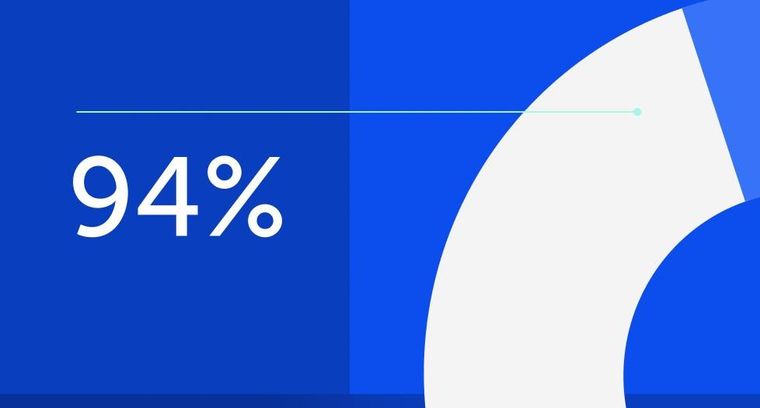
94% of researchers rate our articles as excellent or good
Learn more about the work of our research integrity team to safeguard the quality of each article we publish.
Find out more
ORIGINAL RESEARCH article
Front. Neurosci., 28 February 2017
Sec. Neuroendocrine Science
Volume 11 - 2017 | https://doi.org/10.3389/fnins.2017.00092
This article is part of the Research TopicTrends in Regulatory PeptidesView all 27 articles
Aims: Apelin is a predicted substrate for ACE2, a novel therapeutic target. Our aim was to demonstrate the endogenous presence of the putative ACE2 product [Pyr1]apelin-13(1–12) in human cardiovascular tissues and to confirm it retains significant biological activity for the apelin receptor in vitro and in vivo. The minimum active apelin fragment was also investigated.
Methods and Results: [Pyr1]apelin-13 incubated with recombinant human ACE2 resulted in de novo generation of [Pyr1]apelin-13(1–12) identified by mass spectrometry. Endogenous [Pyr1]apelin-13(1–12) was detected by immunostaining in human heart and lung localized to the endothelium. Expression was undetectable in lung from patients with pulmonary arterial hypertension. In human heart [Pyr1]apelin-13(1–12) (pKi = 8.04 ± 0.06) and apelin-13(F13A) (pKi = 8.07 ± 0.24) competed with [125I]apelin-13 binding with nanomolar affinity, 4-fold lower than for [Pyr1]apelin-13 (pKi = 8.83 ± 0.06) whereas apelin-17 exhibited highest affinity (pKi = 9.63 ± 0.17). The rank order of potency of peptides to inhibit forskolin-stimulated cAMP was apelin-17 (pD2 = 10.31 ± 0.28) > [Pyr1]apelin-13 (pD2 = 9.67 ± 0.04) ≥ apelin-13(F13A) (pD2 = 9.54 ± 0.05) > [Pyr1]apelin-13(1–12) (pD2 = 9.30 ± 0.06). The truncated peptide apelin-13(R10M) retained nanomolar potency (pD2 = 8.70 ± 0.04) but shorter fragments exhibited low micromolar potency. In a β-arrestin recruitment assay the rank order of potency was apelin-17 (pD2 = 10.26 ± 0.09) >> [Pyr1]apelin-13 (pD2 = 8.43 ± 0.08) > apelin-13(R10M) (pD2 = 8.26 ± 0.17) > apelin-13(F13A) (pD2 = 7.98 ± 0.04) ≥ [Pyr1]apelin-13(1–12) (pD2 = 7.84 ± 0.06) >> shorter fragments (pD2 < 6). [Pyr1]apelin-13(1–12) and apelin-13(F13A) contracted human saphenous vein with similar sub-nanomolar potencies and [Pyr1]apelin-13(1–12) was a potent inotrope in paced mouse right ventricle and human atria. [Pyr1]apelin-13(1–12) elicited a dose-dependent decrease in blood pressure in anesthetized rat and dose-dependent increase in forearm blood flow in human volunteers.
Conclusions: We provide evidence that ACE2 cleaves [Pyr1]apelin-13 to [Pyr1]apelin-13(1–12) and this cleavage product is expressed in human cardiovascular tissues. We have demonstrated biological activity of [Pyr1]apelin-13(1–12) at the human and rodent apelin receptor in vitro and in vivo. Our data show that reported enhanced ACE2 activity in cardiovascular disease should not significantly compromise the beneficial effects of apelin based therapies for example in PAH.
Apelins are a family of peptides that activate the apelin receptor (also known as APJ) and have an emerging importance in the physiology and pathophysiology of the cardiovascular system (Yang et al., 2015). Apelin peptides are present in human vascular and cardiac endothelial cells (Kleinz and Davenport, 2004) and plasma, with [Pyr1]apelin-13 identified as the most abundant cardiovascular isoform (De Mota et al., 2004; Maguire et al., 2009; Zhen et al., 2013). Apelins mediate three major actions in vitro. Interaction with the apelin receptor on cardiac myocytes causes increased cardiac contractility and inotropic action, with apelin an order of magnitude more potent than endothelin-1. In vessels with an intact endothelium, apelin acts to release vasodilators that may oppose the actions of vasoconstrictors. We have also shown that removal of endothelium unmasks a constrictor response mediated by apelin receptors present on the vascular smooth muscle (Maguire et al., 2009). Importantly, in healthy volunteers and heart failure patients, the major effect of apelin infused into the forearm in vivo was nitric oxide dependent arterial dilatation (Japp et al., 2008, 2010; Barnes et al., 2013; Brame et al., 2015). In heart failure patients, intracoronary [Pyr1]apelin-13 caused coronary vasodilatation and increased cardiac contractility (Japp et al., 2010; Barnes et al., 2013). Systemic infusions of [Pyr1]apelin-13 in both volunteers and patients increased cardiac index and lowered mean arterial blood pressure and peripheral vascular resistance (Japp et al., 2010; Barnes et al., 2013). Apelin is down-regulated in pulmonary arterial hypertension (PAH), a devastating disease characterized by vascular remodeling resulting in progressive obliteration of the pulmonary circulation, leading to right ventricle (RV) hypertrophy and right heart failure (Alastalo et al., 2011; Chandra et al., 2011). Therefore the apelin receptor may represent a novel target for future drug development.
Human angiotensin converting enzyme 2 (ACE2) has 40% sequence similarity with the C-terminal dipeptidyl-peptidase, ACE (Donoghue et al., 2000; Tipnis et al., 2000). ACE2 is expressed for example in heart, kidney and lung (Donoghue et al., 2000; Hamming et al., 2004) and is implicated in pathological conditions such as heart failure where it is up-regulated (Zisman et al., 2003; Goulter et al., 2004). A major role of ACE2 is to degrade angiotensin II to angiotensin (1–7) which then acts as a beneficial vasodilator and anti-proliferation agent, counter-balancing the actions of the vasoconstrictor angiotensin II (Santos et al., 2003, 2008). ACE2 is also a viral receptor for the severe acute respiratory syndrome coronavirus (Li et al., 2008) which down-regulates the enzyme from the cell surface resulting in angiotensin II-induced lung injury (Kuba et al., 2005). This has been the rational for the development of recombinant human ACE2 (rhACE2) in clinical trials for acute lung injury (Haschke et al., 2013). Interestingly, enhancing ACE2 activity pharmacologically or by gene transfer was effective in preventing or reversing PAH (Shenoy et al., 2011; Dai et al., 2015). Some beneficial actions of ACE2 are thought to be mediated by the conversion of angiotensin II to angiotensin(1–7). However, the possibility of interaction of ACE2 with other peptides was not clear until a screen of over 120 biologically active peptides reported only two others to be hydrolyzed with high catalytic efficiency by ACE2; dynorphin A 1-13, which has no reported vasoactivity and apelin-13, or apelin-36, resulting in the removal of the C-terminal phenylalanine, producing the metabolites apelin-13(1–12) or apelin-36(1–35) (Vickers et al., 2002). The loss of the terminal phenylalanine in apelin has been assumed to be a mechanism of degradation and inactivation of the peptide. Our aim was to understand the impact of this ACE2 cleavage reaction on the apelin signaling pathway.
Specifically, our objectives were firstly to confirm that [Pyr1]apelin-13(1–12) (Figure 1) can be produced by ACE2 hydrolysis of [Pyr1]apelin-13 and find evidence that [Pyr1]apelin-13(1–12) is an endogenous peptide and determine its distribution in human cardiovascular tissues. Secondly, to demonstrate that [Pyr1]apelin-13(1–12) binds to the apelin receptor and can activate down-stream signaling pathways in cell based assays. Thirdly, we have determined that [Pyr1]apelin-13(1–12) retains significant biological activity, compared with our previously reported data (Maguire et al., 2009; Brame et al., 2015) for [Pyr1]apelin-13, in vitro using vascular and cardiac human and rodent tissues and by systemic infusions of [Pyr1]apelin-13(1–12) in vivo in the rat; finally, since the predominant action of apelin infused into the human forearm is vasodilatation, we have performed first-in-man studies with [Pyr1]apelin-13(1–12) to explore the physiological action of the peptide in healthy volunteers. We also wished to explore the structure activity relationship (SAR) of the apelin peptides and therefore additional experiments were performed with the N-terminal extended putative endogenous peptide apelin-17, alanine substituted apelin-13(F13A), and the shorter fragments apelin-13(R10M), apelin-13(R9P) and apelin-13(P9M) (Figure 1). Our data expand our knowledge on the structure activity relationship of apelin peptides and demonstrate the significant biological activity of the ACE2 metabolite [Pyr1]apelin-13(1–12). These data support the hypothesis that therapeutic strategies enhancing ACE2 activity or up-regulation of ACE2 in cardiovascular disease, both of which may result in enhanced breakdown of [Pyr1]apelin-13, may not significantly compromise the beneficial effects of endogenous apelin signaling via generation of [Pyr1]apelin-13(1–12).
Figure 1. Aligned amino acid sequences of apelin peptides. Hydrophobic amino acids are shown in green, uncharged polar amino acids in yellow, basic amino acids in blue and pyroglutamate in red.
Human tissues samples were obtained with informed consent (Papworth Hospital Research Tissue Bank REC08/H0304/56) and local ethical approval (REC05/Q0104/142).
Synthesis of [Pyr1]apelin-13(1–12) from [Pyr1]apelin-13 was confirmed by incubating [Pyr1]apelin-13 (5 nmol) with rhACE2 enzyme (5 pmol, GSK, Ware, UK) in buffer (pH 6.5) containing 2-(N-morpholino)ethanesulfonic acid (MES, 500 mmol/L), NaCl (300 mmol/L) and ZnCl2 (10 μmol/L) for 2 h at 37°C followed by quenching with 10 μmol ethylenediaminetetraacetic acid (EDTA) (see Vickers et al., 2002 for similar protocol). The reaction mixture was analyzed by Maldi-TOF mass spectrometry after samples were desalted using Millipore μC18ZipTip (MA, USA), washed with 5% acetic acid and eluted with CHCA matrix (in 50% aqueous acetonitrile containing 0.1% trifluoroacetic acid) to a stainless steel sample slide. Samples were air dried and analyzed using a Waters Maldi MicroMX time-of-flight mass spectrometer (MA, USA). Calibration was external using polyethylene glycol. The 10 Hz-laser power was just above threshold. The spectra were the sum of 1,000 shots collected from a spiral track of the sample area. Data were processed using Waters MassLynx software (MA, USA). Control reactions included incubation of [Pyr1]apelin-13(1–12) (5 nmol) with rhACE2, incubation of [Pyr1]apelin-13 (5 nmol) or [Pyr1]apelin-13(1–12) (5 nmol) without rhACE2 and incubation of rhACE2 (5 pmol) without peptides.
Peroxidase-anti-peroxidase and dual-labeling immunofluorescent staining were conducted as described (Kleinz et al., 2005) using frozen sections of human cardiomyopathy heart (n = 4), histologically normal (n = 6) and PAH (n = 4) lung. Affinity purified rabbit-anti-[Pyr1]apelin-13(1–12) antiserum (1:25–1:100 dilution) was custom synthesized (antibody raised against the relevant apelin peptide fragment CKGPMP, that lacks the C-terminal phenylalanine) and selectivity confirmed by comparison with the corresponding fragment containing the C-terminal phenylalanine (CKGPMPF) by ELISA (Figure 2A). In contrast the commercially available apelin-12 antibody (Phoenix Pharmaceuticals Inc. CA, USA) crossed reacted with [Pyr1]apelin-13 but not [Pyr1]apelin-13(1–12) (Figure 2B). ACE2 (1:50, R&D Systems, MN, USA and 1:200, Abcam, Cambridge, UK) antisera were also used. Von Willebrand factor (vWF) (1:50, Dako, Glostrup, Denmark) was used as an endothelial marker. The peroxidase stained sections were examined with a bright field microscope (Olympus, Southend-on-Sea, UK) and imaged using a CC12 camera and CellD Soft Imaging System (Olympus), whereas fluorescent staining were imaged using a Leica Scanning Confocal Microscope (TCS SP2, Leica Microsystems, Milton Keynes, UK). Image processing with rolling ball method and histogram redistribution were applied equally to the entire image and channel overlay were carried out using ImageJ.
Figure 2. Selectivity of antisera determined by ELISA. (A) [Pyr1]apelin-13(1–12) antiserum demonstrated selectivity to the antigen CKGPMP (, corresponding to [Pyr1]Apelin-13(1–12)) relative to CKGPMPF (
, corresponding to [Pyr1]Apelin-13). (B) The apelin-12 antiserum demonstrated selectivity to [Pyr1]Apelin-13 (
) relative to [Pyr1]Apelin-13(1–12) (
).
Assays were performed in human heart as previously described (Brame et al., 2015). Briefly, homogenate of human left ventricle (LV) was incubated for 90 min with 0.1 nmol/L [Glp65, Nle75, Tyr77][125I]apelin-13 in assay buffer (mmol/L; Tris 50, MgCl2 5, pH 7.4, 22°C), in the presence of increasing concentrations of [Pyr1]apelin-13, [Pyr1]apelin-13(1–12), apelin-13(F13A), and apelin-17 (0.01 nmol/L–100 μmol/L) or, for SAR, a single concentration (1 μmol/L) apelin-13(R10M), apelin-13(R9P) and apelin-13(P9M). Non-specific binding was defined using 2 μmol/L [Pyr1]apelin-13. Equilibrium was broken by centrifugation (20,000 g for 10 min, 4°C) and pellets washed with Tris-HCl buffer (50 mmol/L, pH 7.4, 4°C), re-centrifuged and pellets counted for detection of bound radioactivity. Competition binding data were analyzed using GraphPad Prism 6 (GraphPad Software, Inc. La Jolla, CA, USA) to obtain values of pKi (the negative log10 of the dissociation constant derived from the IC50 for the competing ligands, the radioligand concentration and radioligand affinity by the Cheng and Prusoff equation). Experiments were performed in triplicate.
Inhibition of cAMP accumulation, β-arrestin recruitment and receptor internalization by apelin isoforms, modified and truncated apelin peptides were studied using cells expressing the human apelin receptor (DiscoverX, CA, USA) as per the manufacturer's instructions. In all assays the resulting chemiluminescent signal was measured as relative light units (RLU) using a LumiLITE™ Microplate Reader (DiscoveRx, Fremont, CA). In the cAMP assay 15 μmol/L forskolin was used to stimulate cAMP production and concentration-response curves were constructed to [Pyr1]apelin-13, [Pyr1]apelin-13(1–12), apelin-17, apelin-13(F13A) (all 1 pmol/L–30 nmol/L), apelin-13(R10M), apelin-13 (R9P) and apelin-13(P9M) (all 1 nmol/L–10 μmol/L). Agonist responses were expressed as a % of the forskolin response. In the β-arrestin and internalization assays concentration-response curves were constructed to [Pyr1]apelin-13, [Pyr1]apelin-13(1–12), apelin-13(F13A), apelin-13(R10M), (all 10 pmol/L–1 μmol/L), apelin-17 (1 pmol/L-100 nmol/L), apelin-13 (R9P) and apelin-13(P9M) (both 1 nmol/L–300 μmol/L). Agonist responses were expressed as a % of the maximum response to [Pyr1]apelin-13. Data were analyzed using a 4-parameter logistic equation using GraphPad Prism 6 to determine values of potency, pD2 (−log10 EC50, where EC50 is the concentration producing half maximal response) and maximum response (EMAX). n-Values are given as number of replicates/number of experiments.
Using the data from the cAMP and β-arrestin assays with the predominant cardiac isoform [Pyr1]apelin-13 used as the reference ligand, the relative activation of G protein-dependent and -independent signaling pathways by [Pyr1]apelin-13, [Pyr1]apelin-13(1–12) and apelin-17 were compared using bias analysis as described by van der Westhuizen et al. (2014).
Additional β-arrestin assay experiments were performed with [Pyr1]apelin-13 that had been incubated with ACE2 as described above. In this assay control concentration-response curves were constructed to [Pyr1]apelin-13 and [Pyr1]apelin-13(1–12) and these were compared to concentration-response curves constructed to both agonists following pre-incubation with rhACE2.
Vascular smooth muscle apelin receptor-mediated contraction was exploited in a bioassay to compare the in vitro potency of apelin peptides. Experiments were carried out as previously described (Maguire, 2002) in endothelium-denuded saphenous vein with concentration-response curves constructed to [Pyr1]apelin-13(1–12) and apelin-13(F13A) (1 pmol/L–300 nmol/L). Agonist responses were expressed as a % of a terminal response to KCl (100 mmol/L). The inotropic action of [Pyr1]apelin-13(1–12) was determined in mouse paced RV (n = 6) and for comparison in two samples of human paced atrial appendage strips as described (Maguire et al., 2009). Data were expressed as % of the terminal response to CaCl2. Data from vascular and cardiac experiments were analyzed using a 4-prameter logistic curve (GraphPad Prism 6) to determine values of pD2 and EMAX.
All experiments were performed according to local ethics committee (University College, London) and Home Office (UK) guidelines under the 1986 Scientific Procedures Act and conformed to the Directive 2010/63/EU. The effects of systemic infusion of [Pyr1]apelin-13(1–12) (incremental bolus doses 1–300 nmol/300 μL) on blood pressure, heart rate, stroke volume and cardiac output were assessed in male Wistar rats (300 ± 25 g body weight) as described (Brame et al., 2015), that were anesthetized with isoflurane (5% induction, 2% maintenance, continuous monitoring throughout). The left carotid artery and right jugular vein were cannulated (0.96 mm polyvinyl chloride tubing). Mean arterial pressure (MAP) was measured throughout the procedure via a pressure transducer (Powerlab AD Instruments, Chalgrove, UK) connected to the arterial line. Baseline hemodynamics were recorded using Chart 7.0 acquisition software and a 16 channel Powerlab system (AD Instruments, Chalgrove, UK) after a 30 min stabilization period. Thoracic echocardiography was performed at a scanning depth of 0–2 cm using a 14 MHz probe (Vivid 7 Dimension, GE Healthcare, Bedford, UK). Pulsed-wave Doppler was used to determine aortic blood flow velocities in the aortic arch. Stroke volume (SV) was determined as the product of the velocity–time integral (VTI) and vessel cross-sectional area. Data from six consecutive cardiac cycles were used to determine heart rate (HR) and a marker of left ventricular contractility, peak velocity (PV). Values of SV and HR were used to calculate cardiac output (CO). Respiration rate was determined from movement of the diaphragm using time-motion (M)-model. At the end of the study rats were euthanized by intravenous pentobarbitone and exsanguination.
Studies were performed in healthy volunteers (n = 12) in the University of Cambridge Vascular Research Unit, Addenbrooke's Hospital, Cambridge, UK. Volunteer characteristics are given in Table 1. This study was carried out in accordance with the recommendations of the National Research Ethics Service Committee East of England-Cambridge Central with written informed consent from all subjects. All subjects gave written informed consent in accordance with the Declaration of Helsinki. The protocol was approved by the National Research Ethics Service Committee East of England-Cambridge Central (REC 11/EE/0305). Changes in forearm blood flow (FBF) in response to [Pyr1]apelin-13(1–12) (1, 10, 100 nmol/min) were measured as previously described (Brame et al., 2015).
Exclusion criteria were ischemic heart disease, respiratory, renal or neurological disease, diabetes mellitus, hypertension, BMI > 30, BMI <18; smoker; pregnant; use of vasoactive medication or NSAIDS/aspirin within 48 h of study; current involvement in other research studies. An Omron HEM705CP oscillimetric sphygmomanometer was used to measure blood pressure and heart rate at baseline and then every 6 min in the contralateral arm. Periodically, a cuff around the upper arm was inflated for ~8 s to 40 mmHg then deflated for 4 s to interrupt venous return and during a 3 min measurement hand circulation was excluded by inflation of wrist cuffs to 200 mmHg. Changes in forearm volume were measured by mercury-in-silastic strain gauge with FBF subsequently expressed as ml/100 ml forearm volume per min. For infusion of peptides via a 16-gauge catheter (Portex, Kent, UK), the brachial artery (non-dominant arm) was cannulated (27-gauge needle, Cooper's Needle Works, Birmingham, UK) under local anesthesia (lignocaine 1%, Hameln Pharmaceuticals Ltd., Gloucester, UK). FBF was measured in both arms and the response to [Pyr1]apelin-13(1–12) presented as absolute change in forearm blood flow from the pre-infusion baseline value.
[Pyr1]apelin-13(1–12), supplied in sealed glass vials and stored at −40°C until required, was allowed to warm to room temperature and diluted with physiological saline to produce stock solutions that were then filtered (0.2 μm flat filter, Portex, Hythe, UK) before further dilution in saline. [Pyr1]apelin-13(1–12) was infused in three incremental doses during each visit. Doses were previously optimized in a pilot study. Each dose was infused for 6 min with a 20 min saline infusion washout period before the next dose was administered. At the end of the study sodium nitroprusside was infused at 3μg/min for 6 min as a positive control followed by a saline infusion as a negative control.
Measurements are mean ± standard error of the mean (SEM). Data analysis and statistical testing were performed using GraphPad Prism 6 to determine values of affinity (pKi) calculated from competition IC50 values using the Cheng and Prusoff equation, potency [pD2 (−log10 EC50, the concentration producing 50% of maximum response)] and maximum response (EMAX) as appropriate. Cell assay pD2 values were compared by one-way ANOVA followed by Tukey's multiple comparison test. For rat in vivo experiments the effect of [Pyr1]apelin-13(1–12) on BP, PV and VTI were expressed as % change from vehicle control in the same animal with other variables (SV and CO) expressed in absolute values. The effect of increasing doses of [Pyr1]apelin-13(1–12) on each parameter was compared to baseline vehicle control using repeated measures one way ANOVA followed by Dunnett's multiple comparison test. Similarly in the human FBF study the response to successive increasing doses of [Pyr1]apelin-13(1–12) was compared to pre-infusion baseline value using repeated measures one-way ANOVA followed by Dunnett's multiple comparison test.
All chemical reagents were purchased from Sigma (Poole, UK), unless otherwise stated. [Pyr1]apelin-13(1–12) was custom synthesized to GLP standard using Fmoc chemistry on a solid phase support matrix to 98% purity by Maldi-TOF Mass spectroscopy and RP-HPLC analysis. Peptides were tested for sterility and demonstrated to be pyrogen free and biological activity confirmed using the β-arrestin assay. All apelin peptides were synthesized by Severn Biotech (Kidderminster, UK).
As shown in the mass spectra, [Pyr1]apelin-13 (Figure 3A) and [Pyr1]apelin-13(1–12) (Figure 3B) alone produced signals at 1533.8 and 1386.7 m/z, respectively. Incubating [Pyr1]apelin-13 with rhACE2 resulted in a signal at the mass-to-charge ratio of 1386.7, corresponding to de novo generation of [Pyr1]apelin-13(1–12), with a weak signal representing the remaining parent peptide (Figure 3C). In contrast, incubating [Pyr1]apelin-13(1–12) with rhACE2 (Figure 3D) did not produce shorter apelin fragments. Finally, rhACE2 enzyme alone (Figure 3E) did not result in interfering signals in the relevant mass range.
Figure 3. Cleavage of [Pyr1]apelin-13 to [Pyr1]apelin-13(1–12) by rhACE2 in vitro. Maldi-TOF spectra for (A) [Pyr1]apelin-13, (B) [Pyr1]apelin-13(1–12), (C) [Pyr1]apelin-13 incubated with rhACE2, (D) [Pyr1]apelin-13(1–12) incubated with rhACE2, (E) rhACE2 alone.
Endogenous [Pyr1]apelin-13(1–12) peptide was detectable in human cardiovascular tissues and localized to the endothelium (Figure 4). [Pyr1]apelin-13(1–12)-like immunoreactivity (-LI) was detected in vascular (Figure 4A) and endocardial (Figure 4B) endothelium identified by positive staining with vWF (Figure 4C) in sections of human cardiomyopathy heart, where ACE2 expression has been reported to be increased (Zisman et al., 2003; Goulter et al., 2004). [Pyr1]apelin-13(1–12)-LI (Figure 4D) and vWF-LI (Figure 4E) were also co-expressed (Figure 4F) in human lung. Importantly, [Pyr1]apelin-13(1–12)-LI (Figure 4G) and ACE2-LI (Figure 4H) co-localized (Figure 4I) in pulmonary blood vessels. Importantly, as apelin is reduced in PAH, the presence of [Pyr1]apelin-13(1–12) was investigated in sections of human PAH lung. Compared to normal lung (Figure 4J), [Pyr1]apelin-13(1–12)-LI was not detectable in the vascular endothelium of PAH lung (Figures 4K,L).
Figure 4. Detection of endogenous [Pyr1]apelin-13(1–12) peptide in human cardiovascular tissues. Bright field microphotographs of [Pyr1]apelin-13(1–12)-LI in (A) vascular and (B) endocardial endothelium and (C) vWF-LI in cardiomyopathy human heart. Confocal microphotographs of (D) [Pyr1]apelin-13(1–12)-LI (green), (E) vWF-LI (red) and (F) their overlay in normal human lung. (G) [Pyr1]apelin-13(1–12)-LI (green), (H) ACE2-LI (red) and (I) their overlay in normal human lung. Bright field microphotographs of (J) [Pyr1]apelin-13(1–12)-LI in normal human lung and (K,L) the absence of [Pyr1]apelin-13(1–12)-LI in PAH human lung tissue. LV, cardiomyopathy human heart; AP, [Pyr1]apelin-13(1–12); NL, normal human lung; PAHL, PAH human lung. Scale bar = 200 μm.
[Pyr1]apelin-13(1–12) competed with [125I]apelin-13 binding with nanomolar affinity, pKi = 8.04 ± 0.06 (n = 3), that was 4-fold lower than the parent molecule [Pyr1]apelin-13 (pKi = 8.83 ± 0.06, n = 3) (Figure 5A). Apelin-13(F13A) exhibited comparable affinity to [Pyr1]apelin-13(1–12) (pKi = 8.07 ± 0.24, n = 3) whereas the extended peptide apelin-17 competed with highest affinity (pKi = 9.63 ± 0.17, n = 3). Of the shorter fragments apelin-13(R10M) (1 μM) competed for 100% of specific binding whereas apelin-13(R9P) and apelin-13(P9M) were less effective competing for 38% and 62% respectively.
Figure 5. [Pyr1]apelin-13(1–12) binds to and activates the human apelin receptor. (A) Competition binding curve for [Pyr1]apelin-13 () and [Pyr1]apelin-13(1–12) (
) in human left ventricle (n = 3). In cell based assays [Pyr1]apelin-13 (
), [Pyr1]apelin-13(1–12) (
), and apelin-17 (
) (B) inhibited forskolin-stimulated cAMP production; (C) induced β-arrestin recruitment and (D) triggered apelin receptor internalization.
In the signaling assays, [Pyr1]apelin-13(1–12) inhibited forskolin-stimulated cAMP production with sub-nanomolar potency, pD2 = 9.30 ± 0.06 (n = 8/3) and was 2-fold less potent than the reference agonist [Pyr1]apelin-13, pD2 = 9.67 ± 0.04 (n = 7/5) but comparable to apelin-13(F13A) (pD2 = 9.54 ± 0.05, n = 2/1). Apelin-17 (pD2 = 10.31 ± 0.28, n = 5/2) was ~5 times more potent than [Pyr1]apelin-13. All ligands fully inhibited cAMP production (Figure 5B). Apelin-13(R10M) retained nanomolar potency (pD2 = 8.70 ± 0.04, n = 3/1) whereas inhibition was incomplete for apelin-13(R9P) and apelin-13(P9M) at 10 μM.
In the G protein-independent β-arrestin recruitment assay [Pyr1]apelin-13 (pD2 = 8.43 ± 0.08, n = 25/10), [Pyr1]apelin-13(1–12) (pD2 = 7.84 ± 0.06, n = 17/6) and apelin-13(F13A) (pD2 = 7.98 ± 0.04, n = 6/2) were 15-40-fold less potent than in the cAMP assay, with [Pyr1]apelin-13 ~4-fold more potent than [Pyr1]apelin-13(1–12) and apelin-13(F13A). Interestingly, unlike the shorter peptides, apelin-17 exhibited comparable potency as an agonist in both the β-arrestin and cAMP assays with a pD2 = 10.26 ± 0.09 (n = 11/4) (Figure 5C). Apelin-13(R10M) (pD2 = 8.26 ± 0.17, n = 9/3) was ~2-fold less potent than [Pyr1]apelin-13 and curves were incomplete for apelin-13(R9P) and aplin-13(P9M) at 300 μM.
Similar to the β-arrestin assay, in the internalization assay [Pyr1]apelin-13(1–12) (pD2 = 8.19 ± 0.06) was 5-fold less potent than [Pyr1]apelin-13 (pD2 = 8.94 ± 0.17). Both peptides were full agonists with comparable efficacy values (EMAX values were 97 ± 2% and 99 ± 3% respectively) (Figure 5D).
Comparing the cAMP and β-arrestin data for the three endogenous peptides with [Pyr1]apelin-13 as the reference agonist, analysis (Table 2) demonstrated a bias factor of 0.24 for [Pyr1]apelin-13(1–12) and 68 for apelin-17 indicating that compared to [Pyr1]apelin-13, [Pyr1]apelin-13(1–12) was 4-fold G protein biased and apelin-17 was markedly β-arrestin biased.
In the β-arrestin recruitment assay [Pyr1]apelin-13 was significantly more potent than [Pyr1]apelin-13(1–12) (p < 0.0001), [Pyr1]apelin-13 incubated with rhACE2 (p < 0.001) or [Pyr1]apelin-13(1–12) incubated with rhACE2 (p < 0.0001). Both of the rhACE2 combinations exhibited comparable potency to [Pyr1]apelin-13(1–12) (p > 0.05) (Table 3). Therefore the reaction product of [Pyr1]apelin-13 and rhACE2 more closely resembled [Pyr1]apelin-13(1–12) than [Pyr1]apelin-13.
Table 3. Relative potencies of apelin peptides without and with pre-incubation with recombinant human ACE2 in a β-arrestin assay.
[Pyr1]apelin-13(1–12) (pD2 = 9.63 ± 0.35, EMAX = 24 ± 6%, n = 9) and apelin-13(F13A) (pD2 = 9.72 ± 0.36, EMAX = 23 ± 4%, n = 9) contracted saphenous vein with comparable sub-nanomolar potencies and maximum responses (Figure 6A) to the data that we had previously obtained with [Pyr1]apelin-13 in this assay (Brame et al., 2015).
Figure 6. Responses to (A) [Pyr1]apelin-13(1–12) (, n = 9) and apelin-13(F13A) (
, n = 9) in endothelium-denuded human saphenous vein in vitro. (B) Inotropic responses to (B) [Pyr1]apelin-13(1–12) (n = 6) and (C) isoprenaline (n = 4) in mouse paced right ventricle.
In mouse paced RV [Pyr1]apelin-13(1–12) produced a concentration-dependent increase in force of contraction with pD2 = 11.68 ± 0.33, EMAX 49 ± 16% CaCl2 (n = 6) (Figure 6B), compared to isoprenaline with pD2 = 7.88 ± 0.42, EMAX = 78 ± 17% CaCl2 (n = 4) (Figure 6C). Limited human atrial appendage strips were available to test for the inotropic action of [Pyr1]apelin-13(1–12). In tissue from two patients [Pyr1]apelin-13(1–12) acted as a positive inotrope with a sub-nanomolar potency value (pD2 = 9.61, EMAX = 38% CaCl2, n = 2).
In anesthetized rat, [Pyr1]apelin-13(1–12) (n = 5) elicited a dose-dependent decrease in BP (Figure 7A). The decrease in BP was significantly different (P < 0.0001) from baseline at doses of 10–300 nmol. Other cardiac parameters, SV, CO, PV and VTI were not altered by [Pyr1]apelin-13(1–12) (Figures 7B–E).
Figure 7. In vivo actions of [Pyr1]apelin-13(1–12) in rat. (A) Administration of intravenous [Pyr1]apelin-13(1–12) (, n = 5) resulted in a significant dose-dependent decrease in blood pressure in rat in vivo. Other parameters (B) stroke volume (SV), (C) cardiac output (CO), (D) peak velocity (PV) and velocity-time interval (VTI) were unaffected at any dose of [Pyr1]apelin-13(1–12). Significantly different from baseline; *P < 0.05, **P < 0.01, ***P < 0.001. One-way ANOVA repeated measures compared to baseline with Dunnett's multiple comparison test.
In human volunteers (n = 12), [Pyr1]apelin-13(1–12) (Figure 8) produced comparable dose-dependent increases in forearm blood flow to that which we have shown for [Pyr1]apelin-13 (Brame et al., 2015). No significant effects of either peptide on heart rate or blood pressure were observed at any dose (data not shown).
Figure 8. In vivo actions of [Pyr1]apelin-13(1–12) in human volunteers. Infusion of [Pyr1]apelin-13(1–12) in healthy human volunteers (, n = 12) resulted in a dose-dependent increase in forearm blood flow. Significantly different from baseline; *P < 0.05, **P < 0.01. One-way ANOVA repeated measures compared to baseline with Dunnett's multiple comparison test.
We have characterized the synthesis, receptor pharmacology and functional activity of the ACE2 metabolite of [Pyr1]apelin-13, [Pyr1]apelin-13(1–12), in vitro and in vivo. For the first time we have detected endogenous [Pyr1]apelin-13(1–12) in human cardiovascular tissues and demonstrated biological activity of this metabolite in vitro and in a first-in-man study.
Apelin-13 and apelin-36 peptides were previously reported to be substrates of purified human ACE2 enzyme (Vickers et al., 2002). In this study, we confirmed (Wang et al., 2016) that ACE2 also catalyzed the conversion of [Pyr1]apelin-13, the predominant cardiovascular form of apelin, to [Pyr1]apelin-13(1–12) in vitro. This result was not unexpected since the PMPF sequence of [Pyr1]apelin-13 (Figure 1) conforms to the consensus sequence for ACE2-mediated hydrolysis; Pro-X(1–3residues)-Pro-Hydrophobic (Vickers et al., 2002). In support, in the β-arrestin assay the product of de novo ACE2 metabolism demonstrated comparable potency to synthetic [Pyr1]apelin-13(1−12)(Table 3). Our results are consistent with a study reporting that a small proportion of [Pyr1]apelin-13 was cleaved into [Pyr1]apelin-13(1–12) in rat in vivo (Murza et al., 2014).
We have previously demonstrated endothelial expression of apelin (Kleinz and Davenport, 2004; Kleinz et al., 2005) using an antibody specific for apelin isoforms containing the C-terminal phenylalanine (Figure 2B). This is consistently reported by others using alternative strategies to detect apelin expression (Sheikh et al., 2008). ACE2 is also expressed in the endothelium (Donoghue et al., 2000; Hamming et al., 2004), raising the possibility of endothelial processing of [Pyr1]apelin-13 to produce [Pyr1]apelin-13(1–12). For this study, we generated an antibody that cross-reacts with apelin isoforms without the C-terminal phenylalanine that has allowed selective identification of [Pyr1]apelin-13(1–12) in human heart and lung. [Pyr1]apelin-13(1–12)-LI was specifically localized to the endothelium, where the apelin receptor was also expressed, suggesting the possibility of autocrine signaling by this metabolite in these tissues. Importantly, in human PAH lung, where endothelial apelin expression is known to be reduced (Alastalo et al., 2011; Kim et al., 2013), no staining for [Pyr1]apelin-13(1–12) was detected.
ACE2-mediated hydrolysis has been assumed to inactivate apelin peptides. Wang and colleagues have recently reported that the ACE2 product of [Pyr1]apelin-13 exhibits reduced or absent cardiovascular actions compared to the parent molecule (Wang et al., 2016). However, emerging evidence from structure activity studies suggested that the C-terminal phenylalanine was not a critical residue for apelin biological activity (Fan et al., 2003; Medhurst et al., 2003) and our hypothesis was that compared to [Pyr1]apelin-13, [Pyr1]apelin-13(1–12) may retain significant activity. Indeed, our results showed that [Pyr1]apelin-13(1–12) has nanomolar affinity for the native human apelin receptor exhibiting only a 3-fold reduction in binding affinity compared the parent peptide. This is consistent with previous studies where the C-terminal phenylalanine of [Pyr1]apelin-13 or apelin-13 was replaced with alanine (F13A) (Fan et al., 2003; Medhurst et al., 2003) or removed from apelin-17 (K16P)(El Messari et al., 2004; Iturrioz et al., 2010) with only minimal loss of receptor affinity. Similarly, substitution of the C-terminal phenylalanine of [Pyr1]apelin-13 with D-phenylalanine resulted in only a 20-fold decrease in receptor binding affinity, which was modest compared to substitution of other residues known to be important for binding (Murza et al., 2012). Overall, these studies show that loss of the C-terminal phenylalanine from apelin isoforms does not significantly alter receptor binding.
The apelin receptor is Gαi-coupled (Habata et al., 1999) therefore we next showed that [Pyr1]apelin-13(1–12) inhibited forskolin-stimulated cAMP with potency only 2-fold less than [Pyr1]apelin-13. This is in agreement with the previous reports of alanine and D-phenylalanine substituted [Pyr1]apelin-13 (Medhurst et al., 2003; Murza et al., 2012) and K16P (El Messari et al., 2004; Iturrioz et al., 2010; Ceraudo et al., 2014). Therefore, our study confirms that Gαi-induced signaling is preserved in response to [Pyr1]apelin-13(1–12) receptor binding. In addition to G protein-dependent signaling, activation of the apelin receptor causes β-arrestin recruitment and receptor internalization (Evans et al., 2001). In these assays, [Pyr1]apelin-13(1–12) again behaved as a full agonist at the human apelin receptor with only a 5-fold reduction in potency compared to [Pyr1]apelin-13. This ability to induce receptor internalization without the C-terminal phenylalanine was reported for apelin-13(F13A) (Fan et al., 2003), however, K16P was shown by a second group to exhibit markedly reduced potency and efficacy in β-arrestin recruitment and internalization of rat apelin receptor (El Messari et al., 2004; Iturrioz et al., 2010; Ceraudo et al., 2014). Importantly, compared to [Pyr1]apelin-13, [Pyr1]apelin-13(1–12) is not a biased agonist in G protein-dependent and -independent signaling.
Apelins are modulators of vascular tone and cardiac contractility in vitro and in vivo (Japp et al., 2008, 2010; Maguire et al., 2009; Barnes et al., 2013; Brame et al., 2015). Therefore, as expected we found that [Pyr1]apelin-13(1–12) contracted human saphenous vein with equal potency and efficacy compared with our previous data for [Pyr1]apelin-13 (Maguire et al., 2009; Brame et al., 2015) and was a potent inotrope in mouse paced right ventricle and human paced atria. We next investigated the in vivo actions of [Pyr1]apelin-13(1–12). In anesthetized rat compared to our previously published data for [Pyr1]apelin-13 (Brame et al., 2015), the ACE2 metabolite caused a smaller but significant drop in blood pressure which contrasts with Wang and colleagues (Wang et al., 2016) who reported that [Pyr1]apelin-13, but not [Pyr1]apelin-13(1–12), showed a sustained effect on blood pressure for up to 60 min after administration to mice in vivo, although both peptides appear to show an initial comparable reduction in blood pressure over the first 10–15 min in these experiments. Interestingly, these authors also showed that [Pyr1]apelin-13 is rapidly cleaved by ACE2 in vitro and in vivo suggesting that the ACE2 cleavage product may contribute to their observed sustained response to [Pyr1]apelin-13 and as might be expected the half-life of the cleavage product is likely to be short and therefore the response to infused [Pyr1]apelin-13(1–12) is observed for only the initial 10~15 min of the experiment. In agreement with this hypothesis, in our rat studies (Brame et al., 2015) the half-life of [Pyr1]apelin-13 was less than 3 min and in humans less than 8 min (Japp et al., 2008). Importantly, in a first-in-man study, infusion of [Pyr1]apelin-13(1–12) resulted in an increase in forearm blood flow that was the same magnitude as we had previously obtained with [Pyr1]apelin-13 (Brame et al., 2015), confirming that this metabolite is a vasodilator in humans in vivo.
The 55-amino acid proapelin, derived from the 77-residue prepropeptide, contains a number of paired basic amino acids residues that are possible cleavage sites for endopeptidases to produce 13 to 36-residue isoforms (Tatemoto et al., 1998; Habata et al., 1999). While the identities of these peptidases remain unknown, a study reported the direct cleavage of proapelin to apelin-13 by proprotein convertase subtilisin/kexin 3 (PCSK3, or furin), bypassing the generation of longer isoforms (Shin et al., 2013). Post-translational modification results in the predominant apelin isoform detected in human cardiovascular tissue and plasma, [Pyr1]apelin-13 (De Mota et al., 2004; Kleinz and Davenport, 2004; Maguire et al., 2009; Zhen et al., 2013). The putative ACE2 metabolite of this cardiac peptide, [Pyr1]apelin-13(1–12), is the focus of our study but we have also explored the pharmacology of several other important apelin isoforms to better understand the apelin structure activity relationships, namely the minimum active fragment apelin-13(R10M), apelin-13(F13A) and apelin-17.
As described above, amino acid substitution studies have proposed a consensus on those amino acids within apelin-13 that are important for receptor binding and activation (Narayanan et al., 2015). The C-terminal phenylalanine (F13), adjacent proline and the N-terminal pyroglutamic acid were not identified as important. We have investigated whether the truncated peptides apelin-13(R10M), apelin-13(R9P) and apelin-13(P9M) retained significant binding and functional activity with the hypothesis that apelin-13(R10M) was likely to represent the minimum active fragment as it was the shortest fragment containing all the identified important amino acids. Results of the competition binding experiments and β-arrestin recruitment assays supported this hypothesis as do data from previous publications showing diminished Gαi-mediated signaling and calcium mobilization when the methionine (position 11 in apelin-13) was removed (Medhurst et al., 2003; Zhang et al., 2014), while others have demonstrated activity of apelin-12 (apelin-13 without the N-terminal pyroglutamic acid) in vivo (Pisarenko et al., 2011). Residues arginine 2 and methionine 11 are indispensable as they are required to form the crucial RPRL motif or provide steric volume (Langelaan et al., 2009; Macaluso and Glen, 2010; Gerbier et al., 2015). In contrast, although the C-terminal phenylalanine has been shown to make specific contacts within the binding pocket of the apelin receptor (Iturrioz et al., 2010), our experimental data suggested that its removal did not abolish binding or functional activity.
Early alanine scanning studies indicated that replacing the C-terminal phenylalanine with alanine did not abolish Gαi-mediated signaling, calcium mobilization (Medhurst et al., 2003) and receptor internalization (Fan et al., 2003). However, conflicting data have been reported for F13A, suggesting that it was an antagonist of the apelin receptor in terms of Gαi-mediated signaling ([Pyr1]apelin-13(F13A); De Mota et al., 2000) and hypotensive effect (apelin-13(F13A); Lee et al., 2005). In this study, we found that apelin-13(F13A) resembled [Pyr1]apelin-13(1–12) in receptor binding, cell based assays and the vasoconstrictor bioassay. Based on our results apelin-13(F13A) behaves as an apelin agonist with no evidence of receptor antagonism.
Apelin-17, despite its unclear biosynthetic pathway, has been reported to have equal or higher binding affinity and potency in inhibiting cAMP accumulation and inducing receptor internalization compared with [Pyr1]apelin-13 (Medhurst et al., 2003; El Messari et al., 2004). We investigated apelin-17 in our assays and consistently found higher binding affinity and higher potency than [Pyr1]apelin-13 in the cAMP and β-arrestin assays. Intriguingly, apelin-17 appeared to be more biased for β-arrestin compared to G protein signaling relative to the reference agonist [Pyr1]apelin-13. This suggests that N-terminal extension of apelin-13 may result in peptides that stabilize different conformations of the apelin receptor and may be a mechanism by which apelin receptor activation is fine-tuned at the cellular level.
Past studies have suggested a possible link between apelin, its receptor, ACE2 and the renin angiotensin system. For example, apelin knockout mice showed aging or stress-associated cardiac contractility defects, similar to the cardiac phenotype of ACE2 knockout mice (Kuba et al., 2007) and apelin receptor knockout mice are more sensitive to the pressor effect of angiotensin II (Ishida et al., 2004). Interestingly, ACE2 expression is reduced in apelin knockout mice and apelin up-regulates ACE2 expression, indicating that apelin may reciprocally impact ACE2 (Sato et al., 2013). At the receptor level, the apelin receptor has been shown to physically interact with the angiotensin receptor type I (AT1R) (Chun et al., 2008), forcing it into a low affinity state and reducing the binding and signaling of angiotensin II (Siddiquee et al., 2013). Reduced apelin expression due to heart failure or angiotensin II administration can be restored by AT1R blockade (Iwanaga et al., 2006). The opposing effects of apelin/ACE2 and angiotensin II have been shown in health and diseases such as heart failure, atherosclerosis and obesity/diabetes (Ashley et al., 2006; Gurzu et al., 2006; Iwanaga et al., 2006; Zhong et al., 2007; Chun et al., 2008; Barnes et al., 2013; Siddiquee et al., 2013) (Figure 9). Our study contributes further evidence that ACE2, that is up-regulated in disease, acting on [Pyr1]apelin-13 may result in the generation of the active metabolite, [Pyr1]apelin-13(1–12), and therefore a compensatory maintenance of apelin receptor signaling. However, a limitation of our study is that we have not measured levels of apelin peptides in plasma of patients receiving rhACE2 therapy, but we would hypothesize that levels of [Pyr1]apelin-13(1–12) would be elevated and [Pyr1]apelin-13 decreased compared to controls.
Figure 9. Schematic diagram showing the interactions between apelin/apelin receptor with ACE2 of the renin angiotensin system. New contributions of this study are shown in red arrows. Ang, angiotensin; APJ, apelin receptor; AT1R, angiotensin receptor type I; MAS, Mas receptor.
In conclusion, this study confirmed that ACE2 cleaves [Pyr1]apelin-13 into [Pyr1]apelin-13(1–12) and has demonstrated biological activity of [Pyr1]apelin-13(1–12) at the human apelin receptor in vitro and in the cardiovascular system of rat and human in vivo. The results also clarify R10M as the shortest active apelin fragment, apelin-13(F13A) as an agonist and apelin-17 as a more potent agonist than [Pyr1]apelin-13. Therefore, our study shows that reported enhanced ACE2 activity in cardiovascular disease should not significantly compromise the beneficial effects of apelin based therapies for example in PAH.
All authors contributed either to the conception or design of the work (PY, AB, AD, MS, RG, JC, IW, APD, and JM), or acquired and analyzed data or interpreted data (PY, RK, AB, AD, MS, APD, and JM). All authors agree to be accountable for all aspects of the work.
This work was supported by the Wellcome Trust Programme in Metabolic and Cardiovascular Disease [096822/Z/11/Z to APD]; Wellcome Trust [WT 107715 to APD]; Medical Research Council [MC_PC_14116 to APD]; Wellcome Trust Programme in Translational Medicines and Therapeutics [085686 to APD], and in part by the National Institute for Health Research Cambridge Biomedical Research Centre; and the Pulmonary Hypertension Association UK.
The authors declare that the research was conducted in the absence of any commercial or financial relationships that could be construed as a potential conflict of interest.
We thank the theater and consultant staff of Papworth hospital for tissue collection.
Alastalo, T. P., Li, M., Perez Ve, J., Pham, D., Sawada, H., Wang, J. K., et al. (2011). Disruption of PPARγ/β-catenin-mediated regulation of apelin impairs BMP-induced mouse and human pulmonary arterial EC survival. J. Clin. Invest. 121, 3735–3746. doi: 10.1172/JCI43382
Ashley, E., Chun, H. J., and Quertermous, T. (2006). Opposing cardiovascular roles for the angiotensin and apelin signaling pathways. J. Mol. Cell. Cardiol. 41, 778–781. doi: 10.1016/j.yjmcc.2006.08.013
Barnes, G. D., Alam, S., Carter, G., Pedersen, C. M., Lee, K. M., Hubbard, T. J., et al. (2013). Sustained cardiovascular actions of APJ agonism during renin-angiotensin system activation and in patients with heart failure. Circ. Heart Fail. 6, 482–491. doi: 10.1161/CIRCHEARTFAILURE.111.000077
Brame, A. L., Maguire, J. J., Yang, P., Dyson, A., Torella, R., Cheriyan, J., et al. (2015). Design, characterization, and first-in-human study of the vascular actions of a novel biased apelin receptor agonist. Hypertension. 65, 834–840. doi: 10.1161/HYPERTENSIONAHA.114.05099
Ceraudo, E., Galanth, C., Carpentier, E., Banegas-Font, I., Schonegge, A. M., Alvear-Perez, R., et al. (2014). Biased signaling favoring gi over β-arrestin promoted by an apelin fragment lacking the C-terminal phenylalanine. J. Biol. Chem. 289, 24599–24610. doi: 10.1074/jbc.M113.541698
Chandra, S. M., Razavi, H., Kim, J., Agrawal, R., Kundu, R. K., de Jesus Perez, V., et al. (2011). Disruption of the apelin-APJ system worsens hypoxia-induced pulmonary hypertension. Arterioscler. Thromb. Vasc. Biol. 31, 814–820. doi: 10.1161/ATVBAHA.110.219980
Chun, H. J., Ali, Z. A., Kojima, Y., Kundu, R. K., Sheikh, A. Y., Agrawal, R., et al. (2008). Apelin signaling antagonizes Ang II effects in mouse models of atherosclerosis. J. Clin. Invest. 118, 3343–3354. doi: 10.1172/jci34871
Dai, H., Jiang, L., Xiao, Z., and Guang, X. (2015). ACE2-angiotensin-(1-7)-Mas axis might be a promising therapeutic target for pulmonary arterial hypertension. Nat. Rev. Cardiol. 12:374. doi: 10.1038/nrcardio.2015.6-c1
De Mota, N., Lenkei, Z., and Llorens-Cortès, C. (2000). Cloning, pharmacological characterization and brain distribution of the rat apelin receptor. Neuroendocrinology 72, 400–407. doi: 10.1159/000054609
De Mota, N., Reaux-Le Goazigo, A., El Messari, S., Chartrel, N., Roesch, D., Dujardin, C., et al. (2004). Apelin, a potent diuretic neuropeptide counteracting vasopressin actions through inhibition of vasopressin neuron activity and vasopressin release. Proc. Natl. Acad. Sci. U.S.A. 101, 10464–10469. doi: 10.1073/pnas.0403518101
Donoghue, M., Hsieh, F., Baronas, E., Godbout, K., Gosselin, M., Stagliano, N., et al. (2000). A novel angiotensin-converting enzyme-related carboxypeptidase (ACE2) converts angiotensin I to angiotensin 1–9. Circ. Res. 87, E1–E9. doi: 10.1161/01.RES.87.5.e1
El Messari, S., Iturrioz, X., Fassot, C., De Mota, N., Roesch, D., and Llorens-Cortes, C. (2004). Functional dissociation of apelin receptor signaling and endocytosis: implications for the effects of apelin on arterial blood pressure. J. Neurochem. 90, 1290–1301. doi: 10.1111/j.1471-4159.2004.02591.x
Evans, N. A., Groarke, D. A., Warrack, J., Greenwood, C. J., Dodgson, K., Milligan, G., et al. (2001). Visualizing differences in ligand-induced beta-arrestin-GFP interactions and trafficking between three recently characterized G protein-coupled receptors. J. Neurochem. 77, 476–485. doi: 10.1046/j.1471-4159.2001.00269.x
Fan, X., Zhou, N., Zhang, X., Mukhtar, M., Lu, Z., Fang, J., et al. (2003). Structural and functional study of the apelin-13 peptide, an endogenous ligand of the HIV-1 coreceptor, APJ. Biochemistry 42, 10163–10168. doi: 10.1021/bi030049s
Gerbier, R., Leroux, V., Couvineau, P., Alvear-Perez, R., Maigret, B., Llorens-Cortes, C., et al. (2015). New structural insights into the apelin receptor: identification of key residues for apelin binding. FASEB J. 29, 314–322. doi: 10.1096/fj.14-256339
Goulter, A. B., Goddard, M. J., Allen, J. C., and Clark, K. L. (2004). ACE2 gene expression is up-regulated in the human failing heart. BMC Med. 2:19. doi: 10.1186/1741-7015-2-19
Gurzu, B., Petrescu, B. C., Costuleanu, M., and Petrescu, G. (2006). Interactions between apelin and angiotensin II on rat portal vein. J. Renin Angiotensin Aldosterone Syst. 7, 212–216. doi: 10.3317/jraas.2006.040
Habata, Y., Fujii, R., Hosoya, M., Fukusumi, S., Kawamata, Y., Hinuma, S., et al. (1999). Apelin, the natural ligand of the orphan receptor APJ, is abundantly secreted in the colostrum. Biochim. Biophys. Acta 1452, 25–35. doi: 10.1016/S0167-4889(99)00114-7
Hamming, I., Timens, W., Bulthuis, M. L., Lely, A. T., Navis, G. J., and van Goor, H. (2004). Tissue distribution of ACE2 protein, the functional receptor for SARS coronavirus. A first step in understanding SARS pathogenesis. J. Pathol. 203, 631–637. doi: 10.1002/path.1570
Haschke, M., Schuster, M., Poglitsch, M., Loibner, H., Salzberg, M., Bruggisser, M., et al. (2013). Pharmacokinetics and pharmacodynamics of recombinant human angiotensin-converting enzyme 2 in healthy human subjects. Clin. Pharmacokinet. 52, 783–792. doi: 10.1007/s40262-013-0072-7
Ishida, J., Hashimoto, T., Hashimoto, Y., Nishiwaki, S., Iguchi, T., Harada, S., et al. (2004). Regulatory roles for APJ, a seven-transmembrane receptor related to angiotensin-type 1 receptor in blood pressure in vivo. J. Biol. Chem. 279, 26274–26279. doi: 10.1074/jbc.M404149200
Iturrioz, X., Gerbier, R., Leroux, V., Alvear-Perez, R., Maigret, B., and Llorens-Cortes, C. (2010). By interacting with the C-terminal Phe of apelin, Phe255 and Trp259 in helix VI of the apelin receptor are critical for internalization. J. Biol. Chem. 285, 32627–32637. doi: 10.1074/jbc.M110.127167
Iwanaga, Y., Kihara, Y., Takenaka, H., and Kita, T. (2006). Down-regulation of cardiac apelin system in hypertrophied and failing hearts: possible role of angiotensin II-angiotensin type 1 receptor system. J. Mol. Cell. Cardiol. 41, 798–806. doi: 10.1016/j.yjmcc.2006.07.004
Japp, A. G., Cruden, N. L., Amer, D. A., Li, V. K., Goudie, E. B., Johnston, N. R., et al. (2008). Vascular effects of apelin in vivo in man. J. Am. Coll. Cardiol. 52, 908–913. doi: 10.1016/j.jacc.2008.06.013
Japp, A. G., Cruden, N. L., Barnes, G., van Gemeren, N., Mathews, J., Adamson, J., et al. (2010). Acute cardiovascular effects of apelin in humans: potential role in patients with chronic heart failure. Circulation 121, 1818–1827. doi: 10.1161/CIRCULATIONAHA.109.911339
Kim, J., Kang, Y., Kojima, Y., Lighthouse, J. K., Hu, X., Aldred, M. A., et al. (2013). An endothelial apelin-FGF link mediated by miR-424 and miR-503 is disrupted in pulmonary arterial hypertension. Nat. Med. 19, 74–82. doi: 10.1038/nm.3040
Kleinz, M. J., and Davenport, A. P. (2004). Immunocytochemical localization of the endogenous vasoactive peptide apelin to human vascular and endocardial endothelial cells. Regul. Pept. 118, 119–125. doi: 10.1016/j.regpep.2003.11.002
Kleinz, M. J., Skepper, J. N., and Davenport, A. P. (2005). Immunocytochemical localisation of the apelin receptor, APJ, to human cardiomyocytes, vascular smooth muscle and endothelial cells. Regul. Pept. 126, 233–240. doi: 10.1016/j.regpep.2004.10.019
Kuba, K., Imai, Y., Rao, S., Gao, H., Guo, F., Guan, B., et al. (2005). A crucial role of angiotensin converting enzyme 2 (ACE2) in SARS coronavirus-induced lung injury. Nat. Med. 11, 875–879. doi: 10.1038/nm1267
Kuba, K., Zhang, L., Imai, Y., Arab, S., Chen, M., Maekawa, Y., et al. (2007). Impaired heart contractility in Apelin gene-deficient mice associated with aging and pressure overload. Circ. Res. 101, e32–e42. doi: 10.1161/CIRCRESAHA.107.158659
Langelaan, D. N., Bebbington, E. M., Reddy, T., and Rainey, J. K. (2009). Structural insight into G-protein coupled receptor binding by apelin. Biochemistry 48, 537–548. doi: 10.1021/bi801864b
Lee, D. K., Saldivia, V. R., Nguyen, T., Cheng, R., George, S. R., and O'Dowd, B. F. (2005). Modification of the terminal residue of apelin-13 antagonizes its hypotensive action. Endocrinology 146, 231–236. doi: 10.1210/en.2004-0359
Li, F., Li, L., Qin, X., Pan, W., Feng, F., Chen, F., et al. (2008). Apelin-induced vascular smooth muscle cell proliferation: the regulation of cyclin D1. Front. Biosci. 13, 3786–3792. doi: 10.2741/2967
Macaluso, N. J., and Glen, R. C. (2010). Exploring the ‘RPRL’ motif of apelin-13 through molecular simulation and biological evaluation of cyclic peptide analogues. Chem. Med. Chem. 5, 1247–1253. doi: 10.1002/cmdc.201000061
Maguire, J. J. (2002). Endothelin-converting enzyme activity in vascular smooth muscle preparations in vitro. Methods Mol. Biol. 206, 165–177. doi: 10.1385/1-59259-289-9:165
Maguire, J. J., Kleinz, M. J., Pitkin, S. L., and Davenport, A. P. (2009). [Pyr1]apelin-13 identified as the predominant apelin isoform in the human heart: vasoactive mechanisms and inotropic action in disease. Hypertension 54, 598–604. doi: 10.1161/HYPERTENSIONAHA.109.134619
Medhurst, A. D., Jennings, C. A., Robbins, M. J., Davis, R. P., Ellis, C., Winborn, K. Y., et al. (2003). Pharmacological and immunohistochemical characterization of the APJ receptor and its endogenous ligand apelin. J. Neurochem. 84, 1162–1172. doi: 10.1046/j.1471-4159.2003.01587.x
Murza, A., Belleville, K., Longpré, J. M., Sarret, P., and Marsault, E. (2014). Stability and degradation patterns of chemically modified analogs of apelin-13 in plasma and cerebrospinal fluid. Biopolymers 101, 297–303. doi: 10.1002/bip.22498
Murza, A., Parent, A., Besserer-Offroy, E., Tremblay, H., Karadereye, F., Beaudet, N., et al. (2012). Elucidation of the structure-activity relationships of apelin: influence of unnatural amino acids on binding, signaling, and plasma stability. ChemMedChem 7, 318–325 doi: 10.1002/cmdc.201100492
Narayanan, S., Harris, D. L., Maitra, R., and Runyon, S. P. (2015). Regulation of the apelinergic system and its potential in cardiovascular disease: peptides and small molecules as tools for discovery. J. Med. Chem. 58, 7913–7927. doi: 10.1021/acs.jmedchem.5b00527
Pisarenko, O. I., Serebryakova, L. I., Pelogeykina, Y. A., Studneva, I. M., Khatri, D. N., Tskitishvili, O. V., et al. (2011). In vivo reduction of reperfusion injury to the heart with apelin-12 peptide in rats. Bull. Exp. Biol. Med. 152, 79–82. doi: 10.1007/s10517-011-1459-9
Santos, R. A., Ferreira, A. J., Simões, E., and Silva, A. C. (2008). Recent advances in the angiotensin-converting enzyme 2-angiotensin(1–7)-Mas axis. Exp. Physiol. 93, 519–527. doi: 10.1113/expphysiol.2008.042002
Santos, R. A., Simoes e Silva, A. C., Maric, C., Silva, D. M., Machado, R. P., de Buhr, I., et al. (2003). Angiotensin-(1–7) is an endogenous ligand for the G protein-coupled receptor Mas. Proc. Natl. Acad. Sci. U.S.A. 100, 8258–8263. doi: 10.1073/pnas.1432869100
Sato, T., Suzuki, T., Watanabe, H., Kadowaki, A., Fukamizu, A., Liu, P. P., et al. (2013). Apelin is a positive regulator of ACE2 in failing hearts. J. Clin. Invest. 123, 5203–5211. doi: 10.1172/JCI69608
Sheikh, A. Y., Chun, H. J., Glassford, A. J., Kundu, R. K., Kutschka, I., Ardigo, D., et al. (2008). In vivo genetic profiling and cellular localization of apelin reveals a hypoxia-sensitive, endothelial-centered pathway activated in ischemic heart failure. Am. J. Physiol. Heart Circ. Physiol. 294, H88–H98. doi: 10.1152/ajpheart.00935.2007
Shenoy, V., Qi, Y., Katovich, M. J., and Raizada, M. K. (2011). ACE2, a promising therapeutic target for pulmonary hypertension. Curr. Opin. Pharmacol. 11, 150–155. doi: 10.1016/j.coph.2010.12.002
Shin, K., Pandey, A., Liu, X. Q., Anini, Y., and Rainey, J. K. (2013). Preferential apelin-13 production by the proprotein convertase PCSK3 is implicated in obesity. FEBS Open Bio 3, 328–333. doi: 10.1016/j.fob.2013.08.001
Siddiquee, K., Hampton, J., McAnally, D., May, L., and Smith, L. (2013). The apelin receptor inhibits the angiotensin II type 1 receptor via allosteric trans-inhibition. Br. J. Pharmacol. 168, 1104–1117. doi: 10.1111/j.1476-5381.2012.02192.x
Tatemoto, K., Hosoya, M., Habata, Y., Fujii, R., Kakegawa, T., Zou, M. X., et al. (1998). Isolation and characterization of a novel endogenous peptide ligand for the human APJ receptor. Biochem. Biophys. Res. Commun. 251, 471–476. doi: 10.1006/bbrc.1998.9489
Tipnis, S. R., Hooper, N. M., Hyde, R., Karran, E., Christie, G., and Turner, A. J. (2000). A human homolog of angiotensin-converting enzyme. Cloning and functional expression as a captopril-insensitive carboxypeptidase. J. Biol. Chem. 275, 33238–33243. doi: 10.1074/jbc.M002615200
van der Westhuizen, E. T., Breton, B., Christopoulos, A., and Bouvier, M. (2014). Quantification of ligand bias for clinically relevant β2-adrenergic receptor ligands: implications for drug taxonomy. Mol. Pharmacol. 85, 492–509. doi: 10.1124/mol.113.088880
Vickers, C., Hales, P., Kaushik, V., Dick, L., Gavin, J., Tang, J., et al. (2002). Hydrolysis of biological peptides by human angiotensin-converting enzyme-related carboxypeptidase. J. Biol. Chem. 277, 14838–14843. doi: 10.1074/jbc.M200581200
Wang, W., McKinnie, S. M., Farhan, M., Paul, M., McDonald, T., McLean, B., et al. (2016). Angiotensin-converting enzyme 2 metabolizes and partially inactivates Pyr-Apelin-13 and Apelin-17: physiological effects in the cardiovascular system. Hypertension 68, 365–377. doi: 10.1161/HYPERTENSIONAHA.115.06892
Yang, P., Maguire, J. J., and Davenport, A. P. (2015). Apelin, Elabela/Toddler, and biased agonists as novel therapeutic agents in the cardiovascular system. Trends Pharmacol. Sci. 36, 560–567. doi: 10.1016/j.tips.2015.06.002
Zhang, Y., Maitra, R., Harris, D. L., Dhungana, S., Snyder, R., and Runyon, S. P. (2014). Identifying structural determinants of potency for analogs of apelin-13: integration of C-terminal truncation with structure-activity. Bioorg. Med. Chem. 22, 2992–2997. doi: 10.1016/j.bmc.2014.04.001
Zhen, E. Y., Higgs, R. E., and Gutierrez, J. A. (2013). Pyroglutamyl apelin-13 identified as the major apelin isoform in human plasma. Anal. Biochem. 442, 1–9. doi: 10.1016/j.ab.2013.07.006
Zhong, J. C., Yu, X. Y., Huang, Y., Yung, L. M., Lau, C. W., and Lin, S. G. (2007). Apelin modulates aortic vascular tone via endothelial nitric oxide synthase phosphorylation pathway in diabetic mice. Cardiovasc. Res.74, 388–395. doi: 10.1016/j.cardiores.2007.02.002
Zisman, L. S., Keller, R. S., Weaver, B., Lin, Q., Speth, R., Bristow, M. R., et al. (2003). Increased angiotensin-(1–7)-forming activity in failing human heart ventricles: evidence for upregulation of the angiotensin-converting enzyme Homologue ACE2. Circulation 108, 1707–1712. doi: 10.1161/01.CIR.0000094734.67990.99
Keywords: apelin, apelin receptor, [Pyr1]apelin-13(1–12), ACE2, human heart, pulmonary arterial hypertension, forearm plethysmography, biased signaling
Citation: Yang P, Kuc RE, Brame AL, Dyson A, Singer M, Glen RC, Cheriyan J, Wilkinson IB, Davenport AP and Maguire JJ (2017) [Pyr1]Apelin-13(1–12) Is a Biologically Active ACE2 Metabolite of the Endogenous Cardiovascular Peptide [Pyr1]Apelin-13. Front. Neurosci. 11:92. doi: 10.3389/fnins.2017.00092
Received: 02 December 2016; Accepted: 10 February 2017;
Published: 28 February 2017.
Edited by:
Hubert Vaudry, University of Rouen, FranceReviewed by:
Kazuhiro Takahashi, Tohoku University, JapanCopyright © 2017 Yang, Kuc, Brame, Dyson, Singer, Glen, Cheriyan, Wilkinson, Davenport and Maguire. This is an open-access article distributed under the terms of the Creative Commons Attribution License (CC BY). The use, distribution or reproduction in other forums is permitted, provided the original author(s) or licensor are credited and that the original publication in this journal is cited, in accordance with accepted academic practice. No use, distribution or reproduction is permitted which does not comply with these terms.
*Correspondence: Janet J. Maguire, amptMTAwM0BtZWRzY2hsLmNhbS5hYy51aw==
Disclaimer: All claims expressed in this article are solely those of the authors and do not necessarily represent those of their affiliated organizations, or those of the publisher, the editors and the reviewers. Any product that may be evaluated in this article or claim that may be made by its manufacturer is not guaranteed or endorsed by the publisher.
Research integrity at Frontiers
Learn more about the work of our research integrity team to safeguard the quality of each article we publish.