- School of Biological and Chemical Sciences, Queen Mary University of London, London, UK
The standard model of adrenocortical zonation holds that the three main zones, glomerulosa, fasciculata, and reticularis each have a distinct function, producing mineralocorticoids (in fact just aldosterone), glucocorticoids, and androgens respectively. Moreover, each zone has its specific mechanism of regulation, though ACTH has actions throughout. Finally, the cells of the cortex originate from a stem cell population in the outer cortex or capsule, and migrate centripetally, changing their phenotype as they progress through the zones. Recent progress in understanding the development of the gland and the distribution of steroidogenic enzymes, trophic hormone receptors, and other factors suggests that this model needs refinement. Firstly, proliferation can take place throughout the gland, and although the stem cells are certainly located in the periphery, zonal replenishment can take place within zones. Perhaps more importantly, neither the distribution of enzymes nor receptors suggest that the individual zones are necessarily autonomous in their production of steroid. This is particularly true of the glomerulosa, which does not seem to have the full suite of enzymes required for aldosterone biosynthesis. Nor, in the rat anyway, does it express MC2R to account for the response of aldosterone to ACTH. It is known that in development, recruitment of stem cells is stimulated by signals from within the glomerulosa. Furthermore, throughout the cortex local regulatory factors, including cytokines, catecholamines and the tissue renin-angiotensin system, modify and refine the effects of the systemic trophic factors. In these and other ways it more and more appears that the functions of the gland should be viewed as an integrated whole, greater than the sum of its component parts.
Introduction—The Standard Model
The nature and significance of the zonation of the mammalian adrenal cortex has attracted considerable interest during the fifteen decades following the first description of its three main zones, zona glomerulosa, zona fasciculata, and zona reticularis, by Arnold (1866). Later, Gottschau (1883) was the first to suggest that adrenocortical cells originate in the outer part of the gland, and migrate centripetally, thus becoming phenotypically glomerulosa, then fasciculata and reticularis in sequence. Zwemer et al. (1938) illustrated a direct lineage of cortical cells from the connective tissue capsule. Before the functions of the gland were understood, studies were directed to further descriptions of adrenocortical morphology and development, in mammals and other vertebrates (Dostoiewsky, 1886; Rabl, 1891; Wiesel, 1902). By the 1940s it was clear that the adrenal cortex, as well as the medulla, is crucially involved in the response to stress, and further, that secretions of the cortex fell into three functional classes: androgens, and what Selye and Jensen were the first to call mineralocorticoids and glucocorticoids (Selye, 1946; Selye and Jensen, 1946). Coupled with the observable changes in appearance and abundance of the zones in different physiological situations it then became apparent that the different zones had different functions, and androgens, mineralocorticoids, and glucocorticoids were now thought to be products of the zona reticularis, zona glomerulosa, and zona fasciculata respectively (Vines, 1938; Swann, 1940; Chester Jones, 1957; Deane, 1962). Moreover, their regulation was different, and though the inner adrenocortical zones, fasciculata and reticularis, were dependent on an intact pituitary and the secretion of ACTH, the glomerulosa was not (Chester Jones, 1957; Deane, 1962; Vinson, 2003). Later, the essential role of the renin-angiotensin system in the regulation of aldosterone was elucidated (Gross, 1958; Laragh et al., 1960; Carpenter et al., 1961), though other factors, possibly many, are also involved (Vinson et al., 1992b; Ehrhart-Bornstein et al., 1998; Mulrow, 1999; Vinson, 2003).
Together, such evidence has led to the development of what may be thought of as the Standard Model of adrenocortical zonation:
(i) Adrenocortical cells arise in the outer part of the gland and migrate centripetally, with changes in phenotype as they progress from the glomerulosa through the fasciculata to the reticularis, where they undergo apoptosis.
(ii) Each of the three main differentiated zones secretes a specific profile of steroid hormones.
(iii) Each of the zones is under separate and independent regulatory control.
There have, however, always been gaps in interpretation that have scarcely been addressed. Among these is the challenge of unraveling the mechanisms underlying the profound changes of phenotype that the cells undergo during their lifespan. Again, but less frequently considered, what drives cell migration in the mature gland? Additionally, and rarely addressed: what is the significance of the zonal arrangement—what advantage is conferred by the configuration of the different zones as concentric shells?
It is the purpose of this review to examine both whether the Standard Model remains compatible with the literature of the last few years, and also whether there are now clues to these additional problems.
What Do We Mean by Adrenocortical Zonation?
It is important to define the terms we use. Zonation is a descriptive term, which has been applied by different observers to mean sometimes quite different things. Without a strict, generally adhered-to definition, it is most appropriate to go back to the pioneers of the terminology, and follow their usage. Since the earliest descriptors of adrenocortical zonation were morphological and topographical, and these alone were used for 70–80 years, it follows that, without a contrary formal decision, agreed perhaps by a caucus of international exponents of the field, zonation remains defined by cellular morphology and topology alone (Figures 1, 2).
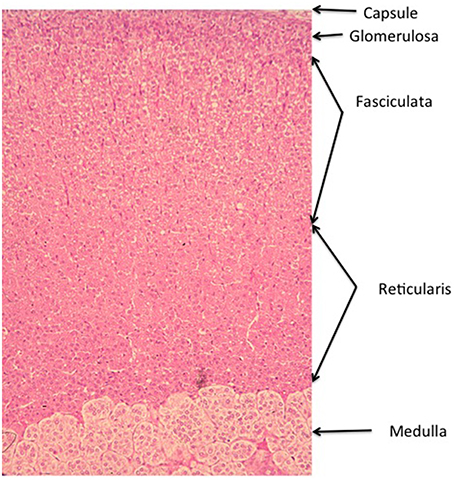
Figure 1. Zonation of the Wistar rat adrenal cortex. The main zones of the cortex. The arrangement of the concentric bands of cells is representative of most mammal species, though there is some species variation. In the adult human gland, the glomerulosa is sparse and confined to discrete islets, whereas in others, such as the dog, the glomerulosa is much more marked, with large whorls of cells justifying the comparison with the renal glomerulus. Additional zones may occur in other species, including the X zone in the mouse gland, and the fetal zone in the developing and newborn human gland (See Chester Jones, 1957; Deane, 1962; Neville and O'Hare, 1981; Vinson et al., 1992b).
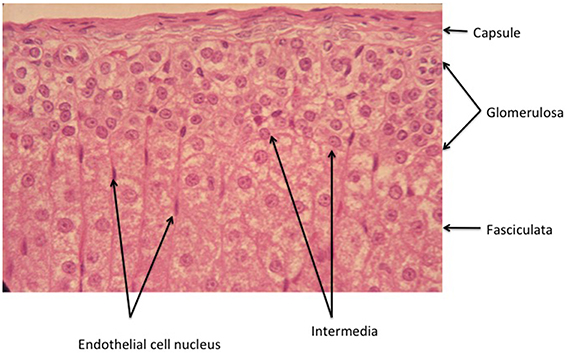
Figure 2. Higher power view of the capsule/glomerulosa/outer fasciculata view of the rat adrenal cortex. Note that in this tissue from an ~2 month old animal, the zona intermedia is very sparse. There is a marked differentiation between the glomerulosa and the fasciculata, emphasizing the precision with which the signals that determine positioning of the different cell types must operate (see text).
The names of the three main zones are descriptive of the arrangement of their cells. Thus, the zona glomerulosa is so called because the whorls or baskets of cells that compose it somewhat recall the glomeruli of the kidney (Latin glomus, ball) though this is more apparent in some species (e.g., the dog) than others (rat, human). The cells of the zona fasciculata lie in parallel radial cords, and the name stems originally from the Roman symbol of office, the fasces or bundle of birch rods (etymologically the same origin, incidentally, as fascist), cf. the anatomical term fascicle (bundle of nerves or muscle fibers), while the cords of cells of the reticularis form a network (Latin: rete). Essentially, the smaller, more densely staining glomerulosa cells are characterized by their lamelliform cristae, whereas the larger and paler fasciculata cells have tubulo-vesicular cristae. The reticularis cells retain tubulo-vesicular cristae, and are again more densely staining and smaller than fasciculata cells. Lipid distribution varies with the state of stimulation, but in general is greater in the inner zones. These criteria have been reviewed on many occasions (Chester Jones, 1957; Deane, 1962; Neville and O'Hare, 1981; Vinson et al., 1992b).
Thus, zonation is not described by function, nor by the presence of this or that steroid product, or molecular marker. As it happens, and as this article will make clear, function follows morphology/topology only partially. For example, in the rat adrenal, only outer zona glomerulosa cells express CYP11B2 (see Table 1 for abbreviations) in sodium sufficient animals, and the inner glomerulosa does not. The non-CYP11B2-containing glomerulosa region was first called the white or undifferentiated zone (ZU) (Mitani et al., 1994, 1995), while others have called it the “zona intermedia” (Engeland and Levay-Young, 1999; Ulrich-Lai et al., 2006). This is confusing, because the term zona intermedia was previously applied to a morphologically distinct flattened cell type, quite different from the glomerulosa, that is limited to a very few cells at the glomerulosa-fasciculata border (Cater and Lever, 1954; Chester Jones, 1957; Deane, 1962; Nussdorfer, 1986). Indeed Cater and Lever (Cater and Lever, 1954) report it as absent from 2 month old rat adrenals, while it is very marked in older animals. It is sparsely evident in the illustration in Figure 2. It should be noted that these descriptions apply to the rat adrenal, and the presence of a discrete ZU in other species is not clear (see below).
Similarly, others have interpreted, and used, CYP11B2 as a “highly specific zona glomerulosa marker” (Freedman et al., 2013). It isn't, since (as noted above) it is expressed by only a small population of glomerulosa cells.
In yet another re-writing of the literature on adrenocortical zonation, some authors appear to define the zona reticularis, not on the basis of its morphology, but as the site of DHEA and androstenedione production. Defined in this way, the rat adrenal, it is claimed, does not contain a zona reticularis (de Joussineau et al., 2012; Pihlajoki et al., 2015), when it quite obviously does!—see Figure 1—moreover reticularis cells have been isolated from rat adrenals, their steroidogenic potential analyzed, and even shown to produce androstenedione, albeit in small amounts (Bell et al., 1978, 1979), see also (Pignatelli et al., 2006).
Origin of the Adrenal Cortex, Cell Proliferation, and Migration
The adrenal cortex originates from a section of the coelomic epithelium, firstly as part of the SF1-expressing adrenogonadal primordium, then separating as the adrenal anlage into approximately its ultimate location, ventrolateral to the dorsal aorta. At this site, it is invaded by cells from the neural crest, and eventually becomes encapsulated as the discrete adrenal gland (Xing et al., 2015). An interplay between the transcription factors SF1 and Dax1 determines the extent to which steroidogenic enzymes are induced, and adrenocortical cells become differentiated: Dax 1, as a co-repressor of SF1, sustains the pluripotential stem cell-like phenotype (Xing et al., 2015).
In the established cortex, the central concept that there is a stem cell population located in the capsule or immediate subcapsular region (Zwemer et al., 1938; Lombardo and Cortesini, 1988) has largely been supported by evidence from several types of experiment that track the fate of labeled cells.
Earlier studies followed labeled cells after the pulse administration of tritiated thymidine in the rat (Wright, 1971; Wright et al., 1973; Zajicek et al., 1986), latterly BrdU was used in mice (Chang et al., 2013). These gave broadly similar results, showing initial labeling in the outer part of the gland, with centripetal migration and phenotype change at a rate of 13–20 μm per day in the mouse adrenal (Chang et al., 2013), reaching the medulla after 104 days from initial labeling in the rat gland (Zajicek et al., 1986). Others have used transgenic mice bearing the 21OH/LacZ gene (Morley et al., 1996) or other constructs (Walczak and Hammer, 2015). Using 21OH/LacZ transgenic mice gives typical results in that mice bearing this transgene develop stripes of beta-gal staining cells in the adrenal extending from the capsule to the medulla, consistent with the concept of an outer region of proliferation with subsequent inward migration. It has to be said that the staining is not consistent with the distribution of 21-hydroxylase in the normal mouse adrenal, which is present only as a trace in the glomerulosa, though strongly expressed in the adrenal inner zones (Chang et al., 2013). Similar radial distribution of labeled cells has shown such lineages in mice bearing for example shhgfpcre;R26-YFP cells: shh is highly transcribed in the outer cortex in the mouse (though only in the ZU in the rat), so this parallels the results obtained with 21OH/LacZ. A similar cell lineage is seen in mice with the Gli1-creT2 R26-X label: Gli1 is normally transcribed in the inner capsule in cells that apparently represent stem cells. Since the capsular expression of Gli1 is induced by shh signaling, this suggests what King and co-authors call a bootstrapping loop, by which the recruitment of new cortical cells from the capsule is stimulated, and both shh and Gli1 cells are transformed into other cell types throughout the cortex (King et al., 2009). In fact this process is reinforced by the glomerulosa specific Dlk1 (also known as Pref-1), a member of the Notch family, which is co-expressed with shh in the rat, and, like shh, also signals to the capsule, inducing Gli-1 transcription and cortical cell recruitment (Guasti et al., 2013b; Finco et al., 2015). In mouse adrenals regenerating after dexamethasone treatment, fasciculata cells were similarly shown to be formed directly from CYP11B2-Cre-bearing zona glomerulosa cells in an SF-1 dependent process (Freedman et al., 2013), although the possibility of an intermediate shh-expressing form does not seem to be excluded.
There is often some ambiguity in such studies in that although the Standard Model is supported, alternative lineages are not necessarily excluded. Thus, fasciculata cells may be formed in the mouse adrenal from sources independent of the glomerulosa (Freedman et al., 2013). That proliferation may occur at various sites in the gland (Payet et al., 1980; Kirillov and Popova, 1985; McEwan et al., 1996, 1999) does not of itself counter the view that the stem cells are capsular or subcapsular. However, there is some additional complexity revealed by the timed appearance of BrdU labeled cells following pulse labeling in the mouse adrenal cortex that suggests there may be variation in the fates of individual cells (Chang et al., 2013), for example, some cells may be retained in the glomerulosa. Perhaps this may be expected from the evidence of the expanded glomerulosa, and CYP11B2 expression, that results from a low sodium diet or angiotensin II treatment (McEwan et al., 1996, 1999). In control mice, Ki67 staining showed proliferation predominantly in the glomerulosa and also in the outer fasciculata, where it was significantly increased by a pulse of ACTH. These fasciculata cells also expressed 21-hydroxylase, whereas the BrdU and Ki67 stained glomerulosa cells were steroidogenically inert, confirming that proliferation occurs in differentiated as well as partially differentiated cells (Chang et al., 2013).
Another example of such ambiguity is found in rats, where the compensatory hypertrophy following unilateral adrenalectomy may depend on proliferation in the fasciculata (Engeland et al., 2005) thus 5 days after enucleation proliferation may occur in both the glomerulosa and the inner part of the regenerating fasciculata, and after 10–28 days in the outer fasciculata (Ennen et al., 2005). Different sources of cells may also arise in other experimental conditions, for example in the Prkar1a knockout mouse, there is a strong ACTH-independent centrifugal expansion of corticosterone secreting cells, apparently from the X-zone (Sahut-Barnola et al., 2010; de Joussineau et al., 2012). All these potential sites for proliferation and migration of cells are indicated in Figure 3.
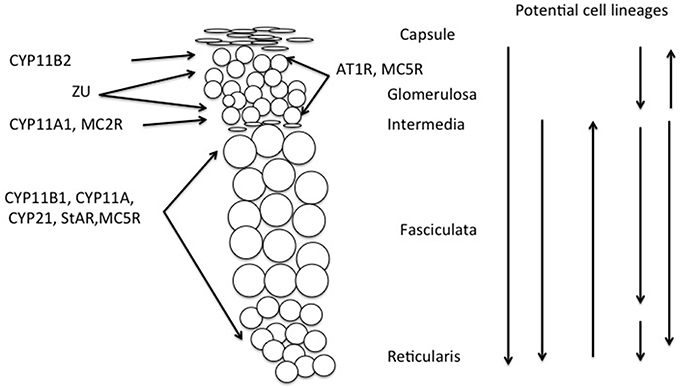
Figure 3. Schematic showing distribution of melanocortin receptors and steroidogenic enzymes in the outer adrenal cortex of the rat (cf. Figure 1). This illustrates that cellular interaction appears to be required to explain both the synthesis of aldosterone and its regulation by ACTH, thus requiring modification of the Standard Model of adrenocortical zonation. Vertical arrows indicate potential origins of proliferation and direction of migration of adrenocortical cells. Although the stem cell population is usually held to be in the capsular/subcapsular region, evidence for all of these possible sites for subsequent proliferation has been reported, (see text and Kim and Hammer, 2007; Chang et al., 2013; Freedman et al., 2013).
The assumption that, since adrenocortical cells originate in the outer part of the gland, and migrate centripetally, apoptosis is essentially a reticularis event, also requires re-examination, and it now appears that apoptosis occurs throughout all three zones in humans (Wolkersdorfer and Bornstein, 1998), and in the rat (Petrovic-Kosanovic et al., 2013). Like the cortex-wide proliferation that has been described, this presumably confers flexible tissue remodeling at all levels in the gland. ACTH inhibits apoptosis, thus stabilizing cortical structure in vivo (Carsia et al., 1996; Thomas et al., 2004), and in vitro according to some authors (Carsia et al., 1996) although not according to others, who describe ACTH-induced apoptosis in cultured rat cells (Mattos et al., 2011).
Maintenance of Zonal Differentiation
Here zonal differentiation implies the anatomical and functional features that distinguish the cells of the different zones. As emphasized above, and will be seen in Table 2, functional differences are not always definitive, many properties may be shared. Nevertheless, it is remarkable how the differentiation between glomerulosa and fasciculata, for example, is maintained (cf. Figures 1, 2). The selection and maintenance of cellular phenotype clearly depends on location within the gland. Thus, the three adrenocortical zones always occupy the same relative position, glomerulosa outermost, reticularis innermost, adjacent to the medulla, and the fasciculata between. How can this be achieved? As discussed further below, differentiated zonal function is maintained by systemic factors, including (in experimental animals at least) angiotensin II, potassium ions and α-MSH in the glomerulosa, and ACTH in the fasciculata and reticularis. At the same time these activators are clearly not the originating stimuli for differentiation since their receptors are already zonally distributed in order for them to have a zonally specific response—they therefore reinforce the actions of existing morphogens. An example of how this can be disturbed is given by experiments in which the disruption of the tandem potassium channels TASK1 and TASK3 gives rise to extended expression of CYP11B2 in the mouse fasciculata, resulting in aldosterone secretion that is insensitive to sodium intake, though sensitive to ACTH regulation. This is only true of females, so this unprecedented chaotically unzoned hypersecretion of aldosterone is remediable by testosterone treatment (Heitzmann et al., 2008). In contrast to the action of testosterone, which tends to restore the normal zonal, i.e., exclusively glomerulosa, distribution of CYP11B2, inactivation of the Cav2.3 calcium channel in TASK1−∕− mice reduces aldosterone to normal levels, but leaves CYP11B2 in the fasciculata (El Wakil, 2013).
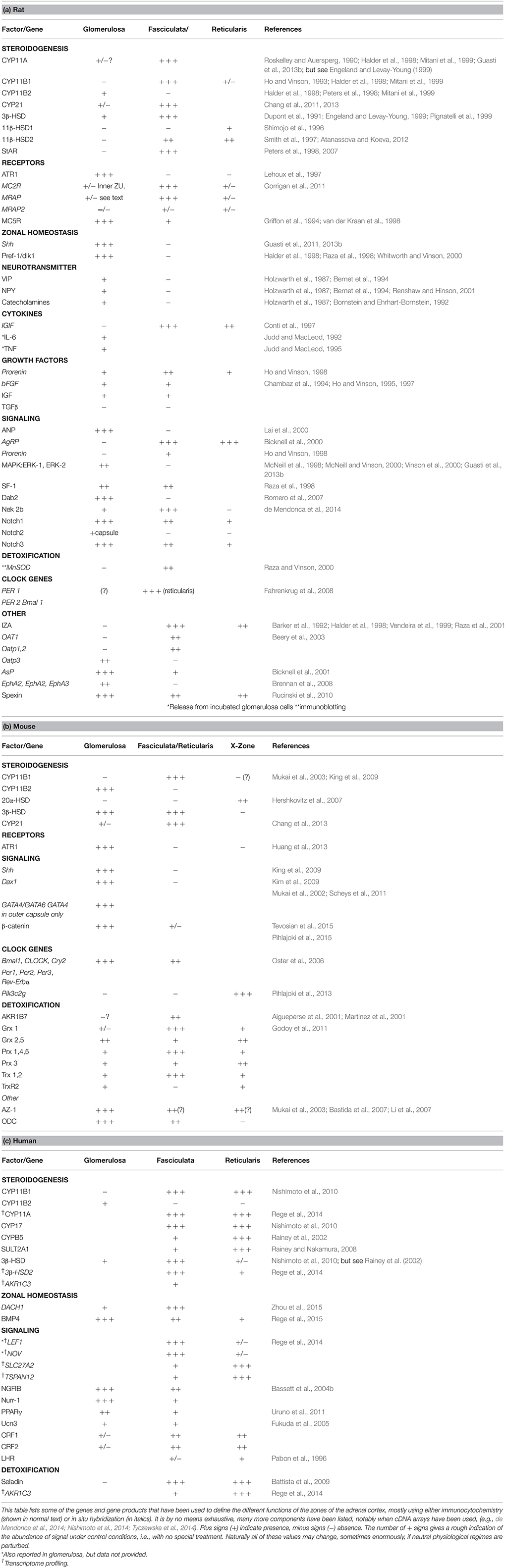
Table 2. Characteristics of zonal function in (a) rat (b) mouse, and (c) human adult adrenal glands.
So, if systemic factors cannot be responsible for localization of cell type and the organization of the zones, these must instead depend on factors within the gland. It may be speculated what such factors might be. We have previously considered these to be of three types, morphogens or paracrine agents produced within the gland, growth factors that essentially amplify the morphogenic signal, and transcription factors (Vinson and Ho, 1998; Vinson, 2003). In essence this is illustrated by the action of shh in upregulating Gli1, or the Wnt/catenin systems, described more fully below.
The concept that morphogens, secreted by a fixed source, diffuse along a concentration gradient to inform more or less remote cells of their position, and trigger appropriate responses, is sometimes difficult to envisage, because of the randomness of diffusion, and its susceptibility to local variation due for example to tissue inhomogeneity (Lander, 2013), and, in the case of the adrenal, also because of the relatively massive centripetal blood flow (Vinson et al., 1985b; Vinson and Hinson, 1992). The actions of morphogen gradients have been most widely studied in the context of limb development and regeneration in mice and other vertebrates. In mice, for example, bone morphogenetic proteins, BMPs, which are closely related to the TGF family, stimulate local growth and regeneration (Urist, 1965; Reddi, 1998; Bandyopadhyay et al., 2006; Tsuji et al., 2006; Yu et al., 2012) and have been primarily recognized for their roles in stimulating chondrogenesis and osteogenesis. However, they are also important in other tissues including the prostate (Thomas et al., 1998) breast and prostate cancer (Buijs et al., 2010), and in the pituitary and other components of the reproductive system (Shimasaki et al., 2004). Here wnt and shh signaling is crucial, as in the early development of the adrenal (below). In the limb, induction of shh is brought about by retinoic acid, which generates positional information at various stages in development (Tickle, 2006). This is achieved with a robustness that is remarkable, given the inherent variation in retinoic acid availability, depending as it does on dietary sources. But retinoic acid gradients may not depend solely on release and diffusion, but additionally on sites of degradation, as in the zebrafish brain (White et al., 2007; Schilling et al., 2012). It is possible that this applies to other morphogens, which could be critical when not only morphogen concentration but the duration of target cell exposure is critical, as in the mouse limb response to shh (Tickle, 2006). Crucially, it is thought that sharp boundaries between different cell types, such as found in the adrenal cortex, can still be achieved in the presence of a fluctuating and shallow morphogen gradient, by means of various mechanisms that depend in part on cell plasticity and in part on intercellular signaling. In other systems, these may include for example the retinoic acid-sensitive autoactivating genes Krox 20 and Hoxb1 that are mutually inhibitory in the zebrafish hindbrain (Zhang et al., 2012). Other mechanisms of noise-induced switching have been described (Schilling et al., 2012). Nevertheless, interfering with gap junctions affects positional clarity (Tickle, 2006), so direct cell-cell signaling is also involved (Lander, 2013).
With regard to the adrenal, it is clear that the same morphogens may play a role as in vertebrate limb regeneration, notably Wnt, Shh and BMPs. BMPs are expressed in all zones of the human adrenal cortex, but particularly the glomerulosa (see Table 2). Additionally, in H295R cells, BMP4 suppresses CYP17 RNA and protein, and DHEA secretion (Rege et al., 2015). In contrast, overexpression of the transcription factor DACH1 activates TGFβ and canonical Wnt signaling pathways, thereby suppressing CYP11B2 and aldosterone secretion (Zhou et al., 2015). Considerable advances in recent years have highlighted the particular importance of the Wnt/catenin system (MacDonald et al., 2009). Secreted Wnt glycoproteins bind to GPCRs of the frizzled family, bringing about increased cell content of the coactivator β-catenin, now known to be essential for the differentiation of SF1 positive adrenocortical cells from the adrenal capsule, and for their proliferation (Kim et al., 2008, 2009). The Wnt/catenin signaling system is involved in adrenocortical development in other ways also, by interaction with the nuclear receptor Dax1 and inhibin, both of which modulate adrenal/gonadal selection. Dax1is induced by SF1, but inhibits SF1 stimulated gene transcription (Ito et al., 1997; Yu et al., 1998). DAX1 is prominent in the subcapsular region in the mouse adrenal (but completely absent from the X-zone) (Mukai et al., 2002), together with β-catenin (Pihlajoki et al., 2015). Inhibin-α, which suppresses gonadal phenotype, is induced by SF1 and β-catenin (Gummow et al., 2003). The overall outcome of these effects in combination, is both to establish the adrenocortical fate of the subcapsular cells, while maintaining their further developmental plasticity (Wood and Hammer, 2010; Simon and Hammer, 2012). This, in effect, defines the glomerulosa (Berthon et al., 2012). SF1 is absolutely required for the development of the gland, and for the cell redifferentiation associated with centripetal migration (Freedman et al., 2013).
The search for stimulators of migration has however so far produced meager results. It is possible that the extracellular matrix may be involved: in bovine adrenal cells, laminin secretion is regulated by ACTH, and, in Boyden chamber experiments, laminin acted as a chemoattractant in promoting adrenal cell migration. However, the distribution of laminin is uniform throughout the cortex, and therefore seems unlikely to promote adrenocortical cell cellular migration (Pellerin et al., 1997). Other extracellular matrix proteins, including fibronectin and collagens I and IV, have been shown to affect steroidogenic enzyme expression in rat adrenal cells in culture, and in particular to modulate basal and ACTH stimulated steroid secretion (Otis et al., 2007). Additionally, rat glomerulosa cells secrete fibronectin, which promotes proliferation, while angiotensin II interferes with this process at the level of integrin binding, thus inhibiting proliferation while enhancing steroidogenesis (Otis et al., 2008).
Equally, how the inward migration of cells is achieved while the position of the zones relative to each other remains constant also continues to be a matter of some mystery. It is possible to speculate that various mechanisms might play a role. It is entirely possible that structures within the gland external to the adrenocortical cells themselves provide positional information. Such structures could include the neural network or elements of the vasculature, which though not necessarily visible in conventional microscopy are nevertheless important components both of which affect adrenocortical function (Figure 4; Vinson and Hinson, 1992; Vinson et al., 1994) and may be independent of ACTH (Bocian-Sobkowska et al., 1997). Alternatively, the relative positions of the capsule, external to the cortex, and of the inner medulla might suggest that a morphogenetic landscape could be generated by signals from these two poles. Certainly medullary products can affect inner cortical function (Ehrhart-Bornstein et al., 2000; Haidan et al., 2000). Or, another possibility is that positional information is provided by the adrenocortical cells themselves. Cortical cells, arranged as they are in cords traversing the cortex, themselves provide a polarity, indeed each cell may have an apical and a basal pole. Clearly this is true at least of some of them: cells of the outermost glomerulosa layer (which express both CYP11B2, AT1R, and MC5R) about the capsule at one pole, and other, perhaps less differentiated cells at the base. The innermost glomerulosa cells in the ZU abut either true zona intermedia or outer fasciculata cells, while the innermost reticularis cells lie directly adjacent to the medulla in the rat: in other species, such as the postpubertal male mouse there may be a band of connective tissue around the medulla. Such cell-cell contact is known to be important in positional information in other tissues (Tickle, 2006; Lander, 2013). The Eph/ephrin forward-reverse signaling system provides a possible mechanism for such cellular orientation and migration within the zones, and elements of the system have been demonstrated in the rat adrenal cortex. In particular, EphA2 is strongly transcribed in the rat glomerulosa, and responds to the physiological stimuli of a low sodium diet, captopril, or betamethasone treatment in a manner that suggests association with cell phenotype (Brennan et al., 2008).
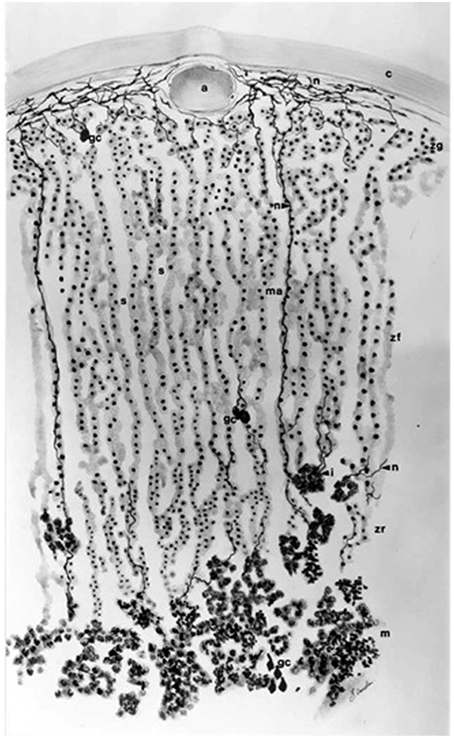
Figure 4. General arrangement of the vasculature and innervation of the rat adrenal cortex. Most nerves are located in the capsular (c) and subcapsular region, with arborization around the vasculature, including arterioles (a) and around the cells of the glomerulosa (zg). Nerve fibers are rare in the fasciculata (zf), but some traverse the width of the zone, often associated with medullary arteries (ma, 6–8 per gland), which specifically supply the medulla. Most of the blood reaching the medulla passes centripetally through the sinuses, which, in contrast to the thicker walled medullary arteries, are bordered by a single layer of very attenuated endothelial cells (cf. Figure 2). Near the medulla (m) a few short nerve fibers reach the reticularis (zr) from islets of chromaffin cells (i) in the inner cortex and from the medulla itself. There is now considerable evidence that products from both neural tissue and vasculature can affect corticosteroid secretion. They may also provide positional information for migrating and transforming cortical cells (see text). Drawing by Bridget Landon. Reproduced with permission from Vinson et al. (1994).
Functional Zonation of the Adrenal Cortex
A summary of some of the components expressed in the zones of the rat mouse and human cortex is given in Table 2. The Standard Model of adrenocortical zonation, as summarized in the Introduction, interprets functional zonation in terms of steroid hormone output alone. It is quite evident that in fact many other adrenocortical cell products are zone specific, and functional zonation is not just about steroids. Many of these other zonally organized functions, such as receptors and signaling pathways that transduce trophic hormone action, can be considered to complement the steroidogenic function. Others, such as clock genes, or enzymes metabolizing xenobiotics, may not be quite so easily accommodated in this way.
Although the same corticosteroids, cortisol, corticosterone, and aldosterone (though not usually all three) appear throughout the non-mammalian vertebrates, functional zonation of the adrenal cortex is a universal feature in mammals alone. While the appearance of the zones in different mammalian species may vary, aspects of their function do not. Thus, it is clear that the CYP11B2 catalyzed production of aldosterone is invariably limited to a population of cells in the zona glomerulosa, and CYP11B1 generates cortisol (or corticosterone in rats, mice, and some other species) predominantly in the fasciculata, and to a lesser extent, in the reticularis. Though this is consistent with the Standard Model, it is nevertheless now clear that some qualification is required.
The qualification arises because the extent of de novo steroidogenesis that can be unequivocally associated with each zone is limited. The human adrenal, for example, is complex. Here the well-defined CYP11B2-expressing glomerulosa seen in young individuals gives way in advancing years to a sparse glomerulosa generally confined to scattered islets (Neville and O'Hare, 1981; Aiba and Fujibayashi, 2011), interspersed by cells that may be analogous to those of the rat ZU [and are called the “progenitor zone” by Aiba and Fujibayashi (2011)], but which express 3β-HSD. Later, CYP11B2 expressing cell clusters (APCC) develop at the glomerulosa/fasciculata border, and extend into the fasciculata (Aiba and Fujibayashi, 2011; Nishimoto et al., 2015): these appear from the age of about 30 years. These additional sites of aldosterone production, arising as a consequence of somatic mutation of ion channels, are common in normal subjects, but the authors hypothesize that they predispose to primary aldosteronism (Nishimoto et al., 2015).
In the rat zona glomerulosa, starkly, of the steroidogenic enzymes only CYP11B2 is at all strongly expressed, and that only in the outermost glomerulosa: other required components for complete steroidogenesis, including CYP11A and CYP21 are barely detectable (Table 2), as is StAR according to some authors (Peters et al., 1998, 2007), but not others (Lehoux et al., 1998; Tyczewska et al., 2014). 3β-HSD appears to be present in all zones in the rat, as it is in the mouse (Table 2) though it is not expressed in the glomerulosa of the bovine adrenal (Ishimura and Fujita, 1997) while in humans it is relatively sparse in the glomerulosa according to some authors (Nishimoto et al., 2010), but not others (Rainey et al., 2002). Guasti et al. (2013a) located CYP11A in the inner undifferentiated zone (ZU) of the rat adrenal zona glomerulosa, quite clearly—indeed by definition—not a site of CYP11B2 expression, which, as noted, is normally confined to a very few cells in the outer glomerulosa. Notably, this site of CYP11A expression coincides with an intense signal for MC2R mRNA, otherwise apparently lacking in the rat glomerulosa (Gorrigan et al., 2011). The intensity of MC2R mRNA staining in this region is not inconsistent with the observations of Gallo-Payet and Escher (1985) who found greater ACTH binding in the glomerulosa than other zones, but who did not distinguish between CYP11B2 expressing and non-expressing glomerulosa cells. Also contrary to expectation, StAR and CYP11A are not always co-expressed. Thus not only does the inner ZU site of CYP11A not coincide with CYP11B2, with the caveats above in mind, it does not here coincide with StAR either: Peters and colleagues have consistently demonstrated StAR expression throughout the fasciculata, but, in untreated animals, hardly at all in any of the morphologically distinct smaller glomerulosa cells in the rat, including the ZU (Peters et al., 1998, 2007).
SF1 though is very definitely present throughout the adrenal cortex (Morohashi et al., 1994; Raza et al., 1998). Although SF1 is absolutely required for the development of the gland and its steroidogenic capacity (Lala et al., 1992; Parker and Schimmer, 1993; Parker et al., 1995; Freedman et al., 2013), its role must be very different in the cells of the different zones. Thus, although exposure to high SF1 inhibits CYP11B2 expression (Ye et al., 2009), the presence of SF1 in the glomerulosa is required not only for functional CYP11B2, but also for the glomerulosa-fasciculata cell type transformation that is a feature of adrenal cell centripetal migration and redifferentiation (Freedman et al., 2013).
In contrast to the glomerulosa, all fasciculata cells strongly express all of the enzymes required for glucocorticoid production enzymes, CYP11A1, CYP21, CYP11B1, 3β-HSD, and StAR, together with, in human (and other species') glands, CYP17 as well. These components are also present in the reticularis though (with the possible exception of CYP17) in lower abundance.
This then raises the question: how is aldosterone produced in the rat and human gland, if the entire required suite of enzymes and StAR are not co-expressed? Twenty years ago, we suggested that (at least) two cell types are required for aldosterone synthesis and secretion in the rat adrenal (Vinson et al., 1995; Vinson, 2004). The evidence for this is primarily that in the rat the efficient production of aldosterone in vitro requires an intact gland, and as it is disrupted, from whole tissue, first to separated zones, then to enzyme dispersed cells, aldosterone yields in vitro are progressively diminished, whereas corticosterone output is unchanged, and 18-OH-DOC, a major product of the fasciculata in the rat (Tait et al., 1970; Nonaka and Okamoto, 1991; Okamoto and Nonaka, 1992), is increased (Vinson et al., 1985a). Together with other evidence this suggested 18-OH-DOC might be an aldosterone precursor in this species (Vinson et al., 1992a, 1995; Vinson, 2004). Objections may be raised on the basis that the hemiketal structure of 18-OH-DOC is stable, and not easily converted, that the blood flow is centripetal, hence precluding steroid movement from inner zone to the glomerulosa, and that conversion of exogenous precursors is invariably inefficient. However, tracer 18-OH-DOC is certainly converted to aldosterone by rat glomerulosa tissue, and is sensitive to sodium status (Fattah et al., 1977). How it could reach the glomerulosa in vivo remains open to speculation, but if we accept that shh and wnt diffuse centrifugally (see above), there seems no reason why other substances should not. Or there may be cell-cell transport: it is not the proteolytic enzmes used in cell dispersal methods that diminishes aldosterone output, but the physical disruption of the capsular tissue (Raven et al., 1983). Finally, in the rat 18-OH-DOC circulates at concentrations sufficient to be utilized as substrate (Vinson, 2004).
It is not suggested that the same mechanism would necessarily operate in any species other than the rat, which seems to be unique in that 18-OH-DOC is a major product of the fasciculata. Nevertheless, in this connection it is intriguing that in the human gland there exist more obvious mechanisms for the transfer of steroid substrate or other products from the inner zones to the glomerulosa. First, there are arteriovenous loops which derive from the cortical arterioles in the capsule region, pass through the glomerulosa and fasciculata, but then loop back to the outer cortex to a site near their origin. Secondly, as the adrenal vein leaves the gland, the cortex is inverted and wraps around the vein as the cortical cuff, which retains its zonation, (though inverted). Here the blood supply is from the arteriae comitantes in the wall of the vein. Drainage from this region is through vessels from the medulla through the cortex (i.e., from reticularis to the fasciculata and glomerulosa), thence to the central vein (Figure 5) (Dobbie and Symington, 1966; Neville and O'Hare, 1981). Finally, in older subjects, CYP11B2 expressing aldosterone producing cell clusters project into the fasciculata (Nishimoto et al., 2015), providing the possibility of much more intimate contact between the different cell types.
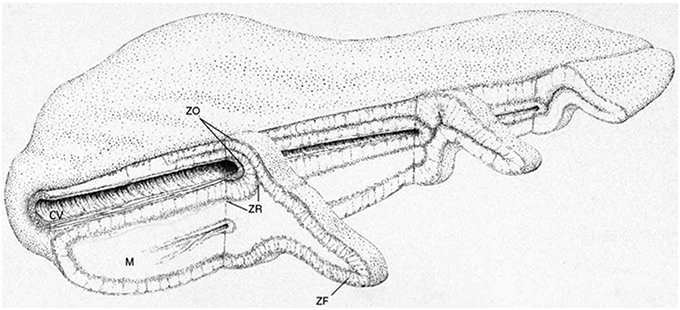
Figure 5. General structure of the human adrenal gland showing the cortical cuff. The inward folding adjacent to the central vein results in a doubling of the cortical thickness and the juxtaposition in this region of the inner zona glomerulosa and the central vein. C, cortex; M, medulla; ZG, zona glomerulosa; ZF, zona fasciculata; ZR, zona reticularis; CV, central vein. Drawing by B. Landon. Figure reproduced with permission from Vinson and Hinson (1992).
A more specific function for the reticularis has sometimes seemed to be less clear cut, especially in those species in which 17-hydroxylase (CYP17) is sparse, but in the human, and some other species, it is the major source of C19 steroids, especially dehydroepiandrosterone, both as the free steroid and as the sulfate. This general concept has been strengthened by methods developed to separate individual cell types, and assess their steroid output in vitro, and most recently by microdissection and transcriptome profiling (see Table 2). Notably, these methods have shown that CYP11A1 (side chain cleavage) and CYP17 (17-hydroxylase) gene transcripts are similar in the human fasciculata and reticularis, but Cyt B5A, sulfotransferase SULT2A1, and 17β-HSD (aldo-ketose reductase AKR1C3) are significantly more prominent in the reticularis (Rainey et al., 2002; Rege et al., 2014). The combined activity of these components accounts for the characteristic secretion of large amounts of DHEAS by the reticularis: Cyt B5 has been recognized as an important regulator of CYP17, and its presence is required for the efficient side chain cleavage activity of CYP17, though not for its 17α-hydroxylase function (Hall, 1991; Lee-Robichaud et al., 1995; Soucy and Luu-The, 2002). Cyt B5 acts through allosteric action rather than (as originally thought) provision of the second electron required for side chain cleavage (Auchus et al., 1998; Akhtar et al., 2005). It is the heightened secretion of DHEA and DHEAS by the reticularis that incurs the onset of adrenarche in humans (Belgorosky et al., 2008).
How then is androstenedione, the second most abundant androgen secreted by the human adrenal, actually synthesized? As we now see, in the human gland, the fasciculata cell expresses 3β-HSD, but the reticularis only a trace. On the other hand, the fasciculata expresses relatively little Cyt B5, required for C21 steroid side chain cleavage, whereas the reticularis expresses a lot. Perhaps interaction between the cell types, as postulated for aldosterone biosynthesis above, provides an answer, with transfer of DHEA from the reticularis to the fasciculata for the final stage of 3β-HSD catalyzed dehydrogenation/isomerization to give the Δ4-3-ketone configuration of androstenedione.
Zonation in the adrenal cortex of the mouse is more complex than the broad divisions into glomerulosa, fasciculata, and reticularis, usually taken as the mammalian norm, because of the additional presence of an inner zone, the X-zone, that is only apparent in the prepubertal animals of both sexes, and in the nulliparous adult female. It does not appear to be responsive to ACTH, and it has been suggested that it is supported by LH (Chester Jones, 1955). It involutes at puberty in the male mouse, and at first pregnancy in the female leaving a band of connective tissue between the reticularis and the medulla. Its involution is exquisitely sensitive to testosterone, and although from more recent studies it appears that involution precedes the increase in blood testosterone associated with puberty in the male (Hershkovitz et al., 2007), involution does not occur in castrates, and castration of adult males results in the development of a secondary X-zone lying outside the connective tissue residue of the original (Chester Jones, 1955, 1957). The function of the X-zone has remained largely obscure, though it is known to express 20α-hydroxysteroid dehydrogenase, but not 3β-hydroxysteroid dehydrogenase, which occurs widely throughout the rest of the cortex (Hershkovitz et al., 2007). To some extent the lack of data regarding this zone is compounded by the wide variation in its appearance, indeed in some inbred laboratory animals, notably the DDD strain, the extent of X-zone vacuolation suggests the presence of little functional tissue at all (Suto, 2012).
Other components that have a clear zonal distribution are enzymes that are thought to protect the gland against toxic products associated with a high degree of cytochrome P450 activity. Thus the aldo-keto reductase-like enzyme, AKR1B7 disposes of isocaproic aldehyde, the byproduct of CYP11A1 mediated cholesterol side chain cleavage, and manganese superoxide dismutase may protect against free radicals arising from leaky mitochondrial cytochrome P450 activity, Both of these enzymes are expressed in inner adrenocortical zones (in mouse and rat respectively) (Sahut-Barnola et al., 2000; Martinez et al., 2001; Raza et al., 2005), but not in the glomerulosa. In this context, seladin-1 (DHCR24), highly expressed in human and rat fasciculata cells, may be involved in oxidative stress management, and use of a specific seladin-1 inhibitor enhances ROS formation in response to ACTH (Battista et al., 2009). It may also be involved in specific regulation of DHEA secretion (see below).
The multifunctional protein that we originally called the Inner Zone Antigen (IZA) because it is present in the rat adrenocortical fasciculata and reticularis but not glomerulosa (Laird et al., 1988; Barker et al., 1992) was later identified with the so-called progesterone receptor membrane component 1 (PGRMC1) (Min et al., 2004; Cahill, 2007), and is now also designated as the sigma 2 receptor (Xu et al., 2011; Zeng et al., 2016). In the rat adrenal this protein specifically supports 21-hydroxylation (Laird et al., 1988; Min et al., 2005). The inner zone distribution of all three of these components, AKR1B7, MnSOD, IZA/PGRMC1/Sigma2, and their absence from the glomerulosa is entirely consistent with the view that the glomerulosa is not a significant site of de novo steroidogenesis.
Another metabolic function of the adrenal has been described that has a distinct zonal distribution, this is the capacity for xenobiotic metabolism conferred by CYP2D16, a member of the CYP2D cytochrome P450 subfamily, in the reticularis of the guinea pig (Martin and Black, 1983; Black et al., 1989; Jiang et al., 1996; Yuan et al., 1998, 2001). CYP2D16 increases with age, and is more abundant in males than in females, there are also strain differences (Huang et al., 1996). In contrast to CYP17, which is enhanced, CYP2D16 is diminished by ACTH treatment (Yuan et al., 1998), as is 21-hydroxylase in the reticularis, but not in the outer zones (Colby et al., 1992). It is also bimodally distributed in that it is abundant in the majority of individual animals, but low in others (Colby et al., 2000). CYP2D isoforms themselves have 21-hydroxylase activity however, and are responsible for this function in the rat brain (Kishimoto et al., 2004). Type 5 17β-HSD, another member of the aldo-keto reductase family, also designated AKR1C3, is prominently transcribed in the human reticularis (Rege et al., 2014). Its function, presumably, is the reduction of 17-ketosteroids, such as androstenedione to testosterone (Nakamura et al., 2009). Conceivably it may also be protective, perhaps, like CYP2D16, metabolizing xenobiotics or (like AKR1B7) metabolizing isocaproic aldehyde. Interestingly, in other cell types, AKR1C3 also suppresses cell differentiation (Desmond et al., 2003).
Also not directly part of the corticosteroid synthesizing apparatus are the clock genes, which are zonally distributed, though quite differently, in rat and mouse adrenals. Thus Per 1, Per 2, and Bmal 1 are most strongly expressed in the reticularis in rats, but, together with other clock genes (see Table 2) in glomerulosa and fasciculata in mice. Their expression depends on clock time, and is instrumental in maintaining the circadian rhythm inherent in corticosteroid secretion, in conjunction with hypothalamic/pituitary input (and in turn its regulation by the SCN clock system) and SCN regulated autonomic innervation (Ishida et al., 2005; Chung S. et al., 2011; Leliavski et al., 2015).
The secretion of endogenous ouabain is also zonal, in that bovine glomerulosa cells secrete several times as much as fasciculata cells, both under control conditions, and also when stimulated by angiotensin II or ACTH (Laredo et al., 1995). The presence of signaling molecules NOV, SCL27A2, and TSPAN12, not previously associated with adrenal function, and their differential transcription in the zones of the human adrenal cortex (Rege et al., 2014; see Table 2) also add to the view that our understanding of zonation is far from complete.
Regulation of Adrenocortical Zonation
Trophic Regulation of Zonal Function
In part, the maintenance of the differentiated cortex follows the activation of specific signaling pathways. Glomerulosa steroidogenic function, that is the expression of CYP11B2 and the final stage of aldosterone synthesis, for example, is induced by (among other factors) angiotensin II stimulation, via angiotensin type 1 receptors (ATR1a and b) resulting in the activation of calcium mediated signaling pathways, whereas the fasciculata, CYP11B1 expression and cortisol/corticosterone production are ACTH dependent, modulated by the melanocortin type 2 receptor (MC2R) acting through cAMP formation.
Regulation of the Glomerulosa
Angiotensin II, the potassium ion and ACTH are the most widely studied regulators of aldosterone secretion and glomerulosa function. They have both acute effects and longer term trophic actions.
There is ample evidence that angiotensin II supports glomerulosa structure and aldosterone secretion in a manner which parallels that of dietary sodium restriction: this topic has been reviewed on many occasions, and need not be repeated here (Mulrow, 1999; Hattangady et al., 2012; Bollag, 2014). The potassium ion also has direct stimulatory actions, both on acute secretion of aldosterone (Haning et al., 1970; Aguilera and Catt, 1978), and, longer term, in induction of CYP11B2 (Tremblay et al., 1992; Yagci et al., 1996). Its action is mediated through voltage gated calcium channels (Haning et al., 1970; Aguilera and Catt, 1986; Kenyon et al., 1991) but also may require the presence of angiotensin II, perhaps generated by the intra-adrenal RAS, as well (Yamaguchi et al., 1990; Tremblay et al., 1992; Gupta et al., 1995; Vinson et al., 1998), certainly the actions of angiotensin II and potassium ions are linked (Shepherd et al., 1991).
ACTH, has long been recognized as an acute stimulator of aldosterone secretion (Haning et al., 1970; Aguilera and Catt, 1978; Braley et al., 1992; Williams, 2005; Hattangady et al., 2012; Bollag, 2014). In the rat, this presents an enigma, how is this achieved?—given what we now know about the distribution of MC2R and CYP11A expressing cell types, which in the glomerulosa are seemingly confined to the inner ZU and do not coincide with CYP11B2 (Gorrigan et al., 2011; Guasti et al., 2013a). But glomerulosa preparations, such as used by many authors (Haning et al., 1970; Aguilera and Catt, 1978; Braley et al., 1992; Williams, 2005) clearly will contain both CYP11B2 and CYP11A/MC2R-expressing cell types, as well as, usually, some fasciculata cells as well. This raises the possibility of cell-cell interaction, developed further below.
Chronically, on the other hand, ACTH does not support the glomerulosa cell phenotype, but instead downregulates CYP11B2, induces CYP11B1, and in these and other ways imposes the fasciculata phenotype instead (Aguilera et al., 1981; Ho and Vinson, 1993; Ni et al., 1995; Mitani et al., 1996). Though aldosterone secretion is also sensitive to ACTH stimulation (Hattangady et al., 2012), MC2R inactivated mice have normal glomerulosa, but greatly involuted fasciculata (Chida et al., 2007), whereas POMC knock-out may disrupt the adrenal entirely (see below). This may be attributed to the additional loss of α-MSH which in rats acts specifically on the glomerulosa in which MC5R abound (van der Kraan et al., 1998; Liakos et al., 2000): α-MSH acting on MC5R is 10 times more potent than ACTH (1-39), and 100 times more than γ-MSH in stimulating cAMP (Griffon et al., 1994). There is however a problem in that α-MSH stimulation of aldosterone production by the rat adrenal is not convincingly linked to cAMP, which is only stimulated at high α-MSH concentrations, whereas IP3 and PKC pathways are activated at lower levels (Kapas et al., 1992, 1994); others have suggested that a further MSH receptor remains to be discovered (Bicknell et al., 2000). In hypophysectomised rats, α-MSH, not ACTH is the POMC product that restores aldosterone secretion and its response to sodium depletion (Shenker et al., 1985), cf. (Vinson et al., 1980; Costa et al., 2011).
Downstream of the increased intracellular calcium evoked by potassium or angiotensin II, the orphan nuclear receptors Nurr1 and NFGFIB are both implicated in CYP11B2 expression, which also responds to COUP, SF-1 and CREB through promoter sites (Bassett et al., 2004b,c). NFGFIB, somewhat more widespread through the (human) cortex than Nurr1 (see Table 2), also mediates the expression of 3β-HSD2, which converts the Δ5,3β-hydroxy configuration of 17α-hydroxypregnenolone or DHEA to the corresponding Δ4,3-ketones (Bassett et al., 2004a,d). Angiotensin II also inhibits CYP17 expression, via PKC and src-kinase mediated pathways.
Many other factors have been found to modulate aldosterone secretion, at least under experimental conditions, these include particularly the neurotransmitters (Torda et al., 1988; Janossy et al., 1998; Vinson, 2003; Whitworth et al., 2003) cytokines, which are also produced within the gland (Judd and MacLeod, 1992; Ehrhart-Bornstein et al., 1998; Judd, 1998; Call et al., 2000), vascular products, including endothelins, nitric oxide, and adrenomedullin (Hinson and Kapas, 1998; Delarue et al., 2004), and adipokines (Willenberg et al., 2008).
Actions of ACTH on Adrenal Proliferation and Zonation
The earliest observations on the effects of hypophysectomy and ACTH treatment on adrenal form and function, showing the absolute dependence of the inner adrenocortical zones, but not the glomerulosa, on an intact functioning pituitary, was borne out by later experiments using specific gene knockout methods. First, the elimination of POMC products, including ACTH, was shown to prevent the complete development of the adrenal gland. In one study, the adrenals of POMC−∕− mice were not macroscopically discernable, and both corticosterone and aldosterone were undetectable (Yaswen et al., 1999). However, in another report, although both fasciculata and reticularis/X-zone were greatly reduced in the mutant animals, the glomerulosa was relatively unaffected, and this was reflected in the milder effect on circulating aldosterone, while corticosterone was virtually eliminated (Coll et al., 2004). The administration of ACTH restored both circulating corticosterone (but not aldosterone) and adrenocortical structure to normal (Coll et al., 2004). In a later study, MC2R−∕− animals were also shown to have normal glomerulosa structure, but greatly reduced inner adrenocortical zones, combined with undetectable plasma corticosterone while aldosterone was reduced by two thirds (Chida et al., 2007). These effects, of POMC [at least in the (Coll et al., 2004) study] and MC2R knockout, appear accurately to reproduce the actions of hypophysectomy (Chester Jones, 1957; Deane, 1962; Vinson, 2003). It is curious that the rat adrenal also expresses AgRP, an α-MSH antagonist (Yang et al., 1999) but only in the fasciculata and reticularis, and not the glomerulosa (Bicknell et al., 2000): in the mouse, the agouti locus is associated with X-zone, but not reticularis, morphology (Tanaka et al., 1994, 2006).
Other studies suggest that even within the zona fasciculata different areas may respond differently to chronic stress, which induced hyperplasia in the outer fasciculata, and hypertrophy in the inner fasciculata, while glomerulosa cell size was reduced (Ulrich-Lai et al., 2006).
Peptides derived from pro-γ-MSH, a 16kD fragment from the N-terminus of the POMC molecule secreted by the pituitary, that do not contain the γ-MSH sequence are thought to have a role in stimulating adrenocortical proliferation (Estivariz et al., 1982, 1988) and POMC 1-28 in particular has been shown to have this activity. An adrenal serine protease, designated AsP, which is thought to generate mitogenic peptides from pro-γ-MSH at the adrenocortical cell surface, has been characterized (Bicknell et al., 2001; Bicknell, 2003). Support for this concept has been provided by some subsequent authors, who found that POMC 1-28 stimulated adrenocortical proliferation in dexamethasone or hypophysectomised rats, though primarily in the glomerulosa (Torres et al., 2010; Mendonca and Lotfi, 2011), but others found it had no effect on adrenal growth in POMC−∕− mice, although there was a good response to ACTH (Coll et al., 2006). In contrast, in vitro, rat POMC 1-28 stimulated proliferation in cultured rat glomerulosa and fasciculata/reticularis cells, but ACTH was inhibitory (Mattos et al., 2011). The discrepancy between the stimulatory actions of ACTH on adrenal cell proliferation in vivo and its inhibitory actions in vitro is difficult to resolve. It is possible that other tissue components, such as the vasculature (Figure 4), absent from cell cultures, are required for the proliferative actions of ACTH in vivo (Thomas et al., 2004).
Although dependence on ACTH is paramount, other factors are known to modulate fasciculata cell secretory activity, including cytokines, which are differentially produced throughout the cortex, and have specific actions on individual cell types (Spangelo et al., 1995; Judd, 1998; Koldzic-Zivanovic et al., 2006; Woods and Judd, 2008), and neurotransmitters (Ehrhart-Bornstein et al., 2000). The possibility of medullary, neural and vascular regulation of cortical function is emphasized by the intimate relationship between these adrenal gland components (Figure 4).
Regulation of the Reticularis
The control of adrenal androgen secretion is problematic, in that although supported by ACTH, secretion of androstenedione and DHEA is dissociated from cortisol under some conditions, and particularly during adrenarche which has led some authors to postulate additional pituitary or other factors (McKenna et al., 1997; l'Allemand and Biason-Lauber, 2000), and, more recently, melatonin was found to stimulate DHEA release from cultured adrenals of the solitary hamster Phodopus sungorus (Rendon et al., 2015). However, the factors causing the increase of adrenal androgen secretion during adrenarche are largely unknown, as are those causing the later decline, or adrenopause (Dharia and Parker, 2004; Bird, 2012) leading some authors to postulate the importance of intra-adrenal factors; these could include growth factors (l'Allemand and Biason-Lauber, 2000), cytokines or even steroids. Cortisol stimulates DHEA secretion in H295R cells by inhibiting 3β-HSD (Topor et al., 2011), DHEA modulates chromaffin cell proliferation and differentiation (Chung K. F. et al., 2011) and LH receptors are present in human fasciculata and reticularis, albeit at a low level (Pabon et al., 1996; Rao, 2010). However, another provocative finding in this regard is that inhibition of seladin-1, which is strongly expressed in the fasciculata and reticularis of the human adrenal, specifically inhibits the ACTH induced secretion of DHEA in human adrenal cells, while having no effect on cortisol output (Battista et al., 2009). This awaits physiological interpretation.
The Significance of Adrenocortical Zonation—How Does the Standard Model Stand Up?
That adrenocortical cells arise in the capsular or subcapsular region of the gland and migrate inwards is now virtually unchallenged. That proliferation can occur throughout the gland is also clear, but does not affect the main thesis, even given there appear to be exceptional situations in which migration may be reversed.
It is also clear that the distribution of enzymes and other components between the zones show sharp phenotypic differences between the glomerulosa and the fasciculata, and slightly less sharp differences between the fasciculata and the reticularis, hence the inwardly migrating cells undergo more or less profound phenotypic changes in their lifespan. What remains unclear is how this is achieved, although recent advances in our understanding of the role of morphogens such as wnt and shh give some powerful guides: these go a long way to explaining how the glomerulosa becomes differentiated from the capsule. Remaining to be determined is how the fasciculata becomes distinct from the glomerulosa, or the reticularis from the fasciculata. What morphogens are involved here?
As well as the speculation that they could conceivably be products of neural or vascular components of the gland (Figure 4), one school of thought holds that it could be the steroid products themselves. From in vitro studies, and on the basis that there may be a gradient of steroids from the outer to the inner part of the cortex, Hornsby and colleagues postulated that this results in high concentrations of steroids in the inner zones, which, by acting as pseudosubstrates, could directly compromise cytochrome P450 structure and function, in this way perhaps inhibiting hydroxylase activity in the reticularis, for example (Hornsby, 1987). Though this is contrary to the usual concept of morphogen diffusion which posits a decrease in concentration with distance from the source, it acquires new life, and a new mechanism, with the characterization of GR in the human adrenal cortex, particularly in the reticularis (Paust et al., 2006).
That the individual zones have defined steroidogenic roles is clear. Whether they are completely autonomous is less so. Rather, because of the paucity of other steroidogenic enzymes in the CYP11B2 expressing cells of the glomerulosa, it is arguable that the substrate for CYP11B2 must come from the fasciculata, and the different ways this could occur are via cell-cell contact, or by uptake from the general circulation, as presumably is the case for extra-adrenal CYP11B2 thought by some to be expressed in the vasculature and other tissues (Hatakeyama et al., 1996; Taves et al., 2011; though not by others, MacKenzie et al., 2012) or by diffusion. Similarly, the production of androstenedione by the human gland would seem, on the basis of the zonal distribution of enzymes possibly to be a reflection of the availability of DHEA from the reticularis to 3β-HSD in the fasciculata.
If that is the case, then the regulation of these secretory products must also reflect co-operation between zones. Given the distribution of mRNA coding for MC2R in the rat adrenal, in the inner ZU, and fasciculata/reticularis but not in the CYP11B2 region of the glomerulosa (Gorrigan et al., 2011), the well-recognized stimulation of aldosterone secretion by ACTH is otherwise difficult to explain. Of course it is still possible that it is the MC5R in the glomerulosa to which ACTH binds. The actions of angiotensin II and α-MSH on aldosterone secretion in the rat are equally difficult to understand, because the appropriate receptors, AT1R and MC5R respectively, do not appear to coincide with CYP11A1, thus cholesterol side chain cleavage, the recognized site in the pathway for stimulation of steroid hormone secretion, seemingly cannot be directly affected by these agents (Figure 3).
Taken together, all this evidence seems to suggest it may be mistaken to think of the adrenal cortex, or even the whole adrenal gland, as simply a collection of different types of cells, each independently performing its role in isolation. Rather we should think of these different cell types as acting in concert. We have understood for some time now that there are intraadrenal mechanisms that modify and refine the signals from the systemic regulators. These comprise medullary products, cytokines, the adrenal renal-angiotensin system, among others, and have been reviewed elsewhere (Ehrhart-Bornstein et al., 1998). Extending this has been conceptually problematic in view of the apparently confounding effect of the blood flow through the gland, which would seemingly limit the action any cell might have on other than its closest neighbors by secreted products.
We now know that secreted products from adrenocortical cells can indeed pervade the gland through diffusion, and this has been demonstrated by the wnt-catenin and shh-Gli signaling systems. So if it is possible for these products then there is no reason why it cannot be the case for others, including perhaps catecholamines, cytokines, and the steroids themselves. This may give a partial explanation for the architecture of the gland, one of the problems posed at the start of this article—why are the zones arranged as concentric spheres? One answer may be that this has the potential to maximize the likelihood of diffused products arriving at their targets. If we take as an example the secretion of shh from its origin in the ZU, random walk diffusion to its target in the inner capsule is better achieved when the capsule completely envelops the glomerulosa than it would be if the system were more open, or composed of sheets, like a mesentery or secretory epithelium.
In addition, there is absolutely no reason to suppose the steroids themselves do not move between the zones, as substrates or modifiers. How else can we explain how aldosterone or androstenedione are synthesized? And, given that MC2R and CYP11A1 are expressed in the ZU and fasciculata, but not the CYP11B2 expressing cells of the rat glomerulosa, how else can we explain how ACTH has its undoubted action on aldosterone secretion, other than by the enhanced provision of steroid substrates from the ZU or the fasciculata to the CYP11B2 cells in the glomerulosa?
Like the human body itself, the function of the adrenal gland is an integrated whole, much greater than the sum of its parts. We should aim to think of it that way.
Author Contributions
GV researched and wrote this article.
Conflict of Interest Statement
The author declares that the research was conducted in the absence of any commercial or financial relationships that could be construed as a potential conflict of interest.
Acknowledgments
I am most grateful to Drs. Peter King and Leonardo Guasti, of the Centre for Endocrinology, William Harvey Research Institute, Queen Mary University of London, London EC1M 6BQ, for their careful reading of the manuscript and for their helpful comments and suggestions.
References
Aguilera, G., and Catt, K. J. (1978). Loci of action of regulators of aldosterone biosynthesis in isolated glomerulosa cells. Endocrinology 104, 1046–1052. doi: 10.1210/endo-104-4-1046
Aguilera, G., and Catt, K. J. (1986). Participation of voltage-dependent calcium channels in the regulation of adrenal glomerulosa function by angiotensin II and potassium. Endocrinology 118, 112–118. doi: 10.1210/endo-118-1-112
Aguilera, G., Fujita, K., and Catt, K. J. (1981). Mechanisms of inhibition of aldosterone secretion by adrenocorticotropin. Endocrinology 108, 522–528. doi: 10.1210/endo-108-2-522
Aiba, M., and Fujibayashi, M. (2011). Alteration of subcapsular adrenocortical zonation in humans with aging: the progenitor zone predominates over the previously well-developed zona glomerulosa after 40 years of age. J. Histochem. cytochem. 59, 557–564. doi: 10.1369/0022155411404071
Aigueperse, C., Val, P., Pacot, C., Darne, C., Lalli, E., Sassone-Corsi, P., et al. (2001). SF-1 (steroidogenic factor-1), C/EBPbeta (CCAAT/enhancer binding protein), and ubiquitous transcription factors NF1 (nuclear factor 1) and Sp1 (selective promoter factor 1) are required for regulation of the mouse aldose reductase-like gene (AKR1B7) expression in adrenocortical cells. Mol. Endocrinol. 15, 93–111. doi: 10.1210/mend.15.1.0577
Akhtar, M. K., Kelly, S. L., and Kaderbhai, M. A. (2005). Cytochrome b(5) modulation of 17a-hydroxylase and 17-20 lyase (CYP17) activities in steroidogenesis. J. Endocrinol. 187, 267–274. doi: 10.1677/joe.1.06375
Arnold, J. (1866). Ein beitrag zu der feineren structur und demchemismus der nebennieren. Virchows Archiv Pathologie, Anatomie, Physiologie 39, 64–117. doi: 10.1007/BF01979887
Atanassova, N., and Koeva, Y. (2012). “Hydroxysteroid dehydrogenases – biological role and clinical importance – review, Chapter 6,” in Dehydrogenases, ed R. A. Canuto (Intech), 115–164.
Auchus, R. J., Lee, T. C., and Miller, W. L. (1998). Cytochrome b5 augments the 17,20-lyase activity of human P450c17 without direct electron transfer. J. Biol. Chem. 273, 3158–3165. doi: 10.1074/jbc.273.6.3158
Bandyopadhyay, A., Tsuji, K., Cox, K., Harfe, B. D., Rosen, V., and Tabin, C. J. (2006). Genetic analysis of the roles of BMP2, BMP4, and BMP7 in limb patterning and skeletogenesis. PLoS Genet. 2:e216. doi: 10.1371/journal.pgen.0020216
Barker, S., Laird, S. M., Ho, M. M., Vinson, G. P., and Hinson, J. P. (1992). Characterisation of a rat adrenocortical inner zone specific antigen and identification of a putative precursor. J. Mol. Endocr. 9, 95–102. doi: 10.1677/jme.0.0090095
Bassett, M. H., Suzuki, T., Sasano, H., de Vries, C. J. M., Jimenez, P. T., Carr, B. R., et al. (2004a). The orphan nuclear receptor NGFIB regulates transcription of 3 beta-hydroxysteroid dehydrogenase - Implications for the control of adrenal functional zonation. J. Biol. Chem. 279, 37622–37630. doi: 10.1074/jbc.M405431200
Bassett, M. H., Suzuki, T., Sasano, H., White, P. C., and Rainey, W. E. (2004b). The orphan nuclear receptors NURR1 and NGFIB regulate adrenal aldosterone production. Mol. Endocrinol. 18, 279–290. doi: 10.1210/me.2003-0005
Bassett, M. H., White, P. C., and Rainey, W. E. (2004c). The regulation of aldosterone synthase expression. Mol. Cell. Endocrinol. 217, 67–74. doi: 10.1016/j.mce.2003.10.011
Bassett, M. H., White, P. C., and Rainey, W. E. (2004d). A role for the NGFI-B family in adrenal zonation and adrenocortical disease. Endocr. Res. 30, 567–574. doi: 10.1081/ERC-200043715
Bastida, C. M., Cremades, A., Castells, M. T., Lopez-Contreras, A. J., Lopez-Garcia, C., Sanchez-Mas, J., et al. (2007). Sexual dimorphism of ornithine decarboxylase in the mouse adrenal: influence of polyamine deprivation on catecholamine and corticoid levels. Am. J. Physiol. Endocrinol. Metab. 292, E1010–E1017. doi: 10.1152/ajpendo.00316.2006
Battista, M. C., Roberge, C., Martinez, A., and Gallo-Payet, N. (2009). 24-dehydrocholesterol reductase/seladin-1: a key protein differentially involved in adrenocorticotropin effects observed in human and rat adrenal cortex. Endocrinology 150, 4180–4190. doi: 10.1210/en.2009-0410
Beery, E., Middel, P., Bahn, A., Willenberg, H. S., Hagos, Y., Koepsell, H., et al. (2003). Molecular evidence of organic ion transporters in the rat adrenal cortex with adrenocorticotropin-regulated zonal expression. Endocrinology 144, 4519–4526. doi: 10.1210/en.2002-221001
Belgorosky, A., Baquedano, M. S., Guercio, G., and Rivarola, M. A. (2008). Adrenarche: postnatal adrenal zonation and hormonal and metabolic regulation. Horm. Res. 70, 257–267. doi: 10.1159/000157871
Bell, J. B., Gould, R. P., Hyatt, P. J., Tait, J. F., and Tait, S. A. (1978). Properties of rat adrenal zona reticularis cells: preparation by gravitational sedimentation. J. Endocrinol. 77, 25–41. doi: 10.1677/joe.0.0770025
Bell, J. B., Gould, R. P., Hyatt, P. J., Tait, J. F., and Tait, S. A. (1979). Properties of rat adrenal zona reticularis cells: production and stimulation of certain steroids. J. Endocrinol. 83, 435–447. doi: 10.1677/joe.0.0830435
Bernet, F., Bernard, J., Laborie, C., Montel, V., Maubert, E., and Dupouy, J. P. (1994). Neuropeptide-Y (NPY)- and vasoactive-intestinal-peptide (VIP)-induced aldosterone secretion by rat capsule/glomerular zone could be mediated by catecholamines via b(1) adrenergic-receptors. Neurosci. Lett. 166, 109–112. doi: 10.1016/0304-3940(94)90852-4
Berthon, A., Martinez, A., Bertherat, J., and Val, P. (2012). Wnt/beta-catenin signalling in adrenal physiology and tumour development. Mol. Cell. Endocrinol. 351, 87–95. doi: 10.1016/j.mce.2011.09.009
Bicknell, A. B. (2003). Identification of a serine protease involved with the regulation of adrenal growth. Ann. N.Y. Acad. Sci. 994, 118–122. doi: 10.1111/j.1749-6632.2003.tb03170.x
Bicknell, A. B., Lomthaisong, K., Gladwell, R. T., and Lowry, P. J. (2000). Agouti related protein in the rat adrenal cortex: implications for novel autocrine mechanisms modulating the actions of pro-opiomelanocortin peptides. J. Neuroendocrinol. 12, 977–982. doi: 10.1046/j.1365-2826.2000.00543.x
Bicknell, A. B., Lomthaisong, K., Woods, R. J., Hutchinson, E. G., Bennett, H. P., Gladwell, R. T., et al. (2001). Characterization of a serine protease that cleaves pro-gamma-melanotropin at the adrenal to stimulate growth. Cell 105, 903–912. doi: 10.1016/S0092-8674(01)00403-2
Bird, I. M. (2012). In the zone: understanding zona reticularis function and its transformation by adrenarche. J. Endocrinol. 214, 109–111. doi: 10.1530/JOE-12-0246
Black, V. H., Barilla, J. R., and Martin, K. O. (1989). Effects of age, adrenocorticotropin, and dexamethasone on a male-specific cytochrome P450 localized in the inner zone of the guinea pig adrenal. Endocrinology 124, 2494–2248. doi: 10.1210/endo-124-5-2494
Bocian-Sobkowska, J., Malendowicz, L. K., and Wozniak, W. (1997). Comparative stereological studies on zonation and cellular composition of adrenal glands of normal and anencephalic human fetuses. II. Cellular composition of the gland. Histol. Histopathol. 12, 391–399.
Bollag, W. B. (2014). Regulation of aldosterone synthesis and secretion. Compr. Physiol. 4, 1017–1055. doi: 10.1002/cphy.c130037
Bornstein, S. R., and Ehrhart-Bornstein, M. (1992). Ultrastructural evidence for a paracrine regulation of the rat adrenal cortex mediated by the local release of catecholamines from chromaffin cells. Endocrinology 131, 3126–3318.
Braley, L. M., Adler, G. K., Mortensen, R. M., Conlin, P. R., Chen, R., Hallahan, J., et al. (1992). Dose effect of adrenocorticotropin on aldosterone and cortisol biosynthesis in cultured bovine adrenal glomerulosa cells: in vitro correlate of hyperreninemic hypoaldosteronism. Endocrinology 131, 187–194.
Brennan, C. H., Chittka, A., Barker, S., and Vinson, G. P. (2008). Eph receptors and zonation in the rat adrenal cortex. J. Endocrinol. 198, 185–191. doi: 10.1677/JOE-08-0084
Buijs, J. T., Petersen, M., van der Horst, G., and van der Pluijm, G. (2010). Bone morphogenetic proteins and its receptors; therapeutic targets in cancer progression and bone metastasis? Curr. Pharm. Des. 16, 1291–1300. doi: 10.2174/138161210791033987
Cahill, M. A. (2007). Progesterone receptor membrane component 1: an integrative review. J. Steroid Biochem. Mol. Biol. 105, 16–36. doi: 10.1016/j.jsbmb.2007.02.002
Call, G. B., Husein, O. F., McIlmoil, C. J., Adams, A., Heckmann, R. A., and Judd, A. M. (2000). Bovine adrenal cells secrete interleukin-6 and tumor necrosis factor in vitro. Gen. Comp. Endocrinol. 118, 249–261. doi: 10.1006/gcen.2000.7458
Carpenter, C. C., Davis, J. O., and Ayers, C. R. (1961). Relation of renin, angiotensin II, and experimental renal hypertension to aldosterone secretion. J. Clin. Invest. 40, 2026–2042. doi: 10.1172/JCI104429
Carsia, R. V., Macdonald, G. J., Gibney, J. A., Tilly, K. I., and Tilly, J. L. (1996). Apoptotic cell death in the rat adrenal gland: an in vivo and in vitro investigation. Cell Tissue.Res. 283, 247–254. doi: 10.1007/s004410050535
Cater, D. B., and Lever, J. D. (1954). The zona intermedia of the adrenal cortex; a correlation of possible functional significance with development, morphology and histochemistry. J. Anat. 88, 437–454.
Chambaz, E. M., Cochet, C., Souchelnitskiy, S., Defaye, G., and Feige, J. J. (1994). Growth-factors and pericellular components as a local signaling relay in the action of systemic hormones. Horm. Res. 42, 35–38. doi: 10.1159/000184142
Chang, S. P., Morrison, H. D., Nilsson, F., Kenyon, C. J., West, J. D., and Morley, S. D. (2013). Cell proliferation, movement and differentiation during maintenance of the adult mouse adrenal cortex. PLoS ONE 8:e81865. doi: 10.1371/journal.pone.0081865
Chang, S. P., Mullins, J. J., Morley, S. D., and West, J. D. (2011). Transition from organogenesis to stem cell maintenance in the mouse adrenal cortex. Organogenesis 7, 267–280. doi: 10.4161/org.7.4.18060
Chida, D., Nakagawa, S., Nagai, S., Sagara, H., Katsumata, H., Imaki, T., et al. (2007). Melanocortin 2 receptor is required for adrenal gland development, steroidogenesis, and neonatal gluconeogenesis. Proc. Natl. Acad. Sci. U.S.A. 104, 18205–18210. doi: 10.1073/pnas.0706953104
Chung, K. F., Qin, N., Androutsellis-Theotokis, A., Bornstein, S. R., and Ehrhart-Bornstein, M. (2011). Effects of dehydroepiandrosterone on proliferation and differentiation of chromaffin progenitor cells. Mol. Cell. Endocrinol. 336, 141–148. doi: 10.1016/j.mce.2010.11.028
Chung, S., Son, G. H., and Kim, K. (2011). Circadian rhythm of adrenal glucocorticoid: its regulation and clinical implications. Biochim. Biophys. Acta 1812, 581–591. doi: 10.1016/j.bbadis.2011.02.003
Colby, H. D., Huang, Y., Jiang, Q., and Voigt, J. M. (2000). Bimodal expression of CYP2D16 in the guinea pig adrenal cortex. Pharmacology 61, 78–82. doi: 10.1159/000028384
Colby, H. D., Levitt, M., Pope, M. R., and Johnson, P. B. (1992). Differential effects of adrenocorticotropic hormone on steroid hydroxylase activities in the inner and outer zones of the guinea pig adrenal cortex. J. Steroid Biochem. Mol. Biol. 42, 329–335. doi: 10.1016/0960-0760(92)90136-7
Coll, A. P., Challis, B. G., Yeo, G. S., Snell, K., Piper, S. J., Halsall, D., et al. (2004). The effects of proopiomelanocortin deficiency on murine adrenal development and responsiveness to adrenocorticotropin. Endocrinology 145, 4721–4727. doi: 10.1210/en.2004-0491
Coll, A. P., Fassnacht, M., Klammer, S., Hahner, S., Schulte, D. M., Piper, S., et al. (2006). Peripheral administration of the N-terminal pro-opiomelanocortin fragment 1-28 to Pomc-/- mice reduces food intake and weight but does not affect adrenal growth or corticosterone production. J. Endocrinol. 190, 515–525. doi: 10.1677/joe.1.06749
Conti, B., Jahng, J. W., Tinti, C., Son, J. H., and Joh, T. H. (1997). Induction of interferon-gamma inducing factor in the adrenal cortex. J. Biol. Chem. 272, 2035–2037. doi: 10.1074/jbc.272.4.2035
Costa, J. L., Forbes, S., Brennan, M. B., and Hochgeschwender, U. (2011). Genetic modifications of mouse proopiomelanocortin peptide processing. Mol. Cell. Endocrinol. 336, 14–22. doi: 10.1016/j.mce.2010.12.032
de Joussineau, C., Sahut-Barnola, I., Levy, I., Saloustros, E., Val, P., Stratakis, C. A., et al. (2012). The cAMP pathway and the control of adrenocortical development and growth. Mol. Cell. Endocrinol. 351, 28–36. doi: 10.1016/j.mce.2011.10.006
de Mendonca, P. O., Costa, I. C., and Lotfi, C. F. (2014). The involvement of Nek2 and Notch in the proliferation of rat adrenal cortex triggered by POMC-derived peptides. PLoS ONE 9:e108657. doi: 10.1371/journal.pone.0108657
Deane, H. W. (1962). “The anatomy, chemistry, and physiology of adrenocortical tissue,” in Handbuch der Experimentalle Pharmakologie, Vol. 14 (Berlin: Springer), 1–85.
Delarue, C., Conlon, J. M., Remy-Jouet, I., Fournier, A., and Vaudry, H. (2004). Endothelins as local activators of adrenocortical cells. J. Mol. Endocrinol. 32, 1–7. doi: 10.1677/jme.0.0320001
Desmond, J. C., Mountford, J. C., Drayson, M. T., Walker, E. A., Hewison, M., Ride, J. P., et al. (2003). The aldo-keto reductase AKR1C3 is a novel suppressor of cell differentiation that provides a plausible target for the non-cyclooxygenase-dependent antineoplastic actions of nonsteroidal anti-inflammatory drugs. Cancer Res. 63, 505–512.
Dharia, S., and Parker, C. R. Jr. (2004). Adrenal androgens and aging. Semin. Reprod. Med. 22, 361–368. doi: 10.1055/s-2004-861552
Dobbie, J. W., and Symington, T. (1966). The human adrenal gland with special reference to the vasculature. J. Endocrinol. 34, 479–489. doi: 10.1677/joe.0.0340479
Dostoiewsky, A. (1886). Ein Beitrag zur mikroskopischen Anatomie der Nebennieren bei Säugethieren. Archiv für mikroskopische Anatomie 27, 272–296. doi: 10.1007/BF02955421
Dupont, E., Rheaume, E., Simard, J., Luu-The, V., Labrie, F., and Pelletier, G. (1991). Ontogenesis of 3 beta-hydroxysteroid dehydrogenase/delta 5-delta 4 isomerase in the rat adrenal as revealed by immunocytochemistry and in situ hybridization. Endocrinology 129, 2687–2292. doi: 10.1210/endo-129-5-2687
Ehrhart-Bornstein, M., Haidan, A., Alesci, S., and Bornstein, S. R. (2000). Neurotransmitters and neuropeptides in the differential regulation of steroidogenesis in adrenocortical-chromaffin co-cultures. Endocr. Res. 26, 833–842. doi: 10.3109/07435800009048606
Ehrhart-Bornstein, M., Hinson, J. P., Bornstein, S. R., Scherbaum, W. A., and Vinson, G. P. (1998). Intraadrenal interactions in the regulation of adrenocortical steroidogenesis. Endocr. Rev. 19, 101–143. doi: 10.1210/edrv.19.2.0326
El Wakil, A. (2013). Inactivation of Cav3.2 T-type calcium channels regulates partially ectopic aldosterone [production in Task1 knckout mice without correcting their adrenocortical zonation pattern. Egypt J. Exp. Biol. 9, 95–100.
Engeland, W. C., Ennen, W. B., Elayaperumal, A., Durand, D. A., and Levay-Young, B. K. (2005). Zone-specific cell proliferation during compensatory adrenal growth in rats. Am. J. Physiol. Endocrinol.metab. 288, E298–E306. doi: 10.1152/ajpendo.00307.2004
Engeland, W. C., and Levay-Young, B. K. (1999). Changes in the glomerulosa cell phenotype during adrenal regeneration in rats. Am. J. Physiol. 276, R1374–R1182.
Ennen, W. B., Levay-Young, B. K., and Engeland, W. C. (2005). Zone-specific cell proliferation during adrenocortical regeneration after enucleation in rats. Am. J.Physiol. Endocrinol. Metab. 289, E883–E891. doi: 10.1152/ajpendo.00031.2005
Estivariz, F. E., Iturriza, F., McLean, C., Hope, J., and Lowry, P. J. (1982). Stimulation of adrenal mitogenesis by N-terminal proopiocortin peptides. Nature 297, 419–422. doi: 10.1038/297419a0
Estivariz, F. E., Morano, M. I., Carino, M., Jackson, S., and Lowry, P. J. (1988). Adrenal regeneration in the rat is mediated by mitogenic N-terminal pro-opiomelanocortin peptides generated by changes in precursor processing in the anterior pituitary. J. Endocrinol. 116, 207–216. doi: 10.1677/joe.0.1160207
Fahrenkrug, J., Hannibal, J., and Georg, B. (2008). Diurnal rhythmicity of the canonical clock genes Per1, Per2 and Bmal1 in the rat adrenal gland is unaltered after hypophysectomy. J. Neuroendocrinol. 20, 323–329. doi: 10.1111/j.1365-2826.2008.01651.x
Fattah, D. I., Whitehouse, B. J., and Vinson, G. P. (1977). Biosynthesis of Aldosterone from 18-hydroxylated precursors by rat adrenal tissue in vitro. J. Endocrinol. 75, 187–195. doi: 10.1677/joe.0.0750187
Finco, I., LaPensee, C. R., Krill, K. T., and Hammer, G. D. (2015). Hedgehog signaling and steroidogenesis. Annu. Rev. Physiol. 77, 105–129. doi: 10.1146/annurev-physiol-061214-111754
Freedman, B. D., Kempna, P. B., Carlone, D. L., Shah, M. S., Guagliardo, N. A., Barrett, P. Q., et al. (2013). Adrenocortical zonation results from lineage conversion of differentiated zona glomerulosa cells. Dev. Cell 26, 666–673. doi: 10.1016/j.devcel.2013.07.016
Fukuda, T., Takahashi, K., Suzuki, T., Saruta, M., Watanabe, M., Nakata, T., et al. (2005). Urocortin 1, urocortin 3/stresscopin, and corticotropin-releasing factor receptors in human adrenal and its disorders. J. Clin. Endocrinol. Metab. 90, 4671–4678. doi: 10.1210/jc.2005-0090
Gallo-Payet, N., and Escher, E. (1985). Adrenocorticotropin receptors in rat adrenal glomerulosa cells. Endocrinology 117, 38–46. doi: 10.1210/endo-117-1-38
Godoy, J. R., Funke, M., Ackermann, W., Haunhorst, P., Oesteritz, S., Capani, F., et al. (2011). Redox atlas of the mouse.immunohistochemical detection of glutaredoxin-, peroxiredoxin-, and thioredoxin-family proteins in various tissues of the laboratory mouse. Biochim. Biophys. Acta 1810, 2–92. doi: 10.1016/j.bbagen.2010.05.006
Gorrigan, R. J., Guasti, L., King, P., Clark, A. J., and Chan, L. F. (2011). Localisation of the melanocortin-2-receptor and its accessory proteins in the developing and adult adrenal gland. J. Mol. Endocrinol. 46, 227–232. doi: 10.1530/JME-11-0011
Gottschau, M. (1883). Struktur und embryonale Entwicklung der Nebennieren bei Säugetieren. Arch. Anat. Physiol. Lpz. 9, 412–458.
Griffon, N., Mignon, V., Facchinetti, P., Diaz, J., Schwartz, J. C., and Sokoloff, P. (1994). Molecular cloning and characterization of the rat fifth melanocortin receptor. Biochem. Biophys. Res. Commun. 200, 1007–1014. doi: 10.1006/bbrc.1994.1550
Gross, F. (1958). Renin und Hypertensin: Physiologische oder pathologische Wirkstoffe. Klin Wochenschr 36, 693–706. doi: 10.1007/BF01493136
Guasti, L., Candy Sze, W. C., McKay, T., Grose, R., and King, P. J. (2013a). FGF signalling through Fgfr2 isoform IIIb regulates adrenal cortex development. Mol. Cell. Endocrinol. 371, 182–188. doi: 10.1016/j.mce.2013.01.014
Guasti, L., Cavlan, D., Cogger, K., Banu, Z., Shakur, A., Latif, S., et al. (2013b). Dlk1 up-regulates Gli1 expression in male rat adrenal capsule cells through the activation of beta1 integrin and ERK1/2. Endocrinology 154, 4675–4684. doi: 10.1210/en.2013-1211
Guasti, L., Paul, A., Laufer, E., and King, P. (2011). Localization of Sonic hedgehog secreting and receiving cells in the developing and adult rat adrenal cortex. Mol. Cell. Endocrinol. 336, 117–122. doi: 10.1016/j.mce.2010.11.010
Gummow, B. M., Winnay, J. N., and Hammer, G. D. (2003). Convergence of Wnt signaling and steroidogenic factor-1 (SF-1) on transcription of the rat inhibin alpha gene. J. Biol. Chem. 278, 26572–26579. doi: 10.1074/jbc.M212677200
Gupta, P., Francosaenz, R., and Mulrow, P. J. (1995). Locally generated angiotensin-II in the adrenal-gland regulates basal, corticotropin-stimulated, and potassium-stimulated aldosterone secretion. Hypertension 25, 443–448. doi: 10.1161/01.HYP.25.3.443
Haidan, A., Bornstein, S. R., Liu, Z., Walsh, L. P., Stocco, D. M., and Ehrhart-Bornstein, M. (2000). Expression of adrenocortical steroidogenic acute regulatory (StAR) protein is influenced by chromaffin cells. Mol. Cell. Endocrinol. 165, 25–32. doi: 10.1016/S0303-7207(00)00267-7
Halder, S. K., Takemori, H., Hatano, O., Nonaka, Y., Wada, A., and Okamoto, M. (1998). Cloning of a membrane-spanning protein with epidermal growth factor- like repeat motifs from adrenal glomerulosa cells. Endocrinology 139, 3316–3328. doi: 10.1210/en.139.7.3316
Hall, P. F. (1991). Cytochrome P-450 C21scc: one enzyme with two actions: hydroxylase and lyase. J. Steroid Biochem. Mol. Biol. 40, 527–532. doi: 10.1016/0960-0760(91)90272-7
Haning, R., Tait, S. A. S., and Tait, J. F. (1970). In vitro effects of ACTH, angiotensins, serotonin, and potassium on steroid output and conversion of corticosterone to aldosterone by isolated adrenal cells. Endocrinology 87, 1147–1167. doi: 10.1210/endo-87-6-1147
Hatakeyama, H., Miyamori, I., Takeda, Y., Yamamoto, H., and Mabuchi, H. (1996). The expression of steroidogenic enzyme genes in human vascular cells. Biochem. Mol. Biol. Int. 40, 639–645. doi: 10.1080/15216549600201233
Hattangady, N. G., Olala, L. O., Bollag, W. B., and Rainey, W. E. (2012). Acute and chronic regulation of aldosterone production. Mol. Cell. Endocrinol. 350, 151–162. doi: 10.1016/j.mce.2011.07.034
Heitzmann, D., Derand, R., Jungbauer, S., Bandulik, S., Sterner, C., Schweda, F., et al. (2008). Invalidation of TASK1 potassium channels disrupts adrenal gland zonation and mineralocorticoid homeostasis. EMBO J. 27, 179–187. doi: 10.1038/sj.emboj.7601934
Hershkovitz, L., Beuschlein, F., Klammer, S., Krup, M., and Weinstein, Y. (2007). Adrenal 20alpha-hydroxysteroid dehydrogenase in the mouse catabolizes progesterone and 11-deoxycorticosterone and is restricted to the X-zone. Endocrinology 148, 976–988. doi: 10.1210/en.2006-1100
Hinson, J. P., and Kapas, S. (1998). The role of endothelial cell products in the regulation of adrenocortical function: actions of endothelin, nitric oxide, adrenomedullin and PAMP. Horm. Metab. Res. 30, 334–340. doi: 10.1055/s-2007-978894
Ho, M. M., and Vinson, G. P. (1993). 11 beta-Hydroxylase gene expression in the rat adrenal cortex. J. Endocrinol. 139, 301–306. doi: 10.1677/joe.0.1390301
Ho, M. M., and Vinson, G. P. (1995). Endocrine control of the distribution of basic fibroblast growth- factor, insulin-like growth-factor-i and transforming growth factor- beta(1) messenger-rnas in adult-rat adrenals using nonradioactive in- situ hybridization. J. Endocrinol. 144, 379–387. doi: 10.1677/joe.0.1440379
Ho, M. M., and Vinson, G. P. (1997). Peptide growth factors and the adrenal cortex. Microsc. Res. Tech. 36, 558–568.
Ho, M. M., and Vinson, G. P. (1998). Transcription of (pro)renin mRNA in the rat adrenal cortex, and the effects of ACTH treatment and a low sodium diet. J. Endocrinol. 157, 217–223. doi: 10.1677/joe.0.1570217
Holzwarth, M. A., Cunningham, L. A., and Kleitman, N. (1987). The role of adrenal nerves in the regulation of adrenocortical functions. Ann. N.Y. Acad. Sci. 512, 449–464. doi: 10.1111/j.1749-6632.1987.tb24980.x
Hornsby, P. J. (1987). Physiological and pathological effects of steroids on the function of the adrenal cortex. J. Steroid Biochem. 27, 1161–1171. doi: 10.1016/0022-4731(87)90203-2
Huang, Y., Jiang, Q., Voigt, J. M., Debolt, K. M., and Colby, H. D. (1996). Strain differences in adrenal CYP2D16 expression in guinea pigs. Relationship to xenobiotic metabolism. Biochem. Pharmacol. 52, 1925–1929. doi: 10.1016/S0006-2952(96)00665-X
Huang, Z., Ohno, N., Terada, N., Saitoh, Y., Chen, J., and Ohno, S. (2013). Immunohistochemical detection of angiotensin II receptors in mouse cerebellum and adrenal gland using “in vivo cryotechnique”. Histochem. Cell Biol. 140, 477–490. doi: 10.1007/s00418-013-1084-y
Ishida, A., Mutoh, T., Ueyama, T., Bando, H., Masubuchi, S., Nakahara, D., et al. (2005). Light activates the adrenal gland: timing of gene expression and glucocorticoid release. Cell Metab. 2, 297–307. doi: 10.1016/j.cmet.2005.09.009
Ishimura, K., and Fujita, H. (1997). Light and electron microscopic immunohistochemistry of the localization of adrenal steroidogenic enzymes. Microsc. Res. Tech. 36, 445–453.
Ito, M., Yu, R., and Jameson, J. L. (1997). DAX-1 inhibits SF-1-mediated transactivation via a carboxy-terminal domain that is deleted in adrenal hypoplasia congenita. Mol. Cell. Biol. 17, 1476–1483. doi: 10.1128/MCB.17.3.1476
Janossy, A., Orso, E., Szalay, K. S., Juranyi, Z., Beck, M., Vizi, E. S., et al. (1998). Cholinergic regulation of the rat adrenal zona glomerulosa. J. Endocrinol. 157, 305–315. doi: 10.1677/joe.0.1570305
Jiang, Q., Huang, Y., Voigt, J. M., DeBolt, K. M., Kominami, S., Takemori, S., et al. (1996). Differential effects of adrenocorticotropin in vivo on cytochromes P4502D16 and P450c17 in the guinea pig adrenal cortex. Endocrinology 137, 4811–4486. doi: 10.1210/en.137.11.4811
Judd, A. M. (1998). Cytokine expression in the rat adrenal cortex. Horm. Metab. Res. 30, 404–410. doi: 10.1055/s-2007-978905
Judd, A. M., and MacLeod, R. M. (1992). Adrenocorticotropin increases interleukin-6 release from rat adrenal zona glomerulosa cells. Endocrinology 130, 1245–1254.
Judd, A. M., and MacLeod, R. M. (1995). Differential release of tumor necrosis factor and IL-6 from adrenal zona glomerulosa cells in vitro. Am. J. Physiol. 268, E114–E120.
Kapas, S., Orford, C. D., Barker, S., Vinson, G. P., and Hinson, J. P. (1992). Studies on the intracellular mechanism of action of alpha-melanocyte-stimulating hormone on rat adrenal zona glomerulosa. J. Mol. Endocrinol. 9, 47–54. doi: 10.1677/jme.0.0090047
Kapas, S., Purbrick, A., Barker, S., Vinson, G. P., and Hinson, J. P. (1994). Alpha-melanocyte-stimulating hormone-induced inhibition of angiotensin II receptor-mediated events in the rat adrenal zona glomerulosa. J. Mol. Endocrinol. 13, 95–104. doi: 10.1677/jme.0.0130095
Kenyon, C. J., Shepherd, R. M., Fraser, R., Pediani, J. D., and Elder, H. Y. (1991). The role of potassium and other ions in the control of aldosterone synthesis. Endocr. Res. 17, 225–236. doi: 10.1080/07435809109027199
Kim, A. C., Barlaskar, F. M., Heaton, J. H., Else, T., Kelly, V. R., Krill, K. T., et al. (2009). In search of adrenocortical stem and progenitor cells. Endocr. Rev. 30, 241–263. doi: 10.1210/er.2008-0039
Kim, A. C., and Hammer, G. D. (2007). Adrenocortical cells with stem/progenitor cell properties: recent advances. Mol. Cell. Endocrinol. 265–266, 10–16. doi: 10.1016/j.mce.2006.12.028
Kim, A. C., Reuter, A. L., Zubair, M., Else, T., Serecky, K., Bingham, N. C., et al. (2008). Targeted disruption of beta-catenin in Sf1-expressing cells impairs development and maintenance of the adrenal cortex. Development 135, 2593–2602. doi: 10.1242/dev.021493
King, P., Paul, A., and Laufer, E. (2009). Shh signaling regulates adrenocortical development and identifies progenitors of steroidogenic lineages. Proc. Natl. Acad. Sci. U.S.A. 106, 21185–21190. doi: 10.1073/pnas.0909471106
Kirillov, O. I., and Popova, S. B. (1985). Adrenal cortex of rat: diurnal variation of nuclear volumes and mitotic index of cells in zona fasciculata. Exp. Clin. Endocrinol. 86, 375–337. doi: 10.1055/s-0029-1210514
Kishimoto, W., Hiroi, T., Shiraishi, M., Osada, M., Imaoka, S., Kominami, S., et al. (2004). Cytochrome P450 2D catalyze steroid 21-hydroxylation in the brain. Endocrinology 145, 699–705. doi: 10.1210/en.2003-1109
Koldzic-Zivanovic, N., Tu, H., Juelich, T. L., Rady, P. L., Tyring, S. K., Hudnall, S. D., et al. (2006). Regulation of adrenal glucocorticoid synthesis by interleukin-10: a preponderance of IL-10 receptor in the adrenal zona fasciculata. Brain Behav. Immun. 20, 460–468. doi: 10.1016/j.bbi.2005.09.003
Lai, F. J., Shin, S. J., Lee, Y. J., Lin, S. R., Jou, W. Y., and Tsai, J. H. (2000). Up-regulation of adrenal cortical and medullary atrial natriuretic peptide and gene expression in rats with deoxycorticosterone acetate-salt treatment. Endocrinology 141, 325–332. doi: 10.1210/en.141.1.325
Laird, S. M., Vinson, G. P., and Whitehouse, B. J. (1988). Monoclonal antibodies against rat adrenocortical cell antigens. Acta Endocrinol. 119, 420–426. doi: 10.1530/acta.0.1190420
Lala, D. S., Rice, D. A., and Parker, K. L. (1992). Steroidogenic factor I, a key regulator of steroidogenic enzyme expression, is the mouse homolog of fushi tarazu-factor I. Mol. Endocrinol. 6, 1249–1258.
l'Allemand, D., and Biason-Lauber, A. (2000). Intra-adrenal regulation of androgen synthesis. Eur. J. Clin. Invest. 30(Suppl. 3), 28–33. doi: 10.1046/j.1365-2362.2000.0300s3028.x
Lander, A. D. (2013). How cells know where they are. Science 339, 923–927. doi: 10.1126/science.1224186
Laragh, J. H., Angers, M., Kelly, W. G., and Lieberman, S. (1960). Hypotensive agents and pressor substances. The effect of epinephrine, norepinephrine, angiotensin II, and others on the secretory rate of aldosterone in man. JAMA 174, 234–240. doi: 10.1001/jama.1960.03030030014003
Laredo, J., Hamilton, B. P., and Hamlyn, J. M. (1995). Secretion of endogenous ouabain from bovine adrenocortical cells: role of the zona glomerulosa and zona fasciculata. Biochem. Biophys. Res. Commun. 212, 487–493. doi: 10.1006/bbrc.1995.1996
Lee-Robichaud, P., Wright, J. N., Akhtar, M. E., and Akhtar, M. (1995). Modulation of the activity of human 17 alpha-hydroxylase-17,20-lyase (CYP17) by cytochrome b5: endocrinological and mechanistic implications. Biochem. J. 308, 901–998. doi: 10.1042/bj3080901
Lehoux, J. G., Bird, I. M., Briere, N., Martel, D., and Ducharme, L. (1997). Influence of dietary sodium restriction on angiotensin II receptors in rat adrenals. Endocrinology 138, 5238–5547. doi: 10.1210/en.138.12.5238
Lehoux, J. G., Fleury, A., and Ducharme, L. (1998). The acute and chronic effects of adrenocorticotropin on the levels of messenger ribonucleic acid and protein of steroidogenic enzymes in rat adrenal in vivo. Endocrinology 139, 3913–3922. doi: 10.1210/en.139.9.3913
Leliavski, A., Dumbell, R., Ott, V., and Oster, H. (2015). Adrenal clocks and the role of adrenal hormones in the regulation of circadian physiology. J. Biol. Rhythms 30, 20–34. doi: 10.1177/0748730414553971
Li, D., Mukai, K., Suzuki, T., Suzuki, R., Yamashita, S., Mitani, F., et al. (2007). Adrenocortical zonation factor 1 is a novel matricellular protein promoting integrin-mediated adhesion of adrenocortical and vascular smooth muscle cells. FEBS J. 274, 2506–2522. doi: 10.1111/j.1742-4658.2007.05786.x
Liakos, P., Chambaz, E. M., Feige, J. J., and Defaye, G. (2000). Expression and regulation of melanocortin receptor-5 (MC5-R) in the bovine adrenal cortex. Mol. Cell. Endocrinol. 159, 99–107. doi: 10.1016/S0303-7207(99)00196-3
Lombardo, L. D., and Cortesini, C. (1988). The origin and differentiation of adrenocortical cells in rats with portacaval shunt. A structural and ultrastructural study. Histol. Histopathol. 3, 163–171.
MacDonald, B. T., Tamai, K., and He, X. (2009). Wnt/beta-catenin signaling: components, mechanisms, and diseases. Dev. Cell 17, 9–26. doi: 10.1016/j.devcel.2009.06.016
MacKenzie, S. M., Connell, J. M., and Davies, E. (2012). Non-adrenal synthesis of aldosterone: a reality check. Mol. Cell. Endocrinol. 350, 163–167. doi: 10.1016/j.mce.2011.06.026
Martin, K. O., and Black, V. H. (1983). Effects of age and adrenocorticotropin on microsomal enzymes in guinea pig adrenal inner and outer cortices. Endocrinology 112, 573–559. doi: 10.1210/endo-112-2-573
Martinez, A., Aigueperse, C., Val, P., Dussault, M., Tournaire, C., Berger, M., et al. (2001). Physiological functions and hormonal regulation of mouse vas deferens protein (AKR1B7) in steroidogenic tissues. Chem. Biol. Interact. 130–132, 903–917. doi: 10.1016/S0009-2797(00)00244-1
Mattos, G. E., Jacysyn, J. F., Amarante-Mendes, G. P., and Lotfi, C. F. (2011). Comparative effect of FGF2, synthetic peptides 1-28 N-POMC and ACTH on proliferation in rat adrenal cell primary cultures. Cell Tissue Res. 345, 343–356. doi: 10.1007/s00441-011-1220-8
McEwan, P. C., Lindop, G. B., and Kenyon, C. J. (1996). Control of cell-proliferation in the rat adrenal-gland in-vivo by the renin-angiotensin system. Am. J. Physiol. 34, E192–E198.
McEwan, P. E., Vinson, G. P., and Kenyon, C. J. (1999). Control of adrenal cell proliferation by AT1 receptors in response to angiotensin II and low-sodium diet. Am. J. Physiol. 276, E303–E309.
McKenna, T. J., Fearon, U., Clarke, D., and Cunningham, S. K. (1997). A critical review of the origin and control of adrenal androgens. Baillieres Clin. Obstet. Gynaecol. 11, 229–248. doi: 10.1016/S0950-3552(97)80035-1
McNeill, H., Puddefoot, J. R., and Vinson, G. P. (1998). MAP kinase in the rat adrenal gland. Endocr. Res. 24, 373–380. doi: 10.3109/07435809809032617
McNeill, H., and Vinson, G. P. (2000). Regulation of MAPK activity in response to dietary sodium in the rat adrenal gland. Endocr. Res. 26, 879–883. doi: 10.3109/07435800009048612
Mendonca, P. O., and Lotfi, C. F. (2011). The proliferative effect of synthetic N-POMC(1-28) peptides in rat adrenal cortex: a possible role for cyclin E. Mol. Cell. Endocrinol. 336, 156–161. doi: 10.1016/j.mce.2010.12.012
Min, L., Strushkevich, N. V., Harnastai, I. N., Iwamoto, H., Gilep, A. A., Takemori, H., et al. (2005). Molecular identification of adrenal inner zone antigen as a heme-binding protein. Febs J. 272, 5832–5843. doi: 10.1111/j.1742-4658.2005.04977.x
Min, L., Takemori, H., Nonaka, Y., Katoh, Y., Doi, J., Horike, N., et al. (2004). Characterization of the adrenal-specific antigen IZA (inner zone antigen) and its role in the steroidogenesis. Mol. Cell. Endocrinol. 215, 143–148. doi: 10.1016/j.mce.2003.11.025
Mitani, F., Miyamoto, H., Mukai, K., and Ishimura, Y. (1996). Effects of long-term stimulation of ACTH-secretion and angiotensin- II-secretion on the rat adrenal-cortex. Endocr. Res. 22, 421–431. doi: 10.1080/07435809609043728
Mitani, F., Mukai, K., Miyamoto, H., Suematsu, M., and Ishimura, Y. (1999). Development of functional zonation in the rat adrenal cortex. Endocrinology 140, 3342–3353. doi: 10.1210/en.140.7.3342
Mitani, F., Ogishima, T., Miyamoto, H., and Ishimura, Y. (1995). Localization of p450aldo and p45011-beta in normal and regenerating rat adrenal-cortex. Endocr. Res. 21, 413–423. doi: 10.3109/07435809509030457
Mitani, F., Suzuki, H., Hata, J. I., Ogishima, T., Shimada, H., and Ishimura, Y. (1994). A novel cell layer without corticosteroid-synthesizing enzymes in rat adrenal-cortex - histochemical detection and possible physiological- role. Endocrinology 135, 431–438.
Morley, S. D., Viard, I., Chung, B. C., Ikeda, Y., Parker, K. L., and Mullins, J. J. (1996). Variegated expression of a mouse steroid 21-hydroxylase/beta- galactosidase transgene suggests centripetal migration of adrenocortical cells. Mol. Endocrinol. 10, 585–598.
Morohashi, K., Iida, H., Nomura, M., Hatano, O., Honda, S., Tsukiyama, T., Niwa, O., Hara, T., Takakusu, A., Shibata, Y., et al. (1994). Functional difference between Ad4BP and ELP, and their distributions in steroidogenic tissues. Mol. Endocrinol. 8, 643–653.
Mukai, K., Mitani, F., Nagasawa, H., Suzuki, R., Suzuki, T., Suematsu, M., et al. (2003). An inverse correlation between expression of a preprocathepsin B-related protein with cysteine-rich sequences and steroid 11beta -hydroxylase in adrenocortical cells. J. Biol. Chem. 278, 17084–17092. doi: 10.1074/jbc.M301477200
Mukai, T., Kusaka, M., Kawabe, K., Goto, K., Nawata, H., Fujieda, K., et al. (2002). Sexually dimorphic expression of Dax-1 in the adrenal cortex. Genes Cells 7, 717–729. doi: 10.1046/j.1365-2443.2002.00556.x
Mulrow, P. J. (1999). Angiotensin II and aldosterone regulation. Regul. Pept. 80, 27–32. doi: 10.1016/S0167-0115(99)00004-X
Nakamura, Y., Hornsby, P. J., Casson, P., Morimoto, R., Satoh, F., Xing, Y., et al. (2009). Type 5 17beta-hydroxysteroid dehydrogenase (AKR1C3) contributes to testosterone production in the adrenal reticularis. J. Clin. Endocrinol. Metab. 94, 2192–2198. doi: 10.1210/jc.2008-2374
Ni, H., Mune, T., Morita, H., Daidoh, H., Hanafusa, J., Shibata, T., et al. (1995). Inhibition of aldosterone turn-off phenomenon following chronic adrenocorticotropin treatment with in vivo administration of antiglucocorticoid and antioxidants in rats. Eur. J. Endocrinol. 133, 578–584. doi: 10.1530/eje.0.1330578
Nishimoto, K., Harris, R. B., Rainey, W. E., and Seki, T. (2014). Sodium deficiency regulates rat adrenal zona glomerulosa gene expression. Endocrinology 155, 1363–1372. doi: 10.1210/en.2013-1999
Nishimoto, K., Nakagawa, K., Li, D., Kosaka, T., Oya, M., Mikami, S., et al. (2010). Adrenocortical zonation in humans under normal and pathological conditions. J. Clin. Endocrinol. Metab. 95, 2296–2305. doi: 10.1210/jc.2009-2010
Nishimoto, K., Tomlins, S. A., Kuick, R., Cani, A. K., Giordano, T. J., Hovelson, D. H., et al. (2015). Aldosterone-stimulating somatic gene mutations are common in normal adrenal glands. Proc. Natl. Acad. Sci. U.S.A. 112, E4591–E4599. doi: 10.1073/pnas.1505529112
Nonaka, Y., and Okamoto, M. (1991). Functional expression of the cDNAs encoding rat 11-b-hydroxylase [cytochrome-p450(11-b)] and aldosterone synthase [cytochrome-p450(11-b, aldo)]. Eur. J. Biochem. 202, 897–902. doi: 10.1111/j.1432-1033.1991.tb16449.x
Okamoto, M., and Nonaka, Y. (1992). Molecular-biology of rat steroid 11-b-hydroxylase [p450(11-b)] and aldosterone synthase [p450(11-b, aldo)]. J. Steroid Biochem. Mol. Biol. 41, 415–419. doi: 10.1016/0960-0760(92)90367-R
Oster, H., Damerow, S., Kiessling, S., Jakubcakova, V., Abraham, D., Tian, J., et al. (2006). The circadian rhythm of glucocorticoids is regulated by a gating mechanism residing in the adrenal cortical clock. Cell Metab. 4, 163–173. doi: 10.1016/j.cmet.2006.07.002
Otis, M., Campbell, S., Payet, M. D., and Gallo-Payet, N. (2007). Expression of extracellular matrix proteins and integrins in rat adrenal gland: importance for ACTH-associated functions. J. Endocrinol. 193, 331–347. doi: 10.1677/JOE-07-0055
Otis, M., Campbell, S., Payet, M. D., and Gallo-Payet, N. (2008). In adrenal glomerulosa cells, angiotensin II inhibits proliferation by interfering with fibronectin-integrin signaling. Endocrinology 149, 3435–3445. doi: 10.1210/en.2008-0282
Pabon, J. E., Li, X., Lei, Z. M., Sanfilippo, J. S., Yussman, M. A., and Rao, C. V. (1996). Novel presence of luteinizing hormone/chorionic gonadotropin receptors in human adrenal glands. J. Clin. Endocrinol. Metab. 81, 2397–2400.
Parker, K. L., and Schimmer, B. P. (1993). Transcriptional regulation of the adrenal steroidogenic enzymes. Trends Endocrinol. Metab. 4, 46–50. doi: 10.1016/S1043-2760(05)80014-1
Parker, K. L., Ikeda, Y., and Luo, X. (1995). The nuclear receptor sf-1 acts at multiple levels of endocrine development. Mol. Biol. Cell 6:1344.
Paust, H. J., Loeper, S., Else, T., Bamberger, A. M., Papadopoulos, G., Pankoke, D., et al. (2006). Expression of the glucocorticoid receptor in the human adrenal cortex. Exp. Clin. Endocrinol. Diabetes 114, 6–10. doi: 10.1055/s-2005-873007
Payet, N., Lehoux, J. G., and Isler, H. (1980). Effect of ACTH on the proliferative and secretory activities of the adrenal glomerulosa. Acta Endocr. 93, 365–374. doi: 10.1530/acta.0.0930365
Pellerin, S., Keramidas, M., Chambaz, E. M., and Feige, J. J. (1997). Expression of laminin and its possible role in adrenal cortex homeostasis. Endocrinology 138, 1321–1137. doi: 10.1210/en.138.3.1321
Peters, B., Clausmeyer, S., Obermuller, N., Woyth, A., Kranzlin, B., Gretz, N., et al. (1998). Specific regulation of StAR expression in the rat adrenal zona glomerulosa. an in situ hybridization study. J. Histochem. Cytochem. 46, 1215–1221. doi: 10.1177/002215549804601101
Peters, B., Teubner, P., Clausmeyer, S., Puschner, T., Maser-Gluth, C., Wrede, H. J., et al. (2007). StAR expression and the long-term aldosterone response to high-potassium diet in Wistar-Kyoto and spontaneously hypertensive rats. Am. J. Physiol. Endocrinol. Metab. 292, E16–E23. doi: 10.1152/ajpendo.00454.2005
Petrovic-Kosanovic, D., Ajdzanovic, V., Cakic-Milosevic, M., Koko, V., and Milosevic, V. (2013). The effects of acute heat stress on proliferative and apoptotic processes in the rat adrenal cortex. Arch. Biol. Sci. 65, 905–909. doi: 10.2298/ABS1303905K
Pignatelli, D., Bento, M. J., Maia, M., Magalhaes, M. M., Magalhaes, M. C., and Mason, J. I. (1999). Ontogeny of 3beta-hydroxysteroid dehydrogenase expression in the rat adrenal gland as studied by immunohistochemistry. Endocr. Res. 25, 21–27. doi: 10.1080/07435809909066126
Pignatelli, D., Xiao, F., Gouveia, A. M., Ferreira, J. G., and Vinson, G. P. (2006). Adrenarche in the rat. J. Endocrinol. 191, 301–308. doi: 10.1677/joe.1.06972
Pihlajoki, M., Dorner, J., Cochran, R. S., Heikinheimo, M., and Wilson, D. B. (2015). Adrenocortical zonation, renewal, and remodeling. Front. Endocrinol. 6:27. doi: 10.3389/fendo.2015.00027
Pihlajoki, M., Gretzinger, E., Cochran, R., Kyronlahti, A., Schrade, A., Hiller, T., et al. (2013). Conditional mutagenesis of Gata6 in SF1-positive cells causes gonadal-like differentiation in the adrenal cortex of mice. Endocrinology 154, 1754–1767. doi: 10.1210/en.2012-1892
Rabl, H. (1891). Die Entwicklung und Structur der Nebennieren bei den Vögeln. Archiv für mikroskopische Anatomie 38, 492–523. doi: 10.1007/BF02954398
Rainey, W. E., and Nakamura, Y. (2008). Regulation of the adrenal androgen biosynthesis. J. Steroid Biochem. Mol. Biol. 108, 281–286. doi: 10.1016/j.jsbmb.2007.09.015
Rainey, W. E., Carr, B. R., Sasano, H., Suzuki, T., and Mason, J. I. (2002). Dissecting human adrenal androgen production. Trends Endocrinol. Metab. 13, 234–239. doi: 10.1016/S1043-2760(02)00609-4
Rao, C. V. (2010). Human adrenal LH/hCG receptors and what they could mean for adrenal physiology and pathology. Mol. Cell. Endocrinol. 329, 33–36. doi: 10.1016/j.mce.2010.05.012
Raven, P. W., McCredie, E., McAuley, M., and Vinson, G. P. (1983). Origins of the differences in function of rat adrenal zona glomerulosa cells incubated as intact tissue and as collagenase-prepared cell suspensions. Cell Biochem. Funct. 1, 17–24. doi: 10.1002/cbf.290010104
Raza, F. S., Okamoto, M., Takemori, H., and Vinson, G. P. (2005). Manganese superoxide dismutase activity in the rat adrenal. J. Endocrinol. 184, 77–84. doi: 10.1677/joe.1.05755
Raza, F. S., Puddefoot, J. R., and Vinson, G. P. (1998). Pref-1, SF-1 and adrenocortical zonation. Endocr. Res. 24, 977–981. doi: 10.3109/07435809809032720
Raza, F. S., Takemori, H., Tojo, H., Okamoto, M., and Vinson, G. P. (2001). Identification of the rat adrenal zona fasciculata/reticularis specific protein, inner zone antigen (IZAg), as the putative membrane progesterone receptor. Eur. J. Biochem. 268, 2141–2147. doi: 10.1046/j.1432-1327.2001.02096.x
Raza, F. S., and Vinson, G. P. (2000). Adrenocortical expression of MnSOD. Endocr. Res. 26, 959–963. doi: 10.3109/07435800009048623
Reddi, A. H. (1998). Role of morphogenetic proteins in skeletal tissue engineering and regeneration. Nat. Biotechnol. 16, 247–252. doi: 10.1038/nbt0398-247
Rege, J., Nakamura, Y., Wang, T., Merchen, T. D., Sasano, H., and Rainey, W. E. (2014). Transcriptome profiling reveals differentially expressed transcripts between the human adrenal zona fasciculata and zona reticularis. J. Clin. Endocrinol. Metab. 99, E518–E527. doi: 10.1210/jc.2013-3198
Rege, J., Nishimoto, H. K., Nishimoto, K., Rodgers, R. J., Auchus, R. J., and Rainey, W. E. (2015). Bone Morphogenetic Protein-4 (BMP4): a paracrine regulator of human adrenal C19 steroid synthesis. Endocrinology 156, 2530–2540. doi: 10.1210/en.2014-1942
Rendon, N. M., Rudolph, L. M., Sengelaub, D. R., and Demas, G. E. (2015). The agonistic adrenal: melatonin elicits female aggression via regulation of adrenal androgens. Proc. Biol. Sci. 282:20152080. doi: 10.1098/rspb.2015.2080
Renshaw, D., and Hinson, J. P. (2001). Neuropeptide Y and the adrenal gland: a review. Peptides. 22, 429–438. doi: 10.1016/S0196-9781(01)00353-9
Romero, D. G., Yanes, L. L., de Rodriguez, A. F., Plonczynski, M. W., Welsh, B. L., Reckelhoff, J. F., et al. (2007). Disabled-2 is expressed in adrenal zona glomerulosa and is involved in aldosterone secretion. Endocrinology 148, 2644–2652. doi: 10.1210/en.2006-1509
Roskelley, C. D., and Auersperg, N. (1990). Density separation of rat adrenocortical cells: morphology, steroidogenesis, and P-450scc expression in primary culture. In.Vitro.Cell Dev. Biol. 26, 493–501. doi: 10.1007/BF02624091
Rucinski, M., Porzionato, A., Ziolkowska, A., Szyszka, M., Macchi, V., De Caro, R., et al. (2010). Expression of the spexin gene in the rat adrenal gland and evidences suggesting that spexin inhibits adrenocortical cell proliferation. Peptides 31, 676–682. doi: 10.1016/j.peptides.2009.12.025
Sahut-Barnola, I., de Joussineau, C., Val, P., Lambert-Langlais, S., Damon, C., Lefrancois-Martinez, A. M., et al. (2010). Cushing's syndrome and fetal features resurgence in adrenal cortex-specific Prkar1a knockout mice. PLoS Genet. 6:e1000980. doi: 10.1371/journal.pgen.1000980
Sahut-Barnola, I., Lefrancois-Martinez, A. M., Jean, C., Veyssiere, G., and Martinez, A. (2000). Adrenal tumorigenesis targeted by the corticotropin-regulated promoter of the aldo-keto reductase AKR1B7 gene in transgenic mice. Endocr. Res. 26, 885–898. doi: 10.3109/07435800009048613
Scheys, J. O., Heaton, J. H., and Hammer, G. D. (2011). Evidence of adrenal failure in aging Dax1-deficient mice. Endocrinology 152, 3430–3439. doi: 10.1210/en.2010-0986
Schilling, T. F., Nie, Q., and Lander, A. D. (2012). Dynamics and precision in retinoic acid morphogen gradients. Curr. Opin. Genet. Dev. 22, 562–569. doi: 10.1016/j.gde.2012.11.012
Selye, H. (1946). The general adaptation syndrome and the diseases of adaptation. J. Clin. Endocrinol. Metab. 6, 117–230. doi: 10.1210/jcem-6-2-117
Selye, H., and Jensen, H. (1946). The chemistry of the hormones. Ann. Rev. Biochem. 15, 347–360. doi: 10.1146/annurev.bi.15.070146.002023
Shenker, Y., Villareal, J. Z., Sider, R. S., and Grekin, R. J. (1985). Alpha-melanocyte-stimulating hormone stimulation of aldosterone secretion in hypophysectomized rats. Endocrinology 116, 138–141. doi: 10.1210/endo-116-1-138
Shepherd, R. M., Fraser, R., Nichols, D. J., and Kenyon, C. J. (1991). Efflux of potassium-ions in angiotensin-II-stimulated bovine adrenocortical-cells. J. Endocrinol. 128, 297–304. doi: 10.1677/joe.0.1280297
Shimasaki, S., Moore, R. K., Otsuka, F., and Erickson, G. F. (2004). The bone morphogenetic protein system in mammalian reproduction. Endocr. Rev. 25, 72–101. doi: 10.1210/er.2003-0007
Shimojo, M., Whorwood, C. B., and Stewart, P. M. (1996). 11 beta-Hydroxysteroid dehydrogenase in the rat adrenal. J. Mol. Endocrinol. 17, 121–130. doi: 10.1677/jme.0.0170121
Simon, D. P., and Hammer, G. D. (2012). Adrenocortical stem and progenitor cells: implications for adrenocortical carcinoma. Mol. Cell. Endocrinol. 351, 2–11. doi: 10.1016/j.mce.2011.12.006
Smith, R. E., Li, K. X., Andrews, R. K., and Krozowski, Z. (1997). Immunohistochemical and molecular characterization of the rat 11 beta-hydroxysteroid dehydrogenase type II enzyme. Endocrinology 138, 540–547.
Soucy, P., and Luu-The, V. (2002). Assessment of the ability of type 2 cytochrome b5 to modulate 17,20-lyase activity of human P450c17. J. Steroid Biochem. Mol. Biol. 80, 71–75. doi: 10.1016/S0960-0760(01)00171-6
Spangelo, B. L., Judd, A. M., Call, G. B., Zumwalt, J., and Gorospe, W. C. (1995). Role of the cytokines in the hypothalamic-pituitary-adrenal and gonadal axes. Neuroimmunomodulation 2, 299–312. doi: 10.1159/000097209
Suto, J. (2012). Quantitative trait locus mapping of genes associated with vacuolation in the adrenal X-zone of the DDD/Sgn inbred mouse. BMC Genet. 13:95. doi: 10.1186/1471-2156-13-95
Tait, S. A., Schulster, D., Okamoto, M., Flood, C., and Tait, J. F. (1970). Production of steroids by in vitro superfusion of endocrine tissue. II. steroid output from bisected whole, capsular and decapsulated adrenals of normal intact, hypophysectomized and hypophysectomized-nephrectomized rats as a function of time of superfusion. Endocrinology 86, 360–382. doi: 10.1210/endo-86-2-360
Tanaka, S., Kuwahara, S., Nishijima, K., Ohno, T., and Matsuzawa, A. (2006). Genetic association of mutation at agouti locus with adrenal x zone morphology in BALB/c mice. Exp. Anim. 55, 343–347. doi: 10.1538/expanim.55.343
Tanaka, S., Nishimura, M., and Matsuzawa, A. (1994). Genetic association between agouti locus and adrenal X zone morphology in SM/J mice. Acta Anat. 149, 170–113. doi: 10.1159/000147573
Taves, M. D., Gomez-Sanchez, C. E., and Soma, K. K. (2011). Extra-adrenal glucocorticoids and mineralocorticoids: evidence for local synthesis, regulation, and function. Am. J. Physiol. Endocrinol. Metab. 301, E11–E24. doi: 10.1152/ajpendo.00100.2011
Tevosian, S. G., Jimenez, E., Hatch, H. M., Jiang, T., Morse, D. A., Fox, S. C., et al. (2015). Adrenal development in mice requires GATA4 and GATA6 transcription factors. Endocrinology 156, 2503–2517. doi: 10.1210/en.2014-1815
Thomas, M., Keramidas, M., Monchaux, E., and Feige, J. J. (2004). Dual hormonal regulation of endocrine tissue mass and vasculature by adrenocorticotropin in the adrenal cortex. Endocrinology 145, 4320–4329. doi: 10.1210/en.2004-0179
Thomas, R., Anderson, W. A., Raman, V., and Reddi, A. H. (1998). Androgen-dependent gene expression of bone morphogenetic protein 7 in mouse prostate. Prostate 37, 236–245.
Tickle, C. (2006). Making digit patterns in the vertebrate limb. Nat. Rev. Mol. Cell. Biol. 7, 45–53. doi: 10.1038/nrm1830
Topor, L. S., Asai, M., Dunn, J., and Majzoub, J. A. (2011). Cortisol stimulates secretion of dehydroepiandrosterone in human adrenocortical cells through inhibition of 3betaHSD2. J. Clin. Endocrinol. Metab. 96, E31–E39. doi: 10.1210/jc.2010-0692
Torda, T., Cruciani, R. A., and Saavedra, J. M. (1988). Localization of neuropeptide Y binding sites in the zona glomerulosa of the bovine adrenal gland. Neuroendocrinology 48, 207–210. doi: 10.1159/000125010
Torres, T. E., de Mendonca, P. O., and Lotfi, C. F. (2010). Synthetic modified N-POMC(1-28) controls in vivo proliferation and blocks apoptosis in rat adrenal cortex. Cell Tissue Res. 341, 239–250. doi: 10.1007/s00441-010-0998-0
Tremblay, A., Parker, K. L., and Lehoux, J. G. (1992). Dietary potassium supplementation and sodium restriction stimulate aldosterone synthase but not 11 beta-hydroxylase P-450 messenger ribonucleic acid accumulation in rat adrenals and require angiotensin II production. Endocrinology 130, 3152–3318.
Tsuji, K., Bandyopadhyay, A., Harfe, B. D., Cox, K., Kakar, S., Gerstenfeld, L., et al. (2006). BMP2 activity, although dispensable for bone formation, is required for the initiation of fracture healing. Nat. Genet. 38, 1424–1429. doi: 10.1038/ng1916
Tyczewska, M., Rucinski, M., Ziolkowska, A., Szyszka, M., Trejter, M., Hochol-Molenda, A., et al. (2014). Enucleation-induced rat adrenal gland regeneration: expression profile of selected genes involved in control of adrenocortical cell proliferation. Int. J. Endocrinol. 2014:130359. doi: 10.1155/2014/130359
Ulrich-Lai, Y. M., Figueiredo, H. F., Ostrander, M. M., Choi, D. C., Engeland, W. C., and Herman, J. P. (2006). Chronic stress induces adrenal hyperplasia and hypertrophy in a subregion-specific manner. Am. J. Physiol. Endocrinol. Metab. 291, E965–E973. doi: 10.1152/ajpendo.00070.2006
Urist, M. R. (1965). Bone: formation by autoinduction. Science 150, 893–899. doi: 10.1126/science.150.3698.893
Uruno, A., Matsuda, K., Noguchi, N., Yoshikawa, T., Kudo, M., Satoh, F., et al. (2011). Peroxisome proliferator-activated receptor- suppresses CYP11B2 expression and aldosterone production. J. Mol. Endocrinol. 46, 37–49. doi: 10.1677/JME-10-0088
van der Kraan, M., Adan, R. A., Entwistle, M. L., Gispen, W. H., Burbach, J. P., and Tatro, J. B. (1998). Expression of melanocortin-5 receptor in secretory epithelia supports a functional role in exocrine and endocrine glands. Endocrinology 139, 2348–2355. doi: 10.1210/en.139.5.2348
Vendeira, P., Pignatelli, D., Neves, D., Magalhaes, M. M., Magalhaes, M. C., and Vinson, G. P. (1999). Effects of prolonged infusion of basic fibroblast growth factor and IGF-I on adrenocortical differentiation in the autotransplanted adrenal: an immunohistochemical study. J. Endocrinol. 162, 21–29. doi: 10.1677/joe.0.1620021
Vines, H. W. C. (1938). “The adreno-genital syndrome: some histological observations,”in The Adrenal Cortex and Intersexuality, eds L. R. Broster, C. Allen, H. W. C. Vines, J. Patterson, A. W. Greenwood, G. F. Marrian, and G. C. Butler (London: Chapman and Hall), 137–197.
Vinson, G. P. (2003). Adrenocortical zonation and ACTH. Microsc. Res. Tech. 61, 227–239. doi: 10.1002/jemt.10331
Vinson, G. P. (2004). Glomerulosa function and aldosterone synthesis in the rat. Mol. Cell. Endocrinol. 217, 59–65. doi: 10.1016/j.mce.2003.10.010
Vinson, G. P., Firth, K. M., McNeill, H. M., and Puddefoot, J. R. (2000). “Differentiation of function in the zones of the rat adrenal cortex,” in Molecular Steroidogenesis, eds M. Okamoto, Y. Ishimura, and H. Nawata (Tokyo: Universal Academy Press, Inc.), 205–208.
Vinson, G. P., and Hinson, J. P. (1992). “Blood flow and hormone secretion in the adrenal gland,” in The Adrenal Gland, 2nd Edn., ed V. H. T. James (New York, NY: Raven Press), 71–86.
Vinson, G. P., Hinson, J. P., and Raven, P. W. (1985a). The relationship between tissue preparation and function; methods for the study of control of aldosterone secretion: a review. Cell. Biochem. Funct. 3, 235–253. doi: 10.1002/cbf.290030402
Vinson, G. P., Hinson, J. P., and Toth, I. E. (1994). The neuroendocrinology of the adrenal cortex. J. Neuroendocr. 6, 235–246. doi: 10.1111/j.1365-2826.1994.tb00578.x
Vinson, G. P., and Ho, M. M. (1998). Origins of zonation: the adrenocortical model of tissue development and differentiation. Clin. Exp. Pharmacol. Physiol. 25, S91–S96. doi: 10.1111/j.1440-1681.1998.tb02308.x
Vinson, G. P., Ho, M. M., and Puddefoot, J. R. (1998). Adrenocortical zonation and the adrenal renin-angiotensin system. Endocr. Res. 24, 677–686. doi: 10.3109/07435809809032669
Vinson, G. P., Laird, S. M., Hinson, J. P., and Teja, R. (1992a). Origin of aldosterone in trypsin-stimulated rat adrenal zona glomerulosa incubations. J. Endocrinol. 135, 125–133. doi: 10.1677/joe.0.1350125
Vinson, G. P., Pudney, J. A., and Whitehouse, B. J. (1985b). The mammalian adrenal circulation and the relationship between adrenal blood flow and steroidogenesis. J. Endocrinol. 105, 285–294. doi: 10.1677/joe.0.1050285
Vinson, G. P., Teja, R., Ho, M. M., and Puddefoot, J. R. (1995). A two cell type theory for aldosterone biosynthesis: the roles of 11b-hydroxylase and aldosterone synthase, and a high capacity, tightly binding steroid carrier (TBSC) for 18-hydroxydeoxycorticosterone. J. Endocrinol. 144, 359–368. doi: 10.1677/joe.0.1440359
Vinson, G. P., Whitehouse, B. J., Dell, A., Etienne, T., and Morris, H. R. (1980). Characterisation of an adrenal zona glomerulosa stimulating component of posterior pituitary extracts as a-MSH. Nature 284, 464–467. doi: 10.1038/284464a0
Vinson, G. P., Whitehouse, B. J., and Hinson, J. P. (1992b). The Adrenal Cortex. Englewood Heights, NJ: Prentice-Hall.
Walczak, E. M., and Hammer, G. D. (2015). Regulation of the adrenocortical stem cell niche: implications for disease. Nat. Rev. Endocrinol. 11, 14–28. doi: 10.1038/nrendo.2014.166
White, R. J., Nie, Q., Lander, A. D., and Schilling, T. F. (2007). Complex regulation of cyp26a1 creates a robust retinoic acid gradient in the zebrafish embryo. PLoS Biol. 5:e304. doi: 10.1371/journal.pbio.0050304
Whitworth, E. J., Kosti, O., Renshaw, D., and Hinson, J. P. (2003). Adrenal neuropeptides: regulation and interaction with ACTH and other adrenal regulators. Microsc. Res. Tech. 61, 259–267. doi: 10.1002/jemt.10335
Whitworth, E., and Vinson, G. P. (2000). Zonal differentiation in the rat adrenal cortex. Endocr. Res. 26, 973–998. doi: 10.3109/07435800009048625
Wiesel, J. (1902). Beiträge zur Anatomie und Entwickelung der menschlichen Nebenniere. Anatomische Hefte 19, 483–522. doi: 10.1007/BF02298163
Willenberg, H. S., Schinner, S., and Ansurudeen, I. (2008). New mechanisms to control aldosterone synthesis. Horm. Metab. Res. 40, 435–441. doi: 10.1055/s-2008-1065336
Williams, G. H. (2005). Aldosterone biosynthesis, regulation, and classical mechanism of action. Heart Fail. Rev. 10, 7–13. doi: 10.1007/s10741-005-2343-3
Wolkersdorfer, G. W., and Bornstein, S. R. (1998). Tissue remodelling in the adrenal gland. Biochem. Pharmacol. 56, 163–171. doi: 10.1016/S0006-2952(98)00067-7
Wood, M. A., and Hammer, G. D. (2010). Adrenocortical stem and progenitor cells: unifying model of two proposed origins. Mol. Cell. Endocrinol. 336, 206–212. doi: 10.1016/j.mce.2010.11.012
Woods, A. M., and Judd, A. M. (2008). Interleukin-4 increases cortisol release and decreases adrenal androgen release from bovine adrenal cells. Domes.t Anim. Endocrinol. 34, 372–382. doi: 10.1016/j.domaniend.2007.10.004
Wright, N. A. (1971). Cell proliferation in the prepubertal male rat adrenal cortex: an autoradiographic study. J. Endocrinol. 49, 599–609. doi: 10.1677/joe.0.0490599
Wright, N. A., Voncina, D., and Morley, A. R. (1973). An attempt to demonstrate cell migration from the zona glomerulosa in the prepubertal male rat adrenal cortex. J. Endocrinol. 59, 451–459. doi: 10.1677/joe.0.0590451
Xing, Y., Lerario, A. M., Rainey, W., and Hammer, G. D. (2015). Development of adrenal cortex zonation. Endocrinol. Metab. Clin. North. Am. 44, 243–274. doi: 10.1016/j.ecl.2015.02.001
Xu, J., Zeng, C., Chu, W., Pan, F., Rothfuss, J. M., Zhang, F., et al. (2011). Identification of the PGRMC1 protein complex as the putative sigma-2 receptor binding site. Nat. Commun. 2, 380. doi: 10.1038/ncomms1386
Yagci, A., Oertle, M., Seiler, H., Schmid, D., Campofranco, C., and Muller, J. (1996). Potassium induces multiple steroidogenic enzymes in cultured rat zona glomerulosa cells. Endocrinology 137, 2406–2214. doi: 10.1210/en.137.6.2406
Yamaguchi, T., Naito, Z., Stoner, G. D., Franco Saenz, R., and Mulrow, P. J. (1990). Role of the adrenal renin-angiotensin system on adrenocorticotropic hormone- and potassium-stimulated aldosterone production by rat adrenal glomerulosa cells in monolayer culture. Hypertension 16, 635–641. doi: 10.1161/01.HYP.16.6.635
Yang, Y. K., Thompson, D. A., Dickinson, C. J., Wilken, J., Barsh, G. S., Kent, S. B., et al. (1999). Characterization of Agouti-related protein binding to melanocortin receptors. Mol. Endocrinol. 13, 148–155. doi: 10.1210/mend.13.1.0223
Yaswen, L., Diehl, N., Brennan, M. B., and Hochgeschwender, U. (1999). Obesity in the mouse model of pro-opiomelanocortin deficiency responds to peripheral melanocortin. Nat. Med. 5, 1066–1070. doi: 10.1038/12506
Ye, P., Nakamura, Y., Lalli, E., and Rainey, W. E. (2009). Differential effects of high and low steroidogenic factor-1 expression on CYP11B2 expression and aldosterone production in adrenocortical cells. Endocrinology 150, 1303–1309. doi: 10.1210/en.2008-0667
Yu, L., Han, M., Yan, M., Lee, J., and Muneoka, K. (2012). BMP2 induces segment-specific skeletal regeneration from digit and limb amputations by establishing a new endochondral ossification center. Dev. Biol. 372, 263–273. doi: 10.1016/j.ydbio.2012.09.021
Yu, R. N., Ito, M., and Jameson, J. L. (1998). The murine Dax-1 promoter is stimulated by SF-1 (steroidogenic factor-1) and inhibited by COUP-TF (chicken ovalbumin upstream promoter-transcription factor) via a composite nuclear receptor-regulatory element. Mol. Endocrinol. 12, 1010–1022. doi: 10.1210/mend.12.7.0131
Yuan, B. B., Tchao, R., Funae, Y., Voigt, J. M., and Colby, H. D. (1998). Effects of ACTH administration on zonation of the guinea pig adrenal cortex. Mol. Cell. Endocrinol. 146, 129–136. doi: 10.1016/S0303-7207(98)00189-0
Yuan, B. B., Tchao, R., Voigt, J. M., and Colby, H. D. (2001). Maturational changes in CYP2D16 expression and xenobiotic metabolism in adrenal glands from male and female guinea pigs. Drug metab. Dispos. 29, 194–119.
Zajicek, G., Ariel, I., and Arber, N. (1986). The streaming adrenal cortex: direct evidence of centripetal migration of adrenocytes by estimation of cell turnover rate. J. Endocrinol. 111, 477–482. doi: 10.1677/joe.0.1110477
Zeng, C., Garg, N., and Mach, R. H. (2016). The PGRMC1 protein level correlates with the binding activity of a sigma-2 fluorescent probe (SW120) in rat brain cells. Mol. Imaging Biol. 18, 172–179. doi: 10.1007/s11307-015-0891-z
Zhang, L., Radtke, K., Zheng, L., Cai, A. Q., Schilling, T. F., and Nie, Q. (2012). Noise drives sharpening of gene expression boundaries in the zebrafish hindbrain. Mol. Syst. Biol. 8:613. doi: 10.1038/msb.2012.45
Zhou, J., Shaikh, L. H., Neogi, S. G., McFarlane, I., Zhao, W., Figg, N., et al. (2015). DACH1, a zona glomerulosa selective gene in the human adrenal, activates transforming growth factor-beta signaling and suppresses aldosterone secretion. Hypertension 65, 1103–1110. doi: 10.1161/HYP.0000000000000025
Keywords: glomerulosa, fasciculata, reticularis, X-Zone, cell proliferation, cell migration, cortisol, aldosterone
Citation: Vinson GP (2016) Functional Zonation of the Adult Mammalian Adrenal Cortex. Front. Neurosci. 10:238. doi: 10.3389/fnins.2016.00238
Received: 22 February 2016; Accepted: 17 May 2016;
Published: 15 June 2016.
Edited by:
Nicole Gallo-Payet, University of Sherbrooke, CanadaReviewed by:
William Engeland, University of Minnesota, USAGary Hammer, University of Michigan, USA
Celso E. Gomez-Sanchez, University of Mississippi Medical Center, USA
Copyright © 2016 Vinson. This is an open-access article distributed under the terms of the Creative Commons Attribution License (CC BY). The use, distribution or reproduction in other forums is permitted, provided the original author(s) or licensor are credited and that the original publication in this journal is cited, in accordance with accepted academic practice. No use, distribution or reproduction is permitted which does not comply with these terms.
*Correspondence: Gavin P. Vinson, Zy5wLnZpbnNvbkBxbXVsLmFjLnVr