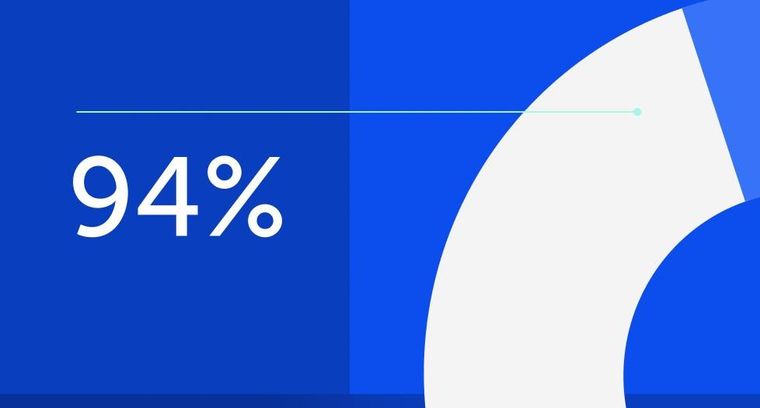
94% of researchers rate our articles as excellent or good
Learn more about the work of our research integrity team to safeguard the quality of each article we publish.
Find out more
MINI REVIEW article
Front. Neurosci., 24 May 2017
Sec. Neuroendocrine Science
Volume 11 - 2017 | https://doi.org/10.3389/fnins.2017.00293
This article is part of the Research TopicNeuroendocrine Control of Feeding BehaviorView all 34 articles
Cannabinoids are lipid messengers that modulate a variety of physiological processes and modify the generation of specific behaviors. In this regard, the cannabinoid receptor type 1 (CB1) represents the most relevant target molecule of cannabinoids so far. One main function of central CB1 signaling is to maintain whole body energy homeostasis. Thus, cannabinoids functionally interact with classical neurotransmitters in neural networks that control energy metabolism and feeding behavior. The promotion of CB1 signaling can increase appetite and stimulate feeding, while blockade of CB1 suppresses hunger and induces hypophagia. However, in order to treat overeating, pharmacological blockade of CB1 by the inverse agonist rimonabant not only suppressed feeding but also resulted in psychiatric side effects. Therefore, research within the last decade focused on deciphering the underlying cellular and molecular mechanisms of central cannabinoid signaling that control feeding and other behaviors, with the overall aim still being the identification of specific targets to develop safe pharmacological interventions for the treatment of obesity. Today, many studies unraveled the subcellular localization of CB1 and the function of cannabinoids in neurons and glial cells within circumscribed brain regions that represent integral parts of neural circuitries controlling feeding behavior. Here, these novel experimental findings will be summarized and recent advances in understanding the mechanisms of CB1-dependent cannabinoid signaling being relevant for central regulation of feeding behavior will be highlighted. Finally, presumed alternative pathways of cannabinoids that are not driven by CB1 activation but also contributing to control of feeding behavior will be introduced.
Central regulation of feeding behavior is indispensable to life, since animals and men have to consume energy in terms of food to exert essential daily functions (Gao and Horvath, 2016). In this regard, a network of neural circuitries evolved that ensures constant energy supply by providing a “pro-feeding” behavioral outcome: in times when food is plentiful, energy intake dominates energy expenditure, so that excessive energy could be stored and used when food was restricted or temporarily not available (Koch and Horvath, 2014).
Cannabinoids, such as THC interfere with central regulation of feeding behavior by acting upon G protein-coupled cannabinoid receptor type 1 (CB1) in the brain (Williams and Kirkham, 1999). However, the underlying molecular and cellular mechanisms of central CB1 signaling in control of feeding and other behaviors are still far from being fully understood (Mazier et al., 2015). Moreover, better insight into the aforementioned network being responsible for central control of feeding behavior is of significant interest, since nowadays, the respective neural circuitries are of substantial clinical relevance. Most importantly, availability of food no longer represents an evolutionary pressure, since food exists in abundance in many (albeit not all) countries around the world. Moreover, energy-dense foods high in carbohydrates and rich in fat can be obtained with little or no efforts. Thus, many people are suffering from chronic overload with nutrients in today's world, which, when accompanied by overall decreased physical activity is often leading to a morbid increase in body fat mass and resulting in obesity. On the other hand, a significant number of patients is affected from a complete loss of appetite (anorexia), which may be caused by psychiatric disorders, or by cancer and infectious diseases, and make these patients suffering from chronic under-nutrition (Scarlett and Marks, 2005; Park et al., 2014). Thus, decoding of the underlying cellular and molecular mechanisms in the central nervous system (CNS) that control feeding behavior may help to develop pharmacological interventions not only for disorders related with anorexia, but also for the treatment of the ever-increasing number of obese patients worldwide (Dietrich and Horvath, 2012).
Since time immemorial, cannabis extracts are used for recreational purposes. However, it is clear today that not only the psychotropic properties but also the well-known appetite stimulating effects of the plant-derived cannabinoid THC are mediated by CB1 activation (Silvestri and Di Marzo, 2013). CB1 belongs to the endocannabinoid system (ECS) that further consists of endocannabinoids (eCBs) as intrinsic CB1 ligands, and of eCB synthesizing and hydrolyzing enzymes (Piomelli, 2003). These enzymes steadily control eCB levels in a temporal and spatial fashion to guaranty functional CB1 signaling in a region and cell type specific manner (Pertwee, 2014). Interestingly, malfunction of the central ECS is associated with overeating and obesity (Engeli, 2008; Mazier et al., 2015). Thus, the main purpose here is to summarize recent experimental findings for central control of feeding behavior in health and disease, with special focus on central CB1 signaling. Finally, presumed alternative, non-CB1 driven pathways by which eCBs might also contribute to feeding regulation will be introduced.
CB1 was discovered almost 30 years ago and later identified as a promising target molecule in the CNS to pharmacologically interfere with feeding behavior (Matsuda et al., 1990; Devane et al., 1992; Williams and Kirkham, 1999). Besides feeding, several other physiological functions, and behaviors being modulated by central CB1 signaling were deciphered so far (Lutz et al., 2015), and many pharmacological, biochemical, and morphological aspects of central CB1 signaling were characterized.
The vast majority of CB1 is located at presynaptic terminals in order to suppress the further release of classical neurotransmitters, such as GABA or glutamate (Castillo et al., 2012). However, different localizations and functions of CB1 were also discovered (Figure 1). In principle, the acute pharmacological promotion of central CB1 signaling can evoke food intake and thus still represents a promising approach to treat anorexia (Williams and Kirkham, 1999; Aigner et al., 2011; Reuter and Martin, 2016). However, it was discovered a couple of years ago that only administration of low to moderate doses of CB1 agonists were able to increase food intake in mice, while moderate to high doses of CB1 agonists decreased feeding (Bellocchio et al., 2010). In this, hypophagia was induced by CB1-mediated reduction of GABAergic transmission, while hyperphagia was stimulated by CB1-driven suppression of glutamatergic conduction (Bellocchio et al., 2010; Busquets Garcia et al., 2016). This fundamental finding in mice might explain the contrary results of different clinical trials on the use of CB1 agonists in order to treat anorexia in humans (Aigner et al., 2011; Reuter and Martin, 2016). Thus, further approaches are needed to carefully reconsider the beneficial effects of CB1 agonists for the treatment of anorexia (Whiting et al., 2015). In contrast to CB1 agonists, the overall blockade of CB1 by rimonabant generally suppressed hunger and induced hypophagia (Colombo et al., 1998; Simiand et al., 1998), but unfortunately also resulted in psychiatric side effects in humans. To develop more specific and safe pharmacological interventions for the treatment of overeating, the recently presented molecular ultrastructure of human CB1 may deliver new opportunities for the design of next-generation CB1 directing pharmaceuticals as novel anti-obesity drugs (Hua et al., 2016; Shao et al., 2016). Moreover, allosteric agents directed against CB1 such as hemopressin or pregnenolone (Heimann et al., 2007; Dodd et al., 2010, 2013; Vallee et al., 2014) may supply medications with a significantly improved side effect profile (Busquets Garcia et al., 2016). Finally, another pharmacological approach aimed at selective blockade of peripheral CB1, which basically was shown to induce metabolic benefits independently from modification of feeding behavior (Nogueiras et al., 2008; Tam et al., 2012). Nevertheless, it is primarily the knowledge about the cell type specific functions of CB1 signaling in different types of neurons, and, as discussed later, also in glial cells, such as astrocytes (Metna-Laurent and Marsicano, 2015), which will determine if and in how far the full therapeutic potential of CB1 pharmacology in feeding regulation can be leveraged.
Figure 1. Principles of central CB1 signaling in control of feeding behavior. (A) Retrograde signaling of eCBs at presynaptic CB1 impacts feeding (Bellocchio et al., 2010). (B) Postsynaptic CB1 at POMC neurons affects feeding in DIO (Morello et al., 2016). (C) Cannabinoids interfere with mitochondrial CB1 in hypothalamic feeding regulation (Koch et al., 2015). (D) Whether activity-dependent subcellular distribution of CB1(Thibault et al., 2013) accounts for control of food intake is still open. (E) Astroglial CB1 regulates the metabolic effects of leptin in cultured astrocytes (Bosier et al., 2013), and thus might contribute to astrocyte-dependent control of feeding behavior in the hypothalamus (Kim et al., 2014). (F) Enzymes of eCB synthesis or degradation control eCB levels in a spatial and temporal manner (Pertwee, 2014). Moreover, eCBs not only function as CB1 ligands, but also as substrates of specific enzymes, such as lipoxygenases (LOX), cyclooxygenases (COX), or cytochrome P450, supporting the idea that the ECS might also transmit metabolic effects independently from CB1 signaling (non-CB1).
In this regard, complexity of central CB1 signaling was further broaden by the observation that CB1, as a G protein-coupled receptor, is not exclusively expressed at the plasma membrane but also located at the outer mitochondrial membrane (Benard et al., 2012; Hebert-Chatelain et al., 2014). By interfering with respiratory chain complex I, mitochondrial CB1 was recently shown to promote the amnesia-inducing effects of CB1 agonists in the hippocampus (Hebert-Chatelain et al., 2016; Harkany and Horvath, 2017). Accordingly, effects of cannabinoids on food intake are also transmitted via CB1-induced mitochondrial adaptations, since induction of feeding by CB1 agonists depended on the expression of mitochondrial uncoupling protein 2 and the formation of reactive oxygen species (ROS) in the hypothalamus (Koch et al., 2015; Kruger, 2016), finally pointing toward region-specific functions of mitochondrial CB1 signaling in the brain (Harkany and Horvath, 2017). However, CB1 driven control of ROS seems to be multifaceted, since cannabinoids reduced leptin-mediated ROS formation in cultured hypothalamic neurons by CB1 dependent peroxisome proliferator-activated receptors (PPAR)-gamma and subsequent catalase activation (Palomba et al., 2015). Overall, about 15% of total brain CB1 is associated with mitochondria (Benard et al., 2012; Hebert-Chatelain et al., 2014), and it appeared that CB1 is present in mitochondria of both pre- and postsynaptic terminals (Busquets Garcia et al., 2016). However, CB1 is most abundantly expressed at the plasma membrane of axonal shafts and presynaptic terminals (Pertwee, 2010), and significant amounts of CB1 in the forebrain are constantly activated, internalized, and recycled at steady state (Thibault et al., 2013). Whether internalization and redistribution of CB1 between axonal plasma membrane and somato-dendritic endosomes account for control of feeding behavior still needs to be investigated. Moreover, functional expression of CB1 is also observed at the postsynaptic plasma membrane (Castillo et al., 2012). In the course of diet-induced obesity (DIO), orexin-A represses satiety-promoting pro-opiomelanocortin (POMC) neurons in the hypothalamic arcuate nucleus (ARC) by eCB-mediated activation of postsynaptic CB1 on POMC neurons (Morello et al., 2016).
In addition to neurons, CB1 is also expressed in astrocytes (Metna-Laurent and Marsicano, 2015; Oliveira Da Cruz et al., 2016), and plays an important role in neuroinflammation (Walter and Stella, 2004), and in physiological neurotransmission (Navarrete and Araque, 2010; Han et al., 2012). Interestingly, astrocyte-dependent energetic support of neurons also involves CB1, since leptin-induced astroglial glycogen accumulation depends on CB1 signaling in cultured astrocytes (Bosier et al., 2013). However, the relevance of astroglial CB1 in distinct hypothalamic feeding centers has to be considered in vivo. Accordingly, structural analyses determined CB1 in the immediate vicinity to astrocytes at tripartite synapses in the ARC (Morozov et al., 2017). Moreover, hypothalamic astrocytes and microglia show morphological adaptations in DIO (Baufeld et al., 2016; Argente-Arizon et al., 2017), and astrocytes, via leptin signaling, actively control hypothalamic neuronal circuits, and feeding (Kim et al., 2014). Thus, it is of significant interest to study the function of CB1 signaling in glial cells under normal and high fat diet (HFD).
Together, studies focusing on the cell type specific expression and subcellular distribution of CB1 delivered unique mechanistic insights into central CB1 signaling, which provides an important prerequisite to uncover the physiological role of CB1 in distinct homeostatic and hedonic feeding centers of the CNS.
Homeostatic feeding centers supervise the body's energy resources and are located in the hypothalamus and caudal brainstem (Koch and Horvath, 2014), while hedonic feeding centers relevant for palatability and rewarding aspects of food are pinpointed to the mesolimbic system (Alonso-Alonso et al., 2015; Pandurangan and Hwang, 2015). Although both control systems are anatomically located in different brain areas, it becomes more likely that they are functionally closely interconnected to each other (Munzberg et al., 2016).
CB1 obtains a conserved distribution in the CNS among different mammalian species (Herkenham et al., 1990). High CB1 expression levels in the hippocampus or basal ganglia are attributed to cannabinoid-induced effects on memory formation and movement (Castillo et al., 2012). Low CB1 expression levels in hypothalamic or caudal brainstem nuclei display significant functions in regulation of feeding behavior (Cardinal et al., 2012; Mazier et al., 2015). In this, distinct groups of hypothalamic neurons measure the body's energy resources by sensing circulating nutrients and detecting metabolic hormones, such as leptin, insulin, or ghrelin (Varela and Horvath, 2012; Vogt and Bruning, 2013; Muller et al., 2015). Moreover, hypothalamic neurons are directly affected by cannabinoids, since infusion of CB1 agonists into distinct hypothalamic nuclei acutely induced feeding (Jamshidi and Taylor, 2001; Koch et al., 2015). Interestingly, hypothalamic CB1 signaling interferes with signal transmission of metabolic hormones. While leptin suppressed feeding correlates with decreased hypothalamic eCB levels (Di Marzo et al., 2001), ghrelin triggered acute feeding accompanies with increased hypothalamic eCB levels, and depends on paraventricular nucleus (PVN) CB1 signaling (Kola et al., 2008). However, CB1 mediated control of feeding in the PVN is more complex than thought before, since under an experimental fasting/re-feeding paradigm, blockade of local CB1 in the PVN increased hyperphagy in hungry mice, and enhanced the hyperphagic effect of ghrelin in fed animals (Soria-Gomez et al., 2014b). Thus, hypothalamic eCBs represent local neuromodulators that are actively involved in rapid rewiring of hypothalamic feeding circuits in accordance to the current prandial state (Pinto et al., 2004). In DIO, imbalanced hypothalamic eCB levels and defective CB1 signaling seem to be the consequence of central leptin resistance (Silvestri and Di Marzo, 2013). In the lateral hypothalamus (LH), CB1 is involved in physiological control of melanin-concentrating hormone and orexin-A neurons (Silvestri and Di Marzo, 2013). In DIO, eCBs in the LH promote hyperphagia by remodeling the synaptic input organization of orexin-A neurons (Alpar and Harkany, 2013; Cristino et al., 2013).
In the ARC, at least two neuronal populations with opposing effects on feeding behavior can be distinguished: the hunger promoting Agouti-related protein/neuropeptide Y (AgRP/NPY) neurons that acutely promote food intake, and POMC neurons that drive gradual onset of satiety (Varela and Horvath, 2012). Systemic blockade of CB1 by rimonabant reduced NPY levels, indicating that AgRP/NPY neurons are controlled by local eCBs (Verty et al., 2009). AgRP/NPY neurons do not contain CB1 (Cota et al., 2003; Horvath, 2003), but CB1 was predominately found at GABAergic terminals innervating AgRP/NPY neurons (Morozov et al., 2017). Thus, local eCBs in the ARC might promote feeding by retrograde dis-inhibition of AgRP/NPY neurons. However, POMC neurons are also affected by cannabinoids via pre- and postsynaptic CB1 (Hentges et al., 2005; Koch et al., 2015; Morello et al., 2016). In fed mice, CB1 agonists rapidly converted POMC neurons from promoters of long-term satiety into acute drivers of hunger (Koch et al., 2015; Patel and Cone, 2015). In DIO, orexin-A repressed POMC neurons by constitutive eCB signaling at postsynaptic CB1 in POMC neurons (Morello et al., 2016). Mapping of hypothalamic neuronal subtypes by single-cell RNA sequencing (Romanov et al., 2017) and molecular indexing of local ARC cell types by gene expression profiling identified novel cell types of putative relevance for regulation of distinct vegetative body functions, including feeding (Campbell et al., 2017). Thus, it would be interesting to dissect the functional relevance of CB1 signaling in these cell types. Accordingly, glutamate-releasing neurons in the ARC that express oxytocin receptors were identified as an integral part of a rapid ARC to PVN satiety pathway (Fenselau et al., 2017). However, whether acute effects of cannabinoids on feeding might be further transmitted by this novel pathway remains elusive. Alongside, local ARC dopaminergic cells were identified that reciprocally control activity of AgRP/NPY and POMC neurons (Zhang and Van Den Pol, 2016). This finding is of substantial interest in order to study CB1 controlled homeostatic feeding, since dopamine modulates rewarding aspects of food mainly through dopaminergic ventral tegmental area (VTA) to nucleus accumbens (NAc) projections (Volkow et al., 2011), and CB1 signaling was shown to modulate dopaminergic signaling in the NAc and VTA to regulate hedonic aspects of feeding (Melis et al., 2007; Di Marzo et al., 2009).
Beside the VTA located in the rostral brainstem, CB1 signaling is also interfering with the functional activity of caudal brainstem nuclei, such as parabrachial nucleus, dorsal motor nucleus of the vagus, and nucleus of the solitary tract. In this, CB1 basically controls food preferences, such as digestion of palatable foods being rich in fat (Busquets Garcia et al., 2016). Finally, hypothalamic AgRP/NPY and POMC neurons are not only directly affected by food intake itself, but also rapidly respond to sensory detection of available food (Chen et al., 2015). It is thus likely that hypothalamic neurons not only transmit internal signals causing hunger or satiety in response to eating and internal sensing of energy resources, but also receive external information on the incentive value of food, such as sight, smell, and taste in order to rapidly react to food stimuli and transmit motivational aspects on feeding being generated via the mesolimbic system (Seeley and Berridge, 2015). Processing of food sensations such as olfactory or gustatory signals indeed involve CB1 signaling, since fasted mice displayed CB1-dependent increased odor detection in the main olfactory bulb (Soria-Gomez et al., 2014a).
Within the ECS, it is the availability of eCBs that provides the routes and directions of CB1 signaling in the brain. While research was long-time focusing on pharmacological modulation of CB1 signaling by direct interaction at CB1 in order to interfere with feeding and other behaviors, numerous evidence arose that targeting of classical enzymes involved in biosynthesis or degradation of eCBs will also allow to induce adaptations in feeding behaviors (Pertwee, 2014). For example, degradation of the eCB 2-arachidonoylglycerol (2-AG) into arachidonic acid and glycerol is basically controlled by three different serine hydrolases: while monoacylglycerol lipase (MAGL) accounts for 85% of 2-AG degradation, alpha/beta-hydrolase domain containing (ABHD) 6, and 12 are responsible for hydrolysis of 5 and 10%, respectively (Savinainen et al., 2012). Indeed, it was shown that knockdown of ABHD6 in the ventromedial hypothalamus resulted in locally elevated 2-AG levels, finally resulting in a blunted fasting-induced feeding response and in a general diminished efficacy of the mice in order to adapt to other metabolic shifts (Fisette et al., 2016).
Generally, eCBs do not resemble to classical neurotransmitters that are stored in synaptic vesicles (Piomelli, 2003). Instead, eCBs, as being arachidonic acid derivatives, are produced on demand from lipid precursors. Most eCBs display a relative short half-life, since they are attracted by both classical eCB degrading enzymes in order to terminate CB1 signaling, and by different classes of enzymes aiming transformation of eCBs into other classes of lipidergic signaling molecules, such as prostamides (Urquhart et al., 2015). The fact that eCBs belong to the family of polyunsaturated fatty acids makes them indeed attractive substrates for enzymatic oxidation, as induced by lipoxygenases (LOX), cyclooxygenases (COX), or cytochrome P450 (Rouzer and Marnett, 2011). Numerous eCBs have been described so far and in addition to 2-AG it is arachidonoylethanolamine (AEA) representing by far the best-studied intrinsic ligand of CB1 today. However, beside CB1 and CB2 as the most relevant G protein-coupled receptors of cannabinoids, it is likely that eCBs also act upon several other G protein-coupled receptors, such as GPR18, GPR55, and GPR119. These former orphan receptors are putative candidates for nomination of CB3, however their relevance in feeding regulation has to be further investigated. Nevertheless, it appeared that GPR18 and GPR55 signaling is involved in processes of metabolic dysfunction (Liu et al., 2015; Rajaraman et al., 2016). Besides G protein-coupled receptors, eCBs such as AEA were also shown to act upon other types of receptors, such as transient receptor potential (TRP) vanilloid 1 (Pertwee, 2010). Moreover, several enzymes involved in eCB biosynthesis, such as the AEA synthesizing N-acyl phosphatidylethanolamine-specific phospholipase D (NAPE-PLD) not only give rise to the CB1 ligand AEA, but also to structural very similar lipid messengers that do not bind and activate CB1. In this, it was shown that oleoylethanolamine (OEA) and palmitoylethanolamine (PEA), as close related lipids of AEA, bind to PPARs (Fu et al., 2003; Lo Verme et al., 2005; Gaetani et al., 2010), which are well-known to contribute in control of glucose, lipid, and energy metabolism (Grygiel-Gorniak, 2014). Thus, the overall metabolic role of the enzymes in the ECS, beside CB1, may deliver future targets for therapeutic interventions in control of feeding behavior. Indeed, targeted lipidomics of different brain regions derived from mice either deficient for CB1, the AEA degrading enzyme FAAH or the aforementioned 2-AG degrading MAGL revealed that AEA and 2-AG hydrolyzing enzymes, when compared to CB1, link the ECS to a broader lipid signaling network in contrasting ways, which again may open an avenue in altering neurotransmission and behaviors independently of CB1 signaling (Leishman et al., 2016a). This assumption is further supported by another lipidomic analysis. In this, mice deficient for NAPE-PLD not only displayed a shift in the concentration of AEA, but also shifted several other lipids, not binding to CB1, such as OEA and PEA, that as mentioned before signal upon different metabolic relevant targets, such as PPARs (Leishman et al., 2016b).
Actually, there has been significant increase of knowledge about central CB1 signaling in control of feeding behavior. Despite the significant setback that occurred in the past on clinical use of CB1 inverse agonists in order to treat overeating, there still is strong confidence in the field that the recent discoveries on central CB1 signaling soon will leverage the therapeutic potential of CB1.
MK designed this review, including Figure 1.
This work was supported by the Deutsche Forschungsgemeinschaft CRC 1052/2 (Obesity Mechanisms).
The author declares that the research was conducted in the absence of any commercial or financial relationships that could be construed as a potential conflict of interest.
Aigner, M., Treasure, J., Kaye, W., Kasper, S., and Disorders, W.T.F.O.E. (2011). World Federation of Societies of Biological Psychiatry (WFSBP) guidelines for the pharmacological treatment of eating disorders. World J. Biol. Psychiatry 12, 400–443. doi: 10.3109/15622975.2011.602720
Alonso-Alonso, M., Woods, S. C., Pelchat, M., Grigson, P. S., Stice, E., Farooqi, S., et al. (2015). Food reward system: current perspectives and future research needs. Nutr. Rev. 73, 296–307. doi: 10.1093/nutrit/nuv002
Alpar, A., and Harkany, T. (2013). Orexin neurons use endocannabinoids to break obesity-induced inhibition. Proc. Natl. Acad. Sci. U.S.A. 110, 9625–9626. doi: 10.1073/pnas.1307389110
Argente-Arizon, P., Guerra-Cantera, S., Garcia-Segura, L. M., Argente, J., and Chowen, J. A. (2017). Glial cells and energy balance. J. Mol. Endocrinol. 58, R59–R71. doi: 10.1530/JME-16-0182
Baufeld, C., Osterloh, A., Prokop, S., Miller, K. R., and Heppner, F. L. (2016). High-fat diet-induced brain region-specific phenotypic spectrum of CNS resident microglia. Acta Neuropathol. 132, 361–375. doi: 10.1007/s00401-016-1595-4
Bellocchio, L., Lafenetre, P., Cannich, A., Cota, D., Puente, N., Grandes, P., et al. (2010). Bimodal control of stimulated food intake by the endocannabinoid system. Nat. Neurosci. 13, 281–283. doi: 10.1038/nn.2494
Benard, G., Massa, F., Puente, N., Lourenco, J., Bellocchio, L., Soria-Gomez, E., et al. (2012). Mitochondrial CB(1) receptors regulate neuronal energy metabolism. Nat. Neurosci. 15, 558–564. doi: 10.1038/nn.3053
Bosier, B., Bellocchio, L., Metna-Laurent, M., Soria-Gomez, E., Matias, I., Hebert-Chatelain, E., et al. (2013). Astroglial CB1 cannabinoid receptors regulate leptin signaling in mouse brain astrocytes. Mol. Metab. 2, 393–404. doi: 10.1016/j.molmet.2013.08.001
Busquets Garcia, A., Soria-Gomez, E., Bellocchio, L., and Marsicano, G. (2016). Cannabinoid receptor type-1: breaking the dogmas. F1000Res 2016:5. doi: 10.12688/f1000research.8245.1
Campbell, J. N., Macosko, E. Z., Fenselau, H., Pers, T. H., Lyubetskaya, A., Tenen, D., et al. (2017). A molecular census of arcuate hypothalamus and median eminence cell types. Nat. Neurosci. 20, 484–496. doi: 10.1038/nn.4495
Cardinal, P., Bellocchio, L., Clark, S., Cannich, A., Klugmann, M., Lutz, B., et al. (2012). Hypothalamic CB1 cannabinoid receptors regulate energy balance in mice. Endocrinology 153, 4136–4143. doi: 10.1210/en.2012-1405
Castillo, P. E., Younts, T. J., Chavez, A. E., and Hashimotodani, Y. (2012). Endocannabinoid signaling and synaptic function. Neuron 76, 70–81. doi: 10.1016/j.neuron.2012.09.020
Chen, Y., Lin, Y. C., Kuo, T. W., and Knight, Z. A. (2015). Sensory detection of food rapidly modulates arcuate feeding circuits. Cell 160, 829–841. doi: 10.1016/j.cell.2015.01.033
Colombo, G., Agabio, R., Diaz, G., Lobina, C., Reali, R., and Gessa, G. L. (1998). Appetite suppression and weight loss after the cannabinoid antagonist SR 141716. Life Sci. 63, PL113–PL117. doi: 10.1016/S0024-3205(98)00322-1
Cota, D., Marsicano, G., Tschop, M., Grubler, Y., Flachskamm, C., Schubert, M., et al. (2003). The endogenous cannabinoid system affects energy balance via central orexigenic drive and peripheral lipogenesis. J. Clin. Invest. 112, 423–431. doi: 10.1172/JCI17725
Cristino, L., Busetto, G., Imperatore, R., Ferrandino, I., Palomba, L., Silvestri, C., et al. (2013). Obesity-driven synaptic remodeling affects endocannabinoid control of orexinergic neurons. Proc. Natl. Acad. Sci. U.S.A. 110, E2229–E2238. doi: 10.1073/pnas.1219485110
Devane, W. A., Hanus, L., Breuer, A., Pertwee, R. G., Stevenson, L. A., Griffin, G., et al. (1992). Isolation and structure of a brain constituent that binds to the cannabinoid receptor. Science 258, 1946–1949. doi: 10.1126/science.1470919
Di Marzo, V., Goparaju, S. K., Wang, L., Liu, J., Batkai, S., Jarai, Z., et al. (2001). Leptin-regulated endocannabinoids are involved in maintaining food intake. Nature 410, 822–825. doi: 10.1038/35071088
Di Marzo, V., Ligresti, A., and Cristino, L. (2009). The endocannabinoid system as a link between homoeostatic and hedonic pathways involved in energy balance regulation. Int. J. Obes. 33(Suppl. 2), S18–S24. doi: 10.1038/ijo.2009.67
Dietrich, M. O., and Horvath, T. L. (2012). Limitations in anti-obesity drug development: the critical role of hunger-promoting neurons. Nat. Rev. Drug Discov. 11, 675–691. doi: 10.1038/nrd3739
Dodd, G. T., Mancini, G., Lutz, B., and Luckman, S. M. (2010). The peptide hemopressin acts through CB1 cannabinoid receptors to reduce food intake in rats and mice. J. Neurosci. 30, 7369–7376. doi: 10.1523/JNEUROSCI.5455-09.2010
Dodd, G. T., Worth, A. A., Hodkinson, D. J., Srivastava, R. K., Lutz, B., Williams, S. R., et al. (2013). Central functional response to the novel peptide cannabinoid, hemopressin. Neuropharmacology 71, 27–36. doi: 10.1016/j.neuropharm.2013.03.007
Engeli, S. (2008). Dysregulation of the endocannabinoid system in obesity. J. Neuroendocrinol. 20(Suppl. 1), 110–115. doi: 10.1111/j.1365-2826.2008.01683.x
Fenselau, H., Campbell, J. N., Verstegen, A. M., Madara, J. C., Xu, J., Shah, B. P., et al. (2017). A rapidly acting glutamatergic ARC–>PVH satiety circuit postsynaptically regulated by alpha-MSH. Nat. Neurosci. 20, 42–51. doi: 10.1038/nn.4442
Fisette, A., Tobin, S., Decarie-Spain, L., Bouyakdan, K., Peyot, M. L., Madiraju, S. R., et al. (2016). Alpha/beta-hydrolase domain 6 in the ventromedial hypothalamus controls energy metabolism flexibility. Cell Rep. 17, 1217–1226. doi: 10.1016/j.celrep.2016.10.004
Fu, J., Gaetani, S., Oveisi, F., Lo Verme, J., Serrano, A., Rodriguez De Fonseca, F., et al. (2003). Oleylethanolamide regulates feeding and body weight through activation of the nuclear receptor PPAR-alpha. Nature 425, 90–93. doi: 10.1038/nature01921
Gaetani, S., Fu, J., Cassano, T., Dipasquale, P., Romano, A., Righetti, L., et al. (2010). The fat-induced satiety factor oleoylethanolamide suppresses feeding through central release of oxytocin. J. Neurosci. 30, 8096–8101. doi: 10.1523/JNEUROSCI.0036-10.2010
Gao, X. B., and Horvath, T. L. (2016). Feeding behavior: hypocretin/orexin neurons act between food seeking and eating. Curr. Biol. 26, R845–R847. doi: 10.1016/j.cub.2016.07.069
Grygiel-Gorniak, B. (2014). Peroxisome proliferator-activated receptors and their ligands: nutritional and clinical implications–a review. Nutr. J. 13:17. doi: 10.1186/1475-2891-13-17
Han, J., Kesner, P., Metna-Laurent, M., Duan, T., Xu, L., Georges, F., et al. (2012). Acute cannabinoids impair working memory through astroglial CB1 receptor modulation of hippocampal LTD. Cell 148, 1039–1050. doi: 10.1016/j.cell.2012.01.037
Harkany, T., and Horvath, T. L. (2017). (S)Pot on mitochondria: cannabinoids disrupt cellular respiration to limit neuronal activity. Cell Metab. 25, 8–10. doi: 10.1016/j.cmet.2016.12.020
Hebert-Chatelain, E., Desprez, T., Serrat, R., Bellocchio, L., Soria-Gomez, E., Busquets-Garcia, A., et al. (2016). A cannabinoid link between mitochondria and memory. Nature 539, 555–559. doi: 10.1038/nature20127
Hebert-Chatelain, E., Reguero, L., Puente, N., Lutz, B., Chaouloff, F., Rossignol, R., et al. (2014). Cannabinoid control of brain bioenergetics: exploring the subcellular localization of the CB1 receptor. Mol. Metab. 3, 495–504. doi: 10.1016/j.molmet.2014.03.007
Heimann, A. S., Gomes, I., Dale, C. S., Pagano, R. L., Gupta, A., De Souza, L. L., et al. (2007). Hemopressin is an inverse agonist of CB1 cannabinoid receptors. Proc. Natl. Acad. Sci. U.S.A. 104, 20588–20593. doi: 10.1073/pnas.0706980105
Hentges, S. T., Low, M. J., and Williams, J. T. (2005). Differential regulation of synaptic inputs by constitutively released endocannabinoids and exogenous cannabinoids. J. Neurosci. 25, 9746–9751. doi: 10.1523/JNEUROSCI.2769-05.2005
Herkenham, M., Lynn, A. B., Little, M. D., Johnson, M. R., Melvin, L. S., De Costa, B. R., et al. (1990). Cannabinoid receptor localization in brain. Proc. Natl. Acad. Sci. U.S.A. 87, 1932–1936. doi: 10.1073/pnas.87.5.1932
Horvath, T. L. (2003). Endocannabinoids and the regulation of body fat: the smoke is clearing. J. Clin. Invest. 112, 323–326. doi: 10.1172/JCI19376
Hua, T., Vemuri, K., Pu, M., Qu, L., Han, G. W., Wu, Y., et al. (2016). Crystal structure of the human cannabinoid receptor CB1. Cell 167, 750 e714–762 e714. doi: 10.1016/j.cell.2016.10.004
Jamshidi, N., and Taylor, D. A. (2001). Anandamide administration into the ventromedial hypothalamus stimulates appetite in rats. Br. J. Pharmacol. 134, 1151–1154. doi: 10.1038/sj.bjp.0704379
Kim, J. G., Suyama, S., Koch, M., Jin, S., Argente-Arizon, P., Argente, J., et al. (2014). Leptin signaling in astrocytes regulates hypothalamic neuronal circuits and feeding. Nat. Neurosci. 17, 908–910. doi: 10.1038/nn.3725
Koch, M., and Horvath, T. L. (2014). Molecular and cellular regulation of hypothalamic melanocortin neurons controlling food intake and energy metabolism. Mol. Psychiatry 19, 752–761. doi: 10.1038/mp.2014.30
Koch, M., Varela, L., Kim, J. G., Kim, J. D., Hernandez-Nuno, F., Simonds, S. E., et al. (2015). Hypothalamic POMC neurons promote cannabinoid-induced feeding. Nature 519, 45–50. doi: 10.1038/nature14260
Kola, B., Farkas, I., Christ-Crain, M., Wittmann, G., Lolli, F., Amin, F., et al. (2008). The orexigenic effect of ghrelin is mediated through central activation of the endogenous cannabinoid system. PLoS ONE 3:e1797. doi: 10.1371/journal.pone.0001797
Kruger, R. P. (2016). Harvesting benefits from cannabinoids. Cell 167, 1663–1665. doi: 10.1016/j.cell.2016.12.001
Leishman, E., Cornett, B., Spork, K., Straiker, A., Mackie, K., and Bradshaw, H. B. (2016a). Broad impact of deleting endogenous cannabinoid hydrolyzing enzymes and the CB1 cannabinoid receptor on the endogenous cannabinoid-related lipidome in eight regions of the mouse brain. Pharmacol. Res. 110, 159–172. doi: 10.1016/j.phrs.2016.04.020
Leishman, E., Mackie, K., Luquet, S., and Bradshaw, H. B. (2016b). Lipidomics profile of a NAPE-PLD KO mouse provides evidence of a broader role of this enzyme in lipid metabolism in the brain. Biochim. Biophys. Acta 1861, 491–500. doi: 10.1016/j.bbalip.2016.03.003
Liu, B., Song, S., Jones, P. M., and Persaud, S. J. (2015). GPR55: from orphan to metabolic regulator? Pharmacol. Ther. 145, 35–42. doi: 10.1016/j.pharmthera.2014.06.007
Lo Verme, J., Fu, J., Astarita, G., La Rana, G., Russo, R., Calignano, A., et al. (2005). The nuclear receptor peroxisome proliferator-activated receptor-alpha mediates the anti-inflammatory actions of palmitoylethanolamide. Mol. Pharmacol. 67, 15–19. doi: 10.1124/mol.104.006353
Lutz, B., Marsicano, G., Maldonado, R., and Hillard, C. J. (2015). The endocannabinoid system in guarding against fear, anxiety and stress. Nat. Rev. Neurosci. 16, 705–718. doi: 10.1038/nrn4036
Matsuda, L. A., Lolait, S. J., Brownstein, M. J., Young, A. C., and Bonner, T. I. (1990). Structure of a cannabinoid receptor and functional expression of the cloned cDNA. Nature 346, 561–564. doi: 10.1038/346561a0
Mazier, W., Saucisse, N., Gatta-Cherifi, B., and Cota, D. (2015). The endocannabinoid system: pivotal orchestrator of obesity and metabolic disease. Trends Endocrinol. Metab. 26, 524–537. doi: 10.1016/j.tem.2015.07.007
Melis, T., Succu, S., Sanna, F., Boi, A., Argiolas, A., and Melis, M. R. (2007). The cannabinoid antagonist SR 141716A (Rimonabant) reduces the increase of extra-cellular dopamine release in the rat nucleus accumbens induced by a novel high palatable food. Neurosci. Lett. 419, 231–235. doi: 10.1016/j.neulet.2007.04.012
Metna-Laurent, M., and Marsicano, G. (2015). Rising stars: modulation of brain functions by astroglial type-1 cannabinoid receptors. Glia 63, 353–364. doi: 10.1002/glia.22773
Morello, G., Imperatore, R., Palomba, L., Finelli, C., Labruna, G., Pasanisi, F., et al. (2016). Orexin-A represses satiety-inducing POMC neurons and contributes to obesity via stimulation of endocannabinoid signaling. Proc. Natl. Acad. Sci. U.S.A. 113, 4759–4764. doi: 10.1073/pnas.1521304113
Morozov, Y. M., Koch, M., Rakic, P., and Horvath, T. L. (2017). Cannabinoid type 1 receptor-containing axons innervate NPY/AgRP neurons in the mouse arcuate nucleus. Mol. Metab. 6, 374–381. doi: 10.1016/j.molmet.2017.01.004
Muller, T. D., Nogueiras, R., Andermann, M. L., Andrews, Z. B., Anker, S. D., Argente, J., et al. (2015). Ghrelin. Mol. Metab. 4, 437–460. doi: 10.1016/j.molmet.2015.03.005
Munzberg, H., Qualls-Creekmore, E., Yu, S., Morrison, C. D., and Berthoud, H. R. (2016). Hedonics act in unison with the homeostatic system to unconsciously control body weight. Front Nutr 3:6. doi: 10.3389/fnut.2016.00006
Navarrete, M., and Araque, A. (2010). Endocannabinoids potentiate synaptic transmission through stimulation of astrocytes. Neuron 68, 113–126. doi: 10.1016/j.neuron.2010.08.043
Nogueiras, R., Veyrat-Durebex, C., Suchanek, P. M., Klein, M., Tschop, J., Caldwell, C., et al. (2008). Peripheral, but not central, CB1 antagonism provides food intake-independent metabolic benefits in diet-induced obese rats. Diabetes 57, 2977–2991. doi: 10.2337/db08-0161
Oliveira Da Cruz, J. F., Robin, L. M., Drago, F., Marsicano, G., and Metna-Laurent, M. (2016). Astroglial type-1 cannabinoid receptor (CB1): a new player in the tripartite synapse. Neuroscience 323, 35–42. doi: 10.1016/j.neuroscience.2015.05.002
Palomba, L., Silvestri, C., Imperatore, R., Morello, G., Piscitelli, F., Martella, A., et al. (2015). Negative regulation of leptin-induced Reactive Oxygen Species (ROS) formation by cannabinoid CB1 receptor activation in hypothalamic neurons. J. Biol. Chem. 290, 13669–13677. doi: 10.1074/jbc.M115.646885
Pandurangan, M., and Hwang, I. (2015). Systemic mechanism of taste, flavour and palatability in brain. Appl. Biochem. Biotechnol. 175, 3133–3147. doi: 10.1007/s12010-015-1488-3
Park, R. J., Godier, L. R., and Cowdrey, F. A. (2014). Hungry for reward: how can neuroscience inform the development of treatment for Anorexia Nervosa? Behav. Res. Ther. 62, 47–59. doi: 10.1016/j.brat.2014.07.007
Patel, S., and Cone, R. D. (2015). Neuroscience: a cellular basis for the munchies. Nature 519, 38–40. doi: 10.1038/nature14206
Pertwee, R. G. (2010). Receptors and channels targeted by synthetic cannabinoid receptor agonists and antagonists. Curr. Med. Chem. 17, 1360–1381. doi: 10.2174/092986710790980050
Pertwee, R. G. (2014). Elevating endocannabinoid levels: pharmacological strategies and potential therapeutic applications. Proc. Nutr. Soc. 73, 96–105. doi: 10.1017/S0029665113003649
Pinto, S., Roseberry, A. G., Liu, H., Diano, S., Shanabrough, M., Cai, X., et al. (2004). Rapid rewiring of arcuate nucleus feeding circuits by leptin. Science 304, 110–115. doi: 10.1126/science.1089459
Piomelli, D. (2003). The molecular logic of endocannabinoid signalling. Nat. Rev. Neurosci. 4, 873–884. doi: 10.1038/nrn1247
Rajaraman, G., Simcocks, A., Hryciw, D. H., Hutchinson, D. S., and Mcainch, A. J. (2016). G protein coupled receptor 18: a potential role for endocannabinoid signaling in metabolic dysfunction. Mol. Nutr. Food Res. 60, 92–102. doi: 10.1002/mnfr.201500449
Reuter, S. E., and Martin, J. H. (2016). Pharmacokinetics of cannabis in cancer cachexia-anorexia syndrome. Clin. Pharmacokinet. 55, 807–812. doi: 10.1007/s40262-015-0363-2
Romanov, R. A., Zeisel, A., Bakker, J., Girach, F., Hellysaz, A., Tomer, R., et al. (2017). Molecular interrogation of hypothalamic organization reveals distinct dopamine neuronal subtypes. Nat. Neurosci. 20, 176–188. doi: 10.1038/nn.4462
Rouzer, C. A., and Marnett, L. J. (2011). Endocannabinoid oxygenation by cyclooxygenases, lipoxygenases, and cytochromes P450: cross-talk between the eicosanoid and endocannabinoid signaling pathways. Chem. Rev. 111, 5899–5921. doi: 10.1021/cr2002799
Savinainen, J. R., Saario, S. M., and Laitinen, J. T. (2012). The serine hydrolases MAGL, ABHD6 and ABHD12 as guardians of 2-arachidonoylglycerol signalling through cannabinoid receptors. Acta Physiol. 204, 267–276. doi: 10.1111/j.1748-1716.2011.02280.x
Scarlett, J. M., and Marks, D. L. (2005). The use of melanocortin antagonists in cachexia of chronic disease. Expert Opin. Investig. Drugs 14, 1233–1239. doi: 10.1517/13543784.14.10.1233
Seeley, R. J., and Berridge, K. C. (2015). The hunger games. Cell 160, 805–806. doi: 10.1016/j.cell.2015.02.028
Shao, Z., Yin, J., Chapman, K., Grzemska, M., Clark, L., Wang, J., et al. (2016). High-resolution crystal structure of the human CB1 cannabinoid receptor. Nature 540, 602–606. doi: 10.1038/nature20613
Silvestri, C., and Di Marzo, V. (2013). The endocannabinoid system in energy homeostasis and the etiopathology of metabolic disorders. Cell Metab. 17, 475–490. doi: 10.1016/j.cmet.2013.03.001
Simiand, J., Keane, M., Keane, P. E., and Soubrie, P. (1998). SR 141716, a CB1 cannabinoid receptor antagonist, selectively reduces sweet food intake in marmoset. Behav. Pharmacol. 9, 179–181.
Soria-Gomez, E., Bellocchio, L., Reguero, L., Lepousez, G., Martin, C., Bendahmane, M., et al. (2014a). The endocannabinoid system controls food intake via olfactory processes. Nat. Neurosci. 17, 407–415. doi: 10.1038/nn.3647
Soria-Gomez, E., Massa, F., Bellocchio, L., Rueda-Orozco, P. E., Ciofi, P., Cota, D., et al. (2014b). Cannabinoid type-1 receptors in the paraventricular nucleus of the hypothalamus inhibit stimulated food intake. Neuroscience 263C, 46–53. doi: 10.1016/j.neuroscience.2014.01.005
Tam, J., Cinar, R., Liu, J., Godlewski, G., Wesley, D., Jourdan, T., et al. (2012). Peripheral cannabinoid-1 receptor inverse agonism reduces obesity by reversing leptin resistance. Cell Metab. 16, 167–179. doi: 10.1016/j.cmet.2012.07.002
Thibault, K., Carrel, D., Bonnard, D., Gallatz, K., Simon, A., Biard, M., et al. (2013). Activation-dependent subcellular distribution patterns of CB1 cannabinoid receptors in the rat forebrain. Cereb. Cortex 23, 2581–2591. doi: 10.1093/cercor/bhs240
Urquhart, P., Nicolaou, A., and Woodward, D. F. (2015). Endocannabinoids and their oxygenation by cyclo-oxygenases, lipoxygenases and other oxygenases. Biochim. Biophys. Acta 1851, 366–376. doi: 10.1016/j.bbalip.2014.12.015
Vallee, M., Vitiello, S., Bellocchio, L., Hebert-Chatelain, E., Monlezun, S., Martin-Garcia, E., et al. (2014). Pregnenolone can protect the brain from cannabis intoxication. Science 343, 94–98. doi: 10.1126/science.1243985
Varela, L., and Horvath, T. L. (2012). Leptin and insulin pathways in POMC and AgRP neurons that modulate energy balance and glucose homeostasis. EMBO Rep. 13, 1079–1086. doi: 10.1038/embor.2012.174
Verty, A. N., Boon, W. M., Mallet, P. E., Mcgregor, I. S., and Oldfield, B. J. (2009). Involvement of hypothalamic peptides in the anorectic action of the CB receptor antagonist rimonabant (SR 141716). Eur. J. Neurosci. 29, 2207–2216. doi: 10.1111/j.1460-9568.2009.06750.x
Vogt, M. C., and Bruning, J. C. (2013). CNS insulin signaling in the control of energy homeostasis and glucose metabolism - from embryo to old age. Trends Endocrinol. Metab. 24, 76–84. doi: 10.1016/j.tem.2012.11.004
Volkow, N. D., Wang, G. J., and Baler, R. D. (2011). Reward, dopamine and the control of food intake: implications for obesity. Trends Cogn. Sci. 15, 37–46. doi: 10.1016/j.tics.2010.11.001
Walter, L., and Stella, N. (2004). Cannabinoids and neuroinflammation. Br. J. Pharmacol. 141, 775–785. doi: 10.1038/sj.bjp.0705667
Whiting, P. F., Wolff, R. F., Deshpande, S., Di Nisio, M., Duffy, S., Hernandez, A. V., et al. (2015). Cannabinoids for medical use: a systematic review and meta-analysis. JAMA 313, 2456–2473. doi: 10.1001/jama.2015.6358
Williams, C. M., and Kirkham, T. C. (1999). Anandamide induces overeating: mediation by central cannabinoid (CB1) receptors. Psychopharmacology 143, 315–317. doi: 10.1007/s002130050953
Keywords: cannabinoid receptor type 1, endocannabinoids, hypothalamus, feeding behavior, anorexia, cachexia, overeating, obesity
Citation: Koch M (2017) Cannabinoid Receptor Signaling in Central Regulation of Feeding Behavior: A Mini-Review. Front. Neurosci. 11:293. doi: 10.3389/fnins.2017.00293
Received: 15 February 2017; Accepted: 09 May 2017;
Published: 24 May 2017.
Edited by:
Hubert Vaudry, University of Rouen, FranceReviewed by:
Daniela Cota, Institut National de la Santé et de la Recherche Médicale, FranceCopyright © 2017 Koch. This is an open-access article distributed under the terms of the Creative Commons Attribution License (CC BY). The use, distribution or reproduction in other forums is permitted, provided the original author(s) or licensor are credited and that the original publication in this journal is cited, in accordance with accepted academic practice. No use, distribution or reproduction is permitted which does not comply with these terms.
*Correspondence: Marco Koch, bWFyY28ua29jaEBtZWRpemluLnVuaS1sZWlwemlnLmRl
Disclaimer: All claims expressed in this article are solely those of the authors and do not necessarily represent those of their affiliated organizations, or those of the publisher, the editors and the reviewers. Any product that may be evaluated in this article or claim that may be made by its manufacturer is not guaranteed or endorsed by the publisher.
Research integrity at Frontiers
Learn more about the work of our research integrity team to safeguard the quality of each article we publish.