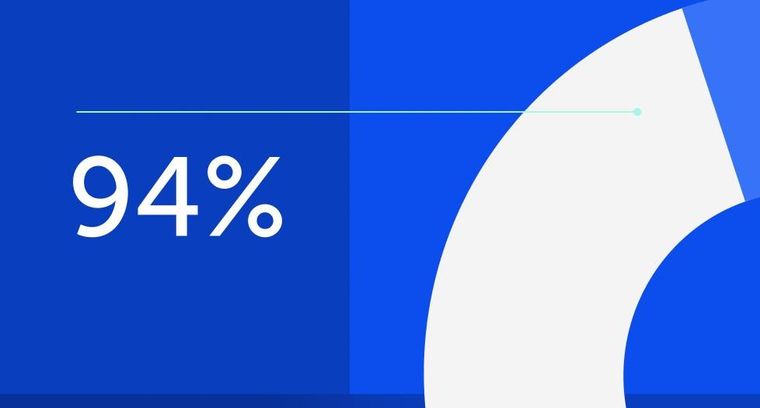
94% of researchers rate our articles as excellent or good
Learn more about the work of our research integrity team to safeguard the quality of each article we publish.
Find out more
REVIEW article
Front. Neurosci., 17 February 2017
Sec. Systems Biology Archive
Volume 11 - 2017 | https://doi.org/10.3389/fnins.2017.00067
This article is part of the Research TopicLimbic-brainstem roles in perception, cognition, emotion and behaviorView all 20 articles
Primates are distinguished from other mammals by their heavy reliance on the visual sense, which occurred as a result of natural selection continually favoring those individuals whose visual systems were more responsive to challenges in the natural world. Here we describe two independent but also interrelated visual systems, one cortical and the other subcortical, both of which have been modified and expanded in primates for different functions. Available evidence suggests that while the cortical visual system mainly functions to give primates the ability to assess and adjust to fluid social and ecological environments, the subcortical visual system appears to function as a rapid detector and first responder when time is of the essence, i.e., when survival requires very quick action. We focus here on the subcortical visual system with a review of behavioral and neurophysiological evidence that demonstrates its sensitivity to particular, often emotionally charged, ecological and social stimuli, i.e., snakes and fearful and aggressive facial expressions in conspecifics. We also review the literature on subcortical involvement during another, less emotional, situation that requires rapid detection and response—visually guided reaching and grasping during locomotion—to further emphasize our argument that the subcortical visual system evolved as a rapid detector/first responder, a function that remains in place today. Finally, we argue that investigating deficits in this subcortical system may provide greater understanding of Parkinson's disease and Autism Spectrum disorders (ASD).
Primates are known for their excellent vision, which is often exemplified by statements about their high visual acuity and trichromatic color vision, characteristics shared with no other mammals (Kay and Kirk, 2000; Ross, 2000; Kirk and Kay, 2004; Jacobs, 2008, 2009). High visual acuity is possible because of the presence of a fovea in the retina (Ross, 2000; Kirk and Kay, 2004), and trichromatic color vision, partly because of the presence of genes that produce different kinds of opsin proteins in the retina (Surridge et al., 2003; Jacobs, 2008, 2009), but importantly, not all primates have a fovea or trichromatic color vision (Stone and Johnston, 1981; Ross, 2000; Kirk and Kay, 2004; Jacobs, 2009). What is really special about primate vision is what goes on in the brain after the retina. Neurons from the retina project to two major brain structures, the lateral geniculate nucleus (LGN) and the superior colliculus (SC) (Kaas and Huerta, 1988). From the LGN, signals are sent to the primary visual cortex (V1) and then to other visual areas in the brain (Kaas and Huerta, 1988; Henry and Vidyasagar, 1991; Kaas, 2004). This pathway may be thought of as part of the cortical visual system. From the SC, signals are sent to the pulvinar (PUL), another subcortical nucleus, and thus this pathway may be thought of as part of the subcortical visual system (Kaas and Huerta, 1988). Some signals from the SC are also sent to distinct layers of the LGN (Casagrande, 1994; Preuss, 2007), and in this way the two visual systems, while able to function independently, are also interconnected to some extent fairly early on in visual processing.
The cortical visual system is indeed expansive in primates, especially in anthropoid primates (monkeys and apes) (Barton, 1998; Kaas, 2013). The cortical visual system has been extensively studied and we will not review it here other than to point out that its functions appear to be different from those of the subcortical visual system. Among other functions, the cortical visual system assists the fovea in providing high visual acuity, and integrates form, color, and movement, for example (Hubel and Livingstone, 1987; Kaas and Huerta, 1988; Tanaka et al., 1991; Kobatake and Tanaka, 1994), to help individuals identify objects and to evaluate potential responses to stimuli in their environments.
Given the low proportion of retinal ganglion cells that project to the SC (around 10% in monkeys; Perry and Cowey, 1984), the subcortical system has traditionally been regarded as residual (e.g., Henry and Vidyasagar, 1991). Evidence that has been building slowly over the years is revealing otherwise, however. A subcortical pathway for object recognition is certainly not unique to primates: correlates to the SC and the PUL in non-primate and non-mammal species generally comprise the tectal-thalamic system, which is involved in predator-prey recognition (Ewert, 1970; Sewards and Sewards, 2002). Nevertheless, the great expansion of visual cortical areas and geniculate layers in primates has generally reduced interest in investigating subcortical structures for processing complex visual stimuli. Here we review behavioral and neurophysiological evidence which suggests that the subcortical visual system evolved as a rapid detector of, and first responder to, stimuli that, for individuals relying on the slower cortical visual system, would have dire consequences. We concentrate on snakes and emotional faces of conspecifics as particularly important and well-studied stimuli.
Snakes have been deadly to primates since primates originated, and, indeed, they are argued to have been so important in the evolutionary history of primates that they were largely responsible for the origin of primates via selection on individuals to visually detect snakes before the strike (Isbell, 2006, 2009). One of the hallmarks of being a primate is an expanded visual sense (Cartmill, 1974, 1992), but snakes can also be extremely difficult to see even with excellent vision, and any advantage that helps in their detection should still be favored today. Since constricting snakes have been predators of primates from the beginning of the primate lineage, and venomous snakes are deadly even today for the largest primates if not seen in time, it is also understandable that primates would fear them. Thus, the ability to detect snakes and the fear of them might be linked. Some studies have measured cortisol, a hormone associated with stress and fear, in primates exposed to snakes and have reported elevated levels (Wiener and Levine, 1992; Levine et al., 1993). It is also important to note, however, that fear of snakes in primates may not be inextricably tied to initial detection of and first response to snakes, even though non-human primates typically react strongly to snakes, including visual detection and focused attention, and sometimes mobbing (Seyfarth et al., 1980; Gursky, 2005; Isbell and Etting, 2017) and ophidiophobia is the most common phobia among humans (Agras et al., 1969; APA, 2013). In fact, the relationship between snakes and primates is more nuanced than the snake predator-primate prey relationship suggests. Primates themselves have also long been predators, and competitors, of snakes (Headland and Greene, 2011).
The selective pressure to “read” expressions on faces likely occurred sometime after the initial pressure from snakes. Early primates are thought to have lived as solitary foragers as many small non-primate mammals do today (Gebo, 2004), and thus would have been less social than most of today's primates. Today most primates live in social groups, have flexible facial expressions, and interact frequently with conspecifics over many years (Burrows, 2008; Dobson, 2009; Dobson and Sherwood, 2011). The ability of individuals to detect and respond quickly to a conspecific that intends to do harm, or that sees a dangerous snake or other predator, should be highly advantageous to survival. Detection of and response to angry or fearful conspecific faces may be accompanied by high emotionality even moreso than with the complicated relationship between primates and snakes.
A third aspect in the lives of primates that has not been associated with fear or the subcortical visual system but that nevertheless requires quick detection and response involves visually guided reaching and grasping during locomotion. Primates evolved as arboreal creatures and they are still largely arboreal today (Cartmill, 1974). The locomotor repertoire of many primate species includes frequent, rapid leaps across gaps. In making such leaps, individuals must be able to visually locate quickly, and manually reach for and grasp, particular branches from many meters up in the complicated structure of the forest canopy. Selection against individuals that were not proficient at making such leaps would have been intense. In this review, we provide available evidence, including that from studies of blindsight, that suggests a connection between the subcortical visual system and visually guided reaching and grasping. Thus, the evidence we present argues for the overriding function of the subcortical visual system being that of rapid visual detection and response in life-or-death situations which require such actions, with facilitation of the fear response under certain conditions. We then conclude this review by examining the potential for linkage between deficits in this subcortical system and certain deficits in Parkinson's disease and ASD, two neurological diseases that are not yet fully understood.
The superior colliculus (SC) is a laminated structure positioned at the tectum of the mesencephalon in the primate brain. Based on its anatomy and functional properties, it is commonly divided into superficial and deep layers of neurons (see May, 2006, for detailed discussion) (Figure 1). The superficial layers of the superior colliculus (sSC) receive direct input from the retina (Leventhal et al., 1981; Perry and Cowey, 1984; Rodieck and Watanabe, 1993). Neurons in sSC have retinotopically organized receptive fields (Lund, 1972; Sparks, 1986). Visual information from sSC reaches both the PUL and LGN in the thalamus (Huerta and Harting, 1983; Stepniewska et al., 2000). Both the PUL and LGN, in turn, maintain reciprocal connections with a number of cortical areas such as V1, V2, and MT (Benevento and Fallon, 1975; Linke et al., 1999; Grieve et al., 2000; Kaas and Lyon, 2007; Schmidt et al., 2010).
Figure 1. Abridged summary diagram of the subcortical (SC-PUL) pathway. sSC, superficial layers of the superior colliculus; dSC, deep layers of the superior colliculus; dPUL, dorsal part of the pulvinar; mPUL, medial pulvinar; lPUL, lateral pulvinar; iPUL, inferior pulvinar; IC, inferior colliculus; SN, substantia nigra; AMY, amygdala. Red dashed line: observed in non-primates (tree-shrew). Blue lines: pulvinar-cortical and cortical-pulvinar connections; gold lines: cortico-collicular connections.
The deep layers (dSC) are further subdivided into intermediate and deep zones and both present a more multimodal response profile, in which neurons respond not only to visual, but also acoustic and somatosensory stimulation (Jay and Sparks, 1987; Groh and Sparks, 1996). Neurons in the dSC are also involved in premotor circuits of eye and head movements (Sparks and Mays, 1981; Lee et al., 1997), although eye movements are not required for the dSC's role in attention (Ignashchenkova et al., 2004). Extensive electrophysiological studies with primates, including single-unit recording and electrical stimulation, show that activity in some dSC neurons induces saccadic shifts and head movements (Cowie and Robinson, 1994; Freedman and Sparks, 1997; Ignashchenkova et al., 2004). In this sense, the dSC receives direct cortical input from the frontal eye field (FEF) and supplemental eye field (SEF) areas and lateral intraparietal cortex (LIP). The dSC also projects back to these cortical areas through thalamic relays (Harting et al., 1980). Interestingly, LIP target neurons receive input from the sSC through pulvinar relays and, in turn, project back to dSC layers (Clower et al., 2001). The dSC is also the target of parietal and prefrontal cortical areas involved in the control of purposeful arm/hand movements (Borra et al., 2014), indicating its role in eye-hand coordination (Lünenburger et al., 2001). Auditory information is mapped within the dSC layers where inferior colliculus projections converge with visual representation (Huerta and Harting, 1984; Jay and Sparks, 1987). There are also inhibitory interlaminar connections between the cells in the sSC and dSC with similar receptive fields (Moschovakis et al., 1988), possibly integrating visual and auditory fields.
Activation of the dSC also results in motor responses, particularly defensive behaviors. In rodents, stimulation of the dSC elicits a range of motor responses, including defensive behaviors such as cowering and freezing (Ellard and Goodale, 1988; Brandão et al., 2003). Recently, it has been shown that activation of dSC neurons in macaques by GABAergic antagonism induces similar responses of cowering and escape behavior (DesJardin et al., 2013). These behaviors are likely to rely on connections between the SC and substantia nigra. The nigrotectal pathway has been described (Beckstead and Frankfurter, 1982; Huerta et al., 1991) as well as collicular input to the substantia nigra in a few primate species (May, 2006).
A main function of the PUL is to assist in visual processing by shifting attention to relevant stimuli and tuning out irrelevant visual information (Ungerleider and Christensen, 1979; LaBerge and Buchsbaum, 1990; Chalupa, 1991; Robinson and Petersen, 1992; Robinson, 1993; Morris et al., 1997; Grieve et al., 2000; Bender and Youakim, 2001). It is the largest nucleus in the thalamus of primates and is especially large in anthropoid primates (Walker, 1938; Jones, 1985; Chalupa, 1991; Stepniewska, 2004; but see Chalfin et al., 2007). It can be divided into several divisions, including a ventral part (vPUL) comprised of the inferior PUL (iPUL) and ventral portions of the lateral PUL (lPUL) (Stepniewska, 2004; Preuss, 2007; Figure 1). The iPUL and ventral lPUL are visual, receiving inputs from the retina and sSC (Clower et al., 2001) and projecting to many different visual areas including V1, V2, and the superior temporal sulcus (STS; Stepniewska, 2004).
The dorsal part of the PUL (dPUL) is comprised of the dorsal portion of the lPUL and the multisensory medial PUL (mPUL). The dPUL may not exist in non-primates but has greatly expanded in anthropoid primates (Preuss, 2007). Like the vPUL, the dPUL is involved in attention and orientation to salient visual stimuli (Robinson and Petersen, 1992), and its expansion suggests an increased importance of the subcortical processing of relevant stimuli. Importantly, although the dPUL has connections with more cortical areas than the vPUL, it does not have connections to V1 (Trojanowski and Jacobson, 1974; Glendenning et al., 1975; Baleydier and Mauguière, 1985, 1987; Selemon and Goldman-Rakic, 1988; Acuña et al., 1990; Garey et al., 1991; Robinson and Petersen, 1992; Baizer et al., 1993; Ma et al., 1998; Gutierrez et al., 2000; Stepniewska, 2004). Furthermore, the dPUL receives inputs from the dSC (Stepniewska, 2004) and projects to the lateral amygdala (Jones and Burton, 1976; Aggleton and Saunders, 2000).
A possible pathway from the sSC and dSC to the dPUL that excludes VI but goes to the amygdala points to a way for visual input to reach the amygdala without cortical involvement. Another possible pathway might involve sSC neurons reaching the vPUL, and then being relayed to the dPUL and amygdala. This route has been demonstrated in non-primate mammals (Day-Brown et al., 2010). To our knowledge, however, this route is lacking in tracer studies in the primate brain. A direct SC-PUL connection to the amygdala has recently been predicted in humans and macaques by means of probabilistic diffusion tensor imaging tractography (DTI; Tamietto et al., 2012; Rafal et al., 2015). It is important to note that DTI provides an indirect anatomical finding that will require confirmation from neurophysiological tracing studies, which is still lacking in primates. In either case, a subcortical visual route that sends signals to the amygdala has attracted the attention of several neuroscientists in the past 10 years or so, as it has critical implications for the study of affective stimuli.
Several recording studies have generated supporting evidence for a SC-PUL role in affective/salient visual stimuli. As a whole, the SC receives visual input mostly from magnocellular and koniocellular channels, which yields information with low-spatial resolution (Miller et al., 1980). In theory, the subcortical visual pathway would relay fast and low-detailed visual information (“fast and coarse”) for immediate response. Recordings from SC and PUL neurons in behaving macaques indicate these nuclei encode facial and threatening stimuli significantly faster than the visual cortex, as early as 25 ms and 40 ms, respectively (Maior et al., 2011; Nguyen et al., 2013, 2014; see below). This has also been supported by magnetoencephalographic (MEG) studies with a dynamic causal modeling (DCM) in which a fast subcortical visual pathway yielded more explanatory power for short latency responses compared to a cortical model (Garrido et al., 2012; Garvert et al., 2014). This ascending (feedforward) information may be further amplified by interactive activity based on reciprocal connections between the SC-PUL pathway and cortical areas (Shipp, 2003; Pessoa and Adolphs, 2010). The cortico-PUL-cortical circuits are involved in amplifying signals and improving signal-to-noise ratios (Shipp, 2003; Pessoa and Adolphs, 2010), as well as modulating interactions between oscillatory processes in different cortical areas, which contributes to visual attention (Serences and Yantis, 2006; Saalmann and Kastner, 2009).
Taken together, anatomical, behavioral, and recording findings in primates are consistent with the current (but as we argue here, limited) view that the SC-PUL pathway functions to direct visual attention to salient emotional stimuli. Below we examine evidence for two kinds of stimuli in particular that have almost certainly had profound effects on primate survival over evolutionary time: snakes and emotionally expressive faces.
Fear plays a critical role in helping organisms deal with potentially dangerous encounters by being associated with rapid and effective defensive responses (immobility, flight, fight, e.g., Blanchard and Blanchard, 1983). Öhman and Mineka (2001, 2003) proposed that defense systems imposed by vulnerability to snakes over evolutionary time (Isbell, 2006, 2009) shaped the appearance of a “fear module” in their prey—an independent behavioral, psychophysiological, and neural system that is relatively encapsulated from more advanced human cognition. Isbell (2006, 2009) has also emphasized the evolutionary importance of snakes by arguing that natural selection has shaped primates to quickly detect snakes and respond appropriately to them, including responding with fearful behavior. According to the Snake Detection Theory (SDT), the pressure posed by snakes over evolutionary time favored the origin of primates by selecting for visual systems that are highly sensitive to snakes (Isbell, 2006, 2009).
Inspired by evolutionary considerations, long-term research programs from several laboratories and spanning several decades have generated a large body of evidence showing that stimuli involving some level of evolutionarily derived threat, such as potentially dangerous animals, engage different neurobehavioral systems from those evoked by more mundane and innocuous stimuli, thus with preferential access to the fear module (see Öhman and Mineka, 2001). As the result of ancient evolutionary co-existence between snakes and primates, fear of snakes is still highly prevalent in both humans (e.g., Agras et al., 1969; Fredrikson et al., 1996; Lang et al., 1997) and monkeys (Mineka et al., 1980). Furthermore, when snakes are paired with aversive events, fear conditioning is more rapid and stable than to neutral stimuli, again both in humans (e.g., Öhman et al., 1978) and other primates (e.g., Cook and Mineka, 1990), and independently of whether it involves direct or vicarious conditioning (i.e., observing other monkeys displaying fear of snakes).
These fear-relevant stimuli also serve as effective fear stimuli even when masked from conscious recognition (Öhman and Soares, 1993, 1994; Carlsson et al., 2004) and shown under perceptually degraded conditions (Kawai and He, 2016), and are more rapidly detected—i.e., have attentional priority—when presented among distractor stimuli (e.g., flowers, mushrooms) in visual search tasks (e.g., Öhman et al., 2001). This preferential processing has been consistently shown with adult humans (e.g., Öhman et al., 2001) and small children (LoBue and DeLoache, 2008; LoBue et al., 2010; Masataka et al., 2010; Hayakawa et al., 2011; Penkunas and Coss, 2013a,b; Yorzinski et al., 2014), as well as with lab-reared, snake-naïve macaques (Shibasaki and Kawai, 2009). The invariant snake-scale patterns are also highly salient visual cues, as shown by several field studies (e.g., Ramakrishnan et al., 2005; Meno et al., 2013; Isbell and Etting, 2017).
Despite multiple demonstrations that the fear module is selectively sensitive and automatically activated by snakes (see Öhman and Mineka, 2003), the results from most of these studies preclude a direct test of the role of evolution in emotion, since no equivalent animal fear stimuli with distinctive evolutionary histories with primates have been used as a comparison stimulus. For humans, spiders may represent an ideal candidate since they involve matched fear levels to those of snakes—reflected in valence, arousal, and dominance ratings (Lang et al., 2005), and are both highly frequent objects of phobias (e.g., Agras et al., 1969; APA, 2013). However, since non-human primates do not react fearfully to spiders but sometimes perceive them as food items, fear of spiders is undoubtedly younger evolutionarily than fear of snakes. This makes them the ideal comparison stimuli for testing the implications of the SDT (e.g., Steen et al., 2004; Isbell, 2009). Although some studies have indeed included spiders as an evolutionary fear-relevant stimulus, unfortunately, the authors combined them into the same category with snakes, thus impeding the study of any potential dissociations between the two (e.g., Öhman et al., 2001).
When spiders and snakes are separated as experimental stimuli, as a growing body of research has demonstrated, humans show preferential detection of snakes compared to spiders. Soares and her colleagues (e.g., Soares et al., 2014) performed a series of behavioral experiments in humans to test predictions of one of the hypotheses of the SDT, i.e., that the vital need to detect dangerous snakes under challenging visual conditions provided a strong source of selection for the evolution of visual solutions to this threat. The results were supportive, and showed that humans preferentially detected snakes (compared to spiders and mushrooms) under taxing visual conditions, namely when the stimuli were presented more rapidly (Soares and Esteves, 2013; Soares et al., 2014), in the visual periphery (Soares et al., 2014), in a cluttered environment (Soares et al., 2009, 2014; Soares, 2012; Soares and Esteves, 2013), and when attention had to be automatically redirected to suddenly appearing snakes in the immediate environment (Soares, 2012; Soares and Esteves, 2013; Soares et al., 2014). Additionally, a study using an interocular suppression technique—the Continuous Flash Suppression (CFS; Tsuchiya and Koch, 2005), known to suppress stimuli from awareness, showed that snakes overcame suppression and accessed awareness faster than spiders (and compared to birds), again in the most visually demanding conditions—when the stimuli were presented to the participant's non-dominant eye (Gomes et al., 2017).
The dissociations between snake and spider processing were recently extended to non-human primates in a visual search study with snake-naïve Japanese macaques, showing that snakes were detected significantly faster than non-threatening animals (koalas), whereas the detection of spiders did not differ from the innocuous stimuli (Kawai and Koda, 2016). Importantly, and in order to study the attentional time course of the privileged processing of snake stimuli, further recent studies have used event-related potentials (ERPs) and complemented these previous findings by showing that snakes depict earlier visual attention in passive viewing tasks compared to spiders (and innocuous animal stimuli), as reflected in larger early posterior negativity (EPN) amplitudes (He et al., 2014; Van Strien et al., 2014a,b, 2016), with the curvilinear shapes of snakes only partially explaining this enhancement (Van Strien et al., 2016). Finally, a study by Grassini et al. (2016) showed that enhanced EPN amplitudes to snakes were only observed when the stimuli were presented under aware conditions. Although this result contradicts previous findings (see Gomes et al., 2017), the authors relied on different methodologies to manipulate awareness. While Grassini et al. (2016) relied on masking procedures, Gomes et al. (2017) used breaking CFS (b-CFS), which seems to enable suppression from visual awareness for longer periods of time (Lin and He, 2009).
Together, this consistent bulk of data showing a preferential specificity for snake processing invites an evolutionary explanation, such as the one offered by the SDT, while also suggesting that spider fear may be confined to humans and generated more through mechanisms of learning (see Soares et al., 2009).
Based on extensive studies, the SC-PUL pathway was proposed as the “low road” of affective visual stimuli to the amygdala (LeDoux, 1996). Fearful (threatening) images passing through both structures would elicit fast amygdalar activation, which, in turn, would trigger autonomic and behavioral responses. A large number of experiments using threatening social stimuli (e.g., fearful or aggressive facial expressions) on human and non-human primates has corroborated this framework (see below). In contrast, evidence for preferential activity of the SC-PUL visual pathway toward snake stimuli is more limited and comes from a handful of recent studies employing lesion, imaging, and electrophysiological recordings. Although they were not specifically designed to test the predictions of SDT, their results largely support a phylogenetic predisposition for fast snake detection.
Regarding the SC, bilateral neurotoxic lesions in infant capuchin monkeys impaired the emotional processing of snakes as threatening stimuli (Maior et al., 2011). Lesioned monkeys in that experiment were uninhibited by the presence of a rubber snake in a threat-reward task, whereas control monkeys refrained from approaching the food reward next to it, even after 12 h of food deprivation. Although this result is suggestive of SC importance in processing visual threat stimuli, it does not, by itself, hint of any preferential processing of snakes specifically because snakes were not compared with other stimuli. It is interesting to note, however, that central visual field or foveal representations in the SC seem also to be very sensitive to snake images in humans. In a human fMRI study by Almeida et al. (2015), snake stimuli presented in SC regions representing the fovea elicited increased activity. This central sensitivity indicates that the SC is not just engaged during orientation to peripherally presented stimuli.
Snake-sensitive neurons were also found in the PUL of Japanese macaques, particularly in its medial and dorsolaterally portions (Le et al., 2013). In this case, snake stimuli elicited faster and stronger responses from PUL neurons than other stimuli, including emotional faces of conspecifics. Latencies were found to be a little longer than in SC neurons (~55 ms), a finding which is in line with expected for the second relay in the subcortical pathway model. Furthermore, low-pass filtering (LPF) of snake images did not affect neuronal firing, and high-pass filtering (HPF) decreased it, suggesting that PUL neurons process low spatial frequency (LSF) stimuli. In a subsequent study, Le et al. (2014) showed that the PUL might code not only for the presence of threatening stimuli but also for the degree of threat. In that study, a larger subset of PUL neurons was more sensitive to snake pictures depicting striking postures than non-striking postures in that response magnitudes were significantly higher to snakes in striking postures. Furthermore, PUL neurons display gamma oscillation in response to snake images, suggesting feedforward processing for images of snakes, consistent with rapid detection of snakes (Le et al., 2016).
Since the pulvinar is highly interconnected with both cortical areas and subcortical nuclei (Acuña et al., 1990; Baizer et al., 1993; Ma et al., 1998), its functions regarding snake processing may be proportionally varied and complex. Recent findings in humans from Almeida et al. (2015) mirror the results described above. The SC, PUL, and the amygdala displayed differential activation to true snake stimuli (vs. cables, strings, etc.). Interestingly, there was a very strong pattern of fMRI activation to centrally presented snake pictures in all three structures. This central sensitivity suggests again that the SC-PUL pathway is not only engaged in attention to peripherally presented stimuli but is also involved in explicit processing of snakes.
Taken together, the findings of these studies point to particular features of snake stimuli processing: (1) Short response latencies: single-cell experiments show faster responses to snakes compared to other threatening stimuli, including expressive faces. SC neurons, on average, fired at slightly shorter latencies than PUL neurons, 20–100 ms and 30–120 ms, respectively. It is possible that extremely short-latency PUL neurons receive direct input from the retina, bypassing SC (Nakagawa and Tanaka, 1984). (2) Stronger response magnitudes: PUL neurons showed stronger firing to snakes compared to facial expressions. SC neurons with central and lower visual fields, on the other hand, showed similar response magnitudes to snake and faces; (3) Spatial frequencies: PUL neurons are known to be sensitive to LSF images (Schiller et al., 1979; Vuilleumier et al., 2003). Accordingly, PUL neuronal responses were unaffected by LPF of snake images, but were significantly decreased by high spatial frequency (HPF) (Le et al., 2013). At low levels of spatial frequencies, images depict broad features without fine visual details. (4) Visual field locations: central visual field areas in the SC seem to be very sensitive to snakes. This suggests that preferential processing of snakes includes early spatial detection as well as explicit central processing. (5) Naivety: SC-PUL activity may be independent of previous interactions with snakes, as monkey subjects were often lab-reared and very unlikely to have seen snakes before the experiments. This is particularly striking in the case of behavioral avoidance of a snake model by sham-lesioned monkeys (Maior et al., 2011).
Faces are important means of communicating potential threats to observers. Facial expressions of anger, for instance, signal imminent aggression toward the observer, while faces expressing fear are indicative of potential danger in the environment. Both facial expressions, therefore, signal a possible threat to the individual, albeit with each having particular features in regard to their detection. In this sense, several studies have shown that the threatening nature of these stimuli is maximal when angry faces are coupled with a direct gaze, which is indicative of a threat directed to the observer, and with an averted gaze in fear faces, since it provides the observer with a more precise indication of where the threat is located (e.g., Adams et al., 2003). Because faces expressing anger and fear may jeopardize the protection of the self (Fridlund, 1994), several researchers have proposed that they are part of an evolved response system, together with snakes and perhaps other predatory animals (for a review, see Öhman et al., 2012).
This notion is supported by substantial behavioral data demonstrating that angry and fearful faces are more effectively detected as targets in visual search tasks (e.g., Öhman et al., 2010; Pinkham et al., 2010), are more difficult to ignore, both in humans (e.g., Fox et al., 2001; Georgiou et al., 2005) and in macaques (Landman et al., 2014; Kawai et al., 2016), potentiate perceptual abilities of non-threatening stimuli presented subsequently (e.g., Becker, 2009), enhance perception and attentional capacities (e.g., Phelps et al., 2006; Bocanegra and Zeelenberg, 2012), increase distraction of task-irrelevant items across the visual field and under increased attentional load conditions (e.g., Lavie et al., 2003; Berggren et al., 2013), show faster acquisition and more resistance to extinction in conditioning procedures (e.g., Öhman et al., 1985, 1995), and gain preferential access to awareness (e.g., Yang et al., 2007).
The pattern of behavioral results arguing in favor of a more efficient ability to detect threatening social stimuli is, however, less clear than that observed for snake stimuli. Although considerable data on the effects of angry faces on visual attention argue in favor of an evolutionarily tuned threat detectability, the literature is mixed, with about half the articles in favor of an evolutionarily tuned threat detection system (a so-called anger superiority effect) and the other half showing more efficient detection of happy faces in attracting attention (for an overview, see Lundqvist et al., 2014; see also Becker et al., 2011). The latter evidence, i.e., that happy faces (compared to threatening faces) are more rapidly detected in visual search tasks, seems to be particularly evident when the stimuli depict photographs of real faces and not schematic ones (Becker et al., 2011; Lundqvist et al., 2014), with the most parsimonious account for these results relying on visual conspicuity and not on the emotional nature of the stimuli (e.g., Calvo and Marrero, 2009). Importantly, a recent meta-analysis of several studies exploring the influence of emotional facial stimuli in visual attention suggested that emotional arousal can explain the mixed findings (Lundqvist et al., 2014). Indeed, most of the previous research in this domain assumed the relationship between emotion and attention from a valence and not from an arousal perspective. The findings from Lundqvist and colleagues make evolutionary sense as arousal reflects the degree of energy and mobilization for eventual fight or flight responses (e.g., Lang and Bradley, 2010), which might then be under the purview of the SC-PUL pathway.
Another study with the goal of resolving conflicting findings in the emotion-attention domain advanced gender as an additional factor modulating the anger superiority effect (Öhman et al., 2010). More specifically, angry male faces are more rapidly and accurately detected in a visual search setting than angry female faces, consistent with the view that males are more associated with hostility, and females, with friendliness.
A similar pattern emerges in emotion recognition tasks, where a pervasive happy face advantage (faster reaction times and higher accuracy) is observed across diverse manipulations (for a meta-analysis, see Nummenmaa and Calvo, 2015). However, these studies have mainly relied on prototypical full-intensity expressions. It might well be expected that there is enhanced recognition accuracy for angry faces at lower intensities since the survival premium of efficient recognition of a threatening face when the emotional intensity is subtle may promote adaptive behavioral responses in situations where potentially aggressive encounters are imminent (for a review, see Öhman et al., 2012).
Conditioning to threat faces also seems to be less robust than that observed for snakes. For instance, in one study verbal instructions eliminated the fear-conditioned responses to angry faces (Rowles et al., 2012), while a different study demonstrated that aversively conditioned angry faces only captured attention under low load conditions (Yates et al., 2010). These findings suggest that faces are probably more context-specific than are snakes.
Finally, a recent study showed that although fearful faces gained preferential access to awareness (using CFS), compared to neutral faces, this advantage relied on HPF information (Stein et al., 2014), thus suggesting involvement of cortical visual processing (e.g., Schiller et al., 1979). These results are inconsistent with the role of the retino-collicular-pulvinar-amygdala pathway (see LeDoux, 1996) in this privileged access to awareness. However, Stein and colleagues also open the possibility that biologically relevant stimuli, such as snakes, show an advantage in accessing awareness based on LSF information, which argues in favor of an SC-PUL pathway to the amygdala. This would highlight that social and predatory fear stimuli may have distinctive neuronal signatures, given their different biological relevance (see Öhman et al., 2012). This reasoning conforms to the relative evolutionary importance of snakes and angry or fearful faces, with the former being important from the beginning of the primate lineage when predatory snakes were present but primates are thought to have been limited in their social interactions (see Isbell, 2006, 2009), and the latter emerging later, as primates became more social, with greater fluidity in social interactions requiring more cortical processing to assess and respond to social cues. However, as will be shown in the next section, the relative importance of SC-PUL and cortical vision in assessing emotion from faces may also be dependent on ontogeny.
Several lines of investigation support the claim that faces are a special class of stimuli in the primate visual system (Grüsser and Landis, 1991; Carey, 1992). The human cortex includes dedicated areas for facial stimuli processing, most notably the fusiform face area (FFA) in the lateral fusiform gyrus (Kanwisher et al., 1997). Together with the FFA, the inferior occipital gyrus, posterior superior temporal sulcus, and the anterior infero-temporal cortex have shown differential activation for faces compared to other objects (Rossion et al., 2012). There is, however, mounting evidence that facial information is also processed in a parallel subcortical circuit involving the SC-PUL.
One important line of evidence refers to the preference for faces displayed by human babies (Johnson et al., 1991). Neonates tend to orient their gaze to face-like stimuli immediately after birth (Goren et al., 1975). At this point in development, cortical structures are not fully mature and show only limited activation (Johnson, 2011; Cohen Kadosh et al., 2013). Control of visually guided tasks in newborns is very likely exerted by visually related subcortical structures (Csibra et al., 1998, 2000). Based on these findings, the two-process theory of face processing posits that an innate disposition to faces is supported by subcortical structures in newborns while cortical regions gradually specialize in facial detection and recognition throughout development (Johnson and Morton, 1991; Johnson et al., 2015).
Newborn preference for upright faces is a potentially thorny issue in this field. Such an effect is present immediately after birth but disappears after 2 months, only to reemerge at around 6 months of age (Mondloch et al., 1999; Nakano and Nakatani, 2014). This U-shaped preference for faces called into question the reliability of earlier findings drawn from newborn studies. Nevertheless, a recent study employing S-cone sensitive stimuli (Nakano et al., 2013) has shed light on this controversy as well as underscored the involvement of the SC in facial detection. Since the SC is “blind” to S-cone stimuli, Nakano and colleagues were able to show that 2-month-old babies have a preference for S-cone upright faces. This indicates that the apparent disappearance of this preference for upright facial stimuli may be the result of changes in the hierarchical organization of visual areas in the brain.
S-cone stimuli have also been used to demonstrate the contribution of subcortical structures to rapid detection of faces. In general, faces induce shorter reaction times compared to non-facial neutral stimuli in neuropsychological studies (Crouzet and Thorpe, 2011). Emotional expressions conveyed by faces seem to induce even shorter reaction times as in the case of fearful faces vs. neutral faces. These effects disappear when facial stimuli in S-cone isolating frequencies are used (Nakano et al., 2013), indicating that the facilitatory effect for fast detection relies on collicular activity. Although S-cone isolating results should be viewed with caution (see Hall and Colby, 2013), this finding is further corroborated by Garvert et al. (2014). Using the MEG approach, they compared the dynamic causal models for different latencies of face processing. The authors found that, at least for short latencies, data from evoked fields pointed to a direct subcortical connection to the amygdala for facial stimuli with varying degrees of emotional expressions.
The influence of the SC-PUL pathway in facial detection is also demonstrated by the neuro-ophthalmological syndrome known as “blindsight” (Sanders et al., 1974; Stoerig and Cowey, 1997). In broad terms it refers to the ability to unconsciously detect and discriminate visual stimuli after destruction of striate cortex (“cortical blindness”). Patients with this syndrome are able to accurately guess motion, position, and some aspects of images presented in their blind visual fields (Weiskrantz, 1996). This effect extends to faces (Solca et al., 2015) and emotionally salient stimuli (more narrowly termed as “affective blindsight”; Celeghin et al., 2015). Neuroscientists have taken advantage of this phenomenon to investigate the underlying processes not normally noticed during conscious experience. One patient (G.Y.) with cortical blindness in the right half-field has been shown to recognize different emotional faces (de Gelder et al., 1999). In a later study with the same patient, fearful faces induced differential amygdalar responses that correlated with activity in posterior thalamus and SC (Morris et al., 2001). Moreover, the presentation of fearful faces to the blind hemifield of hemianopic patients enhanced responses to facial stimuli presented to the cortically-intact hemifield (Anders et al., 2009; Cecere et al., 2014). These findings underscore the importance of SC-PUL in processing affective facial stimuli. Interestingly, one recent study raised the possibility that many visual deficits in patients with Parkinson's disease may be due to the inhibition of the SC and dysfunctional PUL activity (see also Isbell, 2009; Diederich et al., 2014).
There are also several techniques to elicit unconscious responses to faces by subliminal presentation of pictures, such as backward/forward masking and continuous flash suppression (see Axelrod et al., 2015, for review). Combined with fMRI techniques, they have provided further evidence of SC-PUL participation. Subliminal fearful faces in fMRI were correlated with activation of a direct subcortical pathway in a feedforward connection (Williams et al., 2006). Troiani and Schultz (2013) found activation in the SC, amygdala, thalamus (PUL), and hippocampus for suppressed objects (including emotional expressions of fear). Interestingly, suppressed faces failed to elicit activation in the FFA, indicating that SC-PUL processing of such stimuli may occur independently, at least in the short term, without cortical input.
Since neurons in the primate SC-PUL pathway are tuned for broad, LSF information, investigators have used low-frequency and high-frequency filtered pictures to selective activate visual channels. Vuilleumier et al. (2003) used low-frequency filtered fearful faces to induce activation of the SC and PUL. This activation was correlated with a stronger amygdalar response compared to high-filtered faces. Low-pass filtered facial (neutral) stimuli subliminally presented produced congruence when guessing the gender of the faces (Khalid et al., 2013). This is indicative that some aspects of faces may be distinctively coded in the SC and PUL. Indeed, in an elegant protocol based on the monocular segregation of visual inputs, Gabay et al. (2014) provided evidence that the subcortical visual pathway conveys representation of identity in facial stimuli.
Studies with macaques have largely supported the findings from human studies while yielding a more detailed profile of SC and PUL neuronal behavior. Sensitivity to face-like patterns was detected in individual SC neurons in macaque monkeys as early as 25 ms (Nguyen et al., 2014). Although actual faces did not elicit differential responses compared to face-like patterns, these neurons also showed differential responses to different gaze direction. Face-like patterns also elicited responses from lPUL and mPUL neurons within 50 ms and between 50 and 100 ms (Nguyen et al., 2013). The activation in the first 50-ms interval was restricted to a few aspects of the stimuli and it is consistent with the activity of SC neurons toward the same kind of stimuli (Nguyen et al., 2014). The activity in the 50-100-ms interval and later, in contrast, was observed in a greater number of neurons and those encoded more information from the stimuli. This later activation of PUL neurons may involve inputs from descending cortical neurons and it is in keeping with an analysis of gamma oscillations in the PUL of macaques (Le et al., 2016). Gamma oscillations are thought to occur simultaneously in areas processing visually attended stimuli. In this particular case, the pulvinar would be involved in cortico-cortical integration for face stimuli processing. Recording of single-unit neurons in the PUL of macaques showed that these neurons respond to different emotional expressions of human faces (Maior et al., 2010). The latency of response in this case varied from ~40 ms to over 300 ms, which is consistent with both a first, fast and coarse feed-forward response, and a later cortical integration.
Altogether, the available data are consistent with the “fast and coarse” scenario for the SC-PUL pathway. Short latency response from SC neurons seems to give an early indication of facial patterns, including orientation, gender, and identity information. PUL neurons seem to be sensitive to the same aspects but they also participate in early cortical processing of facial expressions. The possible targets for SC-PUL facial-related information include several cortical areas and subcortical nuclei but the pathway is likely to provide information for fast amygdalar facial responses at around 100 ms (Tazumi et al., 2010).
As the previous sections have described, growing evidence suggests that the SC-PUL pathway is a fully functioning system for rapid, non-conscious detection of evolutionarily salient stimuli that often necessitate a rapid response for the responder's continued survival, particularly when dealing with emotionally charged stimuli, i.e., snakes and emotional faces. Some of this knowledge has arisen from investigation of perturbations of the neocortex. Thus, as described above, blindsight, in which the primary visual cortex is non-functioning, reveals the sensitivity of the SC-PUL system in detecting emotional faces (Morris et al., 2001; Vuilleumier et al., 2003).
Growing evidence suggests, however, that we have not yet tapped into all the evolutionarily relevant functions of the SC-PUL system. If the SC-PUL system is well suited as a fast detector/first responder in primates, there is at least one other evolutionarily salient condition that requires a rapid, non-conscious response, and it is observable via studies of blindsight. In addition to affective blindsight, which strongly implicates the SC-PUL's involvement in non-conscious detection of certain types of emotional faces, there is action blindsight, in which the ability to make saccades to targets, and reach for and grasp or, for humans, to also point to targets very quickly, remains (Weiskrantz et al., 1974, 1995; Barbur et al., 1980, 1999; Blythe et al., 1987; Stoerig et al., 1997; Danckert and Rossetti, 2005; Carey et al., 2008). Although the SC-PUL system has been implicated in action blindsight (Danckert and Rossetti, 2005), no evolutionary explanation has yet been offered. Why would it be so important to be able to non-consciously and rapidly reach for and grasp, or point to, objects?
Except for felids and primates, mammals are not known to have visually guided reaching and grasping. Conventionally, this ability has been attributed to visual predation as the earliest primates are thought to have been insectivorous (Cartmill, 1974). However, there is some evidence that visually guided reaching and grasping is not actually universal among primates. Macaques and humans are indeed capable of visually correcting errors in reaching and grasping (Pessiglione et al., 2003; Schettino et al., 2003, 2006; Danckert and Rossetti, 2005). However, despite being highly insectivorous, galagos apparently cannot adjust their arms to grasp a moving target once they initiate the movement (Bishop, 1964).
From a neural point of view, the inability of galagos to use vision to adjust their online reach may be related to their more limited connections between visual areas and regions of the posterior parietal cortex (PPC) that are involved in reaching and grasping (Stepniewska et al., 2005). From an evolutionary point of view, the apparent inability of galagos to adjust their reach with visual feedback may be related to their mode of locomotion. Small non-primate mammals generally move in arboreal habitats by scurrying along the tops of branches, minimizing large leaps across gaps, and using claws to help them grip when necessary. The last common ancestor of all primates is also thought to have been small and arboreal but with nails instead of claws (Gebo, 2004), which would have required a prehensile grip on small branches. Primates now range widely in body size, and have different modes of locomotion with different ways of crossing arboreal gaps: vertical clingers and leapers, such as galagos, leap with their hindlimbs landing first; quadrupedal, above-branch walkers leap with their forelimbs landing first, and; suspensory, below-branch graspers or brachiators use their forelimbs to swing from branch to branch.
We are concerned here with the latter two types, both of which involve forelimb-dominated locomotion. When quadrupedal, above-branch walkers and suspensory, below-branch graspers cross arboreal gaps, they must quickly and accurately with their forelimbs reach for and grasp branches that often differ in orientation and circumference. There would have been strong selection favoring visually guided forelimb reaching and grasping in the ancestors of such primates since missing a target branch just once can be fatal.
De Winter and Oxnard (2001) have shown that locomotor style has influenced the coordinated evolution of certain brain components in primates. Compared with bats and insectivores, primates have expanded several regions of the brain that are involved in voluntary motor control. Furthermore, locomotor styles and correlated expansion of these regions within primates cluster together regardless of phylogenetic relatedness, with scurriers and hind limb-dominated vertical clingers and leapers separated from above-branch leapers, and all separated from suspensory graspers. Humans are outliers among primates, having expanded those same regions of the brain the most (de Winter and Oxnard, 2001). Although, as bipedal walkers and runners, humans no longer need their forelimbs in locomotion, they have developed extensive manual tool manufacture and use, and humans are thought to be the only species that engages in declarative pointing, i.e., the motor behavior of pointing to an object as a way to direct another's attention to it for the purpose of sharing interest in it (Povinelli and Davis, 1994; Butterworth et al., 2002; Tomasello et al., 2007; but see Leavens et al., 2005). Thus, more generally, directed forelimb action may have fueled brain expansion in primates in ways that are different from other mammals.
Here we pull together several indirect lines of evidence to suggest that, in concert with higher cortical systems involved with forelimb-dominated locomotion or forelimb directed action, the SC-PUL system supports rapid, non-conscious, visually guided reaching and grasping, and pointing (see also Isbell, 2009). In the primate neocortex, reaching and grasping, and for humans, pointing, in addition, are heavily represented in the PPC, which is part of the dorsal “vision for action” stream (Previc, 1990; Goodale and Milner, 1992; Goodale and Westwood, 2004). The SC and PUL both contribute to the dorsal stream: the SC sends projections indirectly to the PPC through the PUL (Lyon et al., 2010), and the PUL sends projections directly to the PPC (Selemon and Goldman-Rakic, 1988; Schmahmann and Pandya, 1990).
Stimulation of the SC's deeper layers in vertebrates, including primates, results in bodily movement as well as oculomotor movement (Ewert, 1970; Werner, 1993; Gandhi and Katnani, 2011). Neurons have been found in the SC of macaques and humans that are involved in both oculomotor responses and reaching and grasping (Werner, 1993; Lünenburger et al., 2000; Stuphorn et al., 2000; Nagy et al., 2006), suggesting integration of visual and motor behaviors, which would seem critical in visually guided reaching and grasping. For example, neurons (“gaze-related reach neurons”) fire in the SC when arm movements reach for targets in the direction of the gaze, and arm movements also speed up saccades (“saccade neurons”) to those targets (Lünenburger et al., 2000; Stuphorn et al., 2000; Snyder et al., 2002). As another example, “fixation” neurons in the SC allow primates to visually lock onto a target once it has been located (Krauzlis et al., 2000), and arm movements modulate these fixation neurons (Lünenburger et al., 2001). Finally, neurons in the SC have been found to anchor the gaze of a person to any target to which that person points (Stuphorn et al., 2000; Neggers and Bekkering, 2002).
In macaques, PUL neurons have been found to respond more strongly to visually guided, intentional reaching movements to targets than to passive or exploratory arm movements (Margariños-Ascone et al., 1988; Acuña et al., 1990), whereas neurons in the PPC respond more to passive arm movements (Acuña et al., 1990). In addition, firing rates of PUL neurons are more strongly correlated with rapid arm movements than with force (Margariños-Ascone et al., 1988). A minority of PUL neurons also respond more quickly than neurons in the PPC (Acuña et al., 1990), suggesting that bottom-up processing might occur.
Individual neurons have also been found in the PUL that are responsive to both visual stimuli and movements of the arms and hands, again suggesting integration of visuo-motor abilities (Martín-Rodriguez et al., 1982; Margariños-Ascone et al., 1988). In an fMRI study of humans, the PUL was activated when visual and hand movements occurred together but not when either occurred alone (Ellerman et al., 1998). In macaques, temporary inactivation of the dPUL results in a poorer ability to reach for and grasp objects (Wilke et al., 2010).
Available evidence thus suggests that the SC-PUL system functions more broadly than orienting attention to salient emotional stimuli. It may be more accurate to describe the SC-PUL system as a first detector of and responder to stimuli that require rapid visual detection and motor responses for continued survival. In this regard, the SC-PUL system in non-human primates appears to be tripartite in having heightened sensitivity to (1) snakes as a potential threat, (2) emotionally charged social cues, i.e., emotional faces, and (3) graspable objects in the environment. It has further been suggested that in humans, declarative pointing was built on two of the three functions of the SC-PUL system, having evolved initially from visually guided reaching and grasping and later as a social response that improved avoidance of snakes (Isbell, 2009). Since blindsight studies also demonstrate that the ability to point to “unseen” targets still remains, the evidence thus far suggests that the SC-PUL system participates in the processing of declarative pointing.
By recognizing that the SC-PUL system also plays a role in motor responses for visually guided reaching and grasping, and pointing (in humans), we can expand our current understanding of it and view it as an integrated visual/motor system that is required to detect and respond very quickly, and thus, for maximum benefit, non-consciously.
The combined results of multiple studies corroborate the notion that the SC-PUL pathway forwards “rapid and coarse” visual information about snakes. Indeed, both the SC and PUL fire faster or more strongly for snakes compared to faces (neutral and fearful), snake-like objects, hand, and simple pattern objects. Although facial expressions may signal social and non-social threat, the remaining comparison stimuli are neutral with regard to threat. The SDT, and the involvement of the SC-PUL pathway, is therefore strongly supported from a neurophysiological perspective. Nevertheless, it is worth stressing the limited spectrum of stimuli employed so far. Thus, studies that compare SC-PUL responses to snakes relative to other threatening stimuli, especially natural predators such as felids or raptors, would be informative. This approach would further clarify the role of the SC-PUL in threat detection, innate object recognition, and the SDT. In addition, future studies comparing behavioral and neurophysiological processing of these different types of stimuli in aware and unaware conditions (e.g., by using interocular suppression techniques), would provide new insights on the heated debate regarding the automatic nature of fear stimuli processing. With regard to rapid detection of emotionally charged faces, future studies could investigate whether emotional intensity is a critical factor in search efficiency in detection tasks. Finally, comparative studies are invaluable for understanding the current role of cerebral regions. In general, neuroscientific studies have been performed on a handful of primate species, most of which are closely related macaques. By generalizing results from such few species, our field is nearly blind to ecological and evolutionary clues to the origin and function of brain systems. This seems particularly critical in the case of the SC-PUL system as it is so intimately related to critical survival responses.
Another way to explore the functions of the SC-PUL pathway might be to investigate whether Parkinson's disease (PD) adversely affects the patient's ability to detect emotional faces, to detect and respond appropriately to snakes (and perhaps other biological threats), to reach for and grasp objects, and to point declaratively (Isbell, 2009; Diederich et al., 2014). PD adversely affects the SC-PUL system beginning with the retina and the SC via loss of dopamine from the substantia nigra (Djamgoz et al., 1997; Dommett et al., 2005; Armstrong, 2011). It also damages the PUL and the amygdala (Harding et al., 2002; Diederich et al., 2014).
PD sufferers are indeed less sensitive than non-sufferers to emotional facial expressions (Sprengelmeyer et al., 2003; Armstrong, 2011), perhaps because they are also less sensitive to contrast at lower spatial frequencies (Davidsdottir et al., 2005; Hipp et al., 2014), the frequency realm of the SC-PUL system. As mentioned above, neurons in the SC and PUL are highly sensitive to images of emotional faces (as well as snakes) at low spatial frequencies (Vuilleumier et al., 2003; Le et al., 2013).
Parkinson's patients also have deficits in reaching and grasping (Klockgether and Dichgans, 1994; Lu et al., 2010). For example, PD sufferers who cannot see their hands when they point to or grasp a target can miss the target (Klockgether and Dichgans, 1994). They are often also slower than unaffected people to shape the fingers to grasp, and their shaping movements become even slower without visual feedback (Schettino et al., 2003, 2006; Ansuini et al., 2010). With the automaticity of the dorsal stream, including the SC-PUL circuit, impaired, the burden to adjust is then placed on non-automatic visual and cognitive processes, which may become overloaded, thus causing even greater dysfunction (Lu et al., 2010; Pieruccini-Faria et al., 2014; Nemanich and Earhart, 2016).
While we are unaware of any studies that have deliberately tested PD patients for their responses to snakes or other biologically relevant threats, we note that PD patients often “freeze” as they approach a doorway or an object in their path (Azulay et al., 2006; Okuma, 2006; Cowie et al., 2010; Snijders et al., 2010). Under natural conditions, abrupt freezing is a normal response to rapid visual detection of threatening stimuli, including peripheral and looming objects and dangerous objects in one's path. The SC-PUL visual system is responsive to such stimuli (e.g., looming objects; Billington et al., 2011). Stimulation of the deeper layers of the SC also causes animals to freeze and lesions of the deeper layers abolish defensive behavior (Ellard and Goodale, 1988; Northmore et al., 1988; Sewards and Sewards, 2002; Brandão et al., 2003; DesJardin et al., 2013).
Freezing in PD patients is frequently associated with visual deficits in contrast sensitivity at lower spatial frequencies (Davidsdottir et al., 2005) and in response and speed of saccades (Nemanich and Earhart, 2016), suggesting SC-PUL system impairment. Thus, some of the locomotor deficits in PD might reflect impaired visual detection and an over-response to potential danger.
Future studies might consider investigating the possible role of the SC-PUL visual system in rapid visual detection/rapid motor responses (e.g., freezing) in primates, particularly with regard to snakes and other predators, reaching and grasping, and, in humans, pointing. One promising approach might be to involve patients with PD to test the hypothesis that some of their visual and motor deficits are influenced by damage to the SC-PUL pathway. If our interpretation is correct that freezing is a response to the SC-PUL's danger detection function, with PD the response would then be an over-response whereas the response to emotional facial expressions is an under-response. Testing is needed, however, because it is unclear why these responses would be different.
Several lines of evidence suggest that the SC-PUL pathway might also be involved in the pathology of autism. First, ASD are defined by deficits in social reciprocity and communication, and by unusually restricted, repetitive behaviors (American Psychiatric Association, 2000). Social deficits may be critical to identifying autism's etiology (Schultz, 2005). As reviewed above, SC-PUL neurons respond well to facial photos and face-like patterns (Nguyen et al., 2013, 2014), and population activity of SC-PUL neurons discriminates facial identity, gender, and face orientation in the early latencies (before 100 ms after stimulus onset) (Nguyen et al., 2017). Faces provide important information for triggering social behaviors, and coarse (LSF) information is important for face recognition in newborn babies with relatively immature visual cortical areas (Johnson, 2005; de Heering et al., 2008). Recent studies indicate that holistic face perception is largely supported by low spatial frequencies and suggest that holistic processing precedes the analysis of local features during face perception (Goffaux and Rossion, 2006), and face contours (similar to the face-like patterns in the SC-PUL neurophysiological studies) shortened response latencies to faces in the human occipito-temporal regions (Shibata et al., 2002). This evidence suggests that the SC-PUL pathway plays an important role in social behaviors in early infants before they develop the cortical system for full social behaviors, and that social deficits in autism might be ascribed to some deficits in the SC-PUL system. Consistent with this hypothesis is the finding that declarative pointing, a social behavior that normally develops by about 12 months of age (Tomasello, 2000; Liszkowski et al., 2004), is not done by children with autism (Mundy et al., 1986; Baron-Cohen, 1989, 1995). Moreover, an fMRI study reported that activity in the SC-PUL pathway was substantially reduced in patients with autism in response to facial photos (Kleinhans et al., 2011). A neurophysiological study analyzing evoked potentials also reported that autistic children showed a bias toward HSF stimuli (fearful face, gratings) compared with LSF stimuli, in contrast to control subjects, again suggesting that the subcortical visual pathway including the SC-PUL might be affected in autism (Vlamings et al., 2010). Finally, lesions of the SC induced transient decreases in social behaviors in infant monkeys (Maior et al., 2012).
Second, several studies suggest deficits in disengagement of visual attention as a unique feature of autism in young children (Rodier, 2000; Landry and Bryson, 2004; Elsabbagh et al., 2009, 2013). Orienting attention to a new target requires three sequential mental operations: (1) disengagement of attention from its current focus; (2) moving attention to the new target; and (3) engagement of the new target (Posner et al., 1984; Posner and Petersen, 1990). These studies investigated orienting reactions of young children with and without autism who looked at 3 computer monitors in front of them. Once attention was engaged on a fixation stimulus in the central monitor, a second stimulus was presented on either side, either simultaneously (overlap condition) or successively (gap condition). Reaction time to the peripheral stimuli (new targets) was longer in those children with autism in the overlap condition, in which disengagement of attention to the central monitor was required. Deficits in disengagement are one of the earliest symptoms observed in the development of this disorder and such deficits may underlie the social and cognitive impairments observed in patients with autism (Keehn et al., 2013; Sacrey et al., 2014). The idea that the SC might be involved in attention disengagement processes, and SC malfunctioning and/or malformation might be related to the origin and development of autism, was tested in a behavioral study in which rats were trained in a light-guided spatial choice task (de Araujo et al., 2015). At each trial, the rats had to choose one of two paths, leading either to a large or a small reward, based on cue light(s). In this task, the same cue light (frequent cue light) was repeatedly presented, and another cue light (infrequent cue light) was sometimes presented simultaneously with the frequent cue light. The rats could acquire a large reward if they chose the infrequent cue light, in which both attentional disengagement and shift of attention from the frequent cue light were required. The study indicated that temporary inactivation of the SC selectively impaired performance in this task. A neurophysiological study in rats supports these findings in demonstrating the existence of SC neurons that are related to attention disengagement as well as attention engagement in a comparative task (Ngan et al., 2015). These neurons showed excitatory responses during presentation of a cue light contralateral to the recording sites if the cue required attentional disengagement from an ipsilateral cue light. Furthermore, behavioral latencies to the contralateral cue light requiring attentional disengagement were negatively correlated with response magnitudes of the disengagement-related neurons to the contralateral cue light requiring attentional disengagement. Consistent with these results, a human case study reported that a patient with lesions including the right SC showed deficits in saccades to the contralateral (left) target in an overlap condition requiring disengagement (Pierrot-Deseilligny et al., 1991).
Third, the SC is well known to be involved in saccadic eye movements. Clinical studies reported that children with autism made more frequent saccades during presentation of visual stimuli and in-between stimulus presentations (Kemner et al., 1998), and that inaccurate or slow saccadic movements were often observed in children/infants with autism (Rosenhall et al., 1988; Pensiero et al., 2009). These symptoms may be the result of abnormal activity of the SC or other brainstem areas related to eye movements in autism.
Fourth, the SC is an important structure for sensory gating. Prepulse inhibition (PPI) is an operational measure of sensorimotor gating in which a weak auditory prepulse attenuates the subsequent behavioral responses to a loud startling noise (Braff and Geyer, 1990). Human behavioral studies reported that patients with autism exhibited significantly less PPI (McAlonan et al., 2002; Perry et al., 2007), while there was a downward tendency of PPI in SC-lesioned monkeys (Saletti et al., 2014). In murine models of autism by prenatal exposure to valproic acid or genetic modification, deficits in PPI as well as decreases in parvalbumin-positive neurons in the SC were reported (Dendrinos et al., 2011; Nguyen et al., 2011; Nakamura et al., 2015).
Fifth, clinical studies suggest that dysfunctional serotonin signaling might contribute to abnormal autistic behaviors (Scott and Deneris, 2005). The SC is reported to be involved in a serotonin release in the cortex; electrical stimulation of the SC increased serotonin release in the frontal cortex (Dringenberg et al., 2003). This finding suggests that malfunctioning of the SC could induce a decrease in serotonin release in the cortex, which might induce autistic symptoms.
Sixth, patients with autism, and animals with exposure to valproic acid, show deficits in gamma oscillation in response to sensory stimulation (Gandal et al., 2010). Since PUL neurons show gamma oscillation in response to visual stimuli (Le et al., 2016), malfunctioning of the SC-PUL system could induce deficits in cortical gamma oscillation.
Finally, human morphological studies using MRI reported alteration in the amygdala and thalamus, including the pulvinar, in autism (Tsatsanis et al., 2003; Amaral et al., 2008; Tamura et al., 2010). Although no morphological alterations specific to the SC of patients with autism have been reported, fMRI anatomical comparisons indicate that significant differences in these patients occur in the whole midbrain (including the SC—smaller midbrain) (Brambilla et al., 2003).
Taken together, all of this evidence suggests the involvement of the SC-PUL pathway in ASD. The malfunction of the SC-PUL pathway in the early developmental stage might trigger developmental deficits in the other brain systems, including the cortical system. To our knowledge, this pathway has not been systematically investigated in the context of ASD but the SC and PUL are clearly compelling targets for the behavioral, motor, sensory, and attentional deficits observed in these disorders. Future studies could benefit from incorporating this perspective and examine more directly the role of SC-PUL in ASD.
SCS and RSM organized the structure of the review and wrote the first draft, with the contribution of LAI. All the authors listed have made substantial, direct and intelectual contribution to the work and approved it for publication.
This research was supported partly by Grant-in-Aid for Scientific Research (B) (16H04652) from Japan Society for Promotion of Science (JSPS), Japan, and by FEDER through the operation POCI-01-0145-FEDER-007746 funded by the Programa Operacional Competitividade e Internacionalização—COMPETE2020 and by National Funds through FCT—Fundação para a Ciência e a Tecnologia within CINTESIS, R&D Unit.
The authors declare that the research was conducted in the absence of any commercial or financial relationships that could be construed as a potential conflict of interest.
Acuña, C., Cudeiro, J., Gonzalez, F., Alonso, J. M., and Perez, R. (1990). Lateral-posterior and pulvinar reaching cells – comparison with parietal area 5a: a study in behaving Macaca nemestrina monkeys. Exp. Brain Res. 82, 158–166.
Adams, R. B. Jr., Gordon, H. L., Baird, A. A., Ambady, N., and Kleck, R. E. (2003). Effects of gaze on amygdala sensitivity to anger and fear faces. Science 300:1536. doi: 10.1126/science.1082244
Aggleton, J. P., and Saunders, R. C. (2000). “The amygdala-what's happened in the last decade,” in The Amygdala. A Functional Analysis, 2nd Edn., ed. J. P. Aggleton (Oxford: Oxford University Press), 1–32.
Agras, W. S., Sylvester, D., and Oliveau, D. (1969). The epidemiology of common fear and phobia. Compr. Psychiatry 10, 151–156. doi: 10.1016/0010-440X(69)90022-4
Almeida, I., Soares, S. C., and Castelo-Branco, M. (2015). The distinct role of the amygdala, superior colliculus and pulvinar in processing of central and peripheral snakes. PLoS ONE 10:e0129949. doi: 10.1371/journal.pone.0129949
Amaral, D. G., Schumann, C. M., and Nordahl, C. W. (2008). Neuroanatomy of autism. Trends Neurosci. 31, 137–145. doi: 10.1016/j.tins.2007.12.005
American Psychiatric Association (2000). Diagnostic and Statistical Manual of Mental Disorders DSM-IV-TR, 4th Edn., Text Revision. Washington, DC: American Psychiatric Association.
American Psychological Association (APA) (2013). Diagnostic and Statistical Manual of Mental Disorders, 5th Edn. Washington, DC: American Psychiatric Association.
Anders, S., Eippert, F., Wiens, S., Birbaumer, N., Lotze, M., and Wildgruber, D. (2009). When seeing outweighs feeling: a role for prefrontal cortex in passive control of negative affect in blindsight. Brain 132, 3012–3031. doi: 10.1093/brain/awp212
Ansuini, C., Begliomini, C., Ferrari, T., and Castiello, U. (2010). Testing the effects of end-goal during reach-to grasp movements in Parkinson's disease. Brain Cogn. 74, 169–177. doi: 10.1016/j.bandc.2010.07.015
Armstrong, R. A. (2011). Visual symptoms in parkinson's disease. Parkinson's Dis. 2011:908306. doi: 10.4061/2011/908306
Axelrod, V., Bar, M., and Rees, G. (2015). Exploring the unconscious using faces. Trends Cogn. Sci. 19, 35–45. doi: 10.1016/j.tics.2014.11.003
Azulay, J.-P., Mesure, S., and Blin, O. (2006). Influence of visual cues on gait in Parkinson's disease: contribution to attention or sensory dependence? J. Neurol. Sci. 248, 192–195. doi: 10.1016/j.jns.2006.05.008
Baizer, J. S., Desimone, R., and Ungerleider, L. G. (1993). Comparison of subcortical connections of inferior temporal and posterior parietal cortex in monkeys. Vis. Neurosci. 10, 59–72. doi: 10.1017/S0952523800003229
Baleydièr, C., and Mauguiere, F. (1985). Anatomical evidence for medial pulvinar connections with the posterior cingulate cortex, the retrosplenial area, and the posterior parahippocampal gyrus in monkeys. J. Comp. Neurol. 232, 219–228. doi: 10.1002/cne.902320207
Baleydier, C., and Mauguière, F. (1987). Network organization of the connectivity between parietal area 7 posterior cingulate cortex and medial pulvinar nucleus: a double fluorescent tracer study in monkey. Exp. Brain Res. 66, 385–393. doi: 10.1007/BF00243312
Barbur, J. L., Ruddock, K. H., and Waterfield, V. A. (1980). Human visual responses in the absence of the geniculo-calcarine projection. Brain 103, 905–928. doi: 10.1093/brain/103.4.905
Barbur, J. L., Weiskrantz, L., and Harlow, J. A. (1999). The unseen color aftereffect of an unseen stimulus: insight from blindsight into mechanism of color afterimages. Proc. Natl. Acad. Sci. U.S.A. 96, 11637–11641. doi: 10.1073/pnas.96.20.11637
Baron-Cohen, S. (1989). Perceptual role taking and protodeclarative pointing in autism. Br. J. Dev. Psychol. 7, 113–127. doi: 10.1111/j.2044-835X.1989.tb00793.x
Baron-Cohen, S. (1995). Mindblindness: An Essay on Autism and Theory of Mind. Cambridge, MA: MIT Press.
Barton, R. A. (1998). Visual specialization and brain evolution in primates. Proc. R. Soc. Lond. B 265, 1933–1937. doi: 10.1098/rspb.1998.0523
Becker, D. V., Anderson, U. S., Mortensen, C. R., Neufeld, S. L., and Neel, R. (2011). The face in the crowd effect unconfounded: happy faces, not angry faces, are more efficiently detected in single-and multiple-target visual search tasks. J. Exp. Psychol. Gen. 140, 637–660. doi: 10.1037/a0024060
Becker, M. W. (2009). Panic search: fear produces efficient visual search for nonthreatening objects. Psychol. Sci. 20, 435–437. doi: 10.1111/j.1467-9280.2009.02303.x
Beckstead, R. M., and Frankfurter, A. (1982). The distribution and some morphological features of substantia nigra neurons that project to the thalamus, superior colliculus and pedunculopontine nucleus in the monkey. Neuroscience 7, 2377–2388. doi: 10.1016/0306-4522(82)90202-0
Bender, D. B., and Youakim, M. (2001). Effect of attentive fixation in macaque thalamus and cortex. J. Neurophysiol. 85, 219–234.
Benevento, L. A., and Fallon, J. H. (1975). The ascending projections of the superior colliculus in the rhesus monkey (Macaca mulatta). J. Comp. Neurol. 160, 339–361. doi: 10.1002/cne.901600306
Berggren, N., Richards, N., Taylor, J., and Derakshan, N. (2013). Affective attention under cognitive load: reduced emotional biases but emergent anxiety-related costs to inhibitory control. Front. Hum. Neurosci. 7:188. doi: 10.3389/fnhum.2013.00188
Billington, J., Wilkie, R. M., Field, D. T., and Wann, J. P. (2011). Neural processing of imminent collision in humans. Proc. R. Soc. B 278, 1476–1481. doi: 10.1098/rspb.2010.1895
Bishop, A. (1964). “Use of the hand in lower primates,” in Evolutionary and Genetic Biology of Primates, Vol. 2, ed J. Buettner-Janusch (New York, NY: Academic Press), 133–225.
Blanchard, D. C., and Blanchard, R. J. (1983). Ethoexperimental approaches to the biology of emotion. Annu. Rev. Psychol. 39, 43–68. doi: 10.1146/annurev.ps.39.020188.000355
Blythe, I. M., Kennard, C., and Ruddock, K. H. (1987). Residual vision in patients with retrogeniculate lesions of the visual pathways. Brain 110, 887–905. doi: 10.1093/brain/110.4.887
Bocanegra, B. R., and Zeelenberg, R. (2012). Emotion potentiates response activation and inhibition in masked priming. Front. Integr. Neurosci. 6:109. doi: 10.3389/fnint.2012.00109
Borra, E., Gerbella, M., Rozzi, S., Tonelli, S., and Luppino, G. (2014). Projections to the superior colliculus from inferior parietal, ventral premotor, and ventrolateral prefrontal areas involved in controlling goal-directed hand actions in the macaque. Cereb. Cort. 24, 1054–1065. doi: 10.1093/cercor/bhs392
Braff, D. L., and Geyer, M. A. (1990). Sensorimotor gating and schizophrenia: human and animal model studies. Arch. Gen. Psychiatry 47, 181–188. doi: 10.1001/archpsyc.1990.01810140081011
Brambilla, P., Hardan, A., di Nemi, S. U., Perez, J., Soares, J. C., and Barale, F. (2003). Brain anatomy and development in autism: review of structural MRI studies. Brain Res. Bull. 61, 557–569. doi: 10.1016/j.brainresbull.2003.06.001
Brandão, M. L., Troncoso, A. C., de Souza Silva, M. A., and Huston, J. P. (2003). The relevance of neuronal substrates of defense in the midbrain tectum to anxiety and stress: empirical and conceptual considerations. Eur. J. Pharmacol. 463, 225–233. doi: 10.1016/S0014-2999(03)01284-6
Burrows, A. M. (2008). The facial expression musculature in primates and its evolutionary significance. Bioessays 30, 212–225. doi: 10.1002/bies.20719
Butterworth, G., Franco, F., McKenzie, B., Graupner, L., and Todd, B. (2002). Dynamic aspects of visual event perception and the production of pointing by human infants. J. Develop. Psychol. 20, 1–24. doi: 10.1348/026151002166280
Calvo, M. G., and Marrero, H. (2009). Visual search of emotional faces: the role of affective content and featural distinctiveness. Cogn. Emot. 23, 782–806. doi: 10.1080/02699930802151654
Carey, D. P., Sahraie, A., Trevethan, C. T., and Weiskrantz, L. (2008). Does localization blindsight extend to two-dimensional targets? Neuropsychologia 46, 3053–3060. doi: 10.1016/j.neuropsychologia.2008.06.015
Carey, S. (1992). Becoming a face expert. Philos. Trans. R. Soc. Lond. B. Biol. Sci. 335, 95–102. doi: 10.1098/rstb.1992.0012
Carlsson, K., Petersson, K. M., Lundqvist, D., Karlsson, A., Invgar, M., and Öhman, A. (2004). Fear and the amygdala: manipulation of awareness generates differential cerebral responses to phobic and fear-relevant (but nonfeared) stimuli. Emotion 4, 340–353. doi: 10.1037/1528-3542.4.4.340
Cartmill, M. (1974). Rethinking primate origins. Science 184, 436–443. doi: 10.1126/science.184.4135.436
Cartmill, M. (1992). New views on primate origins. Evol. Anthropol. 6, 105–111. doi: 10.1002/evan.1360010308
Casagrande, V. A. (1994). A third parallel visual pathway to primate area V1. Trends Neurosci. 17, 305–310. doi: 10.1016/0166-2236(94)90065-5
Cecere, R., Bertini, C., Maier, M. E., and Làdavas, E. (2014). Unseen fearful faces influence face encoding: evidence from ERPs in hemianopic patients. J. Cogn. Neurosci. 26, 2564–2577. doi: 10.1162/jocn_a_00671
Celeghin, A., de Gelder, B., and Tamietto, M. (2015). From affective blindsight to emotional consciousness. Conscious. Cogn. 36, 414–425. doi: 10.1016/j.concog.2015.05.007
Chalfin, B. P., Cheung, D. T., Muniz, J. A. P. C., de Lima Silveira, L. C., and Finlay, B. L. (2007). Scaling of neuron number and volume of the pulvinar complex in New World primates: comparisons with humans, other primates, and mammals. J. Comp. Neurol. 504, 265–274. doi: 10.1002/cne.21406
Chalupa, L. M. (1991). “Visual function of the pulvinar,” in The Neural Basis of Visual Function, ed A. G. Leventhal (Boca Raton, FL: CRC Press), 140–159.
Clower, D. M., West, R. A., Lynch, J. C., and Strick, P. L. (2001). The inferior parietal lobule is the target of output from the superior colliculus, hippocampus, and cerebellum. J. Neurosci. 21, 6283–6291.
Cohen Kadosh, K., Johnson, M. H., Henson, R. N., Dick, F., and Blakemore, S. J. (2013). Differential face-network adaptation in children, adolescents and adults. Neuroimage 69, 11–20. doi: 10.1016/j.neuroimage.2012.11.060
Cook, M., and Mineka, S. (1990). Selective associations in the observational conditioning of fear in rhesus monkeys. J. Exp. Psychol. Anim. Behav. 16, 372–389. doi: 10.1037/0097-7403.16.4.372
Cowie, D., Limousin, P., Peters, A., and Day, B. L. (2010). Insights into the neural control of locomotion from walking through doorways in Parkinson's disease. Neuropsychologia 48, 2750–2757. doi: 10.1016/j.neuropsychologia.2010.05.022
Cowie, R. J., and Robinson, D. L. (1994). Subcortical contribu- tions to head movements in macaques. I. Contrasting effects of electrical stimulation of a medial pontomedullary region and the superior colliculus. J. Neurophysiol. 72, 2648–2664.
Crouzet, S. M., and Thorpe, S. J. (2011). Low-level cues and ultra-fast face detection. Front. Psychol. 2:342. doi: 10.3389/fpsyg.2011.00342
Csibra, G., Davis, G., Spratling, M., and Johnson, M. (2000). Gamma oscillations and object processing in the infant brain. Science 290, 1582–1585. doi: 10.1126/science.290.5496.1582
Csibra, G., Tucker, L. A., and Johnson, M. H. (1998). Neural correlates of saccade planning in infants: a high-density ERP study. Int. J. Psychophysiol. 29, 201–215. doi: 10.1016/S0167-8760(98)00016-6
Danckert, J., and Rossetti, Y. (2005). Blindsight in action: what can the different sub-types of blindsight tell us about the control of visually guided actions? Neurosci. Biobehav. Rev. 29, 1035–1046. doi: 10.1016/j.neubiorev.2005.02.001
Davidsdottir, S., Cronin-Golomb, A., and Lee, A. (2005). Visual and spatial symptoms in Parkinson's disease. Vis. Res. 45, 1285–1296. doi: 10.1016/j.visres.2004.11.006
Day-Brown, J. D., Wei, H., Chomsung, R. D., Petry, H. M., and Bickford, M. E. (2010). Pulvinar projections to the striatum and amygdala in the tree shrew. Front. Neuroanat. 4:143. doi: 10.3389/fnana.2010.00143
de Araujo, M. F. P., Matsumoto, J., Ono, T., and Nishijo, H. (2015). An animal model of disengagement; temporary inactivation of the superior colliculus impairs attention disengagement in rats. Behav. Brain Res. 293, 34–40. doi: 10.1016/j.bbr.2015.07.031
de Gelder, B., Vroomen, J., Pourtois, G., and Weiskrantz, L. (1999). Non-conscious recognition of affect in the absence of striate cortex. Neuroreport 10, 3759–3763. doi: 10.3389/fnana.2011.00034
de Heering, A., Turati, C., Rossion, B., Bulf, H., Goffaux, V., and Simion, F. (2008). Newborns' face recognition is based on spatial frequencies below 0.5 cycles per degree. Cognition 106, 444–454. doi: 10.1016/j.cognition.2006.12.012
Dendrinos, G., Hemelt, M., and Keller, A. (2011). Prenatal VPA Exposure and changes in sensory processing by the superior colliculus. Front. Integr. Neurosci. 5:68. doi: 10.3389/fnint.2011.00068
DesJardin, J. T., Holmes, A. L., Forcelli, P. A., Cole, C. E., Gale, J. T., Wellman, L. L., et al. (2013). Defense-like behaviors evoked by pharmacological disinhibition of the superior colliculus in the primate. J. Neurosci. 33, 150–155. doi: 10.1523/JNEUROSCI.2924-12.2013
de Winter, W., and Oxnard, C. E. (2001). Evolutionary radiations and convergences in the structural organization of mammalian brains. Nature 409, 710–714. doi: 10.1038/35055547
Diederich, N. J., Stebbins, G., Schiltz, C., and Goetz, C. G. (2014). Are patients with Parkinson's disease blind to blindsight? Brain 137, 1838–1849. doi: 10.1093/brain/awu094
Djamgoz, M. B. A., Hankins, M. W., Hirano, J., and Archer, S. N. (1997). Neurobiology of retinal dopamine in relation to degenerative states of the tissue. Vis. Res. 37, 3509–3529. doi: 10.1016/S0042-6989(97)00129-6
Dobson, S. D. (2009). Socioecological correlates of facial mobility in nonhuman primates. Amer. J. Phys. Anthropol. 139, 413–420. doi: 10.1002/ajpa.21007
Dobson, S. D., and Sherwood, C. C. (2011). Correlated evolution of brain regions involved in producing and processing facial expressions in anthropoid primates. Biol. Lett. 7, 86–88. doi: 10.1098/rsbl.2010.0427
Dommett, E., Coizet, V., Blaha, C. D., Martindale, J., Lefebvre, V., Walton, N., et al. (2005). How visual stimuli activate dopaminergic neurons at short latency. Science 307, 1476–1479. doi: 10.1126/science.1107026
Dringenberg, H. C., Vanderwolf, C. H., and Noseworthy, P. A. (2003). Superior colliculus stimulation enhances neocortical serotonin release and electrocorticographic activation in the urethane-anesthetized rat. Brain Res. 964, 31–41. doi: 10.1016/S0006-8993(02)04062-3
Ellard, C. G., and Goodale, M. A. (1988). A functional analysis of the collicular output pathways: a dissociation of deficits following lesions of the dorsal tegmental decussation and the ipsilateral collicular efferent bundle in the Mongolian gerbil. Exp. Brain Res. 71, 307–319. doi: 10.1007/BF00247491
Ellerman, J., Siegal, J. D., Strupp, J. P., Ebner, T. J., and Ugurbil, K. (1998). Activation of visuomotor systmes during visually guided movements: a functional MRI study. J. Magn. Reson. 131, 272–285. doi: 10.1006/jmre.1998.1379
Elsabbagh, M., Fernandes, J., Jane Webb, S., Dawson, G., Charman, T., Johnson, M. H., et al. (2013). Disengagement of visual attention in infancy is associated with emerging autism in toddlerhood. Biol. Psychiatry 74, 189–194. doi: 10.1016/j.biopsych.2012.11.030
Elsabbagh, M., Volein, A., Holmboe, K., Tucker, L., Csibra, G., Baron-Cohen, S., et al. (2009). Visual orienting in the early broader autism phenotype: disengagement. J. Child Psychol. Psychiatry 50, 637–642. doi: 10.1111/j.1469-7610.2008.02051.x
Ewert, J.-P. (1970). Neural mechanisms of prey-catching and avoidance behavior in the toad (Bufo bufo L.). Brain Behav. Evol. 3, 36–56. doi: 10.1159/000125462
Fox, E., Russo, R., Bowles, R. J., and Dutton, K. (2001). Do threatening stimuli draw or hold visual attention in subclinical anxiety? J. Exp. Psychol. Gen. 130, 681–700. doi: 10.1037/0096-3445.130.4.681
Fredrikson, M., Annas, P., Fischer, H., and Wik, G. (1996). Gender and age differences in the prevalence of specific fears and phobias. Behav. Res. Ther. 34, 33–39. doi: 10.1016/0005-7967(95)00048-3
Freedman, E. G., and Sparks, D. L. (1997). Activity of cells in the deeper layers of the superior colliculus of the rhesus monkey: evidence for a gaze displacement command. J. Neurophysiol. 78, 1669–1690.
Gabay, S., Burlingham, C., and Behrmann, M. (2014). The nature of face representations in subcortical regions. Neuropsychologia 59, 35–46. doi: 10.1016/j.neuropsychologia.2014.04.010
Gandal, M. J., Edgar, J. C., Ehrlichman, R. S., Mehta, M., Roberts, T. P., and Siegel, S. J. (2010). Validating γ oscillations and delayed auditory responses as translational biomarkers of autism. Biol. Psychiatry 68, 1100–1106. doi: 10.1016/j.biopsych.2010.09.031
Gandhi, N. J., and Katnani, H. A. (2011). Motor functions of the superior colliculus. Annu. Rev. Neurosci. 34, 205–231. doi: 10.1146/annurev-neuro-061010-113728
Garey, L. J., Dreher, B., and Robinson, S. R. (1991). “The organization of the visual thalamus,” in Neuroanatomy of the Visual Pathways and Their Development, eds B. Dreher and S. R. Robinson (Boca Raton, FL: CRC Press), 176–234.
Garrido, M. I., Barnes, G. R., Sahani, M., and Dolan, R. J. (2012). Functional evidence for a dual route to amygdala. Curr. Biol. 22, 129–134. doi: 10.1016/j.cub.2011.11.056
Garvert, M. M., Friston, K. J., Dolan, R. J., and Garrido, M. I. (2014). Subcortical amygdala pathways enable rapid face processing. Neuroimage 102, 309–316. doi: 10.1016/j.neuroimage.2014.07.047
Gebo, D. L. (2004). A shrew-sized origin for primates. Yearb. Phys. Anthropol. 47, 40–62. doi: 10.1002/ajpa.20154
Georgiou, G. A., Bleakley, C., Hayward, J., Russo, R., Dutton, K., Eltiti, S., et al. (2005). Focusing on fear: attention disengagement from emotional faces. Vis. Cogn. 12, 145–158. doi: 10.1080/13506280444000076
Glendenning, K. K., Hall, J. A., Diamond, I. T., and Hall, W. C. (1975). The pulvinar nucleus of Galago senegalensis. J. Comp. Neurol. 161, 419–458. doi: 10.1002/cne.901610309
Goffaux, V., and Rossion, B. (2006). Faces are “spatial”–holistic face perception is supported by low spatial frequencies. J. Exp. Psychol. Hum. Percept. Perform. 32, 1023–1039. doi: 10.1037/0096-1523.32.4.1023
Gomes, N., Silva, S., Silva, C. F., and Soares, S. C. (2017). Beware the serpent: the advantage of ecologically-relevant stimuli in accessing visual awareness. Evol. Hum. Behav. 38, 227–234. doi: 10.1016/j.evolhumbehav.2016.10.004
Goodale, M. A., and Milner, A. D. (1992). Separate visual pathways for perception and action. Trends Neurosci. 15, 20–25. doi: 10.1016/0166-2236(92)90344-8
Goodale, M. A., and Westwood, D. A. (2004). An evolving view of duplex vision: separate but interacting cortical pathways for perception and action. Curr. Opin. Neurobiol. 14, 203–211. doi: 10.1016/j.conb.2004.03.002
Goren, C. C., Sarty, M., and Wu, P. Y. K. (1975). Visual following and pattern-discrimination of face-like stimuli by newborn-infants. Pediatrics 56, 544–549.
Grassini, S., Holm, S. K., Railo, H., and Koivisto, M. (2016). Who is afraid of the invisible snake? Subjective visual awareness modulates posterior brain activity for evolutionarily threatening stimuli. Biol. Psychol. 121, 53–61. doi: 10.1016/j.biopsycho.2016.10.007
Grieve, K. L., Acuña, C., and Cudeiro, J. (2000). The primate pulvinar nuclei: vision and action. Trends Neurol. Sci. 23, 35–39. doi: 10.1016/S0166-2236(99)01482-4
Groh, J. M., and Sparks, D. L. (1996). Saccades to somatosensory targets. I. Behavioral characteristics. J. Neurophysiol. 75, 412–427.
Grüsser, O. J., and Landis, T. (1991). Visual Agnosia and Other Disturbances of Visual Perception and Cognition. London: MacMillan.
Gursky, S. (2005). Predator mobbing in spectral tarsiers. Int. J. Primatol. 26, 207–236. doi: 10.1007/s10764-005-0731-0
Gutierrez, C., Cola, M. G., Seltzer, B., and Cusick, C. (2000). Neurochemical and connectional organization of the dorsal pulvinar complex in monkeys. J. Comp. Neurol. 419, 61–86. doi: 10.1002/(SICI)1096-9861(20000327)419:1<61::AID-CNE4>3.0.CO;2-I
Hall, N., and Colby, C. (2013). Psychophysical definition of S-cone stimuli in the macaque. J. Vis. 13, 1–18. doi: 10.1167/13.2.20
Harding, A. J., Stimson, E., Henderson, J. M., and Halliday, G. M. (2002). Clinical correlates of selective pathology in the amygdala of patients with Parkinson's disease. Brain 125, 2431–2445. doi: 10.1093/brain/awf251
Harting, J. K., Huerta, M. F., Frankfurter, A. J., Strominger, N. L., and Royce, G. J. (1980). Ascending pathways from the monkey superior colliculus: an autoradiographic analysis. J. Comp. Neurol. 192, 853–882. doi: 10.1002/cne.901920414
Hayakawa, S., Kawai, N., and Masataka, N. (2011). The influence of color on snake detection in visual search in human children. Sci. Rep. 1, 1–4. doi: 10.1038/srep00080
He, H., Kubo, K., and Kawai, N. (2014). Spiders do not evoke greater early posterior negativity in the event-related potential as snakes. Neuroreport 25, 1049–1053. doi: 10.1097/WNR.0000000000000227
Headland, T. N., and Greene, H. W. (2011). Hunter-gatherers and other primates as prey, predators, and competitors of snakes. Proc. Natl. Acad. Sci. U.S.A. 108, E1470–E1474. doi: 10.1073/pnas.1115116108
Henry, G. H., and Vidyasagar, T. R. (1991). “Evolution of mammalian visual pathways,” in Vision and Visual Dysfunction: Evolution of the Eye and Visual System, Vol. 2, eds J. R. Cronly-Dillon and R. L. Gregory (Boca Raton, FL: CRC Press), 442–465.
Hipp, G., Diederich, N. J., Pieria, V., and Vaillant, M. (2014). Primary vision and facial emotion recognition in early Parkinson's disease. J. Neurol. Sci. 338, 178–182. doi: 10.1016/j.jns.2013.12.047
Hubel, D. H., and Livingstone, M. S. (1987). Segregation of form, color, and stereopsis in primate area 18. J. Neurosci. 7, 3378–3415.
Huerta, M. F., and Harting, J. K. (1983). Sublamination within the superficial gray layer of the squirrel monkey: an analysis of the tectopulvinar projection using anterograde and retrograde transport methods. Brain Res. 261, 119–126. doi: 10.1016/0006-8993(83)91290-8
Huerta, M. F., and Harting, J. K. (1984). “The mammalian superior colliculus: studies of its morphology and connections,” in Comparative Neurology of the Optic Tectum, ed H. Vanegas (New York, NY: Plenum Press), 687–773.
Huerta, M. F., Van Lieshout, D. P., and Harting, J. K. (1991). Nigrotectal projections in the primate Galago crassicaudatus. Exp. Brain Res. 87, 389–401. doi: 10.1007/BF00231856
Ignashchenkova, A., Dicke, P. W., Haarmeier, T., and Thier, P. (2004). Neuronspecific contribution of the superior colliculus to overt and covert shifts of attention. Nature Neurosci. 7, 56–64. doi: 10.1038/nn1169
Isbell, L. A. (2006). Snakes as agents of evolutionary change in primate brains. J. Hum. Evol. 51, 1–35. doi: 10.1016/j.jhevol.2005.12.012
Isbell, L. A. (2009). The Fruit, the Tree, and the Serpent: Why We See So Well. Cambridge, Mass: Harvard University Press.
Isbell, L. A., and Etting, S. F. (2017). Scales drive visual detection, attention, and memory of snakes in wild vervet monkeys (Chlorocebus pygerythrus). Primates 58, 121–129. doi: 10.1007/s10329-016-0562-y
Jacobs, G. H. (2008). Primate color vision: a comparative perspective. Vis. Neurosci. 25, 619–633. doi: 10.1017/S0952523808080760
Jacobs, G. H. (2009). Evolution of colour vision in mammals. Phil. Trans. Biol. Sci. 364, 2957–2967. doi: 10.1098/rstb.2009.0039
Jay, M. F., and Sparks, D. L. (1987). Sensorimotor integration in the primate superior colliculus. II. Coordinates of auditory signals. J. Neurophysiol. 57, 35–55.
Johnson, M. H. (2005). Subcortical face processing. Nat. Rev. Neurosci. 6, 766–774. doi: 10.1038/nrn1766
Johnson, M. H. (2011). Face processing as a brain adaptation at multiple timescales. Q. J. Exp. Psychol. (Hove) 64, 1873–1888. doi: 10.1080/17470218.2011.590596
Johnson, M. H., Dziurawiec, S., Ellis, H., and Morton, J. (1991). Newborns preferential tracking of face-like stimuli and its subsequent decline. Cognition 40, 1–19. doi: 10.1016/0010-0277(91)90045-6
Johnson, M. H., and Morton, J. (1991). Biology and Cognitive Development: The Case of Face Recognition. Oxford: Blackwell.
Johnson, M. H., Senju, A., and Tomalski, P. (2015). The two-process theory of face processing: modifications based on two decades of data from infants and adults. Neurosci. Biobehav. Rev. 50, 169–179. doi: 10.1016/j.neubiorev.2014.10.009
Jones, E. G., and Burton, H. (1976). A projection from the medial pulvinar to the amygdala in primates. Brain Res. 104, 142–147. doi: 10.1016/0006-8993(76)90654-5
Kaas, J. H. (2004). “Early visual areas: V1, V2, V3, DM, DL, and MT,” in The Primate Visual System, eds J. H. Kaas and C. E. Collins (New York, NY: CRC Press), 139–159.
Kaas, J. H. (2013). The evolution of brains from early mammals to humans. Wiley Interdiscip. Rev. Cogn. Sci. 4, 33–45. doi: 10.1002/wcs.1206
Kaas, J. H., and Huerta, M. F. (1988). “The subcortical visual system of primates,” in Comparative Primate Biology, Vol. 4, eds H. D. Steklis and J. Erwin (New York, NY: Alan R. Liss), 327–391.
Kaas, J. H., and Lyon, D. C. (2007). Pulvinar contributions to the dorsal and ventral streams of visual processing in primates. Brain Res. Rev. 55, 285–296. doi: 10.1016/j.brainresrev.2007.02.008
Kanwisher, N., McDermott, J., and Chun, M. M. (1997). The fusiform face area: a module in human extrastriate cortex specialized for face perception. J. Neurosci. 17, 4302–4311.
Kawai, N., and He, H. (2016). Breaking snake camouflage: humans detect snakes more accurately than other animals in less discernable visual conditions. PLoS ONE 11:e0164342. doi: 10.1371/journal.pone.0164342
Kawai, N., and Koda, H. (2016). Japanese monkeys (Macaca fuscata) quickly detect snakes but not spiders: evolutionary origins of fear-relevant animals. J. Comp. Psychol. 130, 299–303. doi: 10.1037/com0000032
Kawai, N., Kubo, K., Masataka, N., and Hayakawa, S. (2016). Conserved evolutionary history for quick detection of threatening faces. Anim. Cogn. 19, 655–660. doi: 10.1007/s10071-015-0949-y
Kay, R. F., and Kirk, E. C. (2000). Osteological evidence for the evolution of activity pattern and visual acuity in primates. Amer. J. Phys. Anthropol. 113, 235–262. doi: 10.1002/1096-8644(200010)113:2<235::AID-AJPA7>3.0.CO;2-9
Keehn, B., Muller, R. A., and Townsend, J. (2013). Atypical attentional networks and the emergence of autism. Neurosci. Biobehav. Rev. 37, 164–183. doi: 10.1016/j.neubiorev.2012.11.014
Kemner, C., Verbaten, M. N., Cuperus, J. M., Camfferman, G., and van Engeland, H. (1998). Abnormal saccadic eye movements in autistic children. J. Autism Dev Disord. 28, 61–67. doi: 10.1023/A:1026015120128
Khalid, S., Finkbeiner, M., König, P., and Ansorge, U. (2013). Subcortical human face processing? Evidence from masked priming. J. Exp. Psychol. Hum. Percept. Perform. 39, 989–1002. doi: 10.1037/a0030867
Kirk, E. C., and Kay, R. F. (2004). “The evolution of high visual acuity in the Anthropoidea,” in Anthropoid Origins: New Visions, eds C. Ross and R. F. Kay (New York, NY: Kluwer Academic; Plenum Press), 539–602.
Kleinhans, N. M., Richards, T., Johnson, L. C., Weaver, K. E., Greenson, J., Dawson, G., et al. (2011). fMRI evidence of neural abnormalities in the subcortical face processing system in ASD. Neuroimage 54, 697–704. doi: 10.1016/j.neuroimage.2010.07.037
Klockgether, T., and Dichgans, J. (1994). Visual control of arm movement in Parkinson's disease. Mov. Disord. 9, 48–56. doi: 10.1002/mds.870090108
Kobatake, E., and Tanaka, K. (1994). Neuronal selectivities to complex object features in the ventral visual pathway of the macaque cerebral cortex. J. Neurophysiol. 71, 856–867.
Krauzlis, R. J., Basso, M. A., and Wurtz, R. H. (2000). Discharge properties of neurons in the rostral superior colliculus of the monkey during smooth-pursuit eye movements. J. Neurophysiol. 84, 876–891.
LaBerge, D., and Buchsbaum, M. S. (1990). Positron emission tomographic measurements of pulvinar activity during an attention task. J. Neurosci. 10, 613–619.
Landman, R., Sharma, J., Sur, M., and Desimone, R. (2014). Effect of distracting faces on visual selective attention in the monkey. Proc. Natl. Acad. Sci. U.S.A. 111, 18037–18042. doi: 10.1073/pnas.1420167111
Landry, R., and Bryson, S. E. (2004). Impaired disengagement of attention in young children with autism. J. Child Psychol. Psychiatry 45, 1115–1122. doi: 10.1111/j.1469-7610.2004.00304.x
Lang, P., and Bradley, M. (2010). Emotion and the motivational brain. Biol. Psychol. 84, 437–450. doi: 10.1016/j.biopsycho.2009.10.007
Lang, P. J., Bradley, M. M., and Cuthbert, B. N. (1997). International Affective Picture System (IAPS): Technical Manual and Affective Ratings. Gainesville, FL: NIMH; Center for the Study of Emotion and Attention
Lang, P. J., Bradley, M. M., and Cuthbert, B. N. (2005). International Affective Picture System (IAPS): Instruction Manual and Affective Ratings. Technical Report A-6, The Center for Research in Psychophysiology, University of Florida.
Lavie, N., Ro, T., and Russell, C. (2003). The role of perceptual load in processing distractor faces. Psychol. Sci. 14, 510–515. doi: 10.1111/1467-9280.03453
Le, Q. V., Isbell, L. A., Matsumoto, J., Quang, L. V., Nishimaru, H., Hori, E., et al. (2016). Snakes elicit earlier, and monkey faces, later, gamma oscillations in macaque pulvinar neurons. Sci. Rep. 6:20595. doi: 10.1038/srep20595
Le, Q. V., Isbell, L. A., Matsumoto, J., Quang, L. V., Tran, A. H., Maior, R. S., et al. (2014). Monkey pulvinar neurons' differential firing to snake postures. PLoS ONE 9:e114258. doi: 10.1371/journal.pone.0114258
Le, Q. V., Isbell, L. A., Nguyen, M. N., Matsumoto, J., Hori, E., Maior, R. S., et al. (2013). Pulvinar neurons reveal neurobiological evidence of past selection for rapid detection of snakes. Proc. Natl. Acad. Sci. U.S.A. 110, 19000–19005. doi: 10.1073/pnas.1312648110
Leavens, D. A., Hopkins, W. D., and Bard, K. A. (2005). Understanding the point of chimpanzee pointing. Curr. Dir. Psychol. Sci. 14, 185–189. doi: 10.1111/j.0963-7214.2005.00361.x
Lee, P. H., Helms, M. C., Augustine, G. J., and Hall, W. C. (1997). Role of intrinsic synaptic circuitry in collicular sensorimotor integration. Proc. Natl. Acad. Sci. U.S.A. 94, 13299–13304. doi: 10.1073/pnas.94.24.13299
Leventhal, A. G., Rodeick, R. W., and Dreher, B. (1981). Retinal ganglion cell classes in the Old World monkey: morphology and central projections. Science 213, 1139–1142. doi: 10.1126/science.7268423
Levine, S., Atha, K., and Wiener, S. G. (1993). Early experience effects on the development of fear in the squirrel monkey. Behav. Neural Biol. 60, 225–233. doi: 10.1016/0163-1047(93)90428-K
Lin, Z., and He, S. (2009). Seeing the invisible: the scope and limits of unconscious processing in binocular rivalry. Prog. Neurobiol. 87, 195–211. doi: 10.1016/j.pneurobio.2008.09.002
Linke, R., De Lima, A. D., Schwegler, H., and Pape, H. C. (1999). Direct synaptic connections of axons from superior colliculus with identified thalamo-amygdaloid projection neurons in the rat: possible substrates of a subcortical visual pathway to the amygdala. J. Comp. Neurol. 403, 158–170.
Liszkowski, U., Carpenter, M., Henning, A., Striano, T., and Tomasello, M. (2004). Twelve-month-olds point to share attention and interest. Dev. Sci. 7, 297–307. doi: 10.1111/j.1467-7687.2004.00349.x
LoBue, V., and DeLoache, J. S. (2008). Detecting the snake in the grass: attention to fear-relevant stimuli by adults and young children. Psychol. Sci. 19, 284–289. doi: 10.1111/j.1467-9280.2008.02081.x
LoBue, V., Rakinson, D. H., and DeLoache, J. S. (2010). Threat perception across the life span: evidence for multiple converging pathways. Curr. Dir. Psychol. Sci. 19, 375–379. doi: 10.1177/0963721410388801
Lu, C., Bharmal, A., and Kiss, Z. H. (2010). Attention and reach-to-grasp movements in Parkinson's disease. Exp. Brain Res. 205. 69–80. doi: 10.1007/s00221-010-2341-0
Lund, R. D. (1972). Synaptic patterns in the superficial layers of the superior colliculus of the monkey, Macaca mulatta. Exp Brain Res. 15, 194–211. doi: 10.1007/BF00235582
Lundqvist, D., Juth, P., and Öhman, A. (2014). Using facial emotional stimuli in visual search experiments: the arousal factor explains contradictory results. Cogn. Emot. 28, 1012–1029. doi: 10.1080/02699931.2013.867479
Lünenburger, L., Kleiser, R., Stuphorn, V., Miller, L. E., and Hoffmann, K.-P. (2001). “A possible role of the superior colliculus in eye-hand coordination,” in Progress in Brain Research, Vol. 134, eds C. Casanova and M. Ptito (Amsterdam: Elsevier), 109–125.
Lünenburger, L., Kutz, D. F., and Hoffmann, K.-P. (2000). Influence of arm movements on saccades in humans. Eur. J. Neurosci. 12, 4107–4116. doi: 10.1046/j.1460-9568.2000.00298.x
Lyon, D. C., Nassi, J. J., and Callaway, E. M. (2010). A disynaptic relay from superior colliculus to dorsal stream visual cortex in macaque monkey. Neuron 65, 270–279. doi: 10.1016/j.neuron.2010.01.003
Ma, T. P., Lynch, J. C., Donahoe, D. K., Attallah, H., and Rafols, J. A. (1998). Organization of the medial pulvinar nucleus in the macaque. Anat. Rec. 250, 220–237.
Maior, R. S., Hori, E., Barros, M., Teixeira, D. S., Tavares, M. C. H., Ono, T., et al. (2011). Superior colliculus lesions impair threat responsiveness in infant capuchin monkeys. Neurosci. Lett. 504, 257–260. doi: 10.1016/j.neulet.2011.09.042
Maior, R. S., Hori, E., Tomaz, C., Ono, T., and Nishijo, H. (2010). The monkey pulvinar neurons differentially respond to emotional expressions of human faces. Behav. Brain Res. 215, 129–135. doi: 10.1016/j.bbr.2010.07.009
Maior, R. S., Hori, E., Uribe, C. E., Saletti, P. G., Ono, T., Nishijo, H., et al. (2012). A role for the superior colliculus in the modulation of threat responsiveness in primates: towards the ontogenesis of the social brain. Rev. Neurosci. 22, 697–706. doi: 10.1515/revneuro-2012-0055
Margariños-Ascone, C., Buño, W. Jr., and García -Austt, E. (1988). Monkey pulvinar units related to motor activity and sensory response. Brain Res. 445, 30–38.
Martín-Rodriguez, J. G., Buño, W. Jr., and García-Austt, E. (1982). Human pulvinar units, spontaneous activity and sensory-motor influences. Electropenceph. Clin. Neurophys. 54, 388–398.
Masataka, N., Hayakawa, S., and Kawai, N. (2010). Human young children as well as adults demonstrate “superior” rapid snake detection when typical striking posture is displayed by the snake. PLoS ONE 5:e15122. doi: 10.1371/journal.pone.0015122
May, P. (2006). The mammalian superior colliculus: laminar structure and connections. Prog. Brain Res. 151, 321–378. doi: 10.1016/S0079-6123(05)51011-2
McAlonan, G. M., Daly, E., Kumari, V., Critchley, H. D., van Amelsvoort, T., Suckling, J., et al. (2002). Brain anatomy and sensorimotor gating in Asperger's syndrome. Brain 125, 1594–1606. doi: 10.1093/brain/awf150
Meno, W., Coss, R. G., and Perry, S. (2013). Development of snake-directed antipredator behavior by wild white-faced capuchin monkeys: I. Snake-species discrimination. Am. J. Primatol. 75, 281–291. doi: 10.1002/ajp.22106
Miller, M., Pasik, P., and Pasik, T. (1980). Extrageniculostriate vision in the monkey. VII. Contrast sensitivity functions. J. Neurophysiol. 43, 1510–1526.
Mineka, S., Keir, R., and Price, V. (1980). Fear of snakes in wild- and laboratory-reared rhesus monkeys (Macaca mulatta). Anim. Learn. Behav. 8, 653–663. doi: 10.3758/BF03197783
Mondloch, C. J., Lewis, T. L., Budreau, D. R., Maurer, D., Dannemiller, J. L., Stephens, R., et al. (1999). Face perception during early infancy. Psychol. Sci. 10, 419–423. doi: 10.1111/1467-9280.00179
Morris, J. S., DeGelder, B., Weiskrantz, L., and Dolan, R. J. (2001). Differential extrageniculostriate and amygdala responses to presentation of emotional faces in a cortically blind field. Brain 124, 1241–1252. doi: 10.1093/brain/124.6.1241
Morris, J. S., Friston, K. J., and Dolan, R. J. (1997). Neural responses to salient visual stimuli. Proc. R. Soc. Lond. B 264, 769–775. doi: 10.1098/rspb.1997.0109
Moschovakis, A. K., Karabelas, A. B., and Highstein, S. M. (1988). Structure-function relationships in the primate superior colliculus. I. Morphological classification of efferent neurons. J. Neurophysiol. 60, 232–262.
Mundy, P., Sigman, M., Ungerer, J., and Sherman, T. (1986). Defining the socialdeficits of autism: the contribution of non-verbal communication measures. J. Child Psychol. Psychiatry 27, 657–669. doi: 10.1111/j.1469-7610.1986.tb00190.x
Nagy, A., Kruse, W., Rottmann, S., Dannenberg, S., and Hoffmann, K.-P. (2006). Somatosensory-motor neuronal activity in the superior colliculus of the primate. Neuron 52, 525–534. doi: 10.1016/j.neuron.2006.08.010
Nakagawa, S., and Tanaka, S. (1984). Retinal projections to the pulvinar nucleus of the macaque monkey: a re-investigation using autoradiography. Exp. Brain Res. 57, 151–157. doi: 10.1007/BF00231141
Nakamura, T., Matsumoto, J., Takamura, Y., Ishii, Y., Sasahara, M., Ono, T., et al. (2015). Relationships among parvalbumin-immunoreactive neuron density, phase-locked gamma oscillations, and autistic/schizophrenic symptoms in PDGFR-β knock-out and control mice. PLoS ONE 10:e0119258. doi: 10.1371/journal.pone.0119258
Nakano, T., Higashida, N., and Kitazawa, S. (2013). Facilitation of face recognition through the retino-tectal pathway. Neuropsychology 51, 2043–2049. doi: 10.1016/j.neuropsychologia.2013.06.018
Nakano, T., and Nakatani, K. (2014). Cortical networks for face perception in two-month-old infants. Proc. Biol. Sci. 281:20141468. doi: 10.1098/rspb.2014.1468
Neggers, S. F. W., and Bekkering, H. (2002). Coordinated control of eye and hand movements in dynamic reaching. Hum. Mov. Sci. 21, 349–376. doi: 10.1016/S0167-9457(02)00120-3
Nemanich, S. T., and Earhart, G. M. (2016). Freezing gait is associated with increased saccade latency and variability in Parkinson's disease. Clin. Neurophysiol. 127, 2394–2401. doi: 10.1016/j.clinph.2016.03.017
Ngan, N. H., Matsumoto, J., Takamura, Y., Tran, A. H., Ono, T., and Nishijo, H. (2015). Neuronal correlates of attention and its disengagement in the superior colliculus of rat. Front. Integr. Neurosci. 9:9. doi: 10.3389/fnint.2015.00009
Nguyen, M. N., Hori, E., Matsumoto, J., Tran, A. H., Ono, T., and Nishijo, H. (2013). Neuronal responses to face-like stimuli in the monkey pulvinar. Eur. J. Neurosci. 37, 35–51. doi: 10.1111/ejn.12020
Nguyen, M. N., Matsumoto, J., Hori, E., Maior, R. S., Tomaz, C., Tran, A. H., et al. (2014). Neuronal responses to face-like and facial stimuli in the monkey superior colliculus. Front. Behav. Neurosci. 8:85. doi: 10.3389/fnbeh.2014.00085
Nguyen, M. N., Nishimaru, H., Matsumoto, J., Hori, E., Maior, R. S., Tomaz, C., et al. (2017). Population coding of facial information in the monkey superior colliculus and pulvinar. Front. Neurosci. 10:583. doi: 10.3389/fnins.2016.00583
Nguyen, P. T. H., Nakamura, T., Hori, E., Urakawa, S., Uwano, T., Zhao, J., et al. (2011). Cognitive and socio-emotional deficits in platelet-derived growth factor receptor-β gene knockout mice. PLoS ONE 6:e18004. doi: 10.1371/journal.pone.0018004
Northmore, O. P. M., Levine, E. S., and Schneider, G. E. (1988). Behavior evoked by electrical stimulation of the hamster superior colliculus. Exp. Brain Res. 73, 595–605. doi: 10.1007/BF00406619
Nummenmaa, L., and Calvo, M. G. (2015). Dissociation between recognition and detection advantage for facial expressions: a meta-analysis. Emotion 15, 243–256. doi: 10.1037/emo0000042
Öhman, A., Dimberg, U., and Öst, L.-G. (1985). “Animal and social phobias: biological constraints on learned fear responses,” in Theoretical Issues in Behavior Therapy, eds S. Reiss and R. R. Bootzin (New York, NY: Academic Press), 123–175.
Öhman, A., Esteves, F., and Soares, J. (1995). Preparedness and preattentive associative learning: electrodermal conditioning to masked stimuli. J. Psychophysiol. 9, 99–108.
Öhman, A., Flykt, A., and Esteves, F. (2001). Emotion drives attention: detecting the snake in the grass. J. Exp. Psychol. Gen. 130, 466–478. doi: 10.1037/0096-3445.130.3.466
Öhman, A., Fredrikson, M., and Hugdahl, K. (1978). Orienting and defensive responding in the electrodermal system: palmar-dorsal differences and recovery rate during conditioning to potentially phobic stimuli. Psychophysiology 15, 93–101. doi: 10.1111/j.1469-8986.1978.tb01342.x
Öhman, A., Juth, P., and Lundqvist, D. (2010). Finding the face in a crowd: relationships between distractor redundancy, target emotion, and target gender. Cogn. Emot. 24, 1216–1228. doi: 10.1080/02699930903166882
Öhman, A., and Mineka, S. (2001). Fears, phobias, and preparedness: toward an evolved module of fear and fear learning. J. Pers. Soc. Psychol. 80, 381–396. doi: 10.1037/0022-3514.80.3.381
Öhman, A., and Mineka, S. (2003). The malicious serpent: snakes as a prototypical stimulus for an evolved module of fear. Curr. Dir. Psychol. Sci. 12, 5–9. doi: 10.1111/1467-8721.01211
Öhman, A., and Soares, J. F. (1993). On the automatic nature of phobic fear: conditioned electrodermal responses to masked fear-relevant stimuli. J. Abnorm. Psychol. 102, 121–132. doi: 10.1037/0021-843X.102.1.121
Öhman, A., and Soares, J. F. F. (1994). “Unconscious anxiety”: phobic responses to masked stimuli. J. Abnorm. Psychol. 103, 231–240. doi: 10.1037/0021-843X.103.2.231
Öhman, A., Soares, S. C., Juth, P., Lindström, B., and Esteves, F. (2012). Evolutionary derived modulations of attention to two common fear stimuli: serpents and hostile humans. J. Cogn. Psychol. 24, 17–32. doi: 10.1080/20445911.2011.629603
Okuma, Y. (2006). Freezing of gait in Parkinson's disease. J. Neurol. 253 (Suppl. 7), VII/27–VII/32. doi: 10.1007/s00415-006-7007-2
Penkunas, M. J., and Coss, R. G. (2013a). A comparison of rural and urban Indian children's visual detection of threatening and nonthreatening animals. Dev. Sci. 16, 463–475. doi: 10.1111/desc.12043
Penkunas, M. J., and Coss, R. G. (2013b). Rapid detection of visually provocative animals by preschool children and adults. J. Exp. Child Psychol. 114, 522–536. doi: 10.1016/j.jecp.2012.10.001
Pensiero, S., Fabbro, F., Michieletto, P., Accardo, A., and Brambilla, P. (2009). Saccadic characteristics in autistic children. Funct. Neurol. 24, 153–158.
Perry, V. H., and Cowey, A. (1984). Retinal ganglion cells that project to the superior colliculus and pretectum in the macaque monkey. Neuroscience 12, 1125–1137. doi: 10.1016/0306-4522(84)90007-1
Perry, W., Minassian, A., Lopez, B., Maron, L., and Lincoln, A. (2007). Sensorimotor gating deficits in adults with autism. Biol. Psychiatry 61, 482–486. doi: 10.1016/j.biopsych.2005.09.025
Pessiglione, M., Guehl, D., Agid, Y., Hirsch, E. C., Féger, J., and Tremblay, L. (2003). Impairment of context-adapted movement selection in a primate model of presymptomatic Parkinson's disease. Brain 126, 1392–1408. doi: 10.1093/brain/awg139
Pessoa, L., and Adolphs, R. (2010). Emotion processing and the amygdala: from a ‘low road’ to ‘many roads’ of evaluating biological significance. Nat. Rev. Neurosci. 11, 773–783. doi: 10.1038/nrn2920
Phelps, E. A., Ling, S., and Carrasco, M. (2006). Emotion facilitates perception and potentiates the perceptual benefits of attention. Psychol. Sci. 17, 292–299. doi: 10.1111/j.1467-9280.2006.01701.x
Pierrot-Deseilligny, C., Rosa, A., Masmoudi, K., Rivaud, S., and Gaymard, B. (1991). Saccade deficits after a unilateral lesion affecting the superior colliculus. J. Neurol. Neurosurg. Psychiatry 54, 1106–1109. doi: 10.1136/jnnp.54.12.1106
Pieruccini-Faria, F., Jones, J. A., and Almeida, Q. J. (2014). Motor planning in Parkinson's disease patients experiencing freezing of gait: the influence of cognitive load when approaching obstacles. Brain Cogn. 87, 76–85. doi: 10.1016/j.bandc.2014.03.005
Pinkham, A. E., Griffin, M., Baron, R., Sasson, N. J., and Gur, R. C. (2010). The face in the crowd effect: anger superiority when using real faces and multiple identities. Emotion 10, 141–146. doi: 10.1037/a0017387
Posner, M. I., and Petersen, S. E. (1990). The attention system of the human brain. Annu. Rev. Neurosci. 13, 25–42. doi: 10.1146/annurev.ne.13.030190.000325
Posner, M. I., Walker, J. A., Friedrich, F. J., and Rafal, R. D. (1984). Effects of parietal injury on covert orienting of attention. J. Neuroci. 4, 1863–1874.
Povinelli, D. J., and Davis, D. R. (1994). Differences between chimpanzees (Pan troglodytes) and humans in the resting state of the index finger: implications for pointing. J. Comp. Psychol. 108, 134–139. doi: 10.1037/0735-7036.108.2.134
Preuss, T. M. (2007). “Evolutionary specializations of primate brain systems,” in Primate Origins and Adaptations, eds M. J. Ravoso and M. Dagosto (New York, NY: Kluwer Academic; Plenum Press), 625–675.
Previc, F. H. (1990). Functional specialization in the lower and upper visual fields in humans: its ecological origins and neurophysiological implications. Behav. Brain Sci. 13, 519–575. doi: 10.1017/S0140525X00080018
Rafal, R. D., Koller, K., Bultitude, J. H., Mullins, P., Ward, R., Mitchell, A. S., et al. (2015). Connectivity between the superior colliculus and the amygdala in humans and macaque monkeys: virtual dissection with probabilistic DTI tractography. J. Neurophysiol. 114, 1947–1962. doi: 10.1152/jn.01016.2014
Ramakrishnan, U., Coss, R. G., Schank, J., Dharawat, A., and Kim, S. (2005). Snake species discrimination by wild bonnet macaques (Macaca radiata). Ethology 111, 337–356. doi: 10.1111/j.1439-0310.2004.01063.x
Robinson, D. L. (1993). “Functional contributions of the primate pulvinar,” in Progress in Brain Research, Vol. 95, eds T. P. Hicks, S. Molotchinikoff, and T. Ono (Cambridge: Elsevier), 371–380.
Robinson, D. L., and Petersen, S. E. (1992). The pulvinar and visual salience. Trends Neurosci. 15, 127–132. doi: 10.1016/0166-2236(92)90354-B
Rodieck, R. W., and Watanabe, M. (1993). Survey of the morphology of macaque retinal ganglion cells that project to the pretectum, superior colliculus, and parvicellular laminae of the lateral geniculate nucleus. J. Comp. Neurol. 338, 289–303. doi: 10.1002/cne.903380211
Rodier, P. M. (2000). The early origins of autism. Sci. Am. 282, 56–63. doi: 10.1038/scientificamerican0200-56
Rosenhall, U., Johansson, E., and Gillberg, C. (1988). Oculomotor findings in autistic children. J. Laryngol. Otol. 102, 435–439. doi: 10.1017/S0022215100105286
Ross, C. F. (2000). Into the light: the origin of Anthropoidea. Annu. Rev. Anthropol. 29, 147–194. doi: 10.1146/annurev.anthro.29.1.147
Rossion, B., Hanseeuw, B., and Dricot, L. (2012). Defining face perception areas in the human brain: a large-scale factorial fMRI face localizer analysis. Brain Cogn. 79, 138–157. doi: 10.1016/j.bandc.2012.01.001
Rowles, M. E., Lipp, O. V., and Mallan, K. M. (2012). On the resistance to extinction of fear conditioned to angry faces. Psychophysiology 49, 375–380. doi: 10.1111/j.1469-8986.2011.01308.x
Saalmann, Y. B., and Kastner, S. (2009). Gain control in the visual thalamus during perception and cognition. Curr. Opin. Neurobiol. 19, 408–414. doi: 10.1016/j.conb.2009.05.007
Sacrey, L. A. R., Armstrong, V. L., Bryson, S. E., and Zwaigenbaum, L. (2014). Impairments to visual disengagement in autism spectrum disorder: a review of experimental studies from infancy to adulthood. Neurosci. Biobehav. Rev. 47, 559–577. doi: 10.1016/j.neubiorev.2014.10.011
Saletti, R. G., Maior, R. S., Hori, E., de Almeida, R. M., Nishijo, H., and Tomaz, C. (2014). Whole-body prepulse inhibition protocol to test sensorimotor gating mechanisms in monkeys. PLoS ONE 9:e105551. doi: 10.1371/journal.pone.0105551
Sanders, M. D., Warrington, E. K., Marshall, J., and Weiskrantz, L. (1974). “Blindsight”: vision in a field defect. Lancet 1, 707–708. doi: 10.1016/S0140-6736(74)92907-9
Schettino, L. F., Adamovich, S. V., Hening, W., Tunik, E., Sage, J., and Poizner, H. (2006). Hand preshaping in Parkinson's disease: effects of visual feedback and medication state. Exp. Brain Res. 168, 186–202. doi: 10.1007/s00221-005-0080-4
Schettino, L. F., Rajaraman, V., Jack, D., Adamovich, S. V., Sage, J., and Poizner, H. (2003). Deficits in the evolution of hand preshaping in Parkinson's disease. Neuropsychology 42, 82–94. doi: 10.1016/S0028-3932(03)00150-7
Schiller, P. H., Malpeli, J. G., and Schein, S. J. (1979). Composition of geniculostriate input ot superior colliculus of the rhesus monkey. J. Neurophysiol. 42, 1124–1133.
Schmahmann, J. D., and Pandya, D. N. (1990). Anatomical investigation of projections from thalamus to posterior parietal cortex in the rhesus monkey: a WGA-HRP and flourescent tracer study. J. Comp. Neurol. 295, 299–326. doi: 10.1002/cne.902950212
Schmidt, C., Plickert, S., Summers, D., and Zerr, I. (2010). Pulvinar sign in Wernicke's encephalopathy. CNS Spectr. 15, 215–218.
Schultz, R. T. (2005). Developmental deficits in social perception in autism: the role of the amygdala and fusiform face area. Int. J. Dev. Neurosci. 23, 125–141. doi: 10.1016/j.ijdevneu.2004.12.012
Scott, M. M., and Deneris, E. S. (2005). Making and breaking serotonin neurons and autism. Int. J. Dev. Neurosci. 23, 277–285. doi: 10.1016/j.ijdevneu.2004.05.012
Selemon, L. D., and Goldman-Rakic, P. S. (1988). Prefrontal and posterior parietal cortices in the rhesus monkey: evidence for a distributed neural network subserving spatially guided behavior. J. Neurosci. 8, 4049–4068.
Serences, J. T., and Yantis, S. (2006). Selective visual attention and perceptual coherence. Trends Cogn. Sci. 10, 38–45. doi: 10.1016/j.tics.2005.11.008
Sewards, T. V., and Sewards, M. A. (2002). Innate visual object recognition in vertebrates: some proposed pathways and mechanisms. Comp. Biochem. Physiol. A Mol. Integr. Physiol. 132, 861–891. doi: 10.1016/S1095-6433(02)00119-8
Seyfarth, R. M., Cheney, D. L., and Marler, P. (1980). Monkey responses to three different alarm calls: evidence for predator classification and semantic communication. Science 210, 801–803. doi: 10.1126/science.7433999
Shibasaki, M., and Kawai, N. (2009). Rapid detection of snakes by Japanese monkeys (Macaca fuscata): an evolutionarily predisposed visual system. J. Comp. Psychol. 123, 131–135. doi: 10.1037/a0015095
Shibata, T., Nishijo, H., Tamura, R., Miyamoto, K., Eifuku, S., Endo, S., et al. (2002). Generators of visual evoked potentials for faces and eyes in the human brain as determined by dipole localization. Brain Topogr. 5, 51–63. doi: 10.1023/A:1019944607316
Shipp, S. (2003). The functional logic of cortico-pulvinar connections. Philos. Trans. R. Soc. Lond. B Biol. Sci. 358, 1605–1624. doi: 10.1098/rstb.2002.1213
Snijders, A. H., Weerdesteyn, V., Hagen, Y. J., Duysens, J., Giladi, N., and Bloem, B. R. (2010). Obstacle avoidance to elicit freezing of gait during treadmill walking. Mov. Disor. 25, 57–63. doi: 10.1002/mds.22894
Snyder, L. H., Calton, J. L., Dickinson, A. R., and Lawrence, B. M. (2002). Eye-hand coordination: saccades are faster when accompanied by a coordinated arm movement. J. Neurophysiol. 87, 2279–2286.
Soares, S. C. (2012). The lurking snake in the grass: interference of snake stimuli in visually taxing conditions. Evol. Psychol. 10, 187–197. doi: 10.1177/147470491201000202
Soares, S. C., and Esteves, F. (2013). A glimpse of fear: fast detection of threatening targets in visual search with brief stimulus durations. Psych J. 2, 11–16. doi: 10.1002/pchj.18
Soares, S. C., Esteves, F., Lundqvist, D., and Öhman, A. (2009). Some animal specific fears are more specific than others: evidence from attention and emotion measures. Behav. Res. Ther. 47, 1032–1042. doi: 10.1016/j.brat.2009.07.022
Soares, S. C., Lindström, B., Esteves, F., and Öhman, A. (2014). The hidden snake in the grass: superior detection of snakes in challenging attentional conditions. PLoS ONE 9:e114724. doi: 10.1371/journal.pone.0114724
Solca, M., Guggisberg, A. G., Schnider, A., and Leemann, B. (2015). Facial blindsight. Front. Hum. Neurosci. 9:522. doi: 10.3389/fnhum.2015.00522
Sparks, D. L. (1986). Translations of sensory signals into commands for control of saccadic eye movements: role of primate superior colliculus. Physiol. Rev. 66, 118–171.
Sparks, D. L., and Mays, L. E. (1981). “The role of the monkey superior colliculus in the control of saccadic eye movements. A current perspective”, in Progress in Oculomotor Research, Vol. 12, eds A. F. Fuchs and W. Becker (Amsterdam: Elsevier; North Holland), 137–144.
Sprengelmeyer, R., Young, A. W., Hahn, K., Schroeder, U., Woitalla, D., Büttner, T., et al. (2003). Facial expression recognition in people with medicated and unmedicated Parkinson's disease. Neuropsychologia 41, 1047–1057. doi: 10.1016/S0028-3932(02)00295-6
Steen, C. J., Carbonaro, P. A., and Schwartz, R. A. (2004). Arthropods in dermatology. J. Amer. Acad. Dermatol. 50, 819–842l. doi: 10.1016/j.jaad.2003.12.019
Stein, T., Seymour, K., Hebart, M. N., and Sterzer, P. (2014). Rapid fear detection relies on high spatial frequencies. Psychol. Sci. 25, 566–574. doi: 10.1177/0956797613512509
Stepniewska, I. (2004). “The pulvinar complex,” in The Primate Visual System, eds J. H. Kaas and C. E. Collins (New York, NY: CRC Press), 53–80.
Stepniewska, I., Fang, P.-C., and Kaas, J. H. (2005). Microstimulation reveals specialized subregions for different complex movements in posterior parietal cortex of prosimian galagos. Proc. Natl. Acad. Sci. U.S.A. 102, 4878–4883. doi: 10.1073/pnas.0501048102
Stepniewska, I., Qi, H. X., and Kaas, J. H. (2000). Projections of the superior colliculus to subdivisions of the inferior pulvinar in New World and Old World monkeys. Vis. Neurosci. 17, 529–549. doi: 10.1017/S0952523800174048
Stoerig, P., and Cowey, A. (1997). Blindsight in man and monkey. Brain 120, 535–559. doi: 10.1093/brain/120.3.535
Stoerig, P., Kleinschmidt, A., and Frahm, J. (1997). No visual responses in denervated V1: high-resolution functional magnetic resonance imaging of a blindsight patient. Neuroreport 9, 21–25. doi: 10.1097/00001756-199801050-00005
Stone, J., and Johnston, E. (1981). The topography of primate retina: a study of the human, bushbaby, and New- and Old-World monkeys. J. Comp. Neurol. 196, 205–223. doi: 10.1002/cne.901960204
Stuphorn, V., Bauswein, E., and Hoffmann, K.-P. (2000). Neurons in the primate superior colliculus coding for arm movements in gaze-related coordinates. J. Neurophysiol. 83, 1283–1299.
Surridge, A. K., Osorio, D., and Mundy, N. I. (2003). Evolution and selection of trichromatic vision in primates. Trends Ecol. Evol. 18, 198–205. doi: 10.1016/S0169-5347(03)00012-0
Tamietto, M., Pullens, P., de Gelder, B., Weiskrantz, L., and Goebel, R. (2012). Subcortical connections to human amygdala and changes following destruction of the visual cortex. Curr. Biol. 22, 1449–1455. doi: 10.1016/j.cub.2012.06.006
Tamura, R., Kitamura, H., Endo, T., Hasegawa, N., and Someya, T. (2010). Reduced thalamic volume observed across different subgroups of autism spectrum disorders. Psychiatry Res. 184, 186–188. doi: 10.1016/j.pscychresns.2010.07.001
Tanaka, K., Saito, H., Fukada, Y., and Moriya, M. (1991). Coding visual images of objects in the inferotemporal cortex of the macaque monkey. J. Neurophysiol. 66, 170–189.
Tazumi, T., Hori, E., Maior, R. S., Ono, T., and Nishijo, H. (2010). Neural correlates to seen gaze-direction and orientation in the macaque monkey amygdala. Neuroscience 169, 287–301. doi: 10.1016/j.neuroscience.2010.04.028
Tomasello, M. (2000). Culture and cognitive development. Curr. Dir. Psychol. Sci. 9, 37–40. doi: 10.1111/1467-8721.00056
Tomasello, M., Carpenter, M., and Liszkowski, U. (2007). A new look at infant pointing. Child Dev. 78, 705–722. doi: 10.1111/j.1467-8624.2007.01025.x
Troiani, V., and Schultz, R. T. (2013). Amygdala, pulvinar, and inferior parietal cortex con- tribute to early processing of faces without awareness. Front. Hum. Neurosci. 7:241. doi: 10.3389/fnhum.2013.00241
Trojanowski, J. Q., and Jacobson, S. (1974). Medial pulvinar afferents to frontal eye fields in rhesus monkey demonstrated by horseradish peroxidase. Brain Res. 80, 395–411. doi: 10.1016/0006-8993(74)91025-7
Tsatsanis, K. D., Rourke, B. P., Klin, A., Volkmar, F. R., Cicchetti, D., and Schultz, R. T. (2003). Reduced thalamic volume in high-functioning individuals with autism. Biol. Psychiatry 53, 121–129. doi: 10.1016/S0006-3223(02)01530-5
Tsuchiya, N., and Koch, C. (2005). Continuous flash suppression reduces negative afterimages. Nat. Neurosci. 8, 1096–1101. doi: 10.1038/nn1500
Ungerleider, L. G., and Christensen, C. A. (1979). Pulvinar lesions in monkeys produce abnormal scanning of a complex visual array. Neurpsychologia 17, 493–501. doi: 10.1016/0028-3932(79)90056-3
Van Strien, J. W., Christiaans, G., Franken, I. H. A., and Huijding, J. (2016). Curvilinear shapes and the snake detection hypothesis: an ERP study. Psychophysiology 53, 252–257. doi: 10.1111/psyp.12564
Van Strien, J. W., Eijlers, R., Franken, I. H., and Huijding, J. (2014a). Snake pictures draw more early attention than spider pictures in non-phobic women: evidence from event-related brain potentials. Biol. Psychol. 96, 150–157. doi: 10.1016/j.biopsycho.2013.12.014
Van Strien, J. W., Franken, I. H., and Huijding, J. (2014b). Testing the snake-detection hypothesis: larger early posterior negativity in humans to pictures of snakes than to pictures of other reptiles, spiders and slugs. Front. Hum. Neurosci. 8:691. doi: 10.3389/fnhum.2014.00691
Vlamings, P. H., Jonkman, L. M., van Daalen, E., van der Gaag, R. J., and Kemner, C. (2010). Basic abnormalities in visual processing affect face processing at an early age in autism spectrum disorder. Biol. Psychiatry 68, 1107–1113. doi: 10.1016/j.biopsych.2010.06.024
Vuilleumier, P., Armoney, J. L., Driver, J., and Dolan, R. J. (2003). Distinct spatial frequency sensitivities for processing faces and emotional expressions. Nat. Neurosci. 6, 624–631. doi: 10.1038/nn1057
Weiskrantz, L. (1996). Blindsight revisited. Curr. Opin. Neurobiol. 6, 215–220. doi: 10.1016/S0959-4388(96)80075-4
Weiskrantz, L., Barbur, J. L., and Sahraie, A. (1995). Parameters affecting conscious versus unconscious visual discrimination with damage to the visual cortex (V1). Proc. Natl. Acad. Sci. U.S.A. 92, 6122–6126. doi: 10.1073/pnas.92.13.6122
Weiskrantz, L., Warrington, E. K., Sanders, M. D., and Marshall, J. (1974). Visual capacity in the hemianopic field following a restricted occipital ablation. Brain 97, 709–728. doi: 10.1093/brain/97.1.709
Werner, W. (1993). Neurons in the primate superior colliculus are active before and during arm movements to visual targets. Eur. J. Neurosci. 5, 335–340. doi: 10.1111/j.1460-9568.1993.tb00501.x
Wiener, S. G., and Levine, S. (1992). Behavioral and physiological responses of mother and infant squirrel monkeys to fearful stimuli. Dev. Psychobiol. 25, 127–136. doi: 10.1002/dev.420250205
Wilke, M., Turchi, J., Smith, K., Mishkin, M., and Leopold, D. A. (2010). Pulvinar inactivation disrups selection of movement plans. J. Neurosci. 30, 8650–8659. doi: 10.1523/JNEUROSCI.0953-10.2010
Williams, L. M., Liddell, B. J., Kemp, A. H., Bryant, R. A., Meares, R. A., Peduto, A. S., et al. (2006). Amygdala-prefrontal dissociation of subliminal and supraliminal fear. Hum. Brain. Mapp. 27, 652–661. doi: 10.1002/hbm.20208
Yang, E., Zald, D. H., and Blake, R. (2007). Fearful expressions gain preferential access to awareness during continuous flash suppression. Emotion 7, 882–886. doi: 10.1037/1528-3542.7.4.882
Yates, A., Ashwin, C., and Fox, E. (2010). Does emotion processing require attention? The effects of fear conditioning and perceptual load. Emotion 10, 822–830. doi: 10.1037/a0020325
Keywords: superior colliculus, pulvinar, snake detection theory, faces, primates, evolution
Citation: Soares SC, Maior RS, Isbell LA, Tomaz C and Nishijo H (2017) Fast Detector/First Responder: Interactions between the Superior Colliculus-Pulvinar Pathway and Stimuli Relevant to Primates. Front. Neurosci. 11:67. doi: 10.3389/fnins.2017.00067
Received: 30 September 2016; Accepted: 30 January 2017;
Published: 17 February 2017.
Edited by:
Xiaogang Wu, Institute for Systems Biology, USAReviewed by:
Elisabetta Ladavas, University of Bologna, ItalyCopyright © 2017 Soares, Maior, Isbell, Tomaz and Nishijo. This is an open-access article distributed under the terms of the Creative Commons Attribution License (CC BY). The use, distribution or reproduction in other forums is permitted, provided the original author(s) or licensor are credited and that the original publication in this journal is cited, in accordance with accepted academic practice. No use, distribution or reproduction is permitted which does not comply with these terms.
*Correspondence: Hisao Nishijo, bmlzaGlqb0BtZWQudS10b3lhbWEuYWMuanA=
†These authors have contributed equally to this work.
Disclaimer: All claims expressed in this article are solely those of the authors and do not necessarily represent those of their affiliated organizations, or those of the publisher, the editors and the reviewers. Any product that may be evaluated in this article or claim that may be made by its manufacturer is not guaranteed or endorsed by the publisher.
Research integrity at Frontiers
Learn more about the work of our research integrity team to safeguard the quality of each article we publish.