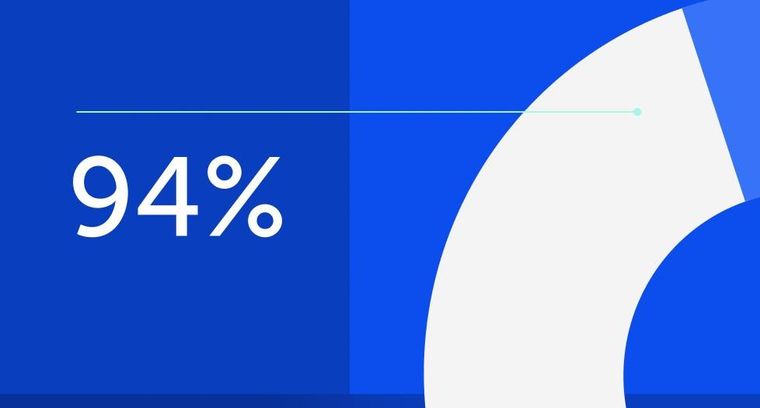
94% of researchers rate our articles as excellent or good
Learn more about the work of our research integrity team to safeguard the quality of each article we publish.
Find out more
MINI REVIEW article
Front. Neurosci., 03 May 2016
Sec. Neurogenesis
Volume 10 - 2016 | https://doi.org/10.3389/fnins.2016.00178
This article is part of the Research Topic50th Anniversary of Adult Neurogenesis: Olfaction, hippocampus and beyondView all 23 articles
New neurons incorporate into the granular cell layer of the dentate gyrus throughout life. Neurogenesis is modulated by behavior and plays a major role in hippocampal plasticity. Along with older mature neurons, new neurons structure the dentate gyrus, and determine its function. Recent data suggest that the level of hippocampal neurogenesis is substantial in the human brain, suggesting that neurogenesis may have important implications for human cognition. In support of that, impaired neurogenesis compromises hippocampal function and plays a role in cognitive deficits in Alzheimer's disease mouse models. We review current work suggesting that neuronal differentiation is defective in Alzheimer's disease, leading to dysfunction of the dentate gyrus. Additionally, alterations in critical signals regulating neurogenesis, such as presenilin-1, Notch 1, soluble amyloid precursor protein, CREB, and β-catenin underlie dysfunctional neurogenesis in Alzheimer's disease. Lastly, we discuss the detectability of neurogenesis in the live mouse and human brain, as well as the therapeutic implications of enhancing neurogenesis for the treatment of cognitive deficits and Alzheimer's disease.
In early development neurons are rapidly produced to form the intricate complexity of the brain and peripheral nervous system. Postnatally, the role of neurogenesis is shifted from brain development into brain plasticity. From then on, neurogenesis takes place only in specific niches in the adult brain, in the subgranular zone (SGZ) of the dentate gyrus (DG) of the hippocampus and the subventricular zone (Kempermann et al., 2015). Recent evidence suggests substantial levels of hippocampal neurogenesis in the human brain, estimating about 700 new neurons a day in the DG (Spalding et al., 2013). Humans replace ~35% of the DG, while rodents are estimated to replace only 10% (Ninkovic and Gotz, 2007; Imayoshi et al., 2008). Recent information also suggests that in humans, the striatum may be a source of adult neurogenesis as well (Ernst et al., 2014). The existence of adult neurogenesis in the human brain supports the notion that neurogenesis has important functional significance and implications for cognitive disorders and their therapy (Eriksson et al., 1998; Ninkovic and Gotz, 2007; Imayoshi et al., 2008; Lazarov and Marr, 2013; Spalding et al., 2013).
The circuitry of the DG, of which new neurons are part, promotes several important functions, namely, pattern separation, conjunctive encoding of multiple sensory output to the dorsal CA3, facilitation of encoding of spatial information based on its output to the dorsal CA3, and encoding of time in new memories (for review, Lazarov and Hollands, 2016). In support of the role of hippocampal neurogenesis in plasticity, learning and memory, increasing evidence suggests that cognitive deficits, difficulty learning new information and memory loss, as occurs in Alzheimer's disease (AD), may be, at least in part, due to impairments in adult neurogenesis (Demars et al., 2010, 2013; Lazarov and Marr, 2010; Lazarov et al., 2010). Some of the foundation for the association between impairments in adult hippocampal neurogenesis and cognitive deficits leading to AD might be due to the fact that several key signals implicated in AD play a role in regulation of hippocampal neurogenesis (Figure 1).
Figure 1. Common mechanisms of neurogenesis and Alzheimer's disease and the implications for learning. (A) Signals that play a role in neurogenesis, such as Notch-1, Wnt/β-catenin, CREB, sAPP, tau, and presenilin-1 are implicated in Alzheimer's disease. (B) Following learning, changes in the neurogenic niche include alterations in Notch and sAPP, increased expression of neurotrophins such as VEGF, BDNF, and IGF which enhance angiogenesis and provide support for the neurogenic niche and lead to increased neurogenesis. Upregulation of CREB signaling by neural progenitor cells and neurons may promote survival and maturation of NPCs. Increased dendritic branching of mature neurons and synaptic plasticity may be mediated by presenilin-1 and APP. The factors mediating these processes are dysfunctional or compromised in Alzheimer's disease, suggesting that defective neurogenesis may affect hippocampal function in Alzheimer's disease.
In the rodent brain, neurogenesis is dramatically decreased during adulthood and further declines during aging (Demars et al., 2013). Recent evidence suggests that in wild type mice reduced proliferation of neural progenitor cells (NPCs) might be one of the processes underlying this phenomenon (Demars et al., 2013). However, other mechanisms, such as altered signaling, increased quiescence of neural stem cells (NSCs) and differentiation toward non-neuronal subtypes have been proposed [see for example Hattiangady and Shetty, 2008]. In humans, the dynamics are less clear. A recent study suggests that there is a moderate decline in neurogenesis with aging (Spalding et al., 2013). However, as of yet, it is unclear how this decline impacts cognitive function in humans or whether similar memory paradigms are regulated by adult neurogenesis as they are in rodents. Observations in humans using high resolution fMRI (Brickman et al., 2014) and cognitive studies (Toner et al., 2009; Stark et al., 2010; Yassa et al., 2011; Brickman et al., 2012) suggest that age-related memory loss begins in the DG. These changes are believed to stem from a decline in the support of the neurogenic niche as well as intrinsic characteristics of NSC (for review Silva-Vargas et al., 2013). Many processes decline in the aging brain along with a decrease in adult neurogenesis. For example, in both rodents and humans the density of synaptic contacts onto granular cells in the DG decreases with age (Flood et al., 1996; Geinisman et al., 2001, 2004). It will be important to determine whether age-dependent decline in neurogenesis compromises the function of the DG and induces susceptibility to memory impairments.
Deficits in adult neurogenesis with age may compromise the structure and function of the entorhinal-hippocampal circuit. This area is particularly vulnerable and heavily affected in AD, the most common form of dementia. AD is characterized by progressive memory loss and cognitive dysfunction (Baulac et al., 2003). Rare, Familail AD (FAD) is caused by mutations in the amyloid precursor protein (APP) and presenilin 1 and 2 (PS1,2) (Selkoe and Wolfe, 2007). However, the majority of AD cases are sporadic and aging is the greatest risk factor for AD. Research done in mouse models of FAD suggests that declining neurogenesis is an early stage event that can be observed as early as 2–3 months of age (Rodriguez et al., 2008; Demars et al., 2010; Hamilton et al., 2010)(for review Lazarov and Marr, 2010, 2013). Nevertheless, it is important to note that some FAD mouse lines, mostly lines that overexpress APP, exhibit enhanced, rather than reduced, neurogenesis (Jin et al., 2004; Chuang, 2010). As discussed below, this might be attributed to the overexpression of soluble APP (sAPP), a proliferation factor of NPCs (Demars et al., 2011, 2013; Lazarov and Demars, 2012). The manifestations of neurogenic impairments in FAD mice are diverse. They include defective maturation/reduced rate of survival of new neurons in the granular cell layer (GCL), compromised dendritic tree branching (Sun et al., 2009; Bonds et al., 2015), imbalance of GABAergic and glutamatergic input onto new granular neurons (Sun et al., 2009), expression of the less potent proliferation factor sAPPβ at the expense of sAPPα in the neurogenic niche (Demars et al., 2011, 2013) and loss of γ-secretase function in NPCs and new neurons (Gadadhar et al., 2011; Bonds et al., 2015).
Below, we highlight several key signaling factors that are implicated in AD and were recently described to regulate neurogenesis. These factors play a role in aging-dependent behavior, circadian rhythm, inflammation, oxidative stress, neurotrophic signaling, hormonal signaling, neurotransmission, vascular signaling, and others. Thus, the multi- factorial effect on neurogenesis exposes the complex relationship between neurogenesis and the progression of AD pathology (for review Lazarov and Marr, 2010, 2013; Lazarov et al., 2010).
Presenillin-1 (PS1) is the catalytic core of γ-secretase, an aspartyl protease, which cleaves numerous substrates, including APP and Notch (De Strooper et al., 1998, 1999). Mutations in PS1 cause FAD, presumably due to loss of γ-secretase function (Xia et al., 2015). A recent paper suggests that PS1 undergoes a conformational change during aging and sporadic AD, and this change may have downstream effects on the processing of its substrates APP and Notch (Wahlster et al., 2013). PS1 regulates NPC differentiation in the adult brain (Gadadhar et al., 2011) via β-catenin, Notch1 and CREB (Bonds et al., 2015). Down regulation of PS1 in hippocampal NPCs compromises the maturation of new neurons, manifested by deficits in their dendritic tree branching, leading to learning and memory deficits (Bonds et al., 2015), suggesting that PS1-induced dysfunction of neurogenesis can impair cognitive function in AD. Transgenic expression of FAD-linked mutant variants of PS1 also impairs neurogenesis and the neurogenic response to experience in an enriched environment (EE) (Wang et al., 2004; Wen et al., 2004; Chevallier et al., 2005; Choi et al., 2008).
Amyloid precursor protein (APP)- APP is a substrate of γ-secretase. Misregulated cleavage of APP in the amyloidogenic pathway is implicated in FAD. While the physiological role of APP is yet to be fully understood, numerous studies suggest a role in synaptic plasticity and neurogenesis (Lazarov and Demars, 2012). The soluble form of APP (sAPPα) regulates NPC proliferation and survival (Demars et al., 2011, 2013). In fact, neurogenesis can be upregulated in the aging mouse brain following injection of sAPPα into the SVZ (Demars et al., 2013). While APP is extensively researched in regards to AD, the regulation of APP with aging is less well studied. However, there is some evidence that APP processing may be altered during aging, perhaps through dysregulation of the circadian system (Dobrowolska et al., 2014). In FAD, there is upregulation of the less potent sAPPβ counterpart at the expense of sAPPα, which may compromise proliferation of NPCs (Demars et al., 2011, 2013). Interestingly, sAPPα plays an important role in migration of NPC during brain development (Young-Pearse et al., 2007, 2008). Other metabolites of APP, such as AICD and Aβ have been suggested to regulate neurogenesis (for review see Lazarov et al., 2010), but more studies are warranted in order to establish their role.
Tau- is a neuronal microtubule-associated protein, the hyperphosphorylation and aggregation of which plays a key role in AD pathology. Significantly, adult born neurons transiently express the tau-3R isoform during development, overlapping with DCX and NeuN co-expression in the DG (Bullmann et al., 2007; Llorens-Martin et al., 2012). Tau phosphorylation in the DG is also temporally and spatially linked to DCX and neuroD expression with activated GSK-β believed to be the main tau kinase in newborn neurons (Fuster-Matanzo et al., 2009; Hong et al., 2010). The genomic based hTau mouse model exhibited reduction in adult neurogenesis, as a result of decreased proliferation, as early as 2 months of age before the appearance of significant tau pathology (Komuro et al., 2015), which may suggest that either impaired hippocampal neurogenesis is an early hallmark of tau pathology in AD or that there is an association between tau pathology and defective neurogenesis in AD. For a comprehensive review about tau and adult neurogenesis see (Fuster-Matanzo et al., 2012).
Notch 1- is a critical neurogenic signal and a substrate of γ-secretase. The intracellular domain cleavage product, NICD, translocates to the nucleus and drives transcription of factors important for maintaining the NSC pool such as Hes and ErbB2 (for review Pierfelice et al., 2011). Notch signaling occurs when the Notch receptor is activated by one of its ligands in the Jagged or Delta-like family of proteins (for review Kopan and Ilagan, 2009). Following physical activity, NPC proliferation is increased in a Notch-dependent manner in the SGZ of the DG, even in aged mice (Lugert et al., 2010). In contrast, Notch signaling is decreased with age, including in the hippocampus (Lugert et al., 2010; Tseng et al., 2014). Down regulation of PS1 in hippocampal NPC results in reduced levels of NICD (Bonds et al., 2015). In mature neurons Notch levels are low, and its function is not fully elucidated (for review see Marathe and Alberi, 2015; Marathe et al., 2015).
Wnt/β-catenin- are critical signaling factors in the regulation of hippocampal neurogenesis (Chenn and Walsh, 2003; Sato et al., 2004; Lie et al., 2005; Shimizu et al., 2008). Wnt3 is expressed in the SGZ of the DG, and overexpression of Wnt3 is sufficient to increase neurogenesis (Lie et al., 2005). Wnts are produced by astrocytes in the adult hippocampal niche and support the proliferation and differentiation of neuronally-restricted NPCs (Lie et al., 2005). Wnts regulate NSC self-renewal by inactivating Glycogen synthase kinase 3 (GSK3) and stabilizing β-catenin (Shimizu et al., 2008). Further, β-catenin promotes NPC proliferation through the activation of LEF/TCF transcription factors (Shimizu et al., 2008). Interestingly, nuclear accumulated β-catenin also induces anti-neurogenic hes1 gene expression through the enhancement of Notch1- and RBP-J-mediated transcription. β-catenin can associate with the NICD, and it is present in a nuclear protein-DNA complex containing the hes1 gene promoter. The β-catenin–NICD complex is efficiently formed when transcriptional coactivators p300 and P/CAF are present. Also, significantly, following its cleavage, the PS1CTF/NTF forms a complex with GSK3 and β-catenin (Tesco et al., 1998; Tesco and Tanzi, 2000). PS1 has been implicated as a negative regulator of the Wnt/β-catenin signaling pathway (Xia et al., 2001). Wnt-independent interaction of β-catenin and PS1 has also been described (Kang et al., 2002). Downregulation of PS1 in adult NPCs compromises the phosphorylation of β-catenin, which may affect β-catenin translocation to the nucleus, leading to alterations in the normal development of NPC (Bonds et al., 2015).
CREB- Cyclic-AMP Response Element Binding protein (CREB) is a critical signaling factor for adult brain plasticity and learning (for review Kandel, 2012). Activation of CREB by phosphorylation on Ser133 (pCREB) is observed in the hippocampus and cortical areas following learning and memory tasks (for review Mayr and Montminy, 2001). Importantly, NPCs, neuroblasts and immature neurons constitutively express pCREB, suggesting that pCREB is a critical component of neurogenesis. Indeed, CREB plays a role in neuronal maturation and survival in hippocampal neurogenesis (for review Ge et al., 2006; Jagasia et al., 2009; Herold et al., 2011; Merz et al., 2011). In rodents, CREB signaling components in the hippocampus decrease with age (Chung et al., 2002; Kudo et al., 2005; Porte et al., 2008). However, these observations were made primarily in mature neurons. Thus, the impact of aging on NPC-specific CREB signaling remains unclear. Also unclear is how aging causes a decrease in CREB signaling, although hypotheses suggest that this could occur either by aging-dependent increased levels of reactive oxygen species, or via decreased NMDA receptor and BDNF expression, which are both important for CREB activation (Chung et al., 2002; Kudo et al., 2005; Porte et al., 2008; Ozgen et al., 2010). Interestingly, exposure to young blood increased CREB activation and neurogenesis in the aged hippocampus, suggesting that systemic factors that are altered with aging may play an important role in CREB signaling and neurogenesis in the brain (Villeda et al., 2011; Villeda and Wyss-Coray, 2013). Impaired CREB signaling in AD has been the subject of much study. CREB signaling is dysregulated in both human AD and in mouse models of FAD (Vitolo et al., 2002; Ma et al., 2007; Caccamo et al., 2010; Bartolotti et al., 2015). In addition, down regulation of PS1 expression in NPCs compromises pCREB expression, leading to defective maturation of new neurons and induction of cognitive deficits (Bonds et al., 2015). While the role of CREB signaling in memory via mature neurons is well documented, the contribution of CREB signaling in NPCs to memory is not fully elucidated, and separating out the contribution of CREB to learning and memory via mature neurons or via NPC function is technically challenging and remains to be investigated (for review see Scott Bitner, 2012; Ortega-Martinez, 2015). Likewise, most of the work on CREB signaling in AD has focused on the transient activation in mature neurons during the formation of long-term memories, and so the contribution of CREB signaling in NPC in the context of AD also remains an open question.
While it is clear that hippocampal neurogenesis takes place in the human brain and that the number of new neurons generated is significant (Spalding et al., 2013), information concerning the fate of neurogenesis in aging and cognitively impaired individuals is scarce. Current techniques allow the examination of neurogenesis postmortem. However, because of the dynamic modulation neurogenesis can undergo following numerous stimuli, such as progressive pathology, the development of methodologies for the detection of neurogenesis in live individuals will be crucial. Up to the present time, tools for the detection of neurogenesis in live humans have been limited. The level of 14C in genomic DNA has been used for the estimation of date of birth of hippocampal neurons and their quantification in postmortem tissue (Spalding et al., 2013). A previous study suggests that adult neurogenesis can be specifically detected by proton nuclear magnetic resonance spectroscopy (1H-MRS, Manganas et al., 2007). However, this method was challenged by Loewenbruck et al. (2011), thus, more studies are warranted for the determination of the specificity, sensitivity and feasibility of 1H-MRS for the detection and quantification of neurogenesis.
The association between decline in neurogenesis and cognitive deterioration during aging, coupled with disruption in neurogenesis and cognitive dysfunction in FAD mouse models suggests that enhancing neurogenesis may be a feasible therapeutic approach (Figure 2). Successful attempts to enhance neurogenesis in rodents have been described. For example Sahay et al. used genetic manipulation of neurogenic pathways, excising the pro-apoptotic gene Bax, to enhance survival of nestin expressing cells (Sahay et al., 2011). They observed enhanced performance in the DG-dependent pattern separation task, where animals must distinguish between two similar contexts. Wang et al. also enhanced cell survival, neuronal differentiation, and dendritic complexity in neurogenic regions through activation of ERK5 map kinase (Wang et al., 2014). Following this manipulation, animals had increased performance in spatial learning and memory in the Morris Water Maze (MWM) task. In MWM and the novel object recognition task they also probed long-term memory and saw improvements as well, suggesting that adult neurogenesis may be a key therapeutic target.
Figure 2. Therapeutic and translational potential of neurogenesis. Examples of current and prospective methods for the modulation and detection of neurogenesis. Means of enhancing neurogenesis include noninvasive, environmental modulations like cognitively complex activities and exercise, as well as molecular interventions like anti-depressants, pro-neurogenic small molecules, hormones or neurotransmitters, or other manipulations of the neurogenic pathways. While readouts of human neurogenesis are typically done in postmortem tissue using radioactive isotopes or analysis of neurogeneic cell markers, imaging techniques such as fMRI, or blood biomarkers will offer non-invasive avenues to determine neurogenesis during life.
Given the evidence from genetic manipulation of neurogenesis in rodents, it is important to consider how neurogenesis could be modulated in humans. One approach is the modulation of lifestyle factors, termed environmental enrichment (EE). Evidence from rodents suggests that EE and running are effective ways to enhance hippocampal plasticity and neurogenesis in particular (Kempermann et al., 1997; van Praag et al., 1999a,b). These behavioral interventions have been found to enhance neurogenesis and ameliorate pathology in AD mouse models (Lazarov et al., 2005; Lazarov and Larson, 2007; Hu et al., 2010, 2013). Significantly, studies have shown that exercise can improve cognitive performance in the elderly (Ahlskog et al., 2011). Brief increases in physical activity (6–12 months) upregulates hippocampal volume and improves both episodic and spatial memory (Klusmann et al., 2010; Erickson et al., 2011; Ruscheweyh et al., 2011). In rodents it has also been shown that EE can increase many of the molecular factors involved in neurogenesis, such as pCREB expression and CRE- gene transcription in the hippocampus of wild-type mice (Hu et al., 2013; Bartolotti et al., 2015). While this observation was not specific to new neurons, it raises the possibility that enhanced CREB signaling may be one mechanism by which EE may increase the survival of new neurons. Nevertheless, EE and running do not target neurogenesis specifically, but have numerous effects on the hippocampus. Several studies describe the manipulation of neurogenesis using small molecules (Longo et al., 2006; Schneider et al., 2008; McNeish et al., 2010; Pieper et al., 2010; Lange et al., 2011; MacMillan et al., 2011; Neely et al., 2012; Petrik et al., 2012; Shi et al., 2013) or pharmacological agents, such as SSRI's or modulators of neurogenic pathways [For example, see Warner-Schmidt and Duman, 2007]. Some of these have been shown to enhance neurogenesis and reverse memory deficits. However, to this point the use of these compounds in AD mouse models has not been explored. In future experiments it will be important to consider the mechanism by which these molecules modulate adult neurogenesis in light of the signaling cascades we have described here. Particularly considering how these cascades are altered in aging and AD, both in rodent models and in humans.
This manuscript is based on data produced by Dr. CH and Mrs. NB. Dr. CH and Ms. NB and Prof. OL wrote this mini-review.
The authors declare that the research was conducted in the absence of any commercial or financial relationships that could be construed as a potential conflict of interest.
This work was supported by NIA R01AG033570, Department of Defense 10917352, NIA 1RC1AG036208-01 ARR, CCTS-0512-06 (OL), PECTS Award CCTS Pre-doctoral Education (NB).
Ahlskog, J. E., Geda, Y. E., Graff-Radford, N. R., and Petersen, R. C. (2011). Physical exercise as a preventive or disease-modifying treatment of dementia and brain aging. Mayo Clin. Proc. 86, 876–884. doi: 10.4065/mcp.2011.0252
Bartolotti, N., Segura, L., and Lazarov, O. (2015). Diminished CRE-induced plasticity is linked to memory deficits in familial Alzheimer's Disease mice. J. Alzheimers Dis. 50, 477–489. doi: 10.3233/JAD-150650
Baulac, S., LaVoie, M. J., Kimberly, W. T., Strahle, J., Wolfe, M. S., Selkoe, D. J., et al. (2003). Functional gamma-secretase complex assembly in Golgi/trans-Golgi network: interactions among presenilin, nicastrin, Aph1, Pen-2, and gamma-secretase substrates. Neurobiol. Dis. 14, 194–204. doi: 10.1016/S0969-9961(03)00123-2
Bonds, J. A., Kuttner-Hirshler, Y., Bartolotti, N., Tobin, M. K., Pizzi, M., Marr, R., et al. (2015). Presenilin-1 dependent neurogenesis regulates hippocampal learning and memory. PLoS ONE 10:e0131266. doi: 10.1371/journal.pone.0131266
Brickman, A. M., Khan, U. A., Provenzano, F. A., Yeung, L. K., Suzuki, W., Schroeter, H., et al. (2014). Enhancing dentate gyrus function with dietary flavanols improves cognition in older adults. Nat. Neurosci. 17, 1798–1803. doi: 10.1038/nn.3850
Brickman, A. M., Meier, I. B., Korgaonkar, M. S., Provenzano, F. A., Grieve, S. M., Siedlecki, K. L., et al. (2012). Testing the white matter retrogenesis hypothesis of cognitive aging. Neurobiol. Aging 33, 1699–1715. doi: 10.1016/j.neurobiolaging.2011.06.001
Bullmann, T., de Silva, R., Holzer, M., Mori, H., and Arendt, T. (2007). Expression of embryonic tau protein isoforms persist during adult neurogenesis in the hippocampus. Hippocampus 17, 98–102. doi: 10.1002/hipo.20255
Caccamo, A., Maldonado, M. A., Bokov, A. F., Majumder, S., and Oddo, S. (2010). CBP gene transfer increases BDNF levels and ameliorates learning and memory deficits in a mouse model of Alzheimer's disease. Proc. Natl. Acad. Sci. U.S.A. 107, 22687–22692. doi: 10.1073/pnas.1012851108
Chenn, A., and Walsh, C. A. (2003). Increased neuronal production, enlarged forebrains and cytoarchitectural distortions in beta-catenin overexpressing transgenic mice. Cereb. Cortex 13, 599–606. doi: 10.1093/cercor/13.6.599
Chevallier, N. L., Soriano, S., Kang, D. E., Masliah, E., Hu, G., and Koo, E. H. (2005). Perturbed neurogenesis in the adult hippocampus associated with presenilin-1 A246E mutation. Am. J. Pathol. 167, 151–159. doi: 10.1016/S0002-9440(10)62962-8
Choi, S. H., Veeraraghavalu, K., Lazarov, O., Marler, S., Ransohoff, R. M., Ramirez, J. M., et al. (2008). Non-cell-autonomous effects of presenilin 1 variants on enrichment-mediated hippocampal progenitor cell proliferation and differentiation. Neuron 59, 568–580. doi: 10.1016/j.neuron.2008.07.033
Chuang, T. T. (2010). Neurogenesis in mouse models of Alzheimer's disease. Biochim. Biophys. Acta 1802, 872–880. doi: 10.1016/j.bbadis.2009.12.008
Chung, Y. H., Kim, E. J., Shin, C. M., Joo, K. M., Kim, M. J., Woo, H. W., et al. (2002). Age-related changes in CREB binding protein immunoreactivity in the cerebral cortex and hippocampus of rats. Brain Res. 956, 312–318. doi: 10.1016/S0006-8993(02)03562-X
Demars, M., Hu, Y. S., Gadadhar, A., and Lazarov, O. (2010). Impaired neurogenesis is an early event in the etiology of familial Alzheimer's disease in transgenic mice. J. Neurosci. Res. 88, 2103–2117. doi: 10.1002/jnr.22387
Demars, M. P., Bartholomew, A., Strakova, Z., and Lazarov, O. (2011). Soluble amyloid precursor protein: a novel proliferation factor of adult progenitor cells of ectodermal and mesodermal origin. Stem Cell Res. Ther. 2, 36. doi: 10.1186/scrt77
Demars, M. P., Hollands, C., Zhao, K. D., and Lazarov, O. (2013). Soluble amyloid precursor protein-alpha rescues age-linked decline in neural progenitor cell proliferation. Neurobiol. Aging. 34, 2431–2440. doi: 10.1016/j.neurobiolaging.2013.04.016
De Strooper, B., Annaert, W., Cupers, P., Saftig, P., Craessaerts, K., Mumm, J. S., et al. (1999). A presenilin-1-dependent gamma-secretase-like protease mediates release of Notch intracellular domain. Nature 398, 518–522. doi: 10.1038/19083
De Strooper, B., Saftig, P., Craessaerts, K., Vanderstichele, H., Guhde, G., Annaert, W., et al. (1998). Deficiency of presenilin-1 inhibits the normal cleavage of amyloid precursor protein. Nature 391, 387–390. doi: 10.1038/34910
Dobrowolska, J. A., Michener, M. S., Wu, G., Patterson, B. W., Chott, R., Ovod, V., et al. (2014). CNS amyloid-beta, soluble APP-alpha and -beta kinetics during BACE inhibition. J. Neurosci. 34, 8336–8346. doi: 10.1523/JNEUROSCI.0540-14.2014
Erickson, K. I., Voss, M. W., Prakash, R. S., Basak, C., Szabo, A., Chaddock, L., et al. (2011). Exercise training increases size of hippocampus and improves memory. Proc. Natl. Acad. Sci. U.S.A. 108, 3017–3022. doi: 10.1073/pnas.1015950108
Eriksson, P. S., Perfilieva, E., Björk-Eriksson, T., Alborn, A. M., Nordborg, C., Peterson, D. A., et al. (1998). Neurogenesis in the adult human hippocampus. Nat. Med. 4, 1313–1317. doi: 10.1038/3305
Ernst, A., Alkass, K., Bernard, S., Salehpour, M., Perl, S., Tisdale, J., et al. (2014). Neurogenesis in the striatum of the adult human brain. Cell 156, 1072–1083. doi: 10.1016/j.cell.2014.01.044
Flood, J. F., Harris, F. J., and Morley, J. E. (1996). Age-related changes in hippocampal drug facilitation of memory processing in SAMP8 mice. Neurobiol. Aging 17, 15–24. doi: 10.1016/0197-4580(95)02007-1
Fuster-Matanzo, A., de Barreda, E. G., Dawson, H. N., Vitek, M. P., Avila, J., and Hernández, F. (2009). Function of tau protein in adult newborn neurons. FEBS Lett. 583, 3063–3068. doi: 10.1016/j.febslet.2009.08.017
Fuster-Matanzo, A., Llorens-Martín, M., Jurado-Arjona, J., Avila, J., and Hernández, F. (2012). Tau protein and adult hippocampal neurogenesis. Front. Neurosci. 6:104. doi: 10.3389/fnins.2012.00104
Gadadhar, A., Marr, R., and Lazarov, O. (2011). Presenilin-1 regulates neural progenitor cell differentiation in the adult brain. J. Neurosci. 31, 2615–2623. doi: 10.1523/JNEUROSCI.4767-10.2011
Ge, S., Goh, E. L., Sailor, K. A., Kitabatake, Y., Ming, G. L., and Song, H. (2006). GABA regulates synaptic integration of newly generated neurons in the adult brain. Nature 439, 589–593. doi: 10.1038/nature04404
Geinisman, Y., Berry, R. W., Disterhoft, J. F., Power, J. M., and Van der Zee, E. A. (2001). Associative learning elicits the formation of multiple-synapse boutons. J. Neurosci. 21, 5568–5573.
Geinisman, Y., Ganeshina, O., Yoshida, R., Berry, R. W., Disterhoft, J. F., and Gallagher, M. (2004). Aging, spatial learning, and total synapse number in the rat CA1 stratum radiatum. Neurobiol. Aging 25, 407–416. doi: 10.1016/j.neurobiolaging.2003.12.001
Hamilton, L. K., Aumont, A., Julien, C., Vadnais, A., Calon, F., and Fernandes, K. J. (2010). Widespread deficits in adult neurogenesis precede plaque and tangle formation in the 3xTg mouse model of Alzheimer's disease. Eur. J. Neurosci. 32, 905–920. doi: 10.1111/j.1460-9568.2010.07379.x
Hattiangady, B., and Shetty, A. K. (2008). Aging does not alter the number or phenotype of putative stem/progenitor cells in the neurogenic region of the hippocampus. Neurobiol. Aging 29, 129–147. doi: 10.1016/j.neurobiolaging.2006.09.015
Herold, S., Jagasia, R., Merz, K., Wassmer, K., and Lie, D. C. (2011). CREB signalling regulates early survival, neuronal gene expression and morphological development in adult subventricular zone neurogenesis. Mol. Cell Neurosci. 46, 79–88. doi: 10.1016/j.mcn.2010.08.008
Hong, X. P., Peng, C. X., Wei, W., Tian, Q., Liu, Y. H., Yao, X. Q., et al. (2010). Essential role of tau phosphorylation in adult hippocampal neurogenesis. Hippocampus 20, 1339–1349. doi: 10.1002/hipo.20712
Hu, Y. S., Long, N., Pigino, G., Brady, S. T., and Lazarov, O. (2013). Molecular mechanisms of environmental enrichment: impairments in Akt/GSK3beta, neurotrophin-3 and CREB signaling. PLoS ONE 8:e64460. doi: 10.1371/journal.pone.0064460
Hu, Y. S., Xu, P., Pigino, G., Brady, S. T., Larson, J., and Lazarov, O. (2010). Complex environment experience rescues impaired neurogenesis, enhances synaptic plasticity, and attenuates neuropathology in familial Alzheimer's disease-linked APPswe/PS1{Delta}E9 mice. FASEB J. 24, 1667–1681. doi: 10.1096/fj.09-136945
Imayoshi, I., Sakamoto, M., Ohtsuka, T., Takao, K., Miyakawa, T., Yamaguchi, M., et al. (2008). Roles of continuous neurogenesis in the structural and functional integrity of the adult forebrain. Nat. Neurosci. 11, 1153–1161. doi: 10.1038/nn.2185
Jagasia, R., Steib, K., Englberger, E., Herold, S., Faus-Kessler, T., Saxe, M., et al. (2009). GABA-cAMP response element-binding protein signaling regulates maturation and survival of newly generated neurons in the adult hippocampus. J. Neurosci. 29, 7966–7977. doi: 10.1523/JNEUROSCI.1054-09.2009
Jin, K., Peel, A. L., Mao, X. O., Xie, L., Cottrell, B. A., Henshall, D. C., et al. (2004). Increased hippocampal neurogenesis in Alzheimer's disease. Proc. Natl. Acad. Sci. U.S.A. 101, 343–347. doi: 10.1073/pnas.2634794100
Kandel, E. R. (2012). The molecular biology of memory: cAMP, PKA, CRE, CREB-1, CREB-2, and CPEB. Mol. Brain 5:14. doi: 10.1186/1756-6606-5-14
Kang, D. E., Soriano, S., Xia, X., Eberhart, C. G., De Strooper, B., Zheng, H., et al. (2002). Presenilin couples the paired phosphorylation of beta-catenin independent of axin: implications for beta-catenin activation in tumorigenesis. Cell 110, 751–762. doi: 10.1016/S0092-8674(02)00970-4
Kempermann, G., Kuhn, H. G., and Gage, F. H. (1997). More hippocampal neurons in adult mice living in an enriched environment. Nature 386, 493–495. doi: 10.1038/386493a0
Kempermann, G., Song, H., and Gage, F. H. (2015). Neurogenesis in the Adult Hippocampus. Cold Spring Harb. Perspect. Med. 5:a018812. doi: 10.1101/cshperspect.a018812
Klusmann, V., Evers, A., Schwarzer, R., Schlattmann, P., Reischies, F. M., Heuser, I., et al. (2010). Complex mental and physical activity in older women and cognitive performance: a 6-month randomized controlled trial. J. Gerontol. A Biol. Sci. Med. Sci. 65, 680–688. doi: 10.1093/gerona/glq053
Komuro, Y., Xu, G., Bhaskar, K., and Lamb, B. T. (2015). Human tau expression reduces adult neurogenesis in a mouse model of tauopathy. Neurobiol. Aging 36, 2034–2042. doi: 10.1016/j.neurobiolaging.2015.03.002
Kopan, R., and Ilagan, M. X. G. (2009). The canonical Notch signaling pathway: unfolding the activation mechanism. Cell 137, 216–233. doi: 10.1016/j.cell.2009.03.045
Kudo, K., Wati, H., Qiao, C., Arita, J., and Kanba, S. (2005). Age-related disturbance of memory and CREB phosphorylation in CA1 area of hippocampus of rats. Brain Res. 1054, 30–37. doi: 10.1016/j.brainres.2005.06.045
Lange, C., Mix, E., Frahm, J., Glass, A., Müller, J., Schmitt, O., et al. (2011). Small molecule GSK-3 inhibitors increase neurogenesis of human neural progenitor cells. Neurosci. Lett. 488, 36–40. doi: 10.1016/j.neulet.2010.10.076
Lazarov, O., and Demars, M. P. (2012). All in the family: how the APPs regulate neurogenesis. Front. Neurosci. 6:81. doi: 10.3389/fnins.2012.00081
Lazarov, O., and Hollands, C. (2016). Hippocampal neurogenesis: learning to remember. Prog Neurobiol. 138-140:1-18. doi: 10.1016/j.pneurobio.2015.12.006
Lazarov, O., and Larson, J. (2007). “Research progress in Alzheimer's disease and dementia,” in Environmental Enrichment: from Mouse AD Model to AD Therapy, Vol. 3. (New York, NY: Nova Science Publishers, Inc.), 303–328.
Lazarov, O., and Marr, R. A. (2010). Neurogenesis and Alzheimer's disease: at the crossroads Exp. Neurol. 223, 267–281. doi: 10.1016/j.expneurol.2009.08.009
Lazarov, O., and Marr, R. A. (2013). Of mice and men: neurogenesis, cognition and Alzheimer's disease. Front. Aging Neurosci. 5:43. doi: 10.3389/fnagi.2013.00043
Lazarov, O., Mattson, M. P., Peterson, D. A., Pimplikar, S. W., and van Praag, H. (2010). When neurogenesis encounters aging and disease. Trends Neurosci. 33, 569–579. doi: 10.1016/j.tins.2010.09.003
Lazarov, O., Robinson, J., Tang, Y. P., Hairston, I. S., Korade-Mirnics, Z., Lee, V. M., et al. (2005). Environmental enrichment reduces Abeta levels and amyloid deposition in transgenic mice. Cell 120, 701–713. doi: 10.1016/j.cell.2005.01.015
Lie, D. C., Colamarino, S. A., Song, H. J., Désiré, L., Mira, H., Consiglio, A., et al. (2005). Wnt signalling regulates adult hippocampal neurogenesis. Nature 437, 1370–1375. doi: 10.1038/nature04108
Llorens-Martin, M., Teixeira, C. M., Fuster-Matanzo, A., Jurado-Arjona, J., Borrell, V., Soriano, E., et al. (2012). Tau isoform with three microtubule binding domains is a marker of new axons generated from the subgranular zone in the hippocampal dentate gyrus: implications for Alzheimer's disease. J. Alzheimers. Dis. 29, 921–930. doi: 10.3233/JAD-2012-112057.
Loewenbruck, K. F., Fuchs, B., Hermann, A., Brandt, M., Werner, A., Kirsch, M., et al. (2011). Proton MR spectroscopy of neural stem cells: does the proton-NMR peak at 1.28 ppm function as a biomarker for cell type or state? Rejuvenation Res. 14, 371–381. doi: 10.1089/rej.2010.1102
Longo, F. M., Yang, T., Xie, Y., and Massa, S. M. (2006). Small molecule approaches for promoting neurogenesis. Curr. Alzheimer Res. 3, 5–10. doi: 10.2174/156720506775697089
Lugert, S., Basak, O., Knuckles, P., Haussler, U., Fabel, K., Götz, M., et al. (2010). Quiescent and active hippocampal neural stem cells with distinct morphologies respond selectively to physiological and pathological stimuli and aging. Cell Stem Cell 6, 445–456. doi: 10.1016/j.stem.2010.03.017
Ma, Q. L., Harris-White, M. E., Ubeda, O. J., Simmons, M., Beech, W., Lim, G. P., et al. (2007). Evidence of Abeta- and transgene-dependent defects in ERK-CREB signaling in Alzheimer's models. J. Neurochem. 103, 1594–1607. doi: 10.1111/j.1471-4159.2007.04869.x
MacMillan, K. S., Naidoo, J., Liang, J., Melito, L., Williams, N. S., Morlock, L., et al. (2011). Development of proneurogenic, neuroprotective small molecules. J. Am. Chem. Soc. 133, 1428–1437. doi: 10.1021/ja108211m
Manganas, L. N., Zhang, X., Li, Y., Hazel, R. D., Smith, S. D., Wagshul, M. E., et al. (2007). Magnetic resonance spectroscopy identifies neural progenitor cells in the live human brain. Science 318, 980–985. doi: 10.1126/science.1147851
Marathe, S., and Alberi, L. (2015). Notch in memories: points to remember. Hippocampus 25, 1481–1488. doi: 10.1002/hipo.22426
Marathe, S., Liu, S., Brai, E., Kaczarowski, M., and Alberi, L. (2015). Notch signaling in response to excitotoxicity induces neurodegeneration via erroneous cell cycle reentry. Cell Death Differ. 22, 1775–1784. doi: 10.1038/cdd.2015.23
Mayr, B., and Montminy, M. (2001). Transcriptional regulation by the phosphorylation-dependent factor CREB. Nat. Rev. Mol. Cell Biol. 2, 599–609. doi: 10.1038/35085068
McNeish, J., Roach, M., Hambor, J., Mather, R. J., Weibley, L., Lazzaro, J., et al. (2010). High-throughput screening in embryonic stem cell-derived neurons identifies potentiators of alpha-amino-3-hydroxyl-5-methyl-4-isoxazolepropionate-type glutamate receptors. J. Biol. Chem. 285, 17209–17217. doi: 10.1074/jbc.M109.098814
Merz, K., Herold, S., and Lie, D. C. (2011). CREB in adult neurogenesis–master and partner in the development of adult-born neurons? Eur. J. Neurosci. 33, 1078–1086. doi: 10.1111/j.1460-9568.2011.07606.x
Neely, M. D., Litt, M. J., Tidball, A. M., Li, G. G., Aboud, A. A., Hopkins, C. R., et al. (2012). DMH1, a highly selective small molecule BMP inhibitor promotes neurogenesis of hiPSCs: comparison of PAX6 and SOX1 expression during neural induction. ACS Chem. Neurosci. 3, 482–491. doi: 10.1021/cn300029t
Ninkovic, J., and Götz, M. (2007). Signaling in adult neurogenesis: from stem cell niche to neuronal networks. Curr. Opin. Neurobiol. 17, 338–344. doi: 10.1016/j.conb.2007.04.006
Ortega-Martínez, S. (2015). A new perspective on the role of the CREB family of transcription factors in memory consolidation via adult hippocampal neurogenesis. Front. Mol. Neurosci. 8:46. doi: 10.3389/fnmol.2015.00046
Ozgen, N., Lau, D. H., Shlapakova, I. N., Sherman, W., Feinmark, S. J., Danilo, P. Jr., et al. (2010). Determinants of CREB degradation and KChIP2 gene transcription in cardiac memory. Heart Rhythm 7, 964–970. doi: 10.1016/j.hrthm.2010.03.024
Petrik, D., Jiang, Y., Birnbaum, S. G., Powell, C. M., Kim, M. S., Hsieh, J., et al. (2012). Functional and mechanistic exploration of an adult neurogenesis-promoting small molecule. FASEB J. 26, 3148–3162. doi: 10.1096/fj.11-201426
Pieper, A. A., Xie, S., Capota, E., Estill, S. J., Zhong, J., Long, J. M., et al. (2010). Discovery of a proneurogenic, neuroprotective chemical. Cell 142, 39–51. doi: 10.1016/j.cell.2010.06.018
Pierfelice, T., Alberi, L., and Gaiano, N. (2011). Notch in the vertebrate nervous system: an old dog with new tricks. Neuron 69, 840–855. doi: 10.1016/j.neuron.2011.02.031
Porte, Y., Buhot, M. C., and Mons, N. (2008). Alteration of CREB phosphorylation and spatial memory deficits in aged 129T2/Sv mice. Neurobiol. Aging 29, 1533–1546. doi: 10.1016/j.neurobiolaging.2007.03.023
Rodríguez, J. J., Jones, V. C., Tabuchi, M., Allan, S. M., Knight, E. M., LaFerla, F. M., et al. (2008). Impaired adult neurogenesis in the dentate gyrus of a triple transgenic mouse model of Alzheimer's disease. PLoS ONE 3:e2935. doi: 10.1371/journal.pone.0002935
Ruscheweyh, R., Willemer, C., Krüger, K., Duning, T., Warnecke, T., Sommer, J., et al. (2011). Physical activity and memory functions: an interventional study. Neurobiol. Aging 32, 1304–1319. doi: 10.1016/j.neurobiolaging.2009.08.001
Sahay, A., Scobie, K. N., Hill, A. S., O'Carroll, C. M., Kheirbek, M. A., Burghardt, N. S., et al. (2011). Increasing adult hippocampal neurogenesis is sufficient to improve pattern separation. Nature 472, 466–470. doi: 10.1038/nature09817
Sato, N., Meijer, L., Skaltsounis, L., Greengard, P., and Brivanlou, A. H. (2004). Maintenance of pluripotency in human and mouse embryonic stem cells through activation of Wnt signaling by a pharmacological GSK-3-specific inhibitor. Nat. Med. 10, 55–63. doi: 10.1038/nm979
Schneider, J. W., Gao, Z., Li, S., Farooqi, M., Tang, T. S., Bezprozvanny, I., et al. (2008). Small-molecule activation of neuronal cell fate. Nat. Chem. Biol. 4, 408–410. doi: 10.1038/nchembio.95
Scott Bitner, R. (2012). Cyclic AMP response element-binding protein (CREB) phosphorylation: a mechanistic marker in the development of memory enhancing Alzheimer's disease therapeutics. Biochem. Pharmacol. 83, 705–714. doi: 10.1016/j.bcp.2011.11.009
Selkoe, D. J., and Wolfe, M. S. (2007). Presenilin: running with scissors in the membrane. Cell 131, 215–221. doi: 10.1016/j.cell.2007.10.012
Shi, J., Longo, F. M., and Massa, S. M. (2013). A small molecule p75(NTR) ligand protects neurogenesis after traumatic brain injury. Stem Cells 31, 2561–2574. doi: 10.1002/stem.1516
Shimizu, T., Kagawa, T., Inoue, T., Nonaka, A., Takada, S., Aburatani, H., et al. (2008). Stabilized beta-catenin functions through TCF/LEF proteins and the Notch/RBP-Jkappa complex to promote proliferation and suppress differentiation of neural precursor cells. Mol. Cell Biol. 28, 7427–7441. doi: 10.1128/MCB.01962-07
Silva-Vargas, V., Crouch, E. E., and Doetsch, F. (2013). Adult neural stem cells and their niche: a dynamic duo during homeostasis, regeneration, and aging. Curr. Opin. Neurobiol. 23, 935–942. doi: 10.1016/j.conb.2013.09.004
Spalding, K., Bergmann, O., Alkass, K., Bernard, S., Salehpour, M., Huttner, H., et al. (2013). Dynamics of hippocampal neurogenesis in adult humans Cell. 153, 1219–1227 doi: 10.1016/j.cell.2013.05.002
Stark, S. M., Yassa, M. A., and Stark, C. E. (2010). Individual differences in spatial pattern separation performance associated with healthy aging in humans. Learn. Mem. 17, 284–288. doi: 10.1101/lm.1768110
Sun, B., Halabisky, B., Zhou, Y., Palop, J. J., Yu, G., Mucke, L., et al. (2009). Imbalance between GABAergic and glutamatergic transmission impairs adult neurogenesis in an animal model of Alzheimer's Disease. Cell Stem Cell 5, 624–633. doi: 10.1016/j.stem.2009.10.003
Tesco, G., Kim, T. W., Diehlmann, A., Beyreuther, K., and Tanzi, R. E. (1998). Abrogation of the presenilin 1/beta-catenin interaction and preservation of the heterodimeric presenilin 1 complex following caspase activation. J. Biol. Chem. 273, 33909–33914. doi: 10.1074/jbc.273.51.33909
Tesco, G., and Tanzi, R. E. (2000). GSK3 beta forms a tetrameric complex with endogenous PS1-CTF/NTF and beta-catenin. Effects of the D257/D385A and FAD-linked mutations. Ann. N.Y. Acad. Sci. 920, 227–232. doi: 10.1111/j.1749-6632.2000.tb06927.x
Toner, C. K., Pirogovsky, E., Kirwan, C. B., and Gilbert, P. E. (2009). Visual object pattern separation deficits in nondemented older adults. Learn. Mem. 16, 338–342. doi: 10.1101/lm.1315109
Tseng, C. Y., Kao, S. H., Wan, C. L., Cho, Y., Tung, S. Y., and Hsu, H. J. (2014). Notch signaling mediates the age-associated decrease in adhesion of germline stem cells to the niche. PLoS Genet. 10:e1004888. doi: 10.1371/journal.pgen.1004888
van Praag, H., Christie, B. R., Sejnowski, T. J., and Gage, F. H. (1999a). Running enhances neurogenesis, learning, and long-term potentiation in mice. Proc. Natl. Acad. Sci. U.S.A. 96, 13427–13431. doi: 10.1073/pnas.96.23.13427
van Praag, H., Kempermann, G., and Gage, F. H. (1999b). Running increases cell proliferation and neurogenesis in the adult mouse dentate gyrus [see comments]. Nat. Neurosci. 2, 266–270. doi: 10.1038/6368
Villeda, S. A., Luo, J., Mosher, K. I., Zou, B., Britschgi, M., Bieri, G., et al. (2011). The ageing systemic milieu negatively regulates neurogenesis and cognitive function. Nature 477, 90–94. doi: 10.1038/nature10357
Villeda, S. A., and Wyss-Coray, T. (2013). The circulatory systemic environment as a modulator of neurogenesis and brain aging. Autoimmun. Rev. 12, 674–677. doi: 10.1016/j.autrev.2012.10.014
Vitolo, O. V., Sant'Angelo, A., Costanzo, V., Battaglia, F., Arancio, O., and Shelanski, M. (2002). Amyloid beta -peptide inhibition of the PKA/CREB pathway and long-term potentiation: reversibility by drugs that enhance cAMP signaling. Proc. Natl. Acad. Sci. U.S.A. 99, 13217–13221. doi: 10.1073/pnas.172504199
Wahlster, L., Arimon, M., Nasser-Ghodsi, N., Post, K. L., Serrano-Pozo, A., Uemura, K., et al. (2013). Presenilin-1 adopts pathogenic conformation in normal aging and in sporadic Alzheimer's disease. Acta Neuropathol. 125, 187–199. doi: 10.1007/s00401-012-1065-6
Wang, R., Dineley, K. T., Sweatt, J. D., and Zheng, H. (2004). Presenilin 1 familial Alzheimer's disease mutation leads to defective associative learning and impaired adult neurogenesis. Neuroscience 126, 305–312. doi: 10.1016/j.neuroscience.2004.03.048
Wang, W., Pan, Y. W., Zou, J., Li, T., Abel, G. M., Palmiter, R. D., et al. (2014). Genetic activation of ERK5 MAP kinase enhances adult neurogenesis and extends hippocampus-dependent long-term memory. J. Neurosci. 34, 2130–2147. doi: 10.1523/JNEUROSCI.3324-13.2014
Warner-Schmidt, J. L., and Duman, R. S. (2007). VEGF is an essential mediator of the neurogenic and behavioral actions of antidepressants. Proc. Natl. Acad. Sci. U.S.A. 104, 4647–4652. doi: 10.1073/pnas.0610282104
Wen, P. H., Hof, P. R., Chen, X., Gluck, K., Austin, G., Younkin, S. G., et al. (2004). The presenilin-1 familial Alzheimer disease mutant P117L impairs neurogenesis in the hippocampus of adult mice. Exp. Neurol. 188, 224–237. doi: 10.1016/j.expneurol.2004.04.002
Xia, D., Watanabe, H., Wu, B., Lee, S. H., Li, Y., Tsvetkov, E., et al. (2015). Presenilin-1 knockin mice reveal loss-of-function mechanism for familial Alzheimer's disease. Neuron 85, 967–981. doi: 10.1016/j.neuron.2015.02.010
Xia, X., Qian, S., Soriano, S., Wu, Y., Fletcher, A. M., Wang, X. J., et al. (2001). Loss of presenilin 1 is associated with enhanced beta-catenin signaling and skin tumorigenesis. Proc. Natl. Acad. Sci. U.S.A. 98, 10863–10868. doi: 10.1073/pnas.191284198
Yassa, M. A., Mattfeld, A. T., Stark, S. M., and Stark, C. E. (2011). Age-related memory deficits linked to circuit-specific disruptions in the hippocampus. Proc. Natl. Acad. Sci. U.S.A. 108, 8873–8878. doi: 10.1073/pnas.1101567108
Young-Pearse, T. L., Bai, J., Chang, R., Zheng, J. B., LoTurco, J. J., and Selkoe, D. J. (2007). A critical function for beta-amyloid precursor protein in neuronal migration revealed by in utero RNA interference. J. Neurosci. 27, 14459–14469. doi: 10.1523/JNEUROSCI.4701-07.2007
Keywords: hippocampus, neurogenesis, Alzheimer's disease, cognition, learning and memory
Citation: Hollands C, Bartolotti N and Lazarov O (2016) Alzheimer's Disease and Hippocampal Adult Neurogenesis; Exploring Shared Mechanisms. Front. Neurosci. 10:178. doi: 10.3389/fnins.2016.00178
Received: 10 February 2016; Accepted: 07 April 2016;
Published: 03 May 2016.
Edited by:
Laura López-Mascaraque, Spanish National Research Council, SpainReviewed by:
Stefano Farioli Vecchioli, National Research Council, ItalyCopyright © 2016 Hollands, Bartolotti and Lazarov. This is an open-access article distributed under the terms of the Creative Commons Attribution License (CC BY). The use, distribution or reproduction in other forums is permitted, provided the original author(s) or licensor are credited and that the original publication in this journal is cited, in accordance with accepted academic practice. No use, distribution or reproduction is permitted which does not comply with these terms.
*Correspondence: Orly Lazarov, b2xhemFyb3ZAdWljLmVkdQ==
†These authors have contributed equally to this work.
Disclaimer: All claims expressed in this article are solely those of the authors and do not necessarily represent those of their affiliated organizations, or those of the publisher, the editors and the reviewers. Any product that may be evaluated in this article or claim that may be made by its manufacturer is not guaranteed or endorsed by the publisher.
Research integrity at Frontiers
Learn more about the work of our research integrity team to safeguard the quality of each article we publish.