperature rise due to optogenetic brain stimulation. Neurophotonics 3:045007. doi: 10.1117/1.NPh.3.4.045007
Arruda, V. R., Stedman, H. H., Haurigot, V., Buchlis, G., Baila, S., Favaro, P., et al. (2010). Peripheral transvenular delivery of adeno-associated viral vectors to skeletal muscle as a novel therapy for hemophilia B. Blood 115, 4678–4688. doi: 10.1182/blood-2009-12-261156
Baldo, A., van den Akker, E., Bergmans, H. E., Lim, F., and Pauwels, K. (2013). General considerations on the biosafety of virus-derived vectors used in gene therapy and vaccination. Curr. Gene Ther. 13, 385–394. doi: 10.2174/15665232113136660005
Beitz, J. M. (2014). Parkinson's disease: a review. Front. Biosci. (Schol Ed.) 6, 65–74. doi: 10.2741/S415
Ben-Menachem, E., and French, J. (2005). VNS Therapy versus the latest antiepileptic drug. Epileptic Disord. 7, S22–S26.
Benabid, A. L., Pollak, P., Gao, D., Hoffmann, D., Limousin, P., Gay, E., et al. (1996). Chronic electrical stimulation of the ventralis intermedius nucleus of the thalamus as a treatment of movement disorders. J. Neurosurg. 84, 203–214. doi: 10.3171/jns.1996.84.2.0203
Benaron, D. A., Hintz, S. R., Villringer, A., Boas, D., Kleinschmidt, A., Frahm, J., et al. (2000). Noninvasive functional imaging of human brain using light. J. Cereb. Blood Flow Metab. 20, 469–477. doi: 10.1097/00004647-200003000-00005
Bernstein, J. G., Han, X., Henninger, M. A., Ko, E. Y., Qian, X., Franzesi, G. T., et al. (2008). Prosthetic systems for therapeutic optical activation and silencing of genetically-targeted neurons. Proc. SPIE Int. Soc. Opt. Eng. 6854:68540H. doi: 10.1117/12.768798
Bessis, N., GarciaCozar, F. J., and Boissier, M. C. (2004). Immune responses to gene therapy vectors: influence on vector function and effector mechanisms. Gene Ther. 11(Suppl. 1):S10–S17. doi: 10.1038/sj.gt.3302364
Bhadra, N., and Peckham, P. (1997). Peripheral nerve stimulation for restoration of motor function. J. Clin. Neurophysiol. 14, 378–393. doi: 10.1097/00004691-199709000-00004
Bjornsson, C. S., Oh, S. J., Al-Kofahi, Y. A., Lim, Y. J., Smith, K. L., Turner, J. N., et al. (2006). Effects of insertion conditions on tissue strain and vascular damage during neuroprosthetic device insertion. J. Neural Eng. 3, 196–207. doi: 10.1088/1741-2560/3/3/002
Blackburn, S. D., Steadman, R. A., and Johnson, F. B. (2006). Attachment of adeno-associated virus type 3h to fibroblast growth factor receptor 1. Arch. Virol. 151, 617–623. doi: 10.1007/s00705-005-0650-6
Boon, P., De Herdt, V., Vonck, K., and Van Roost, D. (2007). Clinical experience with vagus nerve stimulation and deep brain stimulation in epilepsy. Acta Neurochirurgica. Suppl. 97, 273–280. doi: 10.1007/978-3-211-33081-4_30
Bostock, H. (1983). The strength-duration relationship for excitation of myelinated nerve: computed dependence on membrane parameters. J. Physiol. 341, 59–74. doi: 10.1113/jphysiol.1983.sp014792
Boyden, E., Eisenach, J., Greenberg, K., Horsager, A., Matteo, B., Ririe, D., et al. (2012). Methods and Compositions for Decreasing Chronic Pain. WO Patent App. No. PCT/US2011/031297.
Boyden, E. S., Zhang, F., Bamberg, E., Nagel, G., and Deisseroth, K. (2005). Millisecond-timescale, genetically targeted optical control of neural activity. Nat. Neurosci. 8, 1263–1268. doi: 10.1038/nn1525
Brechun, K. E., Arndt, K. M., and Woolley, G. A. (2016). Strategies for the photo-control of endogenous protein activity. Curr. Opin. Struct. Biol. 45, 53–58. doi: 10.1016/j.sbi.2016.11.014
Børretzen, M. N., Bjerknes, S., Sæhle, T., Skjelland, M., Skogseid, I. M., Toft, M., et al. (2014). Long-term follow-up of thalamic deep brain stimulation for essential tremor - patient satisfaction and mortality. BMC Neurol. 14:120. doi: 10.1186/1471-2377-14-120
Busskamp, V., Duebel, J., Balya, D., Fradot, M., Viney, T. J., Siegert, S., et al. (2010). Genetic reactivation of cone photoreceptors restores visual responses in retinitis pigmentosa. Science 329, 413–417. doi: 10.1126/science.1190897
Butson, C. R., Cooper, S. E., Henderson, J. M., and McIntyre, C. C. (2007). Patient-specific analysis of the volume of tissue activated during deep brain stimulation. Neuroimage 34, 661–670. doi: 10.1016/j.neuroimage.2006.09.034
Buzsáki, G., Stark, E., Berényi, A., Khodagholy, D., Kipke, D., Yoon, E., et al. (2015). Tools for probing local circuits: high-density silicon probes combined with optogenetics. Neuron 86, 92–105. doi: 10.1016/j.neuron.2015.01.028
Carter, B. J. (2005). Adeno-associated virus vectors in clinical trials. Hum. Gene Ther. 16, 541–550. doi: 10.1089/hum.2005.16.541
Carvalho-de Souza, J. L., Treger, J. S., Dang, B., Kent, S. B., Pepperberg, D. R., and Bezanilla, F. (2015). Photosensitivity of neurons enabled by cell-targeted gold nanoparticles. Neuron 86, 207–217. doi: 10.1016/j.neuron.2015.02.033
Chen, C. H., McCullagh, E. A., Pun, S. H., Mak, P. U., Vai, M. I., Mak, P. I., et al. (2017). An integrated circuit for simultaneous extracellular electrophysiology recording and optogenetic neural manipulation. IEEE Trans. Biomed. Eng. 64, 557–568. doi: 10.1109/TBME.2016.2609412
Cheng, K. P., Kiernan, E. A., Eliceiri, K. W., Williams, J. C., and Watters, J. J. (2016). Blue light modulates murine microglial gene expression in the absence of optogenetic protein expression. Sci. Rep. 6:21172. doi: 10.1038/srep21172
Chiken, S., and Nambu, A. (2016). Mechanism of deep brain stimulation: inhibition, excitation, or disruption? Neuroscientist 22, 313–322. doi: 10.1177/1073858415581986
Ching, S., and Ritt, J. (2013). Control strategies for underactuated neural ensembles driven by optogenetic stimulation. Front. Neural Circuits 7:54. doi: 10.3389/fncir.2013.00054
Cho, W. H., Barcelon, E., and Lee, S. J. (2016). Optogenetic glia manipulation: possibilities and future prospects. Exp. Neurobiol. 25, 197–204. doi: 10.5607/en.2016.25.5.197
Chow, B. Y., Han, X., Dobry, A. S., Qian, X., Chuong, A. S., Li, M., et al. (2010). High-performance genetically targetable optical neural silencing by light-driven proton pumps. Nature 463, 98–102. doi: 10.1038/nature08652
Christie, I. N., Wells, J. A., Southern, P., Marina, N., Kasparov, S., Gourine, A. V., et al. (2013). FMRI response to blue light delivery in the naïve brain: implications for combined optogenetic fMRI studies. NeuroImage 66, 634–641. doi: 10.1016/j.neuroimage.2012.10.074
Christine, C. W., Starr, P. A., Larson, P. S., Eberling, J. L., Jagust, W. J., Hawkins, R. A., et al. (2009). Safety and tolerability of putaminal aadc gene therapy for parkinson disease. Neurology 73, 1662–1669. doi: 10.1212/WNL.0b013e3181c29356
Collins, M., and Thrasher, A. (2015). Gene therapy: progress and predictions. Proc. Biol. Sci. 282:20143003. doi: 10.1098/rspb.2014.3003
Conti, R., Assayag, O., de, V. S., Guillon, M., and Emiliani, V. (2016). Computer generated holography with intensity-graded patterns. Front. Cell. Neurosci. 10:236. doi: 10.3389/fncel.2016.00236
Corlan, A. D. (2004). Medline Trend: Automated Yearly Statistics of PubMed Results for Any Query. Available online at: http://dan.corlan.net/medline-trend.html
Correia, P. A., Lottem, E., Banerjee, D., Machado, A. S., Carey, M. R., and Mainen, Z. F. (2017). Transient inhibition and long-term facilitation of locomotion by phasic optogenetic activation of serotonin neurons. eLife 6:e20975. doi: 10.7554/eLife.20975
Cox, J. H., Seri, S., and Cavanna, A. E. (2014). Clinical utility of implantable neurostimulation devices as adjunctive treatment of uncontrolled seizures. Neuropsychiatr. Dis. Treatment 10, 2191–2200. doi: 10.2147/NDT.S60854
Dawydow, A., Gueta, R., Ljaschenko, D., Ullrich, S., Hermann, M., Ehmann, N., et al. (2014). Channelrhodopsin-2-XXL, a powerful optogenetic tool for low-light applications. Proc. Natl. Acad. Sci. U.S.A. 111, 13972–13977. doi: 10.1073/pnas.1408269111
Deisseroth, K. (2015). Optogenetics: 10 years of microbial opsins in neuroscience. Nat. Neurosci. 18, 1213–1225. doi: 10.1038/nn.4091
Deisseroth, K., Delp, S., Llewellyn, M., and Payne, C. (2016). Materials and Approaches for Optical Stimulation of the Peripheral Nervous System. US Patent 9,308,392.
Deisseroth, K., Schneider, M., and Mishelevich, D. (2015). System for Optical Stimulation of Target Cells. US Patent 8,926,959.
Deisseroth, K., Yizhar, O., Gunaydin, L., Hegemann, P., and Berndt, A. (2014). Optically-Based Stimulation of Target Cells and Modifications Thereto. US Patent 8,716,447.
Di Pasquale, G., Davidson, B. L., Stein, C. S., Martins, I., Scudiero, D., Monks, A., et al. (2003). Identification of pdgfr as a receptor for aav-5 transduction. Nat. Med. 9, 1306–1312. doi: 10.1038/nm929
Diester, I., Kaufman, M. T., Mogri, M., Pashaie, R., Goo, W., Yizhar, O., et al. (2011). An optogenetic toolbox designed for primates. Nat. Neurosci. 14, 387–397. doi: 10.1038/nn.2749
Doherty, F. C., Schaack, J. B., and Sladek, C. D. (2011). Comparison of the efficacy of four viral vectors for transducing hypothalamic magnocellular neurosecretory neurons in the rat supraoptic nucleus. J. Neurosci. Methods 197, 238–248. doi: 10.1016/j.jneumeth.2011.02.026
Dubois, B., Pillon, B., De Saxce, H., Lhermitte, F., and Agid, Y. (1986). Disappearance of parkinsonian signs after spontaneous vascular ‘thalamotomy’. Arch. Neurol. 43, 815–817. doi: 10.1001/archneur.1986.00520080057021
Dvorzhak, A., Melnick, I., and Grantyn, R. (in press). Astrocytes and presynaptic plasticity in the striatum: evidence and unanswered questions. Brain Res. Bull. doi: 10.1016/j.brainresbull.2017.01.001
Eckmier, A., Daney de Marcillac, W., Mître, A., Jay, T. M., Sanders, M. J., and Godsil, B. P. (2016). Rats can acquire conditional fear of faint light leaking through the acrylic resin used to mount fiber optic cannulas. Learn. Mem. 23, 684–688. doi: 10.1101/lm.042465.116
Emiliani, V., Cohen, A. E., Deisseroth, K., and Häusser, M. (2015). All-optical interrogation of neural circuits. J. Neurosci. 35, 13917–13926. doi: 10.1523/JNEUROSCI.2916-15.2015
Entcheva, E., and Bub, G. (2016). All-optical control of cardiac excitation: combined high-resolution optogenetic actuation and optical mapping. J. Physiol. 594, 2503–2510. doi: 10.1113/JP271559
Enterina, J. R., Wu, L., and Campbell, R. E. (2015). Emerging fluorescent protein technologies. Curr. Opin. Chem. Biol. 27, 10–17. doi: 10.1016/j.cbpa.2015.05.001
F. Hernández, L., Castela, I., Ruiz-DeDiego, I., Obeso, J. A., and Moratalla, R. (2017). Striatal activation by optogenetics induces dyskinesias in the 6-hydroxydopamine rat model of parkinson disease. Mov. Disord. 32, 530–537. doi: 10.1002/mds.26947
Feigin, A., Kaplitt, M. G., Tang, C., Lin, T., Mattis, P., Dhawan, V., et al. (2007). Modulation of metabolic brain networks after subthalamic gene therapy for parkinson's disease. Proc. Natl. Acad. Sci. U.S.A. 104, 19559–19564. doi: 10.1073/pnas.0706006104
François, A., Low, S. A., Sypek, E. I., Christensen, A. J., Sotoudeh, C., Beier, K. T., et al. (2017). A brainstem-spinal cord inhibitory circuit for mechanical pain modulation by GABA and enkephalins. Neuron 93, 822–839.e6. doi: 10.1016/j.neuron.2017.01.008
French, J. A. (2006). Refractory epilepsy: one size does not fit all. Epilepsy Curr. 6, 177–180. doi: 10.1111/j.1535-7511.2006.00137.x
Goold, C. P., and Nicoll, R. A. (2010). Single-cell optogenetic excitation drives homeostatic synaptic depression. Neuron 68, 512–528. doi: 10.1016/j.neuron.2010.09.020
Gradinaru, V., Mogri, M., Thompson, K. R., Henderson, J. M., and Deisseroth, K. (2009). Optical deconstruction of parkinsonian neural circuitry. Science 324, 354–359. doi: 10.1126/science.1167093
Gradinaru, V., Thompson, K. R., and Deisseroth, K. (2008). eNpHR: a natronomonas halorhodopsin enhanced for optogenetic applications. Brain Cell Biol. 36, 129–139. doi: 10.1007/s11068-008-9027-6
Grand, L., Wittner, L., Herwik, S., Göthelid, E., Ruther, P., Oscarsson, S., et al. (2010). Short and long term biocompatibility of neuroprobes silicon probes. J. Neurosci. Methods 189, 216–229. doi: 10.1016/j.jneumeth.2010.04.009
Gray, S. J., Blake, B. L., Criswell, H. E., Nicolson, S. C., Samulski, R. J., McCown, T. J., et al. (2010a). Directed evolution of a novel adeno-associated virus (aav) vector that crosses the seizure-compromised blood-brain barrier (bbb). Mol. Ther. 18, 570–578. doi: 10.1038/mt.2009.292
Gray, S. J., Woodard, K. T., and Samulski, R. J. (2010b). Viral vectors and delivery strategies for cns gene therapy. Ther. Deliv. 1, 517–534. doi: 10.4155/tde.10.50
Greenberg, B. D., Gabriels, L. A., Malone, D. Jr., Rezai, A. R., Friehs, G. M., Okun, M. S., et al. (2010). Deep brain stimulation of the ventral internal capsule/ventral striatum for obsessive-compulsive disorder: worldwide experience. Mol. Psychiatry 15, 64–79. doi: 10.1038/mp.2008.55
Grill, W. M., Norman, S. E., and Bellamkonda, R. V. (2009). Implanted neural interfaces: biochallenges and engineered solutions. Ann. Rev. Biomed. Eng. 11, 1–24. doi: 10.1146/annurev-bioeng-061008-124927
Grosenick, L., Marshel, J. H., and Deisseroth, K. (2015). Closed-loop and activity-guided optogenetic control. Neuron 86, 106–139. doi: 10.1016/j.neuron.2015.03.034
Grossman, N., Nikolic, K., Toumazou, C., and Degenaar, P. (2011). Modeling study of the light stimulation of a neuron cell with channelrhodopsin-2 mutants. IEEE Trans. Biomed. Eng. 58, 1742–1751. doi: 10.1109/TBME.2011.2114883
Grubb, M. S., and Burrone, J. (2010). Activity-dependent relocation of the axon initial segment fine-tunes neuronal excitability. Nature 465, 1070–1074. doi: 10.1038/nature09160
Gysbrechts, B., Wang, L., Trong, N. N. D., Cabral, H., Navratilova, Z., Battaglia, F., et al. (2016). Light distribution and thermal effects in the rat brain under optogenetic stimulation. J. Biophoton. 9, 576–585. doi: 10.1002/jbio.201500106
Hacein-bey abina, S., Garrigue, A., Wang, G. P., Soulier, J., Lim, A., Morillon, E., et al. (2008). Insertional oncogenesis in 4 patients after retrovirus-mediated gene therapy of SCID-X1. J. Clin. Invest. 118, 3132–3142. doi: 10.1172/JCI35700
Hadaczek, P., Forsayeth, J., Mirek, H., Munson, K., Bringas, J., Pivirotto, P., et al. (2009). Transduction of nonhuman primate brain with adeno-associated virus serotype 1: vector trafficking and immune response. Hum. Gene Ther. 20, 225–237. doi: 10.1089/hum.2008.151
Halpern, C. H., Samadani, U., Litt, B., Jaggi, J. L., and Baltuch, G. H. (2008). Deep brain stimulation for epilepsy. Neurotherapeutics 5, 59–67. doi: 10.1016/j.nurt.2007.10.065
Han, X. (2012). In vivo application of optogenetics for neural circuit analysis. ACS Chem. Neurosci. 3, 577–584. doi: 10.1021/cn300065j
Han, X., Qian, X., Bernstein, J. G., Zhou, H. H., Franzesi, G. T., Stern, P., et al. (2009). Millisecond-timescale optical control of neural dynamics in the nonhuman primate brain. Ne 62, 191–198. doi: 10.1016/j.neuron.2009.03.011
Harui, A., Suzuki, S., Kochanek, S., and Mitani, K. (1999). Frequency and stability of chromosomal integration of adenovirus vectors. J. Virol. 73, 6141–6146.
Haurigot, V., Mingozzi, F., Buchlis, G., Hui, D. J., Chen, Y., Basner-Tschakarjan, E., et al. (2010). Safety of AAV factor IX peripheral transvenular gene delivery to muscle in hemophilia B dogs. Mol. Ther. 18, 1318–1329. doi: 10.1038/mt.2010.73
Heitmann, S., Rule, M., Truccolo, W., and Ermentrout, B. (2017). Optogenetic stimulation shifts the excitability of cerebral cortex from type I to type II: oscillation onset and wave propagation. PLoS Comput. Biol. 13:e1005349. doi: 10.1371/journal.pcbi.1005349
Hoffman, L., Subramanian, A., Helin, P., Bois, B. D., Baets, R., Dorpe, P. V., et al. (2016). Low loss CMOS-compatible PECVD silicon nitride waveguides and grating couplers for blue light optogenetic applications. IEEE Photon. J. 8, 1–11. doi: 10.1109/JPHOT.2016.2601782
Hoffman, L., Welkenhuysen, M., Andrei, A., Musa, S., Luo, Z., Libbrecht, S., et al. (2015). “High-density optrode-electrode neural probe using SixNy photonics for in vivo optogenetics,” in 2015 IEEE International Electron Devices Meeting (IEDM) (Washington, DC: Institute of Electrical and Electronics Engineers (IEEE)). doi: 10.1109/IEDM.2015.7409795
Howarth, J. L., Lee, Y. B., and Uney, J. B. (2010). Using viral vectors as gene transfer tools (cell biology and toxicology special issue: Etcs-uk 1 day meeting on genetic manipulation of cells). Cell Biol. Toxicol. 26, 1–20. doi: 10.1007/s10565-009-9139-5
Inagaki, S., Tsutsui, H., Suzuki, K., Agetsuma, M., Arai, Y., Jinno, Y., et al. (2017). Genetically encoded bioluminescent voltage indicator for multi-purpose use in wide range of bioimaging. Sci. Rep. 7:42398. doi: 10.1038/srep42398
Iseri, E., and Kuzum, D. (2017). Implantable optoelectronic probes for in vivo optogenetics. J. Neural Eng. 14:031001. doi: 10.1088/1741-2552/aa60b3
Jarvis, S., and Schultz, S. (2015). Prospects for optogenetic augmentation of brain function. Front. Syst. Neurosci. 9:157. doi: 10.3389/fnsys.2015.00157
Jiang, H., Couto, L. B., Patarroyo-white, S., Liu, T., Nagy, D., Vargas J, A., et al. (2013). Effects of transient immunosuppression on adenoassociated, Effects of transient immunosuppression on adenoassociated, virus-mediated, liver-directed gene transfer in rhesus macaques and implications for human gene therapy. Blood 108, 3321–3328. doi: 10.1182/blood-2006-04-017913
Johnson, M. D., Miocinovic, S., McIntyre, C. C., and Vitek, J. L. (2008). Mechanisms and targets of deep brain stimulation in movement disorders. Neurotherapeutics 5, 294–308. doi: 10.1016/j.nurt.2008.01.010
Kaludov, N., Brown, K. E., Walters, R. W., Zabner, J., and Chiorini, J. A. (2001). Adeno-associated virus serotype 4 (aav4) and aav5 both require sialic acid binding for hemagglutination and efficient transduction but differ in sialic acid linkage specificity. J. Virol. 75, 6884–6893. doi: 10.1128/JVI.75.15.6884-6893.2001
Kantor, B., Bailey, R. M., Wimberly, K., Kalburgi, S. N., and Gray, S. J. (2014). Methods for gene transfer to the central nervous system. Adv. Genet. 87, 125–197. doi: 10.1016/B978-0-12-800149-3.00003-2
Kaplitt, M. G., Feigin, A., Tang, C., Fitzsimons, H. L., Mattis, P., Lawlor, P. A., et al. (2007). Safety and tolerability of gene therapy with an adeno-associated virus (aav) borne gad gene for parkinson's disease: an open label, phase i trial. Lancet 369, 2097–2105. doi: 10.1016/S0140-6736(07)60982-9
Karas, P. J., Mikell, C. B., Christian, E., Liker, M. A., and Sheth, S. A. (2013). Deep brain stimulation: a mechanistic and clinical update. Neurosurg. Focus 35:E1. doi: 10.3171/2013.9.FOCUS13383
Kastanenka, K. V., Hou, S. S., Shakerdge, N., Logan, R., Feng, D., Wegmann, S., et al. (2017). Optogenetic restoration of disrupted slow oscillations halts amyloid deposition and restores calcium homeostasis in an animal model of Alzheimer's disease. PLoS ONE 12:e0170275. doi: 10.1371/journal.pone.0170275
Kay, M. A., Manno, C. S., Ragni, M. V., Larson, P. J., Couto, L. B., McClelland, A., et al. (2000). Evidence for gene transfer and expression of factor IX in haemophilia B patients treated with an AAV vector. Nat. Genet. 24, 257–261. doi: 10.1038/73464
Klapper, S. D., Swiersy, A., Bamberg, E., and Busskamp, V. (2016). Biophysical properties of optogenetic tools and their application for vision restoration approaches. Front. Syst. Neurosci. 10:74. doi: 10.3389/fnsys.2016.00074
Klein, R. L., Dayton, R. D., Leidenheimer, N. J., Jansen, K., Golde, T. E., and Zweig, R. M. (2006). Efficient neuronal gene transfer with AAV8 leads to neurotoxic levels of tau or green fluorescent proteins. Mol. Ther. 13, 517–527. doi: 10.1016/j.ymthe.2005.10.008
Kleinlogel, S. (2016). Optogenetic user's guide to Opto-GPCRs. Front. Biosci. (Landmark Edn.) 21, 794–805. doi: 10.2741/4421
Kochanek, S., Schiedner, G., and Volpers, C. (2001). High-capacity ‘gutless’ adenoviral vectors. Curr. Opin. Mol. Ther. 3, 454–463.
Kovács, A., and Pál, B. (2017). Astrocyte-dependent slow inward currents (SICs) participate in neuromodulatory mechanisms in the pedunculopontine nucleus (PPN). Front. Cell. Neurosci. 11:16. doi: 10.3389/fncel.2017.00016
Koyanagi, M., Takada, E., Nagata, T., Tsukamoto, H., and Terakita, A. (2013). Homologs of vertebrate Opn3 potentially serve as a light sensor in nonphotoreceptive tissue. Proc. Natl. Acad. Sci. U.S.A. 110, 4998–5003. doi: 10.1073/pnas.1219416110
Kozai, T. D., Jaquins-Gerstl, A. S., Vazquez, A. L., Michael, A. C., and Cui, X. T. (2015). Brain tissue responses to neural implants impact signal sensitivity and intervention strategies. ACS Chem. Neurosci. 6, 48–67. doi: 10.1021/cn500256e
Krisky, D. M., Wolfe, D., Goins, W. F., Marconi, P. C., Ramakrishnan, R., Mata, M., et al. (1998). Deletion of multiple immediate-early genes from herpes simplex virus reduces cytotoxicity and permits long-term gene expression in neurons. Gene Ther. 5, 1593–1603. doi: 10.1038/sj.gt.3300766
Krook-Magnuson, E., Armstrong, C., Oijala, M., and Soltesz, I. (2013). On-demand optogenetic control of spontaneous seizures in temporal lobe epilepsy. Nat. Commun. 4:1376. doi: 10.1038/ncomms2376
Kuncel, A. M., Cooper, S. E., Wolgamuth, B. R., Clyde, M. A., Snyder, S. A., Montgomery, E. B., et al. (2006). Clinical response to varying the stimulus parameters in deep brain stimulation for essential tremor. Mov. Disord. 21, 1920–1928. doi: 10.1002/mds.21087
Laxpati, N. G., Kasoff, W. S., and Gross, R. E. (2014). Deep brain stimulation for the treatment of epilepsy: circuits, targets, and trials. Neurotherapeutics 11, 508–526. doi: 10.1007/s13311-014-0279-9
Lee, H. U., Nag, S., Blasiak, A., Jin, Y., Thakor, N., and Yang, I. H. (2016). Subcellular optogenetic stimulation for activity-dependent myelination of axons in a novel microfluidic compartmentalized platform. ACS Chem. Neurosci. 7, 1317–1324. doi: 10.1021/acschemneuro.6b00157
Lehman, R. M., and Augustine, J. R. (2013). Evolution and rebirth of functional stereotaxy in the subthalamus. World Neurosurgery 80, 521–533. doi: 10.1016/j.wneu.2012.03.006
Lentz, T. B., Gray, S. J., and Samulski, R. J. (2012). Viral vectors for gene delivery to the central nervous system. Neurobiol. Dis. 48, 179–188. doi: 10.1016/j.nbd.2011.09.014
Leone, P., Shera, D., McPhee, S. W. J., Francis, J. S., Kolodny, E. H., Bilaniuk, L. T., et al. (2012). Long-term follow-up after gene therapy for canavan disease. Sci. Trans. Med. 4:165ra163. doi: 10.1126/scitranslmed.3003454
LeWitt, P. A., Rezai, A. R., Leehey, M. A., Ojemann, S. G., Flaherty, A. W., Eskandar, E. N., et al. (2011). Aav2-gad gene therapy for advanced parkinson's disease: a double-blind, sham-surgery controlled, randomised trial. Lancet. Neurol. 10, 309–319. doi: 10.1016/S1474-4422(11)70039-4
Lignani, G., Ferrea, E., Difato, F., Amarù, J., Ferroni, E., Lugarà, E., et al. (2013). Long-term optical stimulation of channelrhodopsin-expressing neurons to study network plasticity. Front. Mol. Neurosci. 6:22. doi: 10.3389/fnmol.2013.00022
Lima, S. Q., and Miesenböck, G. (2005). Remote control of behavior through genetically targeted photostimulation of neurons. Cell 121, 141–152. doi: 10.1016/j.cell.2005.02.004
Lin, J. Y., Knutsen, P. M., Muller, A., Kleinfeld, D., and Tsien, R. Y. (2013). ReaChR: a red-shifted variant of channelrhodopsin enables deep transcranial optogenetic excitation. Nat. Neurosci. 16, 1499–1508. doi: 10.1038/nn.3502
Lin, J. Y., Lin, M. Z., Steinbach, P., and Tsien, R. Y. (2009). Characterization of engineered channelrhodopsin variants with improved properties and kinetics. Biophys. J. 96, 1803–1814. doi: 10.1016/j.bpj.2008.11.034
Lin, J.-Y., Xie, C.-L., Zhang, S.-F., Yuan, W., and Liu, Z.-G. (2017). Current experimental studies of gene therapy in Parkinson's disease. Front. Aging Neurosci. 9:126. doi: 10.3389/fnagi.2017.00126
Ling, C., Lu, Y., Kalsi, J. K., Jayandharan, G. R., Li, B., Ma, W., et al. (2010). Human hepatocyte growth factor receptor is a cellular coreceptor for adeno-associated virus serotype 3. Hum. Gene Ther. 21, 1741–1747. doi: 10.1089/hum.2010.075
Liu, S., and Tao, F. (2016). Application of optogenetics-mediated motor cortex stimulation in the treatment of chronic neuropathic pain. J. Transl. Sci. 2, 286–288. doi: 10.15761/JTS.1000153
Lo, W. D., Qu, G., Sferra, T. J., Clark, R., Chen, R., and Johnson, P. R. (1999). Adeno-associated virus-mediated gene transfer to the brain: duration and modulation of expression. Hum. Gene Ther. 10, 201–213. doi: 10.1089/10430349950018995
LoDuca, P. A., Hoffman, B. E., and Herzog, R. W. (2009). Hepatic gene transfer as a means of tolerance induction to transgene products. Curr. Gene Ther. 9, 104–114. doi: 10.2174/156652309787909490
Long, M. A. and Fee, M. S. (2008). Using temperature to analyse temporal dynamics in the songbird motor pathway. Nature 456, 189–194. doi: 10.1038/nature07448
Lowenstein, P. R., Mandel, R. J., Xiong, W.-D., Kroeger, K., and Castro, M. G. (2007). Immune responses to adenovirus and adeno-associated vectors used for gene therapy of brain diseases: the role of immunological synapses in understanding the cell biology of neuroimmune interactions. Curr. Gene Ther. 7, 347–360. doi: 10.2174/156652307782151498
Lumsden, D. E., Ashmore, J., Charles-Edwards, G., Lin, J.-P., Ashkan, K., and Selway, R. (2013). Accuracy of stimulating electrode placement in paediatric pallidal deep brain stimulation for primary and secondary dystonia. Acta Neurochirurg. 155, 823–836. doi: 10.1007/s00701-013-1629-9
Mager, T., Wood, P. G., and Bamberg, E. (2017). Optogenetic control of Ca2+ and voltage-dependent large conductance (BK) potassium channels. J. Mol. Biol. 429, 911–921. doi: 10.1016/j.jmb.2017.02.004
Mahmud, M., and Vassanelli, S. (2016). Differential modulation of excitatory and inhibitory neurons during periodic stimulation. Front. Neurosci. 10:62. doi: 10.3389/fnins.2016.00062
Maimon, B. E., Zorzos, A. N., Bendell, R., Harding, A., Fahmi, M., Srinivasan, S., et al. (2017). Transdermal optogenetic peripheral nerve stimulation. J. Neural Eng. 14:034002. doi: 10.1088/1741-2552/aa5e20
Maks, C. B., Butson, C. R., Walter, B. L., Vitek, J. L., and McIntyre, C. C. (2009). Deep brain stimulation activation volumes and their association with neurophysiological mapping and therapeutic outcomes. J. Neurol. Neurosurg. Psychiatry 80, 659–666. doi: 10.1136/jnnp.2007.126219
Malyshev, A. Y., Roshchin, M. V., Smirnova, G. R., Dolgikh, D. A., Balaban, P. M., and Ostrovsky, M. A. (2017). Chloride conducting light activated channel GtACR2 can produce both cessation of firing and generation of action potentials in cortical neurons in response to light. Neurosci. Lett. 640, 76–80. doi: 10.1016/j.neulet.2017.01.026
Manickan, E., Smith, J. S., Tian, J., Eggerman, T. L., Lozier, J. N., Muller, J., et al. (2006). Rapid kupffer cell death after intravenous injection of adenovirus vectors. Mol. Ther. 13, 108–117. doi: 10.1016/j.ymthe.2005.08.007
Manno, C. S., Pierce, G. F., Arruda, V. R., Glader, B., Ragni, M., Rasko, J. J., et al. (2006). Successful transduction of liver in hemophilia by AAV-Factor IX and limitations imposed by the host immune response. Nat. Med. 12, 342–347. doi: 10.1038/nm1358
Mantoan Ritter, L., Golshani, P., Takahashi, K., Dufour, S., Valiante, T., and Kokaia, M. (2014). WONOEP appraisal: optogenetic tools to suppress seizures and explore the mechanisms of epileptogenesis. Epilepsia 55, 1693–1702. doi: 10.1111/epi.12804
Mastakov, M. Y., Baer, K., Symes, C. W., Leichtlein, C. B., Kotin, R. M., and During, M. J. (2002). Immunological aspects of recombinant adeno-associated virus delivery to the mammalian brain. J. Virol. 76, 8446–8454. doi: 10.1128/JVI.76.16.8446-8454.2002
McAlinden, N., Gu, E., Dawson, M. D., Sakata, S., and Mathieson, K. (2015). Optogenetic activation of neocortical neurons in vivo with a sapphire-based micro-scale LED probe. Front. Neural Circuits 9:25. doi: 10.3389/fncir.2015.00025
McCall, J. G., Qazi, R., Shin, G., Li, S., Ikram, M. H., Jang, K.-I., et al. (2017). Preparation and implementation of optofluidic neural probes for in vivo wireless pharmacology and optogenetics. Nat. Protoc. 12, 219–237. doi: 10.1038/nprot.2016.155
McCreery, D., Lossinsky, A., Pikov, V., and Liu, X. (2006). Microelectrode array for chronic deep-brain microstimulation and recording. IEEE Trans. Biomed. Eng. 53, 726–737. doi: 10.1109/TBME.2006.870215
McCreery, D. B., Yuen, T. G., and Agnew, W. F„ Bullara, L. A. (1997). A characterization of the effects on neuronal excitability due to prolonged microstimulation with chronically implanted microelectrodes. IEEE Trans. Biomed. Eng. 44, 931–939. doi: 10.1109/10.634645
McIntyre, C. C., and Anderson, R. W. (2016). Deep brain stimulation mechanisms: the control of network activity via neurochemistry modulation. J. Neurochem. 139, 338–345. doi: 10.1111/jnc.13649
McIntyre, C. C., and Hahn, P. J. (2010). Network perspectives on the mechanisms of deep brain stimulation. Neurobiol. Dis. 38, 329–337. doi: 10.1016/j.nbd.2009.09.022
Mingozzi, F., and High, K. A. (2013). Immune responses to aav vectors: overcoming barriers to successful gene therapy. Blood 122, 23–36. doi: 10.1182/blood-2013-01-306647
Miocinovic, S., Lempka, S. F., Russo, G. S., Maks, C. B., Butson, C. R., Sakaie, K. E., et al. (2009). Experimental and theoretical characterization of the voltage distribution generated by deep brain stimulation. Exp. Neurol. 216, 166–176. doi: 10.1016/j.expneurol.2008.11.024
Mittelstrass, J. (2011). On transdisciplinarity. Trames J. Hum. Soc. Sci. 15, 329–338. doi: 10.3176/tr.2011.4.01
Miyagawa, Y., Marino, P., Verlengia, G., Uchida, H., Goins, W. F., Yokota, S., et al. (2015). Herpes simplex viral-vector design for efficient transduction of nonneuronal cells without cytotoxicity. Proc. Natl. Acad. Sci. U.S.A. 112, E1632–E1641. doi: 10.1073/pnas.1423556112
Miyashita, T., Shao, Y. R., Chung, J., Pourzia, O., and Feldman, D. E. (2013). Long-term channelrhodopsin-2 (ChR2) expression can induce abnormal axonal morphology and targeting in cerebral cortex. Front. Neural Circuits 7:8. doi: 10.3389/fncir.2013.00008
Moro, E., Esselink, R. J., Xie, J., Hommel, M., Benabid, A. L., and Pollak, P. (2002). The impact on Parkinson's disease of electrical parameter settings in STN stimulation. Neurology 59, 706–713. doi: 10.1212/WNL.59.5.706
Moser, E. I., and Mathiesen, L. (1996). Relationship between neuronal activity and brain temperature in rats. Neuroreport 7:1876. doi: 10.1097/00001756-199607290-00038
Muramatsu, S. (2010). The current status of gene therapy for parkinson's disease. Ann. Neurosci. 17, 92–95. doi: 10.5214/ans.0972-7531.1017209
Nabavi, S., Fox, R., Proulx, C. D., Lin, J. Y., Tsien, R., and Malinow, R. (2014). Engineering a memory with LTD and LTP. Nature 511, 348–352. doi: 10.1038/nature13294
Nagel, G., Ollig, D., Fuhrmann, M., Kateriya, S., Musti, A. M., Bamberg, E., et al. (2002). Channelrhodopsin-1: a light-gated proton channel in green algae. Science 296, 2395–2398. doi: 10.1126/science.1072068
Nakano, T., Mackay, S. M., Wui Tan, E., Dani, K. M., and Wickens, J. (2016). Interfacing with neural activity via femtosecond laser stimulation of drug-encapsulating liposomal nanostructures. eNeuro 3:ENEURO.0107-16.2016. doi: 10.1523/ENEURO.0107-16.2016
Nam, Y., Kim, J.-H., Kim, J.-H., Jha, M. K., Jung, J. Y., Lee, M.-G., et al. (2016). Reversible induction of pain hypersensitivity following optogenetic stimulation of spinal astrocytes. Cell Rep. 17, 3049–3061. doi: 10.1016/j.celrep.2016.11.043
Naughton, J. R., Connolly, T., Varela, J. A., Lundberg, J., Burns, M. J., Chiles, T. C., Christianson, J., and Naughton, M. (2016). Shielded coaxial optrode arrays for neurophysiology. Front. Neurosci. 10:252. doi: 10.3389/fnins.2016.00252
Nayak, S., and Herzog, R. W. (2009). Progress and prospects: immune responses to viral vectors. Gene Ther. 17, 295–304. doi: 10.1038/gt.2009.148
Ng, R., Govindasamy, L., Gurda, B. L., McKenna, R., Kozyreva, O. G., Samulski, R. J., et al. (2010). Structural characterization of the dual glycan binding adeno-associated virus serotype 6. J. Virol. 84, 12945–12957. doi: 10.1128/JVI.01235-10
Nghiem, B. T., Sando, I. C., Gillespie, R. B., McLaughlin, B. L., Gerling, G. J., Langhals, N. B., et al. (2015). Providing a sense of touch to prosthetic hands. Plast. Reconstr. Surg. 135, 1652–1663. doi: 10.1097/PRS.0000000000001289
Niemeyer, G. P., Herzog, R. W., Mount, J., Arruda, V. R., Tillson, D. M., Hathcock, J., et al. (2009). Long-term correction of inhibitor-prone hemophilia b dogs treated with liver-directed aav2-mediated factor ix gene therapy. Blood 113, 797–806. doi: 10.1182/blood-2008-10-181479
Nirenberg, S., and Pandarinath, C. (2012). Retinal prosthetic strategy with the capacity to restore normal vision. Proc. Natl. Acad. Sci. U.S.A. 109, 15012–15017. doi: 10.1073/pnas.1207035109
Nomura, T., Nishimura, Y., Gotoh, H., and Ono, K. (2016). Rapid and efficient gene delivery into the adult mouse brain via focal electroporation. Sci. Rep. 6:29817. doi: 10.1038/srep29817
O'Neill, B., Patel, J. C., and Rice, M. E. (2017). Characterization of optically and electrically evoked dopamine release in striatal slices from digenic knock-in mice with DAT-driven expression of channelrhodopsin. ACS Chem. Neurosci. 8, 310–319. doi: 10.1021/acschemneuro.6b00300
Obermeier, B., Daneman, R., and Ransohoff, R. M. (2013). Development, maintenance and disruption of the blood-brain barrier. Nat. Med. 19, 1584–1596. doi: 10.1038/nm.3407
Olds, J., and Milner, P. (1954). Positive reinforcement produced by electrical stimulation of septal area and other regions of rat brain. J. Comp. Physiol. Psychol. 47, 419–427. doi: 10.1037/h0058775
Osborne, P. (2015). Problematizing disciplinarity, transdisciplinary problematics. Theory Cult. Soc. 32, 3–35. doi: 10.1177/0263276415592245
O'Suilleabhain, P. E., Frawley, W., Giller, C., and Dewey, R. B. (2003). Tremor response to polarity, voltage, pulsewidth and frequency of thalamic stimulation. Neurology 60, 786–790. doi: 10.1212/01.WNL.0000044156.56643.74
Ozden, I., Wang, J., Lu, Y., May, T., Lee, J., Goo, W., et al. (2013). A coaxial optrode as multifunction write-read probe for optogenetic studies in non-human primates. J. Neurosci. Methods 219, 142–154. doi: 10.1016/j.jneumeth.2013.06.011
Penrod, R. D., Wells, A. M., Carlezon, W. A., and Cowan, C. W. (2015). Use of adeno-associated and herpes simplex viral vectors for in vivo neuronal expression in mice. Curr. Protoc. Neurosci. 73, 4.37.1–4.37.31. doi: 10.1002/0471142301.ns0437s73
Perny, M., Muri, L., Dawson, H., and Kleinlogel, S. (2016). Chronic activation of the D156a point mutant of Channelrhodopsin-2 signals apoptotic cell death: the good and the bad. Cell Death Dis. 7:e2447. doi: 10.1038/cddis.2016.351
Pisanello, F., Mandelbaum, G., Pisanello, M., Oldenburg, I. A., Sileo, L., Markowitz, J. E., et al. (2017). Dynamic illumination of spatially restricted or large brain volumes via a single tapered optical fiber. Nat. Neurosci. 20, 1180–1188. doi: 10.1038/nn.4591
Pisanello, F., Sileo, L., and De Vittorio, M. (2016). Micro- and nanotechnologies for optical neural interfaces. Front. Neurosci. 10:70. doi: 10.3389/fnins.2016.00070
Prodanov, D., and Delbeke, J. (2016a). Mechanical and biological interactions of implants with the brain and their impact on implant design. Front. Neurosci. 10:11. doi: 10.3389/fnins.2016.00011
Prodanov, D., and Delbeke, J. (2016b). A model of space-fractional-order diffusion in the glial scar. J. Theor. Biol. 403, 97–109. doi: 10.1016/j.jtbi.2016.04.031
Qing, K., Mah, C., Hansen, J., Zhou, S., Dwarki, V., and Srivastava, A. (1999). Human fibroblast growth factor receptor 1 is a co-receptor for infection by adeno-associated virus 2. Nat. Med. 5, 71–77. doi: 10.1038/8526
Rabinowitz, J. E., Rolling, F., Li, C., Conrath, H., Xiao, W., Xiao, X., et al. (2002). Cross-packaging of a single adeno-associated virus (aav) type 2 vector genome into multiple aav serotypes enables transduction with broad specificity. J. Virol. 76, 791–801. doi: 10.1128/JVI.76.2.791-801.2002
Rajguru, S. M., Matic, A. I., Robinson, A. M., Fishman, A. J., Moreno, L. E., Bradley, A., et al. (2010). Optical cochlear implants: evaluation of surgical approach and laser parameters in cats. Hear. Res. 269, 102–111. doi: 10.1016/j.heares.2010.06.021
Ranck, J. B. Jr. (1975). Which elements are excited in electrical stimulation of mammalian central nervous system: a review. Brain Res. 98, 417–440. doi: 10.1016/0006-8993(75)90364-9
Richardson, R. T., Thompson, A. C., Wise, A. K., and Needham, K. (2017). Challenges for the application of optical stimulation in the cochlea for the study and treatment of hearing loss. Exp. Opin. Biol. Ther. 17, 213–223. doi: 10.1080/14712598.2017.1271870
Rivera, V. M., Gao, G.-P., Grant, R. L., Schnell, M. A., Zoltick, P. W., Rozamus, L. W., et al. (2005). Long-term pharmacologically regulated expression of erythropoietin in primates following aav-mediated gene transfer. Blood 105, 1424–1430. doi: 10.1182/blood-2004-06-2501
Ross, C. J. D., Twisk, J., Bakker, A. C., Miao, F., Verbart, D., Rip, J., et al. (2006). Correction of feline lipoprotein lipase deficiency with adeno-associated virus serotype 1-mediated gene transfer of the lipoprotein lipase S447X beneficial mutation. Hum. Gene. Ther. 17, 487–499. doi: 10.1089/hum.2006.17.487
Rossi, M. A., Go, V., Murphy, T., Fu, Q., Morizio, J., and Yin, H. (2015). A wirelessly controlled implantable LED system for deep brain optogenetic stimulation. Front. Integr. Neurosci. 9:8. doi: 10.3389/fnint.2015.00008
Rossi, P. J., Gunduz, A., Judy, J., Wilson, L., Machado, A., Giordano, J. J., et al. (2016). Proceedings of the third annual deep brain stimulation think tank: a review of emerging issues and technologies. Front. Neurosci. 10:119. doi: 10.3389/fnins.2016.00119
Rubehn, B., Wolff, S. B., Tovote, P., Luthi, A., and Stieglitz, T. (2013). A polymer-based neural microimplant for optogenetic applications: design and first in vivo study. Lab Chip. 13, 579–588. doi: 10.1039/c2lc40874k
Salanova, V., Witt, T., Worth, R., Henry, T. R., Gross, R. E., Nazzaro, J. M., et al. (2015). Long-term efficacy and safety of thalamic stimulation for drug-resistant partial epilepsy. Neurology 84, 1017–1025. doi: 10.1212/WNL.0000000000001334
Salinas, F., Rojas, V., Delgado, V., Agosin, E., and Larrondo, L. F. (2017). Optogenetic switches for light-controlled gene expression in yeast. Appl. Microb. Biotechnol. 101, 2629–2640. doi: 10.1007/s00253-017-8178-8
Scallan, C. D., Jiang, H., Liu, T., Patarroyo-White, S., Sommer, J. M., Zhou, S., et al. (2006). Human immunoglobulin inhibits liver transduction byAAV vectors at low AAV2 neutralizing titers in SCID mice. Blood 107, 1810–1817. doi: 10.1182/blood-2005-08-3229
Scholl, H. P., Strauss, R. W., Singh, M. S., Dalkara, D., Roska, B., Picaud, S., et al. (2016). Emerging therapies for inherited retinal degeneration. Sci. Transl. Med. 8:368rv6. doi: 10.1126/scitranslmed.aaf2838
Schultheis, C., Liewald, J. F., Bamberg, E., Nagel, G., and Gottschalk, A. (2011). Optogenetic long-term manipulation of behavior and animal development. PLoS ONE 6:e18766. doi: 10.1371/journal.pone.0018766
Segev, E., Reimer, J., Moreaux, L. C., Fowler, T. M., Chi, D., Sacher, W. D., et al. (2017). Patterned photostimulation via visible-wavelength photonic probes for deep brain optogenetics. Neurophotonics 4:011002. doi: 10.1117/1.NPh.4.1.011002
Sengupta, A., Chaffiol, A., Mace, E., Caplette, R., Desrosiers, M., Lampic, M., et al. (2016). Red-shifted channelrhodopsin stimulation restores light responses in blind mice, macaque retina, and human retina. EMBO Mol. Med. 8, 1248–1264. doi: 10.15252/emmm.201505699
Shapiro, M. G., Homma, K., Villarreal, S., Richter, C.-P., and Bezanilla, F. (2012). Infrared light excites cells by changing their electrical capacitance. Nat. Commun. 3:736. doi: 10.1038/ncomms1742
Shayakhmetov, D. M., Di Paolo, N. C., and Mossman, K. L. (2010). Recognition of virus infection and innate host responses to viral gene therapy vectors. Mol. Ther. 18, 1422–1429. doi: 10.1038/mt.2010.124
Shen, S., Bryant, K. D., Brown, S. M., Randell, S. H., and Asokan, A. (2011). Terminal n-linked galactose is the primary receptor for adeno-associated virus 9. J. Biol. Chem. 286, 13532–13540. doi: 10.1074/jbc.M110.210922
Shin, D., Samoilova, M., Cotic, M., Zhang, L., Brotchie, J., and Carlen, P. (2007). High frequency stimulation or elevated K+ depresses neuronal activity in the rat entopeduncular nucleus. Neuroscience 149, 68–86. doi: 10.1016/j.neuroscience.2007.06.055
Shin, Y., Yoo, M., Kim, H. S., Nam, S. K., Kim, H. I., Lee, S. K., et al. (2016). Characterization of fiber-optic light delivery and light-induced temperature changes in a rodent brain for precise optogenetic neuromodulation. Biomed. Opt. Exp. 7, 4450–4471. doi: 10.1364/BOE.7.004450
Shiri, Z., Lévesque, M., Etter, G., Manseau, F., Williams, S., and Avoli, M. (2017). Optogenetic low-frequency stimulation of specific neuronal populations abates ictogenesis. J. Neurosci. 37, 2999–3008. doi: 10.1523/JNEUROSCI.2244-16.2017
Silva, S., Basser, P. J., and Miranda, P. C. (2007). The activation function of TMS on a finite element model of a cortical sulcus. Conf. Proc. IEEE Eng. Med. Biol. Soc. 2007, 6657–6660. doi: 10.1109/IEMBS.2007.4353886
Sinnen, B. L., Bowen, A. B., Forte, J. S., Hiester, B. G., Crosby, K. C., Gibson, E. S., et al. (2017). Optogenetic control of synaptic composition and function. Neuron 93, 646–660.e5. doi: 10.1016/j.neuron.2016.12.037
Sironi, V. A. (2011). Origin and evolution of deep brain stimulation. Front. Integr. Neurosci. 5:42. doi: 10.3389/fnint.2011.00042
Smedemark-Margulies, N., and Trapani, J. (2013). Tools, methods, and applications for optophysiology in neuroscience. Front. Mol. Neurosci. 6:18. doi: 10.3389/fnmol.2013.00018
Smith, R. H. (2008). Adeno-associated virus integration: virus versus vector. Gene Ther. 15, 817–822. doi: 10.1038/gt.2008.55
Soltan, A., McGovern, B., Drakakis, E., Neil, M., Maaskant, P., Akhter, M., et al. (2017). High density, high radiance μLED matrix for optogenetic retinal prostheses and planar neural stimulation. IEEE Trans. Biomed. circuits Syst. 11, 347–359. doi: 10.1109/TBCAS.2016.2623949
Sorokin, J. M., Davidson, T. J., Frechette, E., Abramian, A. M., Deisseroth, K., et al. (2017). Bidirectional control of generalized epilepsy networks via rapid real-time switching of firing mode. Neuron 93, 194–210. doi: 10.1016/j.neuron.2016.11.026
Stark, E., Koos, T., and Buzsaki, G. (2012). Diode probes for spatiotemporal optical control of multiple neurons in freely moving animals. J. Neurophysiol. 108, 349–363. doi: 10.1152/jn.00153.2012
Starr, P. A., Vitek, J. L., and Bakay, R. A. (1998). Ablative surgery and deep brain stimulation for parkinson's disease. Neurosurgery 43, 989–1013. discussion: 1013–1015. doi: 10.1097/00006123-199811000-00001
Stensaas, S. S., and Stensaas, L. J. (1976). The reaction of the cerebral cortex to chronically implanted plastic needles. Acta Neuropathol. 35, 187–203.
Stephanova, D. I., and Bostock, H. (1995). A distributed-parameter model of the myelinated human motor nerve fibre: temporal and spatial distributions of action potentials and ionic currents. Biol. Cybern. 73, 275–280. doi: 10.1007/BF00201429
Stujenske, J. M., Spellman, T., and Gordon, J. A. (2015). Modeling the spatiotemporal dynamics of light and heat propagation for in vivo optogenetics. Cell Rep. 12, 525–534. doi: 10.1016/j.celrep.2015.06.036
Summerford, C., and Samulski, R. J. (1998). Membrane-associated heparan sulfate proteoglycan is a receptor for adeno-associated virus type 2 virions. J. Virol. 72, 1438–1445.
Sun, M., Zhang, G.-R., Kong, L., Holmes, C., Wang, X., Zhang, W., et al. (2003). Correction of a rat model of parkinson's disease by coexpression of tyrosine hydroxylase and aromatic amino acid decarboxylase from a helper virus-free herpes simplex virus type 1 vector. Hum. Gene Ther. 14, 415–424. doi: 10.1089/104303403321467180
Sutton, A. C., Yu, W., Calos, M. E., Mueller, L. E., Berk, M., Shim, J., et al. (2013). Elevated potassium provides an ionic mechanism for deep brain stimulation in the hemiparkinsonian rat. Eur. J. Neurosci. 37, 231–241. doi: 10.1111/ejn.12040
Tass, P. A., Qin, L., Hauptmann, C., Dovero, S., Bezard, E., Boraud, T., et al. (2012). Coordinated reset has sustained aftereffects in parkinsonian monkeys. Ann. Neurol. 72, 816–820. doi: 10.1002/ana.23663
Tian, J., Xu, Z., Smith, J. S., Hofherr, S. E., Barry, M. A., and Byrnes, A. P. (2009). Adenovirus activates complement by distinctly different mechanisms in vitro and in vivo: indirect complement activation by virions in vivo. J. Virol. 83, 5648–5658. doi: 10.1128/JVI.00082-09
Toromanoff, A., Adjali, O., Larcher, T., Hill, M., Guigand, L., Chenuaud, P., et al. (2010). Lack of immunotoxicity after regional intravenous (RI) delivery of rAAV to nonhuman primate skeletal muscle. Mol. Ther. 18, 151–160. doi: 10.1038/mt.2009.251
Towne, C., and Thompson, K. R. (2016). Overview on research and clinical applications of optogenetics. Curr. Protoc. Pharmacol. 75, 11.19.1–11.19.21. doi: 10.1002/cpph.13
Turner, J. N., Shain, W., Szarowski, D. H., Andersen, M., Martins, S., Isaacson, M., et al. (1999). Cerebral astrocyte response to micromachined silicon implants. Exp. Neurol. 156, 33–49. doi: 10.1006/exnr.1998.6983
Valon, L., Marín-Llauradó, A., Wyatt, T., Charras, G., and Trepat, X. (2017). Optogenetic control of cellular forces and mechanotransduction. Nat. Commun. 8:14396. doi: 10.1038/ncomms14396
Valtcheva, M., Copits, B. A., Davidson, S., Sheahan, T. D., Pullen, M. Y., McCall, J. M., et al. (2016). Surgical extraction of human dorsal root ganglia from organ donors and preparation of primary sensory neuron cultures. Nat. Protoc. 11, 1877–1888. doi: 10.1038/nprot.2016.111
Venkataramani, S., Davitt, K. M., Xu, H., Zhang, J., Song, Y. K., Connors, B. W., et al. (2007). Semiconductor ultra-violet light-emitting diodes for flash photolysis. J. Neurosci. Methods 160, 5–9. doi: 10.1016/j.jneumeth.2006.08.004
Volkmann, J., Herzog, J., Kopper, F., and Geuschl, G. (2002). Introduction to the programming of deep brain stimulators. Mov. Disord. 17 (Suppl. 3), S181–S187. doi: 10.1002/mds.10162
Walsh, A. J., Tolstykh, G. P., Martens, S., Ibey, B. L., and Beier, H. T. (2016). Action potential block in neurons by infrared light. Neurophotonics 3:040501. doi: 10.1117/1.NPh.3.4.040501
Walters, R. W., Yi, S. M., Keshavjee, S., Brown, K. E., Welsh, M. J., Chiorini, J. A., et al. (2001). Binding of adeno-associated virus type 5 to 2,3-linked sialic acid is required for gene transfer. J. Biol. Chem. 276, 20610–20616. doi: 10.1074/jbc.M101559200
Wang, H., Peca, J., Matsuzaki, M., Matsuzaki, K., Noguchi, J., Qiu, L., et al. (2007). High-speed mapping of synaptic connectivity using photostimulation in Channelrhodopsin-2 transgenic mice. Proc. Natl. Acad. Sci. U.S.A. 104, 8143–8148. doi: 10.1073/pnas.0700384104
Wang, J., Wagner, F., Borton, D. A., Zhang, J., Ozden, I., Burwell, R. D., et al. (2012). Integrated device for combined optical neuromodulation and electrical recording for chronic in vivo applications. J. Neural Eng. 9, 2–15. doi: 10.1088/1741-2560/9/1/016001
Wang, S., Kugelman, T., Buch, A., Herman, M., Han, Y., Karakatsani, M. E., et al. (2017). Non-invasive, focused ultrasound-facilitated gene delivery for optogenetics. Sci. Rep. 7:39955. doi: 10.1038/srep39955
Weible, A. P., Piscopo, D. M., Rothbart, M. K., Posner, M. I., and Niell, C. M. (2017). Rhythmic brain stimulation reduces anxiety-related behavior in a mouse model based on meditation training. Proc. Natl. Acad. Sci. U.S.A. 114, 2532–2537. doi: 10.1073/pnas.1700756114
Weitzman, M. D., and Linden, R. M. (2011). Adeno-associated virus biology. Adeno Associated Virus. 807, 1–23. doi: 10.1007/978-1-61779-370-7_1
Weller, M. L., Amornphimoltham, P., Schmidt, M., Wilson, P. A., Gutkind, J. S., and Chiorini, J. A. (2010). Epidermal growth factor receptor is a co-receptor for adeno-associated virus serotype 6. Nat. Med. 16, 662–664. doi: 10.1038/nm.2145
Wietek, J., and Prigge, M. (2016). Enhancing channelrhodopsins: an overview. Methods Mol. Biol. 1408, 141–165. doi: 10.1007/978-1-4939-3512-3_10
Witt, A., Palmigiano, A., Neef, A., El Hady, A., Wolf, F., and Battaglia, D. (2013). Controlling the oscillation phase through precisely timed closed-loop optogenetic stimulation: a computational study. Front. Neural Circuits 7:49. doi: 10.3389/fncir.2013.00049
Wu, N., Watkins, S. C., Schaffer, P. A., and DeLuca, N. A. (1996). Prolonged gene expression and cell survival after infection by a herpes simplex virus mutant defective in the immediate-early genes encoding icp4, icp27, and icp22. J. Virol. 70, 6358–6369.
Wu, Z., Miller, E., Agbandje-McKenna, M., and Samulski, R. J. (2006). Alpha2,3 and alpha2,6 n-linked sialic acids facilitate efficient binding and transduction by adeno-associated virus types 1 and 6. J. Virol. 80, 9093–9103. doi: 10.1128/JVI.00895-06
Wykes, R. C., Kullmann, D. M., Pavlov, I., and Magloire, V. (2016). Optogenetic approaches to treat epilepsy. J. Neuroscie. Methods 260, 215–220. doi: 10.1016/j.jneumeth.2015.06.004
Xiao, W., Chirmule, N., Berta, S. C., McCullough, B., Gao, G., and Wilson, J. M. (1999). Gene therapy vectors based on adeno-associated virus type 1. J. Virol. 73, 3994–4003.
Xu, Z., Smith, J. S., Tian, J., and Byrnes, A. P. (2010). Induction of shock after intravenous injection of adenovirus vectors: a critical role for platelet-activating factor. Mol. Ther. 18, 609–616. doi: 10.1038/mt.2009.279
Xu, Z., Wang, Y., Chen, B., Xu, C., Wu, X., Wang, Y., et al. (2016). Entorhinal principal neurons mediate brain-stimulation treatments for epilepsy. EBioMedicine 14, 148–160. doi: 10.1016/j.ebiom.2016.11.027
Yang, J., Cumberbatch, D., Centanni, S., Shi, S. Q., Winder, D., Webb, D., et al. (2016). Coupling optogenetic stimulation with NanoLuc-based luminescence (BRET) Ca(++) sensing. Nat. Commun. 7:13268. doi: 10.1038/ncomms13268
Yekhlef, L., Breschi, G. L., and Taverna, S. (2017). Optogenetic activation of VGLUT2-expressing excitatory neurons blocks epileptic seizure-like activity in the mouse entorhinal cortex. Sci. Rep. 7:43230. doi: 10.1038/srep43230
Yizhar, O., Fenno, L. E., Davidson, T. J., Mogri, M., and Deisseroth, K. (2011). Optogenetics in neural systems. Neuron 71, 9–34. doi: 10.1016/j.neuron.2011.06.004
Yoon, H. H., Min, J., Hwang, E., Lee, C. J., Suh, J. K., Hwang, O., et al. (2016). Optogenetic inhibition of the subthalamic nucleus reduces levodopa-induced dyskinesias in a rat model of Parkinson's disease. Stereotact. Funct. Neurosurg. 94, 41–53. doi: 10.1159/000442891
Yoon, H. H., Park, J. H., Kim, Y. H., Min, J., Hwang, E., Lee, C. J., et al. (2014). Optogenetic inactivation of the subthalamic nucleus improves forelimb akinesia in a rat model of Parkinson disease. Neurosurgery 74, 533–541. doi: 10.1227/NEU.0000000000000297
Yun, S. H., and Kwok, S. J. J. (2017). Light in diagnosis, therapy and surgery. Nat. Biomed. Eng. 1:0008.
Zhang, G. R., Wang, X., Yang, T., Sun, M., Zhang, W., Wang, Y., et al. (2000). A tyrosine hydroxylase-neurofilament chimeric promoter enhances long-term expression in rat forebrain neurons from helper virus-free hsv-1 vectors. Brain Res. 84, 17–31. doi: 10.1016/S0169-328X(00)00197-2
Zhang, Y.-P., and Oertner, T. G. (2007). Optical induction of synaptic plasticity using a light-sensitive channel. Nat. Methods 4, 139–141. doi: 10.1038/nmeth988
Zimmermann, D. R., and Dours-Zimmermann, M. T. (2008). Extracellular matrix of the central nervous system: from neglect to challenge. Histochem. Cell Biol. 130, 635–653. doi: 10.1007/s00418-008-0485-9
Zorzos, A. N., Boyden, E. S., and Fonstad, C. G. (2010). Multiwaveguide implantable probe for light delivery to sets of distributed brain targets. Opt. Lett. 35, 4133–4135. doi: 10.1364/OL.35.004133
Keywords: viral vectors, biosafety, optogoenetics, deep brain stimulation, neural prosthesis, Parkinson's disease, epilepsy, neuromodulation
Citation: Delbeke J, Hoffman L, Mols K, Braeken D and Prodanov D (2017) And Then There Was Light: Perspectives of Optogenetics for Deep Brain Stimulation and Neuromodulation. Front. Neurosci. 11:663. doi: 10.3389/fnins.2017.00663
Received: 31 May 2017; Accepted: 14 November 2017;
Published: 12 December 2017.
Edited by:
Diana Deca, Norwegian University of Science and Technology, NorwayReviewed by:
Stefano Vassanelli, Università degli Studi di Padova, ItalyAnton Ilango, University of Magdeburg, Germany
Copyright © 2017 Delbeke, Hoffman, Mols, Braeken and Prodanov. This is an open-access article distributed under the terms of the Creative Commons Attribution License (CC BY). The use, distribution or reproduction in other forums is permitted, provided the original author(s) or licensor are credited and that the original publication in this journal is cited, in accordance with accepted academic practice. No use, distribution or reproduction is permitted which does not comply with these terms.
*Correspondence: Dimiter Prodanov, ZGltaXRlcnBwQGdtYWlsLmNvbQ==; RGltaXRlci5Qcm9kYW5vdkBpbWVjLmJl