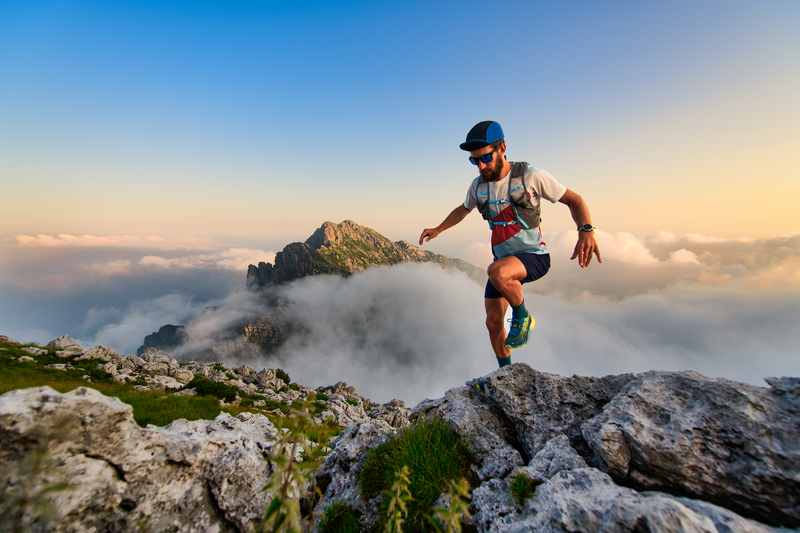
95% of researchers rate our articles as excellent or good
Learn more about the work of our research integrity team to safeguard the quality of each article we publish.
Find out more
MINI REVIEW article
Front. Neurosci. , 21 November 2017
Sec. Decision Neuroscience
Volume 11 - 2017 | https://doi.org/10.3389/fnins.2017.00631
This article is part of the Research Topic Prefrontal Cortex and Executive Functions View all 19 articles
Influences between time and space can be found in our daily life in which we are surrounded by numerous spatial metaphors to refer to time. For instance, when we move files from one folder to another in our computer a horizontal line that grows from left to right informs us about the elapsed and remaining time to finish the procedure and, similarly, in our communication we use several spatial terms to refer to time. Although with some differences in the degree of interference, not only space has an influence on time but both magnitudes influence each other. Indeed, since our childhood our estimations of time are influenced by space even when space should be irrelevant and the same occurs when estimating space with time as distractor. Such interference between magnitudes has also been observed in monkeys even if they do not use language or computers, suggesting that the two magnitudes are tightly coupled beyond communication and technology. Imaging and lesion studies have indicated that same brain areas are involved during the processing of both magnitudes and have suggested that rather than coding the specific magnitude itself the brain represents them as abstract concepts. Recent neurophysiological studies in prefrontal cortex, however, have shown that the coding of absolute and relative space and time in this area is realized by independent groups of neurons. Interestingly, instead, a high overlap was observed in this same area in the coding of goal choices across tasks. These results suggest that rather than during perception or estimation of space and time the interference between the two magnitudes might occur, at least in the prefrontal cortex, in a subsequent phase in which the goal has to be chosen or the response provided.
Einstein's Theory of Relativity postulates that time and space are tightly coupled. This is, time passes in the same way for all non-accelerating observers, but differently for observers traveling at different speeds. Inspired by further questions formulated by Einstein, Piaget conducted a series of experiments about time and space with children. These experiments led him to claim that “time and space form an inseparable whole” (Piaget, 1927), although with some asymmetries in the way that one magnitude influences the other. Such asymmetries can be found in language (Clark, 1973; Traugott, 1978; Alverson, 1994) and co-speech gestures (Núñez et al., 2012). For instance, when using language, it is common to use words or concepts borrowed from the spatial domain. In English, expressions such as “long”, “short”, “move forward,” or “ahead of” are often used to refer to time. Moreover, children learn first spatial than temporal terms and indeed metaphorical expressions of spatial terms are used as temporal terms (Clark, 1973). Such metaphoric manner in which spatial terms are used to refer to time is not only found in linguistics but also in the graphical representation of time. In other words, specific spatial locations relative to a reference point are generally used to indicate either “past” or “future” events (Boroditsky, 2000; Torralbo et al., 2006). These findings have led to metaphorical theories of mental representation that propose that time and space influence each other in an asymmetrical way (Lakoff and Johnson, 1999).
Recent studies have investigated whether the dependency between the two magnitudes also exists when we merely think about them. Indeed, it has been observed that the link between time and space is not uniquely constraint to communication but it is also present when estimating duration and dimension of different stimuli (Xuan et al., 2007; Casasanto and Boroditsky, 2008) or when estimating the passage of time in spatial environments of different sizes (De Long, 1981; Mitchell and Davis, 1987). Moreover, brain lesions affecting spatial processing also influence time estimation (Basso et al., 1996) whereas, on the contrary, people with synesthesia exhibit enhanced magnitude processing (Teuscher et al., 2010; Cohen Kadosh et al., 2011). In addition, the discrimination of time, space, and other magnitudes, obeys Weber-Fechner's law (Stevens and Greenbaum, 1966; Teghtsoonian and Teghtsoonian, 1978) according to which the perceived change in the magnitude of a stimulus is proportional to its initial value of magnitude. Based on this experimental evidence, a Theory of Magnitude (ATOM) proposes that a general neural mechanism is responsible for the computation of magnitudes (Walsh, 2003). According to ATOM, neurons encode, for instance, time and space in an abstract manner independently of the specific magnitude. Besides behavioral evidence, this theory has been also supported by lesion studies showing time and space processing impairments (Critchley, 1953; Basso et al., 1996). However, more recently, independent groups of neurons with a domain-specific coding of magnitude have been found in the prefrontal cortex (Genovesio et al., 2012; Marcos et al., 2017).
We use words from concrete domains to refer and to think about abstract domains because concrete domains are the ones that we can really experience. Such cross-domain use of semantic terms can be found in our language and gestures when we refer to love or time among other abstract concepts (Lakoff and Johnson, 1980; Lakoff and Kovecses, 1987; McNeill, 1992). For instance, metaphoric expressions such as, “Winter is coming” (see Games of Thrones) or “The trip was short” are often used to express temporal concepts and they shape our idea of time through spatial metaphors. This occurs because we can perceive the concrete but only imagine the abstract (Evans, 2004). For instance, through experience, we learn about the spatial domain because we can perceive the distance between two points or the relative position of one object respect to another. Then, we import terms and schemas from this domain to structure our idea of time and to materialize what we have learned, also through experience, about time, such as, that time always moves forward and that all days have a beginning and an end.
Using non-linguistic tasks, psychophysics experiments have examined the influence that time and space have on each other (Casasanto and Boroditsky, 2008; Casasanto et al., 2010; Merritt et al., 2010; Mendez et al., 2011). Casasanto and Boroditsky (2008) tested the ability of humans to reproduce either the duration or the spatial displacement of lines or dots displayed on a screen. Both magnitudes were always presented and one of them served as the testing variable while the other was simply a distractor. Participants' estimations of duration were influenced by the spatial displacement but not vice versa. Moreover, the effect was not related to a difference in accuracy between duration or displacement estimates and was also observed when participants were given prior knowledge about the magnitude to attend to. Interestingly, spatial influence on duration estimates was sensory independent and also present when the duration of the stimuli was provided through visual and auditory modalities. Thus, the effect of space on time was robust across the different experimental manipulations. Moreover, similar to linguistic metaphors, the results suggest that mental representations of time rely on mental representations of space.
A subsequent study examined whether the mental representation of time in adults emerges after cognitive development or whether it already exists in young children (Casasanto et al., 2010). To do that, the authors ran a series of experiments in children aged from 4 to 6 and from 9 to 10 years old. The experiments were analogous to those in adults (Casasanto and Boroditsky, 2008). Although children's ability to judge temporal and space magnitudes was equivalent they were more influenced by the spatial components during temporal estimations than vice versa. This result confirms that children exhibit the same asymmetry found in adults for estimations of time and space. Therefore, together with previous studies, the dependency between time and space in non-linguistic tasks in humans seems to be asymmetrical, similar to the influence observed during communication.
Some behavioral mechanisms are common to several magnitude estimations. For instance, independently of whether subjects are estimating time, distance, or length, there is a tendency, that increases for large sample ranges, to estimate values which are biased toward the mean of the distribution (Hollingworth, 1910; Stevens and Greenbaum, 1966; Teghtsoonian and Teghtsoonian, 1978; Jazayeri and Shadlen, 2010; Petzschner and Glasauer, 2011). Bayesian models have explained such general behavioral phenomena as the result of integrating prior experience and noisy sensory information (Jazayeri and Shadlen, 2010; Petzschner and Glasauer, 2011). Likewise, magnitude sensitivity is similar in different domains using time, space, and number tasks (Hauser et al., 2000; Brannon et al., 2006; Halberda et al., 2006; Beran, 2007). Additionally, a close connection between numbers and space has been observed, in which numbers can have a continuous spatial representation organized along a “mental number line” with, at least in Western culture, small numbers on the left side of space and large numbers on the right (Restle, 1970; Dehaene et al., 1990, 2003a; Hubbard et al., 2005). Numerical processing can cause shifts in covert attention as a function of their magnitude (Casarotti et al., 2007; Dodd et al., 2008; Nicholls et al., 2008), influence motor planning leading to faster responses toward left for small numbers and toward right for big numbers (Fischer, 2003; Fischer et al., 2004) and impact high-level cognitive processes such as, non-propositional reasoning (Brunamonti et al., 2011). On the other hand, visuospatial variables, such as, spatial cueing or visual hemifield presentations, can also influence numerical comparisons (Lavidor et al., 2004; Nicholls and McIlroy, 2010). All these experimental evidence have led to the idea of ATOM that proposes that there is a common processing mechanism for magnitudes.
Functional neuroimaging, neuropsychological, and neurophysiological studies (Critchley, 1953; Basso et al., 1996; Dehaene et al., 2003a; Fias et al., 2003) have also supported the theory of a general mechanism for magnitude processing. For instance, a Positron Emission Topography (PET) study in which monkeys were trained to perform a duration discrimination task (Onoe et al., 2001) showed that the dorsolateral prefrontal cortex and the inferior parietal lobe were activated during task duration performance and that both areas are also involved in the coding of the spatial properties of a task. Another example is the neurophysiological study performed with monkeys by Leon and Shadlen (2003). In this study, the authors showed that neurons in the posterior parietal cortex, an area involved in spatial processing, modulated their activity with the monkeys' perception of elapsed time (Gottlieb et al., 1998; Colby and Goldberg, 1999; Bisley and Goldberg, 2003). Moreover, deficits in both space and time are found after parietal damage (Critchley, 1953).
Additionally, Merritt et al. (2010) investigated whether the relationship between time and space was similar in humans and in non-human primates. Two rhesus monkeys and 16 adult humans had to classify the space or duration of a line displayed on a screen as long or short. Both magnitudes were presented but humans and monkeys had to attend to only one of them while the other was irrelevant. Humans showed an asymmetrical dependency between time and space, exhibiting a higher bias in their responses when they classified line durations as short or long (Figure 1A) than when they classified line lengths (Figure 1B). On the contrary, monkeys exhibited a large effect in their behavior of both, space on time (Figure 1C) and time on space (Figure 1D). One possible explanation for the differences in behavior between humans and monkeys might be due to the language capability, which is absent in monkeys. During linguistic communication, spatial terms are often used to refer to time and that might create an asymmetrical dependency between the two magnitudes even during non-linguistic scenarios. Another possibility is that humans, but not monkeys, are exposed to spatial representations of time through different technologies, such as, the horizontal line that indicates the elapsed and remaining time of a movie displayed on a computer.
Figure 1. Humans and monkeys judgements of duration and line length (adapted from Merritt et al., 2010). (A) Humans estimations of stimulus duration (relevant variable) when crossed with three different values of line length (irrelevant variable): short (6 cm), medium (12 cm), and long (24 cm). (B) Humans estimations of line length (relevant variable) when crossed with three different values of duration (irrelevant variable): short (1,000 ms), medium (2,000 ms), and long (4,000 ms). (C) Same as in (A) but for monkeys estimations of duration. (D) Same as in (B) but for monkeys estimations of line length.
Prefrontal and posterior parietal cortices play an important role during magnitude processing (Nieder et al., 2002, 2006; Dehaene et al., 2003b, 2004; Walsh, 2003; Nieder and Miller, 2004). Both areas have been extensively studied in a variety of spatial processing tasks in monkeys (Wilson et al., 1993; Rainer et al., 1998; Colby and Goldberg, 1999; Bisley and Goldberg, 2003) and humans (Astafiev et al., 2003; Merriam et al., 2003; Orban et al., 2004), as well as in time-interval judgements experiments (Onoe et al., 2001; Leon and Shadlen, 2003; Genovesio et al., 2006). Thus, a frontoparietal network of neurons seem to be involved in the representation of temporal and spatial information (Walsh, 2003; Buhusi and Meck, 2005; Merchant et al., 2013) and, interestingly, the same magnitude across different modalities, such as, the number of visual or auditory events, seems to be represented by the same neurons in the frontal lobe (Nieder, 2017). Moreover, modulation of the neural activity associated with timing has been found in the cerebellum (Perrett, 1998), basal ganglia (Jin et al., 2009), thalamus (Tanaka, 2007), posterior parietal cortex (Leon and Shadlen, 2003), prefrontal cortex (Brody et al., 2003; Genovesio et al., 2006, 2009, 2015; Oshio et al., 2008; Marcos et al., 2017), and premotor cortex (Lucchetti and Bon, 2001; Lebedev et al., 2008; Mita et al., 2009; Merchant et al., 2011; Confais et al., 2012).
A neurophysiological study in macaques with recordings from neurons in the prefrontal cortex tested whether absolute duration and distance was coded by the same neural population (Marcos et al., 2017), testing whether a common magnitude's representation system exists in this area. Two monkeys were trained to perform two tasks: a duration and a distance discrimination task (Figure 2A). They had to select which of two stimuli, sequentially presented on a screen, had lasted longer or was presented farther from a reference point. The durations varied within the 200–1,200 ms range, in steps of 200 ms, while the range of distances changed from 8 to 48 mm, in steps of 8 mm, and were never the same for both stimuli. The performance of both monkeys was high, with a mean of 79 and 81% of correctly classified trials in the duration and the distance tasks, respectively (see also Genovesio et al., 2009, 2011 for further details). Neurons were classified based on their response during the delay period that followed S1 presentation (D1), because it is in this period when S1 duration could be determined. From the initial group of neurons recorded in the prefrontal cortex (n = 428), a total of 113 significantly represented long (1,000–1,200 ms) or short (200–400 ms) durations of S1 while 41 neurons coded long (40–48 mm) or short (8–16 mm) distances of S1. From the two groups only 13 neurons, 11.5 and 31.7% of the duration- and distance-related neurons, exhibited a significant modulation of their response for both magnitudes (Figure 2B). From this subgroup, 9 neurons showed a congruent preference across tasks, but the total number of overlapping neurons (13) was, however, not significant, indicating that the coding of duration and distance is performed independently in the prefrontal cortex. Consistent with this result, the mean firing rate of the two groups of neurons (113 and 41 neurons) showed a difference in response between preferred and non-preferred conditions for the magnitude that they significantly coded but not for the other (Figure 2C).
Figure 2. Duration and distance discrimination tasks (adapted from Marcos et al., 2017). (A) Temporal sequence of event during a trial. Each trial started with a pre-stimulus period in which monkeys were required to press and hold the central switch of the infrared array and was followed by the presentation of the first stimulus (S1). A delay period (D1) separated S1 offset from the presentation of the second stimulus (S2). After S1 offset, a second delay period (D2) preceded the reappearance of the two stimuli (targets), acting as “Go” signal, instructing the monkeys to choose the target that had either lasted longer, in the duration task, or had appeared farther from the central reference point, in the distance task. (B) Number of neurons with duration-related activity (n = 100), distance-related activity (n = 28) or duration- and distance-related activity (n = 13). (C) Mean firing rate for preferred and non-preferred conditions of the population of neurons previously classified as having duration- (Top panels) or distance-related (Bottom panels) modulation during the duration (Left panel) and distance (Right panel) tasks (*p < 0.05/24, paired-sample t-test with Bonferroni correction).
Similar to Marcos et al. (2017) and using the same experimental tasks, Genovesio et al. (2012) reported that neurons in prefrontal cortex also coded the relative duration and distance of the two stimuli in a domain-specific way. The relative magnitude was coded in the early decision period and was dissipated later. Interestingly, contrary to the high independency showed by the neurons representing the relative value of a specific magnitude, the great majority of neurons coded the non-spatial prospective goal (Rainer et al., 1999; Saito et al., 2005; Kusunoki et al., 2009)—the choice that will be subsequently selected (red or blue stimulus)—in a congruent way in both tasks. In particular, 92% of goal selective cells shared not only the same goal preference in the two tasks but also the same preference in a control matching to sample task that did not require any magnitude discrimination and that, therefore, allowed to unmistakably classify the neurons as goal selective. Consistent with the idea of goal coding, their goal selectivity persisted until the response could be provided. This result suggests that an interplay between magnitudes might occur in the prefrontal cortex at the level of goal choices rather than at the level of perceptual decisions.
Additionally, with the same dataset, Genovesio et al. (2016) found that neurons in the prefrontal cortex distinguished whether a delay interval (D1 and D2 in Figure 2A) was short or long in a context-dependent way. Therefore only a minority of the neurons coded the delays' durations in both tasks. Moreover, the authors also observed an independency in the neurons coding the first and second delay within a task showing that neurons distinguished between short and long durations not only across but also within tasks. Interestingly, when a neuron did code the elapsed duration of the two delays it did so in an uncorrelated or weakly correlated way. Such a context dependent timing representation suggest that prefrontal cortex keeps track of specific intervals. This specificity could help making foraging choices between patches of resources that take into account their relative locations, the effort to reach each patch and the relative amount of the resources (Genovesio et al., 2014). Similar high independency in the neural groups coding delays' durations was also observed for the representation of the relative duration of two stimuli (Genovesio et al., 2015). These results show a high context dependency in the representation of time in the prefrontal cortex. Rather than coding the time in an abstract manner, as a common timing mechanism, the neural representation of time in prefrontal cortex seems to be highly context dependent at the single cell level. Thus, neurons in prefrontal cortex do not only code magnitudes in an independent way but also the same magnitude in a context-specific way, supporting the hypothesis that in this area the interference between magnitudes probably occurs at the level of goal choices rather than at the level of magnitudes coding.
Several studies have shown a clear interference between estimations of space and time. Spatial metaphors about time are often encountered in our daily communication and thoughts and are present also in the technological tools that we daily use (Lakoff and Johnson, 1980; Lakoff and Kovecses, 1987; McNeill, 1992). Interestingly, such interference between the two magnitudes emerges since our childhood (Casasanto et al., 2010). The neural mechanisms of that interference are, however, not fully understood. To shed light on this issue, several studies have focused on the neural overlap between brain areas during the perception or estimation of space and time, using functional neuroimaging techniques or through lesion studies. In these cases, a high overlap in the activation of specific brain areas during the estimation of both magnitudes have been observed suggesting a fronto-parietal network of neurons processing magnitudes in a general domain (Walsh, 2003; Buhusi and Meck, 2005; Merchant et al., 2013). Moreover, lesions of some brain areas influence the estimation of both space and time leading also to the idea of a general mechanism for magnitude processing (Critchley, 1953; Basso et al., 1996). However, these approaches allow only for a low spatial and temporal resolutions. A better resolution power can be obtained using neurophysiological techniques. Indeed, recent neurophysiological studies have investigated the coding of space and time at the single-cell level (Genovesio et al., 2012; Marcos et al., 2017). Neurons in the prefrontal cortex represent the absolute and relative values of distance and duration in a domain-specific way whereas, interestingly, they code the goal choices in a general manner. This opens the question of whether, at least in prefrontal cortex, in tasks with multiple domains the interference between magnitudes occurs during their perception and/or estimation or whether it occurs only in a subsequent step when the goal has to be chosen, as suggested by Genovesio et al. (2012). We cannot rule out the possibility that other parts of the brain, such as, the parietal cortex, can have domain-general representations considering that some parietal cortex neurons represent the same rule in both spatial and numerical domains (Eiselt and Nieder, 2013).
All authors listed wrote the paper and have made a substantial, direct and intellectual contribution to the work, and approved it for publication.
This work was supported by the MIUR Grant C26A15TNWK.
The authors declare that the research was conducted in the absence of any commercial or financial relationships that could be construed as a potential conflict of interest.
Alverson, H. (1994). Semantics and Experience: Universal Metaphors of Time in English, Mandarin, Hindi, and Sesotho. Baltimore, MD: Johns Hopkins University Press.
Astafiev, S. V., Shulman, G. L., Stanley, C. M., Snyder, A. Z., Van Essen, D. C., and Corbetta, M. (2003). Functional organization of human intraparietal and frontal cortex for attending, looking, and pointing. J. Neurosci. 23, 4689–4699.
Basso, G., Nichelli, P., Frassinetti, F., and Di Pellegrino, G. (1996). Time perception in a neglected space. Neuroreport 7, 2111–2114. doi: 10.1097/00001756-199609020-00009
Beran, M. J. (2007). Rhesus monkeys (Macaca mulatta) enumerate large and small sequentially presented sets of items using analog numerical representations. J. Exp. Psychol. Anim. Behav. Process. 33, 42–54. doi: 10.1037/0097-7403.33.1.42
Bisley, J. W., and Goldberg, M. E. (2003). Neuronal activity in the lateral intraparietal area and spatial attention. Science 299, 81–86. doi: 10.1126/science.1077395
Boroditsky, L. (2000). Metaphoric structuring: understanding time through spatial metaphors. Cognition 75, 1–28. doi: 10.1016/S0010-0277(99)00073-6
Brannon, E. M., Lutz, D., and Cordes, S. (2006). The development of area discrimination and its implications for number representation in infancy. Dev. Sci. 9, 59–64. doi: 10.1111/j.1467-7687.2006.00530.x
Brody, C. D., Hernandez, A., Zainos, A., and Romo, R. (2003). Timing and neural encoding of somatosensory parametric working memory in macaque prefrontal cortex. Cereb. Cortex 13, 1196–11207. doi: 10.1093/cercor/bhg100
Brunamonti, E., Genovesio, A., Carbè, K., and Ferraina, S. (2011). Gaze modulates non-propositional reasoning: further evidence for spatial representation of reasoning premises. Neuroscience 173, 110–115. doi: 10.1016/j.neuroscience.2010.11.011
Buhusi, C. V., and Meck, W. H. (2005). What makes us tick? Functional and neural mechanisms of interval timing. Nat. Rev. Neurosci. 6, 755–765. doi: 10.1038/nrn1764
Casarotti, M., Michielin, M., Zorzi, M., and Umiltà, C. (2007). Temporal order judgment reveals how number magnitude affects visuospatial attention. Cognition 102, 101–117. doi: 10.1016/j.cognition.2006.09.001
Casasanto, D., and Boroditsky, L. (2008). Time in the mind: using space to think about time. Cognition 106, 579–593. doi: 10.1016/j.cognition.2007.03.004
Casasanto, D., Fotakopoulou, O., and Boroditsky, L. (2010). Space and time in the child's mind: evidence for a cross-dimensional asymmetry. Cogn. Sci. 34, 387–405. doi: 10.1111/j.1551-6709.2010.01094.x
Clark, H. H. (1973). “Space, time, semantics and the child,” in Cognitive Development and the Acquisition of Language, in eds T. E. Moore (New York, NY: Academic Press), 27–63.
Cohen Kadosh, R., Gertner, L., and Terhune, D. B. (2011). Exceptional abilities in the spatial representation of numbers and time: insights from synesthesia. Neuroscientist 18, 208–215. doi: 10.1177/1073858411402835
Colby, C. L., and Goldberg, M. E. (1999). Space and attention in parietal cortex. Annu. Rev. Neurosci. 22, 319–349. doi: 10.1146/annurev.neuro.22.1.319
Confais, J., Kilavik, B. E., Ponce-Alvarez, A., and Riehle, A. (2012). On the anticipatory pre-cue activity in motor cortex. J. Neurosci. 32, 15359–15368. doi: 10.1523/JNEUROSCI.1768-12.2012
De Long, A. J. (1981). Phenomenological space-time: towards an experiential relativity. Science 213, 681–683. doi: 10.1126/science.7256273
Dehaene, S., Dupoux, E., and Mehler, J. (1990). Is numerical comparison digital? Analogical and symbolic effects in two-digit number comparison. J. Exp. Psychol. Hum. Percept. Perform. 16, 626–641. doi: 10.1037/0096-1523.16.3.626
Dehaene, S., Molko, N., Cohen, L., and Wilson, A. J. (2004). Arithmetic and the brain. Curr. Opin. Neurobiol. 14, 218–224. doi: 10.1016/j.conb.2004.03.008
Dehaene, S., Piazza, M., Pinel, P., and Cohen, L. (2003a). Three parietal circuits for number processing. Cogn. Neuropsychol. 20, 487–506. doi: 10.1080/02643290244000239
Dehaene, S., Sergent, C., and Changeuz, J.-P. (2003b). A neuronal network model linking subjective reports and objective physiological data during conscious perception. Proc. Natl. Acad. Sci. U.S.A. 100, 8520–8525. doi: 10.1073/pnas.1332574100
Dodd, M. D., Van der Stigchel, S., Adil Leghari, M., Fung, and Kingstone, A. (2008). Attentional SNARC: there's something special about numbers (let us count the ways). Cognition 108, 810–818. doi: 10.1016/j.cognition.2008.04.006
Eiselt, A. K., and Nieder, A. (2013). Representation of abstract quantitative rules applied to spatial and numerical magnitudes in primate prefrontal cortex. J. Neurosci. 33, 7526–7534. doi: 10.1523/JNEUROSCI.5827-12.2013
Evans, V. (2004). The Structure of Time: Language, Meaning and Temporal Cognition. Amsterdam: John Benjamins.
Fias, W., Lammertyn, J., Reynvoet, B., Dupont, P., and Orban, G. A. (2003). Parietal representation of symbolic and nonsymbolic magnitude. J. Cogn. Neurosci. 15, 47–56. doi: 10.1162/089892903321107819
Fischer, M. H. (2003). Spatial representations in number processing:evidence from a pointing task. Vis. Cogn. 10, 493–508. doi: 10.1080/13506280244000186
Fischer, M. H., Warlop, N., Hill, R. L., and Fias, W. (2004). Oculomotor bias induced by number perception. Exp. Psychol. 51, 91–97. doi: 10.1027/1618-3169.51.2.91
Genovesio, A., Tsujimoto, S., and Wise, S. P. (2009). Feature- and order-based timing representations in the frontal cortex. Neuron 63, 254–266. doi: 10.1016/j.neuron.2009.06.018
Genovesio, A., Cirillo, R., Tsujimoto, S., Mohammad Abdellatif, S., and Wise, S. P. (2015). Automatic comparison of stimulus durations in the primate prefrontal cortex: the neural basis of across-task interference. J. Neurophysiol. 114, 48–56. doi: 10.1152/jn.00057.2015
Genovesio, A., Seitz, L. K., Tsujimoto, S., and Wise, S. P. (2016). Context-dependent duration signals in the primate prefrontal cortex. Cereb. Cortex 26, 3345–3356. doi: 10.1093/cercor/bhv156
Genovesio, A., Tsujimoto, S., and Wise, S. P. (2006). Neuronal activity related to elapsed time in prefrontal cortex. J. Neurophysiol. 95, 3281–3285. doi: 10.1152/jn.01011.2005
Genovesio, A., Tsujimoto, S., and Wise, S. P. (2011). Prefrontal cortex activity during the discrimination of relative distance. J. Neurosci. 31, 3968–3980. doi: 10.1523/JNEUROSCI.5373-10.2011
Genovesio, A., Tsujimoto, S., and Wise, S. P. (2012). Encoding goals but not abstract magnitude in the primate prefrontal cortex. Neuron 74, 656–662. doi: 10.1016/j.neuron.2012.02.023
Genovesio, A., Wise, S. P., and Passingham, R. E. (2014). Prefrontal-parietal function: from foraging to foresight. Trends Cogn. Sci. 18, 72–81. doi: 10.1016/j.tics.2013.11.007
Gottlieb, J. P., Kusunoki, M., and Goldberg, M. E. (1998). The representation of visual salience in monkey parietal cortex. Nature 391, 481–485. doi: 10.1038/35135
Halberda, J., Sires, S. F., and Feigenson, L. (2006). Multiple spatially overlapping sets can be enumerated in parallel. Psychol. Sci. 17, 572–576. doi: 10.1111/j.1467-9280.2006.01746.x
Hauser, M. D., Carey, S., and Hauser, L. B. (2000). Spontaneous number representation in semi-free-ranging rhesus monkeys. Proc. Biol. Sci. 267, 829–833. doi: 10.1098/rspb.2000.1078
Hollingworth, H. L. (1910). The central tendency of judgment. J. Philos. Psychol. Sci. Methods 7, 461–469. doi: 10.2307/2012819
Hubbard, E. M., Piazza, M., Pinel, P., and Dehaene, S. (2005). Interactions between number and space in parietal cortex. Nat. Rev. Neurosci. 6, 435–438. doi: 10.1038/nrn1684
Jazayeri, M., and Shadlen, M. N. (2010). Temporal context calibrates interval timing. Nat. Neurosci. 13, 1020–1026. doi: 10.1038/nn.2590
Jin, D. Z., Fujii, N., and Graybiel, A. M. (2009). Neural representation of time in cortico-basal ganglia circuits. Proc. Natl. Acad. Sci. U.S.A. 106, 19156–19161. doi: 10.1073/pnas.0909881106
Kusunoki, M., Sigala, N., Gaffan, D., and Duncan, J. (2009). Detection of fixed and variable targets in the monkey prefrontal cortex. Cereb. Cortex 19, 2522–2534. doi: 10.1093/cercor/bhp005
Lakoff, G., and Johnson, M. (1999). Philosophy in the Fiesh: The Embodied Mind and its Challenge to Western Thought. Chicago, IL: University of Chicago Press.
Lakoff, G., and Kovecses, Z. (1987). “The cognitive model of anger inherent in American English,” in Cultural Models in Language and Thought, ed N. Q. D. Holland (Cambridge, UK: Cambridge University Press), 195–221.
Lavidor, M., Brinksman, V., and Göbel, S. M. (2004). Hemispheric asymmetry and the mental number line: comparison of double-digit numbers. Neuropsychologia 42, 1927–1933. doi: 10.1016/j.neuropsychologia.2004.05.003
Lebedev, M. A., O'Doherty, J. E., and Nicolelis, M. A. (2008). Decoding of temporal intervals from cortical ensemble activity. J. Neurophysiol. 99, 166–186. doi: 10.1152/jn.00734.2007
Leon, M. I., and Shadlen, M. N. (2003). Representation of time by neurons in the posterior parietal cortex of the macaque. Neuron 38, 317–327. doi: 10.1016/S0896-6273(03)00185-5
Lucchetti, C., and Bon, L. (2001). Time-modulated neuronal activity in the premotor cortex of macaque monkeys. Exp. Brain Res. 141, 254–260. doi: 10.1007/s002210100818
Marcos, E., Tsujimoto, S., and Genovesio, A. (2017). Independent coding of absolute duration and distance magnitudes in the prefrontal cortex. J. Neurophysiol. 117, 195–203. doi: 10.1152/jn.00245.2016
McNeill, D. (1992). Hand and Mind: What Gestures Reveal about Thought. Chicago, IL: University of Chicago Press.
Mendez, J. C., Prado, L., Mendoza, G., and Merchant, H. (2011). Temporal and spatial categorization in human and non-human primates. Front. Integr. Neurosci. 5:50. doi: 10.3389/fnint.2011.00050
Merchant, H., Harrington, D. L., and Meck, W. H. (2013). Neural basis of the perception and estimation of time. Annu. Rev. Neurosci. 36, 313–336. doi: 10.1146/annurev-neuro-062012-170349
Merchant, H., Zarco, W., Perez, O., Prado, L., and Bartolo, R. (2011). Measuring time with multiple neural chronometers during a synchronization-continuation task. Proc. Natl. Acad. Sci. U.S.A. 108, 19784–19789. doi: 10.1073/pnas.1112933108
Merriam, E. P., Genovese, C. R., and Colby, C. L. (2003). Spatial updating in human parietal cortex. Neuron 39, 361–373. doi: 10.1016/S0896-6273(03)00393-3
Merritt, D. J., Casasanto, D., and Brannon, E. M. (2010). Do monkeys think in metaphors? Representations of space and time in monkeys and humans. Cognition 117, 191–202. doi: 10.1016/j.cognition.2010.08.011
Mita, A., Mushiake, H., Shima, K., Matsuzaka, Y., and Tanji, J. (2009). Interval time coding by neurons in the presupplementary and supplementary motor areas. Nat. Neurosci. 12, 502–207. doi: 10.1038/nn.2272
Mitchell, C. T., and Davis, R. (1987). The perception of time in scale model environments. Perception 16, 5–16. doi: 10.1068/p160005
Nicholls, M. E. R., Loftus, A., and Gevers, W. (2008). Look, no hands: a perceptual task shows that number magnitude induces shifts of attention. Psychon. Bull. Rev. 15, 413–418. doi: 10.3758/PBR.15.2.413
Nicholls, M. E., and McIlroy, A. M. (2010). Spatial cues affect men- tal number line bisections. Exp. Psychol. 57, 315–319. doi: 10.1027/1618-3169/a000037
Nieder, A. (2017). Magnitude codes for cross-modal working memory in the primate frontal association cortex. Front. Neurosci. 11:202. doi: 10.3389/fnins.2017.00202
Nieder, A., and Miller, E. K. (2004). A parieto-frontal network for visual numerical information in the monkey. Proc. Natl. Acad. Sci. U.S.A. 101, 7457–7462. doi: 10.1073/pnas.0402239101
Nieder, A., Diester, I., and Tudusciuc, O. (2006). Temporal and spatial enumeration processes in the primate parietal cortex. Science 313, 1431–1435. doi: 10.1126/science.1130308
Nieder, A., Freedman, D. J., and Miller, E. K. (2002). Representation of the quantity of visual items in the primate prefrontal cortex. Science 297, 1708–1711. doi: 10.1126/science.1072493
Núñez, R. E., Cooperrider, K., Doan, D., and Wassmann, J. (2012). Contours of time: topographic construals of past, present, and future in the Yupno valley of Papua New Guinea. Cognition 124, 25–35. doi: 10.1016/j.cognition.2012.03.007
Onoe, H., Komori, M., Onoe, K., Takechi, H., Tsukada, H., and Watanabe, Y. (2001). Cortical networks recruited for time perception: a monkey positron emission tomography (PET) study. Neuroimage 13, 37–45. doi: 10.1006/nimg.2000.0670
Orban, G. A., Van Essen, D., and Vanduffel, W. (2004). Comparative mapping of higher visual areas in monkeys and humans. Trends Cogn. Sci. 8, 315–324. doi: 10.1016/j.tics.2004.05.009
Oshio, K., Chiba, A., and Inase, M. (2008). Temporal filtering by prefrontal neurons in duration discrimination. J. Neurosci. 28, 2333–2343. doi: 10.1111/j.1460-9568.2008.06509.x
Perrett, S. P. (1998). Temporal discrimination in the cerebellar cortex during conditioned eyelid responses. Exp. Brain Res. 121, 115–124. doi: 10.1007/s002210050443
Petzschner, F. H., and Glasauer, S. (2011). Iterative Bayesian estimation as an explanation for range and regression effects: a study on human path integration. J. Neurosci. 31, 17220–17229. doi: 10.1523/JNEUROSCI.2028-11.2011
Rainer, G., Asaad, W. F., and Miller, E. K. (1998). Selective representation of relevant information by neurons in the primate prefrontal cortex. Nature 393, 577–579. doi: 10.1038/31235
Rainer, G., Rao, S. C., and Miller, E. K. (1999). Prospective coding for objects in primate prefrontal cortex. J. Neurosci. 19, 5493–5505.
Restle, F. (1970). Speed of adding and comparing numbers. J. Exp. Psychol. 83, 274–278. doi: 10.1037/h0028573
Saito, N., Mushiake, H., Sakamoto, K., Itoyama, Y., and Tanji, J. (2005). Representation of immediate and final behavioral goals in the monkey prefrontal cortex during an instructed delay period. Cereb. Cortex 15, 1535–1546. doi: 10.1093/cercor/bhi032
Stevens, S. S., and Greenbaum, H. (1966). Regression effect in psychophysical judgment. Percept. Psychophys. 1, 439–446. doi: 10.3758/BF03215821
Tanaka, M. (2007). Cognitive signals in the primate motor thalamus predict saccade timing. J. Neurosci. 27, 12109–12118. doi: 10.1523/JNEUROSCI.1873-07.2007
Teghtsoonian, R., and Teghtsoonian, M. (1978). Range and regression effects in magnitude scaling. Percept. Psychophys. 24, 305–314. doi: 10.3758/BF03204247
Teuscher, U., Brang, D., Ramachandran, V. S., and Coulson, S. (2010). Spatial cueing intime-space synesthetes: an event-related brain potential study. Brain Cogn. 74, 35–46. doi: 10.1016/j.bandc.2010.06.001
Torralbo, A., Santiago, J., and Lupiá-ez, J. (2006). Flexible conceptual projection of time onto spatial frames of reference. Cogn. Sci. 30, 745–757. doi: 10.1207/s15516709cog0000_67
Traugott, E. C. (1978). “On the expression of spatiotemporal relations in language,” in Universals of Human Language: Word Structure, eds J. H. Greenberg, C. A. Ferguson, and E. A. Moravcsik (Stanford, CA: Stanford University Press), 369–400.
Walsh, V. (2003). A theory of magnitude: common cortical metrics of time, space and quantity. Trends Cogn. Sci. 7, 483–488. doi: 10.1016/j.tics.2003.09.002
Wilson, F. A. W., O'Scalaidhe, S. P., and Goldman-Rakic, P. S. (1993). Dissociation of object and spatial processing domains in primate prefrontal cortex. Science 260, 1955–1958. doi: 10.1126/science.8316836
Keywords: monkeys, prefrontal cortex, time perception, working memory, magnitude processing
Citation: Marcos E and Genovesio A (2017) Interference between Space and Time Estimations: From Behavior to Neurons. Front. Neurosci. 11:631. doi: 10.3389/fnins.2017.00631
Received: 25 July 2017; Accepted: 30 October 2017;
Published: 21 November 2017.
Edited by:
Daeyeol Lee, Yale School of Medicine, United StatesReviewed by:
Hansem Sohn, Massachusetts Institute of Technology, United StatesCopyright © 2017 Marcos and Genovesio. This is an open-access article distributed under the terms of the Creative Commons Attribution License (CC BY). The use, distribution or reproduction in other forums is permitted, provided the original author(s) or licensor are credited and that the original publication in this journal is cited, in accordance with accepted academic practice. No use, distribution or reproduction is permitted which does not comply with these terms.
*Correspondence: Aldo Genovesio, YWxkby5nZW5vdmVzaW9AdW5pcm9tYTEuaXQ=
Disclaimer: All claims expressed in this article are solely those of the authors and do not necessarily represent those of their affiliated organizations, or those of the publisher, the editors and the reviewers. Any product that may be evaluated in this article or claim that may be made by its manufacturer is not guaranteed or endorsed by the publisher.
Research integrity at Frontiers
Learn more about the work of our research integrity team to safeguard the quality of each article we publish.