- 1Biochemistry and Molecular Biology Department, Faculty of Veterinary Medicine, Complutense University, Madrid, Spain
- 2Brain Institute, Federal University of Rio Grande do Norte, Natal, Brazil
The generation of cells of the neural lineage within the brain is not restricted to early development. New neurons, oligodendrocytes, and astrocytes are produced in the adult brain throughout the entire murine life. However, despite the extensive research performed in the field of adult neurogenesis during the past years, fundamental questions regarding the cell biology of adult neural stem cells (aNSCs) remain to be uncovered. For instance, it is crucial to elucidate whether a single aNSC is capable of differentiating into all three different macroglial cell types in vivo or these distinct progenies constitute entirely separate lineages. Similarly, the cell cycle length, the time and mode of division (symmetric vs. asymmetric) that these cells undergo within their lineage progression are interesting questions under current investigation. In this sense, live imaging constitutes a valuable ally in the search of reliable answers to the previous questions. In spite of the current limitations of technology new approaches are being developed and outstanding amount of knowledge is being piled up providing interesting insights in the behavior of aNSCs. Here, we will review the state of the art of live imaging as well as the alternative models that currently offer new answers to critical questions.
Introduction
Current Knowledge of the Cytoarchitecture of the Adult Neurogenic Niches; Subependymal Zone and Dentate Gyrus
Stem Cells are defined by their ability to self-renew, giving rise to new stem cells, and their capacity to generate diverse specialized cell types. In the adult nervous system, the term neural stem cells (NSCs) refers to cells that maintain the capacity to self-renew and generate neurons and macroglial cells both in vitro (Reynolds and Weiss, 1996; Costa et al., 2011) and in vivo (Lois and Alvarez-Buylla, 1993; Gould and Cameron, 1996; Kempermann et al., 1997; Menn et al., 2006; Sohn et al., 2015). Adult neural stem cells (aNSCs) continuously generate neurons oligodendrocytes and astrocytes in discrete niches in the brain, although it is unclear whether multipotent or unipotent aNSCs contribute all these different lineages. Historically, the adult neurogenesis has been associated, under physiological conditions, to two specific neurogenic niches: the subependymal zone (SEZ) in the lateral wall of the lateral ventricle, and the subgranular zone (SGZ) of the dentate gyrus in the hippocampus reviewed by Gage (2000) and Kriegstein and Alvarez-Buylla (2009). However, the presence of aNSCs in alternative domains of the adult brain should not be discarded. Indeed, multipotent progenitors have been isolated from the postnatal mouse cerebral cortex (Marmur et al., 1998; Belachew et al., 2003; Seaberg et al., 2005; Costa et al., 2007) or adult mouse cerebral cortex after traumatic and ischemic lesion (Buffo et al., 2008; Sirko et al., 2013). Another interesting adult domain described to contain NSCs is the inner core of the olfactory bulb (OB) of both rodents and humans. Populations of NSCs expressing GFAP, Nestin, Sox2, and RC2 are located within the adult OB giving rise to neurons in vivo. Likewise they can be expanded in vitro as neurospheres, giving rise to astrocytes, oligodendrocytes and neurons. (Pagano et al., 2000; Gritti et al., 2002; Liu and Martin, 2003; Giachino and Taylor, 2009; Vergano-Vera et al., 2009; Moreno-Estelles et al., 2012). The same is applied for human temporal and frontal cortex, amygdala and hippocampus after resection due to a drug-resistant epilepsy, dysplasia, trauma, or brain edema (Arsenijevic et al., 2001). More recent evidence indicate that lesions may activate those “dormant” aNSCs through release of signaling molecules such as vascular endothelial growth factor (VEGF), basic fibroblast growth factor (bGFG), and sonic hedgehog (SHH; Sirko et al., 2013; Luo et al., 2015). Contribution of these quiescent aNSCs to a possible periodical and so far unnoticed turnover of their associated neuronal populations remains to be demonstrated Figure 1.
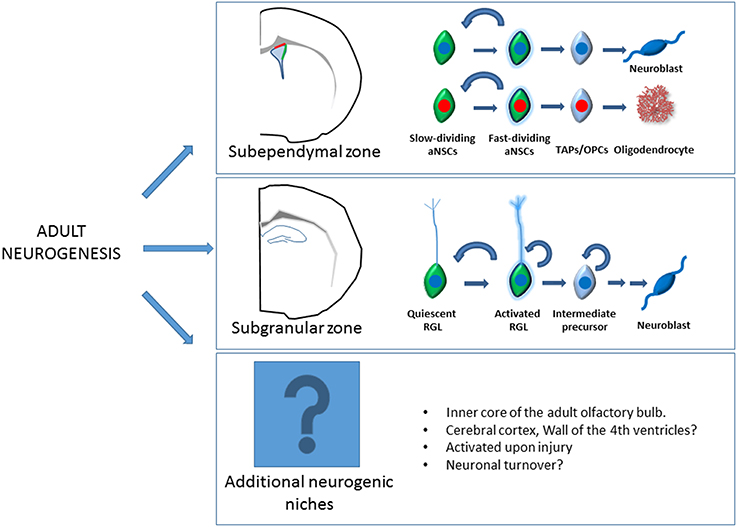
Figure 1. Schematic representation of the adult neurogenesis. Here there are depicted the two main adult neurogenic niches, the subependymal zone in the lateral wall of the lateral ventricle and the subgranular zone in the hippocampus. Live imaging experiments have shown than within the SEZ, neurogenic, and oligodendrogliogenic lineage follows a similar pattern of lineage progression but constitutes independent lineages. Slow dividing astroglia (quiescent type B cells) give rise to fast dividing astroglia (activated type B cells) that subsequently generates Transit amplifying progenitors (TAPs) and finally neuroblast or oligodendrocytes. In the SGZ, quiescent radial glia like (RGL) progenitors become activated giving rise to intermediate progenitors and neuroblast that undergoes a complex process of maturation. Additional neurogenic niches like the olfactory bulb or the cerebral cortex have also been reported. The existence of undiscovered neurogenic niches should not be discarded. Several regions of the adult brain reactivate “dormant” aNSCs through signaling pathways released upon injury. Likewise, contribution of these quiescent aNSCs to the periodical turnover of neural populations still remains to be demonstrated.
Focusing on the two main neurogenic niches of the adult brain, the SEZ harbors a population of aNSCs, known as type B cells, located beneath the ependymal cell layer of the lateral ventricles (Doetsch et al., 1999a,b). Type B has been proposed to share a common lineage with embryonic radial glia (RG) (Merkle et al., 2004). However, whether type B cells constitute the endpoint or RG lineage progression or whether the divergence arrives earlier is a matter of debate. Recent research went into detail about the relationship between RG and aNSCs (Fuentealba et al., 2015; Furutachi et al., 2015). Interestingly, evidences suggest that RG give rise to a subset of progenitors named pre-B1 cells at early stages of embryonic neural development (E 13.5-E 15.5). Pre-B1 cells remain quiescent until adulthood when they become reactivated. Furutachi and colleagues, points to the slowing down of the cell cycle of a subset of RG as the responsible for the origin to the adult pool of NSCs. Moreover, the cyclin-dependent kinase inhibitor p57 is proposed as the key regulator of the RG cell cycle. Selective deletion of p57 blocked the deceleration of the cell cycle, impairing the emergence of adult NSCs. These evidences are also supported by long-term in vivo experiments that described, using the STARTRACK tracing system, the presence of adult progenitors with embryonic origins (E-14), remaining as small, round and quiescent cells on the SEZ (Garcia-Marques and Lopez-Mascaraque, 2013). Type B cells are characterized by the expression of astroglial proteins such as the glial-fibrillary acidic protein (GFAP) and glutamate-aspartate transporter (GLAST; Doetsch et al., 1997; Platel et al., 2009). These cells contact the lateral ventricle through a single primary cilia located in the apical zone where they form junctional complexes among themselves (Mirzadeh et al., 2008). They also exhibit long basal processes that are closely associated with blood vessels, suggesting that vascular derived signals could play a role of the in the regulation of aNSC behavior (Mirzadeh et al., 2008; Shen et al., 2008; Tavazoie et al., 2008; Calvo et al., 2011). Regarding the cell cycle, type B cells are relatively quiescent (Doetsch et al., 1999a) but become activated giving rise to activated astroglia and type C cells or transit amplifying progenitors (TAPs; Pastrana et al., 2009; Costa et al., 2011). TAPs are more active in proliferation, undergoing several rounds of amplifying divisions (Costa et al., 2011; Ponti et al., 2013) before giving rise to neuroblasts or Type A cells. Subsequently, neuroblasts form a chain migrating along the rostral migratory stream toward the olfactory bulb (Lois et al., 1996). After reaching the olfactory bulb neuroblasts differentiate into different subtypes of neurons (Lledo et al., 2006; Fiorelli et al., 2015). The majority becomes GABAergic granule neurons and a minority becomes GABAergic periglomerular neurons. In addition, a small percentage of neuroblasts, generated in the dorsal wall of the SEZ, turn into short-axon glutamatergic, or GABAergic juxtaglomerular neurons (Kosaka and Kosaka, 2008; Brill et al., 2009; Kiyokage et al., 2010) Moreover, at least four subtypes of OB interneurons are generated at the anterior ventral SEZ, arising from microdomains that correlate with the expression domains of the Nkx6.2 and Zic family of transcription factors (Merkle et al., 2014).
Specific molecular markers to identify type B cells population have not been available for a long time. However recent publications employed interesting combinations of markers to differentiate between quiescent and activated type B cells, allowing for their isolation. Vascular cell adhesion molecule 1 (V-CAM1) has been recently described as a marker for quiescent type B cells (Kokovay et al., 2012). Likewise, combination of GFAP, prominin1 and the absence or presence of Epidermal growth factor (EGF) receptors has been suggested as a valid approach for isolation of quiescent and activated type B cells, respectively (Beckervordersandforth et al., 2010; Codega et al., 2014). The use of EGF fluorescent ligands, combined with the CD24 (Calaora et al., 1996) and GFAP expression has also been employed to successfully isolate aNSCs and their progeny from the SEZ (Pastrana et al., 2009). Along the same line, Daynac et al. (2015) employed a triple staining based on the stem cell marker Lewis X (LeX/ssea 1) (Capela and Temple, 2002), EGFR and the neuroblast marker CD24 to isolate the different populations comprised within the lineage progression.
Adult SEZ does not only provide the olfactory bulb with new neurons. In this region, astrocytes and oligodendrocytes are also generated and migrate toward the corpus callosum, rostral migratory stream, white matter tracts of the striatum and the fimbria fornix, under both physiological conditions (Hack et al., 2005; Menn et al., 2006; Gonzalez-Perez and Alvarez-Buylla, 2011; Sohn et al., 2015; Tong et al., 2015), and demyelinating pathologies (Picard-Riera et al., 2002).
The other main area of neurogenesis in the adult brain is the SGZ, which produce dentate gyrus granular neurons involved in learning and memory (Shors et al., 2002; Zhao et al., 2008). This area, located at the interface of the granule cell layer and the hilus, harbors two types of neuronal progenitors. Type 1 progenitors display a radial glia-like morphology with a long process that crosses the entire granule cell layer and small processes horizontally oriented along the SGZ. These progenitors are characterized by the expression of GFAP, Nestin, and Sox2 (Seri et al., 2001; Fukuda et al., 2003; Garcia et al., 2004). Type 2 is the second population of progenitors that may arise from type 1 cells, have only short processes and lack GFAP expression. Type 1 and Type 2 progenitors give rise to intermediate progenitors, which in turn generate neuroblasts (Filippov et al., 2003; Fukuda et al., 2003). Subsequently, immature neurons migrate to the inner granule cell layer and differentiate into dentate granule cells in the hippocampus projecting axons through the hilus toward the CA3 region. These newborn neurons undergo a long process of maturation acquiring finally the electrophysiological and functional properties of their mature partners (reviewed by Ming and Song, 2011; Mongiat and Schinder, 2011; Kempermann et al., 2015).
The regulation of the cell fate specification of aNSCs is a critical point of discussion in which the balance between the role of intrinsic and extrinsic/environmental signaling is still not fully clarified. Recent studies have shown that within the SEZ internal programs would have a dominant role. According to these studies, SEZ seems to be highly regionalized, with neuronal progeny of distinct identity being generated at different areas along the dorsoventral and rostrocaudal axes (Hack et al., 2005; Merkle et al., 2007; Brill et al., 2009). Thus, aNSCs from the SEZ could be intrinsically specified on the basis of their location and they may display a limited potential of differentiation. Progenitors located along the SGZ on the other hand, seem to be more instructed by their microenvironment signals, regardless of the regional origin of the NSCs. For a detailed review of the existing knowledge of adult neurogenesis and the molecular mechanisms controlling it see (Zhao et al., 2008; Kriegstein and Alvarez-Buylla, 2009; Suh et al., 2009; Ming and Song, 2011).
In contrast to this more deterministic view, growing evidence suggest that SEZ progenitors exhibit a higher degree of plasticity, retaining the potential to generate larger diversity of neuronal subtypes (Sequerra et al., 2013; Fiorelli et al., 2015). Indeed, SHH signaling in the ventral SEZ is necessary for the generation of ventral-associated OB neurons (Ihrie et al., 2011; Merkle et al., 2014). Disruption of the SHH signaling redirects the ventral progenitors to a more dorsal cell fate, whereas overexpression of Smoothened leads to a “ventralization” of the dorsal SEZ (Ihrie et al., 2011). Likewise, exposure of postnatal and adult SEZ explants to an embryonic cortical environment, triggered an enhanced generation of glutamatergic neurons in addition to the predominant GABAergic subtypes (Sequerra et al., 2010). In addition, infusion of gamma amino butyric acid (GABA) into the OB induces the differentiation of newly generated cells into dopaminergic neurons (Akiba et al., 2009), suggesting a role for electrical activity in fate specification of those neurons. Thus, a parsimonious explanation for those data is that adult NSCs inherit genetic patterning of the embryonic RG (Merkle et al., 2007; Fuentealba et al., 2015), but this pattern is actively maintained by morphogenic signals and electrical activity in the adult SVZ. Changes in the external conditions could drive aNSCs into different neuronal lineages despite their embryonic origin and location in the SEZ.
Live Imaging of Adult Neural Stem Cells: Applications and Limitations
Understanding the mechanisms controlling the lineage progression of the adult neural stem cells (aNSCs) is critical for the future design of therapies against neurodegenerative diseases. However, the majority of the current knowledge regarding adult neurogenesis comes from population studies in vivo, leaving critical questions regarding the cell biology of the aNSCs unsolved. For example, how stem cells enact the decisions of self-renewal and differentiation on a single cell level is far from being understood. In fact, there is an ongoing discussion in the stem cell field whether such fate decisions are stochastic or deterministic in nature. Likewise, it is a matter of intense debate the concept of multipotency regarding aNSCs, i.e., whether a single aNSC is capable of differentiating into all the different cell types within their lineage or these distinct progenies constitute entirely separate lineages instead. Several studied reported that aNSCs exhibit multipotency when isolated in vitro from both rodents (Reynolds and Weiss, 1996; Gritti et al., 2002; Reynolds and Rietze, 2005; Vergano-Vera et al., 2009) and human brain (Sanai et al., 2004). However, this conclusion is mostly supported by experiments using the neurosphere assay, which has important caveats for assessing clonality (Singec et al., 2006; Pastrana et al., 2011). Moreover, as we will discuss in more details below, those studies also share the common feature of continuous exposure to mitogen factors, which can exert confounding effects on cell fate decisions (Doetsch et al., 2002; Costa et al., 2011). In contrast, recent evidences points to a more restricted outcome of the lineage progression, suggesting the bi-potency or even unipotency as the main hallmark of aNSCs in both the SEZ and the DG (Bonaguidi et al., 2011; Encinas et al., 2011; Ortega et al., 2013; Calzolari et al., 2015). That opens an interesting debate of which factors (as suggested by Ravin et al., 2008; Vergano-Vera et al., 2009) may re-direct the NSCs toward multipotency. Similarly, it is crucial to understand the mechanisms instructing aNSCs toward a specific cell fate in vivo and whether distinct populations of aNSCs contributing neurons or macroglial cells show different behaviors in lineage progression, such as cell cycle length, mode of cell division, and number of cell divisions (symmetric vs. asymmetric; Costa et al., 2011; Ortega et al., 2011, 2013; Ponti et al., 2013). Improved knowledge regarding all these questions will be determinant to understand how the generation of neurons, astrocytes, and oligodendrocytes is regulated in the adult germinative niches, allowing for the design of future therapeutic strategies focused on the replenishment of the cell loss due to pathological scenarios.
Lineage tracing, the identification, and monitoring of all the progeny of a single progenitor hold the key to elucidate these crucial interrogations. Ideal lineage tracing implies label retaining and specific marking of the founder of the clone (aNSC) and all its progeny without spreading the labeling to unrelated cells and without modifying the aNSC/progeny behavior. Several approaches have been used in order to perform lineage tracing: Dyes and radioactive tracers, viral transduction, transplantation, genetic recombination, and multicolor reporter constructs were used both in vivo and in vitro, in order to follow the NSCs and their progeny (reviewed by Kretzschmar and Watt, 2012). All these techniques enclose positive aspects but imply one main and critical drawback: the results and conclusions are based on still pictures along the lineage progression without comprising the whole sequence. This means that cell death, diffusion, dilution, spreading or low efficiency of the markers, among other important shortcomings could lead to incorrect conclusions Figure 2.
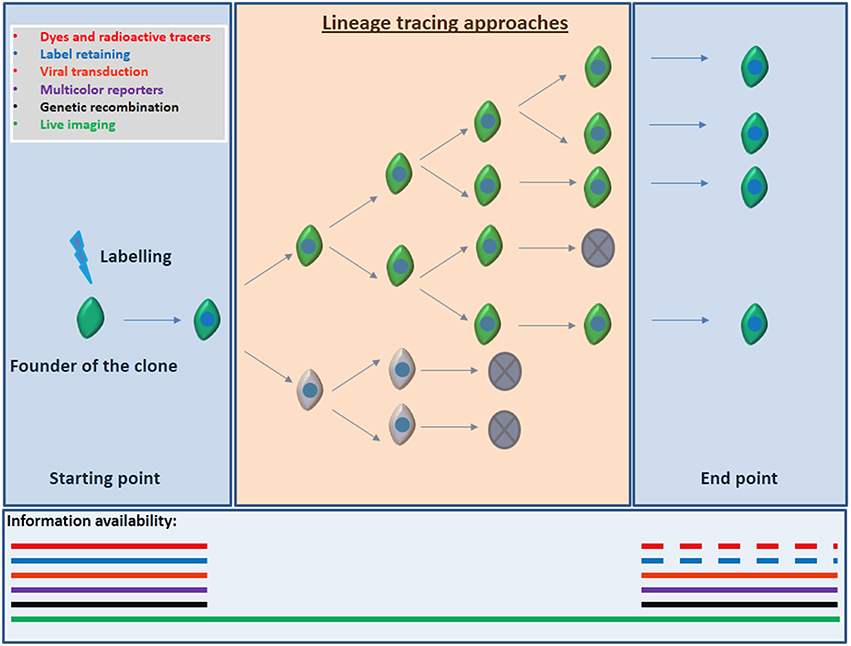
Figure 2. Information available according to the lineage tracing approach employed. Most of the lineage tracers implies a readout based on static end-point experiments. That implies that information regarding cell death, proliferation, differentiation, cell fate decisions, and migration inside or outside of the field of view of cell populations might be missing. Discontinuous segments of lines depict the lack of information that may also occur when using dyes or label retaining due to the dilution of the signal after several rounds of proliferation. Conversely, direct observation by live imaging allows for the visualization of each event from the founder of the clone, till the clone is formed improving the strength of the conclusions obtained from the experiment.
Although, constituting one of the oldest strategies for lineage tracing (Conklin, 1905; Sulston et al., 1983), live imaging followed by single cell tracking remains as the ideal method for the study of aNSCs lineage progression. Indeed, continuous observation of cell lineages over time allows studying the precise relations between a founder aNSC and its final clone, depicting in detail, with the adequate temporal resolution(Ravin et al., 2008; Eilken et al., 2009; Rieger et al., 2009), every intermediate step during the lineage progression. Using live imaging techniques in vitro we could predict crucial characteristics of the aNSCs cell biology (Costa et al., 2011; Ortega et al., 2011) later confirmed by BrdU-chasing or multicolor based clonal analysis in vivo (Ortega et al., 2013; Ponti et al., 2013; Calzolari et al., 2015). However, technical issues currently limit a comprehensive analysis of aNSCs lineage progression in vivo (see discussion below). Here, we will review some of the current techniques that have been developed to study the biology of the aNSCs by using live imaging in combination with single cell tracking. We will highlight the main advantages and limitations of both in vitro and in vivo approaches and discuss possible future directions toward a full understanding of aNSCs behavior, a crucial requirement in order to design future therapy strategies.
Live Imaging of aNSCs in vitro
Before describing in detail the present in vitro analysis that applied live imaging to the study of aNSCs, it is important to mention that the majority of the in vitro studies using aNSCs has been carried out by using the classical in vitro test for stem cell self-renewal, the neurosphere assay (Reynolds and Rietze, 2005). This method has been widely used to identify NSCs based on their capacity to evaluate self-renewal and differentiation at the single-cell level in vitro. However, as already discussed, several evidence indicate that the neurosphere assay may not be appropriate (Singec et al., 2006; Pastrana et al., 2011). Among several problems, is especially important the fact that clonality is not always guaranteed. Moreover, quiescent stem cells may not be detected by the neurosphere assay, in fact it was demonstrated that EGFR positive cells, i.e., activated type B cells and TAPs, are responsible for the generation of neurospheres (Doetsch et al., 2002; Pastrana et al., 2009). This clearly demonstrate that neurosphere assay does not reflect an accurate readout of the number of stem cells in vivo. In addition, this approach does not allow addressing the question of the stem cell mode of cell division due to its high cell density. Finally, the assay depends on the exposure of NSCs to mitogenic growth factors with their well-known confounding effects on cell fate decisions (Doetsch et al., 2002; Costa et al., 2011).
Therefore, live imaging of aNSCs required the development of new methods to perform successful and reliable single cell tracking. As we previously mentioned, the ideal situation would be the long-term imaging of single cells in whole living organisms, in real time and using several molecular readouts. However, the present available technology makes impossible the long-range immobilization of the organism as well as the access to the adult neurogenic areas (SEZ and SGZ) without the employment of highly invasive, and thus non-viable, approaches. In contrast, live imaging can be performed up to weeks in vitro, which allows for the analysis and manipulation of several molecular properties of aNSCs. Moreover, in vitro analysis provides crucial information regarding cell morphology, cell growth, migration, cell divisions patterns, cell cycle length, and cell fate decisions undergone by aNSCs (Costa et al., 2011).
There are several requirements to perform a good quality long-term live imaging experiment (reviewed in Schroeder, 2011). Live imaging followed by single cell tracking requires a complex balance between the imaging optimizations and the viability of the culture. Long or frequent exposition to excitation light, periodical changes in cell culture medium, adequate regulation of the gas composition of the incubator, among others, are technical aspects that may influence cell stress and toxicity. Moreover, the researcher has to establish carefully the settings of several peripheral components. For example, long-term live imaging requires adequate microscope systems. Bright-field and phase contrast are the systems most frequently used, in combination with motorized components that make possible a level of automation, indispensable for long term experiments (i.e., stages, shutters, and filters). Likewise the researcher has to count on incubation and permeable plates system able to keep the correct conditions of CO2 saturation and pH during the developing of the assay. Furthermore, the correct storage and subsequent processing of the results (i.e., images) requires expeditious hardware and software to deal properly with the huge amount of data that implies an experiment of live imaging. Finally, the accomplishment of the live imaging experiments depends often on the availability of specific fluorescent markers that allow the identification of the target population in real-time (Eilken et al., 2014).
Combining long term time-lapse video-microscopy with cultures seeded at clonal density Qian and colleagues provided important improvements in the knowledge of the behavior of embryonic NSCs. Culturing embryonic day (E)13 murine NSCs in medium supplemented with FGF2 they observed that the majority of the clones produced mainly neuroblast. These clones formed a heterogeneous population regarding their capacity of division, undergoing different rounds of amplifying divisions prior to the neuronal differentiation. Moreover, they were able to identify lineages containing both “neuroblast” and “glioblast” progenitors in these cultures (Qian et al., 1998). Lately, the use of time-lapse video-microscopy revealed that the production of neurons and glia followed a defined temporal pattern. Live imaging of different time points of embryonic NSCs isolated in culture indicated that neuroblast are generated first, whereas glia arise later. Furthermore, they confirmed that after the production of neuroblast and glioblast, NSCs in culture acquire features of postnatal progenitors, modifying their response to EGF (Qian et al., 2000). Recently, embryonic cultures (E12.5) in combination with time-lapse video-microscopy and computational processing and tracking have also been employed to elucidate behavioral differences between anterior (motor cortex-related) and posterior (visual cortex-related) neural progenitors (Winter et al., 2015). Single cell tracking of both populations indicate that posterior cortical progenitors exhibit higher speed in the cell cycle, increased cell size, and more motility than the anterior ones. As a consequence of the difference in the cell cycle length, the posterior progenitors generates larger clones that the anterior counterparts.
Clonal density cultures have been also used to image the lineage progression of rat or zebrafish embryonic retinal progenitor cells. Live imaging of single clones, controlling the composition of the extracellular environment and restricting completely the interaction between different clones demonstrated an unexpected degree of stochasticity in the progenitors regarding the decision between proliferation or differentiation into the different cell types located in the retina (Gomes et al., 2011; He et al., 2012). As we described previously for the aNSCs located in the SEZ, exits also an important line of research in retina suggesting that within the progenitor pool of the retina there is an important molecular heterogeneity. This heterogeneity would be determined by the presence of intrinsic genetic programs but also as a plastic response of progenitors to the influence of instructive extrinsic signals as Notch, Shh, GDF11 etc. (Cepko et al., 1996; Livesey and Cepko, 2001; Cayouette et al., 2006; Riesenberg et al., 2009).
Several modifications have been developed in order to improve the efficiency of the live imaging protocols. The use of extracellular matrix proteins in the coating defined a limited area where the cells are allowed to grow, creating then, a restricted environment that make the live imaging easier and more accurate. Combining this method with the manufacturing of a culture chamber that controls media and gas exchange over the microscope stage, Ravin and colleagues performed long term timelapse video-microscopy of E-14.5 NSCs. Manual reconstruction of the NSCs lineage trees revealed that under these culture conditions and in presence of FGF2, tripotent NSCs generate unipotent progenitors through an intermediate bipotent step, involving short phases of self-renewal within each independent stage. They also demonstrate that periodical passage of the progenitors in culture retrieve the tripotent capacity to the NSCs (Ravin et al., 2008).
Other significant improvements in the live imaging of NSCs involve techniques that allow the combination of time-lapse video-microscopy and single cell tracking in high density cultures instead of the previous clonal density. The application of these methods to the embryonic NSCs rendered the discovery of a new population of progenitors located in the marginal zone of the mouse cerebral cortex. This new embryonic niche harbors oligodendrogliogenic, astrogliogenic, and neurogenic progenitors as well as some bipotent NSCs (Costa et al., 2007). Another example was the descriptions of the role of Par-complex proteins in the lineage progression of embryonic NSCs (Costa et al., 2008). Par-complex proteins exhibit an apical gradual expression, being enriched in the ventricular radial glia and decreasing subsequently along the neurogenesis process. Time-lapse video-microscopy demonstrated that gain of function of Par3/Par6 proteins promoted self-renewal of ventricular progenitors whereas loss of function forced them to exit the cell cycle, regulating then, their mode of cell division (Costa et al., 2008). Finally, the use of high density cultures to further study the cell fate switch undergone by cortical NSCs during embryogenesis demonstrated that virtually all cortical progenitors generate neurons before giving rise to glia-committed progenitors (Costa et al., 2009).
Several researchers have tried to establish an automated method of tracking the lineage progression once the live imaging is accomplished. Different computational methods have been tested in murine embryonic NSCs cultures in order to monitor automatically the migration, proliferation, and cell growth of the clones along the timelapse video-microscopy, generating a lineage tree as a read out (Al-Kofahi et al., 2006; Winter et al., 2011). Likewise, Cohen and colleagues employed an automated algorithmic-information-theory-based method in order to obtain morphological and behavioral patterns. This data was used to predict with high levels of accuracy the mode of division (self-renewal vs. differentiation) and the cell fate decision (generated progeny) of rat retinal progenitors cells in culture (Cohen et al., 2010). However is important to mention here that, in contrast to retinal progenitors, most of the stem cells do not display an obvious characteristic morphology immediately after plating or along the culture. Although, some important improvements have been achieved in orders to reduce human validation in embryonic NSC cultures at clonal density (Winter et al., 2011, 2015), culture of NSCs requires often higher cell density, leading to a fast expansion and mixing of the clones generated depicting a much more complex scenario where current automated methods produce too many errors and requires of inevitable manual correction and interaction.
In contrast to their embryonic counterparts, aNSCs were mostly studied using neurosphere assay and other few culture systems conveying high concentrations of mitogenic factors (FGF2, EGF, or both) in culture medium. As we previously mentioned, these factors induce important alterations in the behavior of aNSCs (Doetsch et al., 2002; Costa et al., 2011) and may lead to inaccurate conclusions regarding the potency of these cells. Likewise, the use of live imaging on the study of the lineage progression of NSCs has long been restricted to the field of embryonic development, due to the technical limitations involved in singe-cell tracking in the neurosphere assay. To overcome these limitations, several laboratories developed adherent SEZ cultures that replicate some aspects of the SEZ in vivo (Lim and Alvarez-Buylla, 1999; Scheffler et al., 2005). Scheffler et al. reported an adherent culture, which involves expansion of stem/precursor cells in presence of EGF/FGF2 prior to differentiation (Scheffler et al., 2005). However, following this protocol, neural stem cells adopt tumorigenic properties (Walton et al., 2009), an effect that can be attributed to growth factor treatment (Kuhn et al., 1997; Doetsch et al., 2002). Lim and Alvarez-Buylla developed an interesting strategy of co-culturing adult SEZ cells with postnatal astroglia, which circumvent the necessity of growth factors for maintaining proliferation and neurogenesis. However, the requirement of such astroglial feeder introduces new uncontrollable variables: astroglia themselves secrete both growth factors and extracellular matrix molecules, likely affecting the intrinsic properties of adult neural stem cells; they introduce direct cell-contact mediated effects; they preclude analyse of direct effects of both intrinsic and extrinsic factor over SEZ cells, as astroglia feeder cells would also be affected; finally, due to the high cell density in such co-cultures single cell tracking becomes more cumbersome.
To circumvent these limitations, we recently developed a new method of culturing adult NSCs under adherent conditions and in absence of mitogenic factors (Costa et al., 2011; Ortega et al., 2011; see Table 1). Using this culture method, it was possible to monitor in real-time the lineage progression of aNSCs isolated from the lateral wall of the SEZ up to several days (Costa et al., 2011; Ponti et al., 2013). This technique allowed the direct observation of the intermediate steps between the progression from aNSCs to neurons: slow-dividing astroglia (type B cells) give rise to fast-dividing astroglia, which in turns generates TAPs. TAPs undergo subsequently several (up to 5) rounds of division giving rise to post-mitotic neuroblast in an orderly manner. These observations are in accordance with the sequence proposed by population-based studies in vivo (Doetsch et al., 1999a), but added two new pieces of information: (i) slow-dividing astroglia generate fast-dividing astroglial cells before TAPs and (ii) the number of cell divisions from aNSCs to the final neuronal progeny is limited. Moreover, this live-imaging system allowed the direct observation of asymmetric cell divisions within the lineage of aNSCs, providing a unique model for the study of NSC self-renewal. Interestingly, the asymmetry in the lineage is not observed in the first aNSC division, but rather at later points in the lineage. Finally, we could observe morphological changes characteristic of each cell type, such as the substantial cell growth prior to cell division of aNSCs, and the high motility of immature bipolar neuroblasts shortly after cell division.
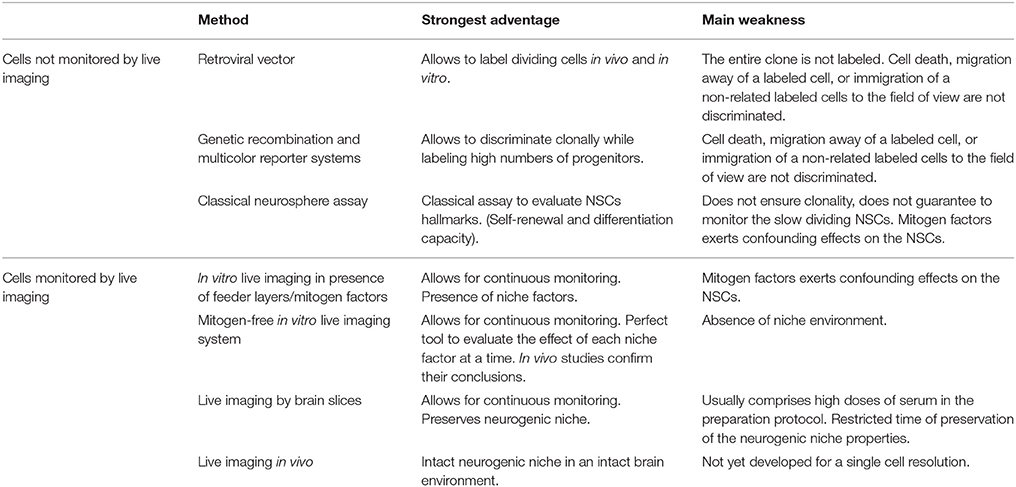
Table 1. Different methods employed for lineage tracing of aNSCs, highlighting some of their strongest advantages, and main weaknesses.
This method was also applied to the study of other cell population generated in the adult SEZ, the oligodendrocytes (Menn et al., 2006; Gonzalez-Perez and Alvarez-Buylla, 2011). When the lateral and dorsal wall of the adult SEZ were cultured together, an increase in the number of oligodendroglial cells was observed, indicating, and enrichment of oligodendrogliogenic aNSCs in the dorsal aspect of the SEZ (Ortega et al., 2013). Timelapse video microscopy followed by single cell tracking revealed that aNSCs generating oligodendroglial progeny in vitro share several hallmarks (cell growth, slow cell cycle, etc.) with neurogenic aNSCs. Moreover, oligodendrogliogenic and the neurogenic aNSCs constitute strictly separate lineages, giving rise to either neurons and glia or oligodendrocytes and glia, respectively. Interestingly, we observed that the oligodendrogliogenic progeny is selectively activated by Wnt canonical signaling, known to be particularly prominent in the dorsal SEZ in vivo (Marinaro et al., 2012). Presence of Wnt recombinant proteins in the culture medium increased the rounds of amplifying divisions undergone by oligodendroglial progenitors by shortening their cell cycle. The expansion of the oligodendrogliogenic lineage induced by Wnt signaling within the SEZ has also been corroborated in vivo in both the adult (Ortega et al., 2013) and the postnatal (Azim et al., 2014) SEZ. It will be interesting to study the lineage progression of aNSCs when exposed to other extrinsic factors known to affect neurogenesis and oligodendrogenesis in the SEZ, such as BMP (ventral; Colak et al., 2008) and Shh (dorsal; Merkle et al., 2014; Tong et al., 2015).
One of the main criticisms regarding the study of cell behavior in culture is precisely that the progenitors are isolated from their “stem cell niche environment.” Therefore, important signals that regulate the aNSCs lineage progression could be missing and then, their behavior could be altered. Nevertheless, live-imaging of adherent SEZ cells under serum-free culture conditions allow to reproduce important steps in the lineage-progression of aNSCs and to describe new phenomena previously unnoticed (Costa et al., 2011; Ortega et al., 2011). In fact, results in vivo (Ponti et al., 2013) confirmed our observations in vitro (Costa et al., 2011) regarding aNSCs lineage progression and the number of cell divisions enacted in the lineage, indicating that important features of aNSCs are maintained in our culture system, despite the absence of niche signals. It is likely that SEZ cells isolated and observed in our experiments had already received environmental influences and the main merit of the system is not to use factor affecting the development of cell lineages.
Organotypic-slice cultures also constitute an interesting ex-vivo approach to study cell-divison and migration over short periods of time in embryonic (Noctor et al., 2004), posnatal (Raineteau et al., 2004; Namba et al., 2007; Yokose et al., 2011), and adult brain (Kamada et al., 2004). Combination of organotypic cultures, time-lapse microscopy, and two-photon microscopy uncovered interesting features of the motility of the SEZ-derived neuroblast (Nam et al., 2007; James et al., 2011). Contrary to expectations, it was shown that neuroblast migratory chains remain stable and immotile for longer periods. Moreover, brain slice cultures were also employed to demonstrate that adult NSCs are capable to regulate the local blood flow within the neurogenic niche by releasing ATP and vasodilating factors (Lacar et al., 2012). Organotypic cultures were also used to described alternatives models of oligodendrocytic-dependent myelination (Sobottka et al., 2011). However, to the best of our knowledge, aNSC lineage progression has not been comprehensively studied in organotypic culture. One important challenge in this system is to maintain healthy slices over long periods of time. In fact, neural precursors have a limited capacity to differentiate into neurons and the structural integrity of the neurogenic niche seems to be compromised during the culture period (Namba et al., 2007). One possible explanation to these problems is the high concentration of serum required to keep slices alive. Lastly, it is important to consider that the different steps observed in the aNSC lineage start in the SEZ, progress over the RMS and end in the olfactory bulb. Therefore, the slice must keep all these structures intact and the live-imaging set up to follow all those steps must be able to image cells at long distances. Nevertheless, we believe that organotypic cultures could be improved, allowing longer survival periods and more physiologic neurogenic rates, and then used to monitor aNSC behavior within the SEZ.
Live Imaging of aNSCs in vivo
As discussed in the previous sections, one potential pitfall of studying aNSCs in vitro could be the absence of the niche. In fact, organotypic-slice cultures may keep some local relations, but do not maintain long-distance axonal influences involved in the control of aNSCs behavior (Tong et al., 2014). Thus, the perfect situation would be to be able to image aNSCs within their niche under physiological conditions in vivo. However, imaging techniques so far available to image the brain in vivo do not allow the study of aNSCs in a single-cell manner.
Up to date, there are three main imaging techniques used to image NSCs and neurogenesis in the adult live brain: Magnetic resonance imaging/spectroscopy (MRI/MRS); Positron emission tomography (PET); and optical imaging (fluorescence and bioluminiscence). However, as discussed below, each of these techniques has limitations that preclude their use to study the behavior of single cells in the live brain.
MRI offers a relatively high morphological resolution in deep regions of the brain (78 × 78 × 370 μm) (Weber et al., 2006). However, this resolution does not allow studying single cells. In fact, MRI has been mostly used to evaluate volumetric modifications in regions where neurogenesis occurs under physiological or pathological conditions and to correlate these changes with possible alterations in the rate of neurogenesis (Couillard-Despres and Aigner, 2011).
To identify specific cell population using MRI, some researches have used iron oxide particle labeling of neural stem and precursors cells in vitro and then transplanting labeled cells into aNSC niches in vivo (Hoehn et al., 2002). Using this technique, it has been possible to image transplanted cells over several weeks in the host brains and follow their migration. However, in vitro labeling of cells may lead to changes in several properties of the cells, hampering the enthusiasm regarding the use of such technique to study the physiological behavior of aNSCs.
In order to overcome this limitation, some groups have attempted to label endogenous neural stem/precursos cells in vivo through the injection of iron particles directly into the lateral ventricles or in the subventricular zone. These works have shown that particles can be uptaken by migrating neuroblasts, allowing the observation of their migration toward the olfactory bulb by MRI. Thus, particle-labeling of cells is a promising technique to study adult neurogenesis in vivo by non-invasive imaging (Panizzo et al., 2009; Granot et al., 2011; Iordanova and Ahrens, 2012).
Nevertheless, several issues must be taken into consideration in these studies: (i) uptake of particles by SVZ cells is unspecific, in other words, it is not possible to distinguish which type of cell (ependymal, type B, C, or A cell) is being observed; (ii) injection of iron particles in vivo may lead to cell toxicity (Crabbe et al., 2010; Vreys et al., 2010); and (iii) iron particles may be uptaken by other cells following death of the primary-carrier cells, which could cause a great confusion in the analysis of data. Together, these technical issues embody serious limitations to the use of MRI to study aNSC behavior in vivo.
An elegant way to identify specific cell populations has been proposed by Manganas et al. (2007). They used MRS to identify possible “metabolic biomarkers” for different cell types in the adult hippocampus. For example, they reported a MRS peak, detected at 1.28 ppm (parts per million), which seemed to correlate with the presence of neural stem cells (Manganas et al., 2007). The authors reported an increase in the 1.28-ppm biomarker in the dentate gyrus of rodents following electric convulsive shock, which could be interpreted as a sign for increased endogenous neurogenesis. Conversely, they described a reduction of the 1.28-ppm biomarker in the hippocampus of adult humans, as compared to preadolescents and adolescents. Based on these findings, they concluded that the 1.28-ppm biomarker could be applied to track and analyze endogenous NSCs in vivo (Manganas et al., 2007). However, Ramm et al. (2009) demonstrated that the 1.28-ppm peak was also evident in mesenchymal stem cells and in non-stem cell lines. Notably, this peak could only be observed in neural progenitor cells cultured under conditions favoring growth arrest or apoptosis, suggesting that such peak could be caused by the appearance of mobile lipid droplets during apoptosis (Ramm et al., 2009). Therefore, the validity of the 1.28-ppm biomarker as a measure for NSCs and neurogenesis requires additional evidence.
PET has also been used to study neurogenesis in the rodent adult brain (Rueger et al., 2010). Using the 18F-labeled 3′-deoxy-3′-fluorothymidine (FLT), a thymidine analog incorporated to newly synthesized DNA molecules, they could observe an increase in the PET signal both in the hippocampus and subventricular regions in the ipsilateral hemisphere subjected to ischaemic lesion (Rueger et al., 2010). However, although such increase has been interpreted as readout for augmented neurogenesis, the signal intensity observed in the hippocampus and SVZ seemed very similar, even though neurogenesis in the SVZ is 10-100 times higher. One possible explanation for this discrepancy could be the low resolution of PET in comparison to MRI.
Needless to say, incorporation of 18F-FLT is unspecific and, consequently, does not allow the identification of cell types imaged by PET. Thus, the use this technique to study endogenous NSCs and neurogenesis in vivo remains a forthcoming alternative.
Compared to MRI/MRS and PET, optical imaging based in fluorescence or bioluminescence is a cheaper and more versatile method to study cells in vivo. Using any microscope equipped for epifluorescence acquisition, for instance, it is possible to exploit all the advantages of transgenic animals expressing fluorescent proteins controlled by specific promoters or virally-transduced markers, prompting the identification of specific cell types, and achieve resolution sufficient for morphological studies at cellular and subcellular levels.
Although, light penetration in the live brain is an important drawback for fluorescence live imaging in the SVZ and hippocampus, the advent of multi-photon confocal microscopy has significantly improved the quality of fluorescence imaging in deep regions of the brain (Lendvai et al., 2000; Grutzendler et al., 2002; Trachtenberg et al., 2002). In a seminal work, Mizrahi et al. used two-photon microscopy to image the olfactory bulb of transgenic mice expressing GFP in juxtaglomerular neurons (JGNs), a population that undergoes adult neurogenesis, over periods of up to 3 months, in vivo. Based on GFP identification, they could perform time-lapse analysis and demonstrate that JGNs in the olfactory bulb have a turnover rate of about 3% per month (Mizrahi et al., 2006). Recently, this imaging technique has been combined to treatment with calcium indicators and used to study the functional integration of newly generated neurons in the adult rodent olfactory bulb (Kovalchuk et al., 2015).
However, neurogenic niches in the SVZ and hippocampal DG exist in much deeper brain regions as compared to the regions studied in the above cited work (Lendvai et al., 2000; Grutzendler et al., 2002; Trachtenberg et al., 2002; Mizrahi et al., 2006), hampering the use of two-photon microscopy to study endogenous aNSCs.
Recently, however, the group of David W. Tank has developed a preparation to image adult hippocampal cells in wake animals (Dombeck et al., 2010). In order to image neuron activity in CA1 during navigation, the authors removed the overlaying cortical tissue by aspiration and placed a stainless cannula that functioned as a window to CA1 of adult rats. Two-photon time-lapse microscopy of CA1 neurons labeled with the calcium indicator GCaMP3 during navigation in a virtual reality system allowed the identification of place cells and the correlation between the location of their place fields in the virtual environment and their anatomical location in the local circuit (Dombeck et al., 2010).
These data indicate that deeper regions of the brain can be imaged following removal of covering structures. However, to the best of our knowledge, this has not yet been tested to image aNSCs either in the DG or SVZ. Furthermore, it is important to keep in mind that surgical procedures to remove brain tissue may have critical influences in aNSC behavior and neurogenesis. In fact, several models have shown changes in the rate of proliferation within neurogenic niches following injury (Arvidsson et al., 2002; Collin et al., 2005; Aponso et al., 2008; Saha et al., 2013).
Imaging of Local Progenitors in the Cerebral Cortex
It has also been proposed that cells with stem/progenitor potential reside within the cortical parenchyma and could be activated under specific condition, such as injuries. Because these cells are located in superficial regions of the brain, it is possible to study their behavior by two-photon time-lapse microscopy.
The cerebral cortex layer 1, the most superficial layer of the cerebral cortex, for example, harbors a population of progenitors capable of generating neurons and glial cells during development (Costa et al., 2007; Breunig et al., 2012). Although, neurogenesis from layer 1 progenitors seems to be restricted to embryonic and postnatal stages under physiological conditions, these progenitors may resume proliferation after ischemic injury in adult animals and generate neurons (Ohira et al., 2010). Given the matchless superficial location of layer 1 progenitors, they are good candidates to imaging by two-photon microscopy.
Another interesting cell population for imaging in the adult brain is the parenchymal astroglia. Following traumatic and ischemic injury, astroglial cells proliferate, and acquire several hallmarks of neural stem cells (Buffo et al., 2008; Sirko et al., 2013), leading to the suggestion that parenchymal astroglia could be an interesting source for neuronal replacement under different neurological conditions (Robel et al., 2011; Chouchane and Costa, 2012).
In a recent work, Bardehle et al. (2013) used in vivo two-photon laser-scanning microscopy to follow the response of GFP-labeled astrocytes in the adult mouse cerebral cortex over several weeks after acute injury. The authors described a selective proliferation of juxtavascular astrocytes after the introduction of a lesion, indicating that astrocyte recruitment after injury relies solely on proliferation in a specific niche (Bardehle et al., 2013). If these proliferating astrocytes are the cells acquiring neural stem cell properties in vitro, two-photon time-lapse imaging of these cells might bring some insights about aNSC behavior in vivo.
Conclusion
Live-imaging of isolated SVZ cells in vitro has contribute to deepen our knowledge about the lineage-progression of adult NSCs. However, in vivo imaging systems still lack resolution to resolve single-cell lineages. Future studies should address the contribution of individual NSCs to different neuronal lineages and the possible influence of signaling molecules in this process, both in vitro and in vivo. Long-term imaging in vivo, however, awaits future developments in microscopy that will permit the observation of cells generated in the SVZ and the following up of these cells through the RMS and in the OB. Alternatively, short time live-imaging of aNSCs within the SEZ in vivo combined with multicolored fate-mapping could help to describe how the progeny of single NSCs, identified in the olfactory bulb by multicolor codes, is generated.
Author Contributions
FO organized and coordinated the preparation of the present review. FO and MC contributed to the writing of the manuscript.
Funding
FO was supported by research grants MICINN (BFU2011-24743), Consolider-Ingenio 2010 “Spanish Ion Channel Initiative” (CSD2008-00005), MEC (BFU2014-53654-P), and BRADE-CM (S2013/ICE-2958) and the Ramon y Cajal Program of the Spanish Ministry of Economy and competitiveness (MEC) (RYC-2013-13290). MC is supported by CNPq, CAPES, and Fundação de Amparo à Pesquisa do Rio de Grande do Norte (FAPERN).
Conflict of Interest Statement
The authors declare that the research was conducted in the absence of any commercial or financial relationships that could be construed as a potential conflict of interest.
The reviewer CV and handling Editor declared their shared affiliation, and the handling Editor states that the process nevertheless met the standards of a fair and objective review.
References
Akiba, Y., Sasaki, H., Huerta, P. T., Estevez, A. G., Baker, H., and Cave, J. W. (2009). gamma-Aminobutyric acid-mediated regulation of the activity-dependent olfactory bulb dopaminergic phenotype. J. Neurosci. Res. 87, 2211–2221. doi: 10.1002/jnr.22055
Al-Kofahi, O., Radke, R. J., Goderie, S. K., Shen, Q., Temple, S., and Roysam, B. (2006). Automated cell lineage construction: a rapid method to analyze clonal development established with murine neural progenitor cells. Cell Cycle 5, 327–335. doi: 10.4161/cc.5.3.2426
Aponso, P. M., Faull, R. L., and Connor, B. (2008). Increased progenitor cell proliferation and astrogenesis in the partial progressive 6-hydroxydopamine model of Parkinson's disease. Neuroscience 151, 1142–1153. doi: 10.1016/j.neuroscience.2007.11.036
Arsenijevic, Y., Villemure, J. G., Brunet, J. F., Bloch, J. J., Deglon, N., Kostic, C., et al. (2001). Isolation of multipotent neural precursors residing in the cortex of the adult human brain. Exp. Neurol. 170, 48–62. doi: 10.1006/exnr.2001.7691
Arvidsson, A., Collin, T., Kirik, D., Kokaia, Z., and Lindvall, O. (2002). Neuronal replacement from endogenous precursors in the adult brain after stroke. Nat. Med. 8, 963–970. doi: 10.1038/nm747
Azim, K., Fischer, B., Hurtado-Chong, A., Draganova, K., Cantu, C., Zemke, M., et al. (2014). Persistent Wnt/beta-catenin signaling determines dorsalization of the postnatal subventricular zone and neural stem cell specification into oligodendrocytes and glutamatergic neurons. Stem Cells 32, 1301–1312. doi: 10.1002/stem.1639
Bardehle, S., Kruger, M., Buggenthin, F., Schwausch, J., Ninkovic, J., Clevers, H., et al. (2013). Live imaging of astrocyte responses to acute injury reveals selective juxtavascular proliferation. Nat. Neurosci. 16, 580–586. doi: 10.1038/nn.3371
Beckervordersandforth, R., Tripathi, P., Ninkovic, J., Bayam, E., Lepier, A., Stempfhuber, B., et al. (2010). In vivo fate mapping and expression analysis reveals molecular hallmarks of prospectively isolated adult neural stem cells. Cell Stem Cell 7, 744–758. doi: 10.1016/j.stem.2010.11.017
Belachew, S., Chittajallu, R., Aguirre, A. A., Yuan, X., Kirby, M., Anderson, S., et al. (2003). Postnatal NG2 proteoglycan-expressing progenitor cells are intrinsically multipotent and generate functional neurons. J. Cell Biol. 161, 169–186. doi: 10.1083/jcb.200210110
Bonaguidi, M. A., Wheeler, M. A., Shapiro, J. S., Stadel, R. P., Sun, G. J., Ming, G. L., et al. (2011). In vivo clonal analysis reveals self-renewing and multipotent adult neural stem cell characteristics. Cell 145, 1142–1155. doi: 10.1016/j.cell.2011.05.024
Breunig, J. J., Gate, D., Levy, R., Rodriguez, J. Jr., Kim, G. B., Danielpour, M., et al. (2012). Rapid genetic targeting of pial surface neural progenitors and immature neurons by neonatal electroporation. Neural Dev 7:26. doi: 10.1186/1749-8104-7-26
Brill, M. S., Ninkovic, J., Winpenny, E., Hodge, R. D., Ozen, I., Yang, R., et al. (2009). Adult generation of glutamatergic olfactory bulb interneurons. Nat. Neurosci. 12, 1524–1533. doi: 10.1038/nn.2416
Buffo, A., Rite, I., Tripathi, P., Lepier, A., Colak, D., Horn, A. P., et al. (2008). Origin and progeny of reactive gliosis: a source of multipotent cells in the injured brain. Proc. Natl. Acad. Sci. U.S.A. 105, 3581–3586. doi: 10.1073/pnas.0709002105
Calaora, V., Chazal, G., Nielsen, P. J., Rougon, G., and Moreau, H. (1996). mCD24 expression in the developing mouse brain and in zones of secondary neurogenesis in the adult. Neuroscience 73, 581–594. doi: 10.1016/0306-4522(96)00042-5
Calvo, C. F., Fontaine, R. H., Soueid, J., Tammela, T., Makinen, T., Alfaro-Cervello, C., et al. (2011). Vascular endothelial growth factor receptor 3 directly regulates murine neurogenesis. Genes Dev. 25, 831–844. doi: 10.1101/gad.615311
Calzolari, F., Michel, J., Baumgart, E. V., Theis, F., Gotz, M., and Ninkovic, J. (2015). Fast clonal expansion and limited neural stem cell self-renewal in the adult subependymal zone. Nat. Neurosci. 18, 490–492. doi: 10.1038/nn.3963
Capela, A., and Temple, S. (2002). LeX/ssea-1 is expressed by adult mouse CNS stem cells, identifying them as nonependymal. Neuron 35, 865–875. doi: 10.1016/S0896-6273(02)00835-8
Cayouette, M., Poggi, L., and Harris, W. A. (2006). Lineage in the vertebrate retina. Trends Neurosci. 29, 563–570. doi: 10.1016/j.tins.2006.08.003
Cepko, C. L., Austin, C. P., Yang, X., Alexiades, M., and Ezzeddine, D. (1996). Cell fate determination in the vertebrate retina. Proc. Natl. Acad. Sci. U.S.A. 93, 589–595. doi: 10.1073/pnas.93.2.589
Chouchane, M., and Costa, M. R. (2012). Cell therapy for stroke: use of local astrocytes. Front. Cell. Neurosci. 6:49. doi: 10.3389/fncel.2012.00049
Codega, P., Silva-Vargas, V., Paul, A., Maldonado-Soto, A. R., Deleo, A. M., Pastrana, E., et al. (2014). Prospective identification and purification of quiescent adult neural stem cells from their in vivo niche. Neuron 82, 545–559. doi: 10.1016/j.neuron.2014.02.039
Cohen, A. R., Gomes, F. L., Roysam, B., and Cayouette, M. (2010). Computational prediction of neural progenitor cell fates. Nat. Methods 7, 213–218. doi: 10.1038/nmeth.1424
Colak, D., Mori, T., Brill, M. S., Pfeifer, A., Falk, S., Deng, C., et al. (2008). Adult neurogenesis requires Smad4-mediated bone morphogenic protein signaling in stem cells. J. Neurosci. 28, 434–446. doi: 10.1523/JNEUROSCI.4374-07.2008
Collin, T., Arvidsson, A., Kokaia, Z., and Lindvall, O. (2005). Quantitative analysis of the generation of different striatal neuronal subtypes in the adult brain following excitotoxic injury. Exp. Neurol. 195, 71–80. doi: 10.1016/j.expneurol.2005.03.017
Conklin, E. G. (1905). The organization and cell lineage of the ascidian egg. J. Acad. Nat. Schi. Phila. 12, 1–19.
Costa, M. R., Bucholz, O., Schroeder, T., and Gotz, M. (2009). Late origin of glia-restricted progenitors in the developing mouse cerebral cortex. Cereb. Cortex 19(Suppl 1), i135–i143. doi: 10.1093/cercor/bhp046
Costa, M. R., Kessaris, N., Richardson, W. D., Gotz, M., and Hedin-Pereira, C. (2007). The marginal zone/layer I as a novel niche for neurogenesis and gliogenesis in developing cerebral cortex. J. Neurosci. 27, 11376–11388. doi: 10.1523/JNEUROSCI.2418-07.2007
Costa, M. R., Ortega, F., Brill, M. S., Beckervordersandforth, R., Petrone, C., Schroeder, T., et al. (2011). Continuous live imaging of adult neural stem cell division and lineage progression in vitro. Development 138, 1057–1068. doi: 10.1242/dev.061663
Costa, M. R., Wen, G., Lepier, A., Schroeder, T., and Gotz, M. (2008). Par-complex proteins promote proliferative progenitor divisions in the developing mouse cerebral cortex. Development 135, 11–22. doi: 10.1242/dev.009951
Couillard-Despres, S., and Aigner, L. (2011). In vivo imaging of adult neurogenesis. Eur. J. Neurosci. 33, 1037–1044. doi: 10.1111/j.1460-9568.2011.07601.x
Crabbe, A., Vandeputte, C., Dresselaers, T., Sacido, A. A., Verdugo, J. M., Eyckmans, J., et al. (2010). Effects of MRI contrast agents on the stem cell phenotype. Cell Transplant. 19, 919–936. doi: 10.3727/096368910X494623
Daynac, M., Morizur, L., Kortulewski, T., Gauthier, L. R., Ruat, M., Mouthon, M. A., et al. (2015). Cell sorting of neural stem and progenitor cells from the adult mouse subventricular zone and live-imaging of their cell cycle dynamics. J. Vis. Exp. 103:e53247. doi: 10.3791/53247
Doetsch, F., Caille, I., Lim, D. A., Garcia-Verdugo, J. M., and Alvarez-Buylla, A. (1999a). Subventricular zone astrocytes are neural stem cells in the adult mammalian brain. Cell 97, 703–716. doi: 10.1016/S0092-8674(00)80783-7
Doetsch, F., Garcia-Verdugo, J. M., and Alvarez-Buylla, A. (1997). Cellular composition and three-dimensional organization of the subventricular germinal zone in the adult mammalian brain. J. Neurosci. 17, 5046–5061.
Doetsch, F., Garcia-Verdugo, J. M., and Alvarez-Buylla, A. (1999b). Regeneration of a germinal layer in the adult mammalian brain. Proc. Natl. Acad. Sci. U.S.A. 96, 11619–11624. doi: 10.1073/pnas.96.20.11619
Doetsch, F., Petreanu, L., Caille, I., Garcia-Verdugo, J. M., and Alvarez-Buylla, A. (2002). EGF converts transit-amplifying neurogenic precursors in the adult brain into multipotent stem cells. Neuron 36, 1021–1034. doi: 10.1016/S0896-6273(02)01133-9
Dombeck, D. A., Harvey, C. D., Tian, L., Looger, L. L., and Tank, D. W. (2010). Functional imaging of hippocampal place cells at cellular resolution during virtual navigation. Nat. Neurosci. 13, 1433–1440. doi: 10.1038/nn.2648
Eilken, H., Rieger, M., Hoppe, P. S., Hermann, A., Smejkal, B., Drew, E., Thum, M., Ninkovic, J., et al. (2014). Continuous long-term detection of live cell surface markers by ‘in culture’ antibody staining. Protoc. Exch. doi: 10.1038/protex.2011.205. [Epub ahead of print].
Eilken, H. M., Nishikawa, S., and Schroeder, T. (2009). Continuous single-cell imaging of blood generation from haemogenic endothelium. Nature 457, 896–900. doi: 10.1038/nature07760
Encinas, J. M., Michurina, T. V., Peunova, N., Park, J. H., Tordo, J., Peterson, D. A., et al. (2011). Division-coupled astrocytic differentiation and age-related depletion of neural stem cells in the adult hippocampus. Cell Stem Cell 8, 566–579. doi: 10.1016/j.stem.2011.03.010
Filippov, V., Kronenberg, G., Pivneva, T., Reuter, K., Steiner, B., Wang, L. P., et al. (2003). Subpopulation of nestin-expressing progenitor cells in the adult murine hippocampus shows electrophysiological and morphological characteristics of astrocytes. Mol. Cell. Neurosci. 23, 373–382. doi: 10.1016/S1044-7431(03)00060-5
Fiorelli, R., Azim, K., Fischer, B., and Raineteau, O. (2015). Adding a spatial dimension to postnatal ventricular-subventricular zone neurogenesis. Development 142, 2109–2120. doi: 10.1242/dev.119966
Fuentealba, L. C., Rompani, S. B., Parraguez, J. I., Obernier, K., Romero, R., Cepko, C. L., et al. (2015). Embryonic origin of postnatal neural stem cells. Cell 161, 1644–1655. doi: 10.1016/j.cell.2015.05.041
Fukuda, S., Kato, F., Tozuka, Y., Yamaguchi, M., Miyamoto, Y., and Hisatsune, T. (2003). Two distinct subpopulations of nestin-positive cells in adult mouse dentate gyrus. J. Neurosci. 23, 9357–9366.
Furutachi, S., Miya, H., Watanabe, T., Kawai, H., Yamasaki, N., Harada, Y., et al. (2015). Slowly dividing neural progenitors are an embryonic origin of adult neural stem cells. Nat. Neurosci. 18, 657–665. doi: 10.1038/nn.3989
Gage, F. H. (2000). Mammalian neural stem cells. Science 287, 1433–1438. doi: 10.1126/science.287.5457.1433
Garcia, A. D., Doan, N. B., Imura, T., Bush, T. G., and Sofroniew, M. V. (2004). GFAP-expressing progenitors are the principal source of constitutive neurogenesis in adult mouse forebrain. Nat. Neurosci. 7, 1233–1241. doi: 10.1038/nn1340
Garcia-Marques, J., and Lopez-Mascaraque, L. (2013). Clonal identity determines astrocyte cortical heterogeneity. Cereb. Cortex 23, 1463–1472. doi: 10.1093/cercor/bhs134
Giachino, C., and Taylor, V. (2009). Lineage analysis of quiescent regenerative stem cells in the adult brain by genetic labelling reveals spatially restricted neurogenic niches in the olfactory bulb. Eur. J. Neurosci. 30, 9–24. doi: 10.1111/j.1460-9568.2009.06798.x
Gomes, F. L., Zhang, G., Carbonell, F., Correa, J. A., Harris, W. A., Simons, B. D., et al. (2011). Reconstruction of rat retinal progenitor cell lineages in vitro reveals a surprising degree of stochasticity in cell fate decisions. Development 138, 227–235. doi: 10.1242/dev.059683
Gonzalez-Perez, O., and Alvarez-Buylla, A. (2011). Oligodendrogenesis in the subventricular zone and the role of epidermal growth factor. Brain Res. Rev. 67, 147–156. doi: 10.1016/j.brainresrev.2011.01.001
Gould, E., and Cameron, H. A. (1996). Regulation of neuronal birth, migration and death in the rat dentate gyrus. Dev. Neurosci. 18, 22–35. doi: 10.1159/000111392
Granot, D., Scheinost, D., Markakis, E. A., Papademetris, X., and Shapiro, E. M. (2011). Serial monitoring of endogenous neuroblast migration by cellular MRI. Neuroimage 57, 817–824. doi: 10.1016/j.neuroimage.2011.04.063
Gritti, A., Bonfanti, L., Doetsch, F., Caille, I., Alvarez-Buylla, A., Lim, D. A., et al. (2002). Multipotent neural stem cells reside into the rostral extension and olfactory bulb of adult rodents. J. Neurosci. 22, 437–445.
Grutzendler, J., Kasthuri, N., and Gan, W. B. (2002). Long-term dendritic spine stability in the adult cortex. Nature 420, 812–816. doi: 10.1038/nature01276
Hack, M. A., Saghatelyan, A., De Chevigny, A., Pfeifer, A., Ashery-Padan, R., Lledo, P. M., et al. (2005). Neuronal fate determinants of adult olfactory bulb neurogenesis. Nat. Neurosci. 8, 865–872. doi: 10.1038/nn1479
He, J., Zhang, G., Almeida, A. D., Cayouette, M., Simons, B. D., and Harris, W. A. (2012). How variable clones build an invariant retina. Neuron 75, 786–798. doi: 10.1016/j.neuron.2012.06.033
Hoehn, M., Kustermann, E., Blunk, J., Wiedermann, D., Trapp, T., Wecker, S., et al. (2002). Monitoring of implanted stem cell migration in vivo: a highly resolved in vivo magnetic resonance imaging investigation of experimental stroke in rat. Proc. Natl. Acad. Sci. U.S.A. 99, 16267–16272. doi: 10.1073/pnas.242435499
Ihrie, R. A., Shah, J. K., Harwell, C. C., Levine, J. H., Guinto, C. D., Lezameta, M., et al. (2011). Persistent sonic hedgehog signaling in adult brain determines neural stem cell positional identity. Neuron 71, 250–262. doi: 10.1016/j.neuron.2011.05.018
Iordanova, B., and Ahrens, E. T. (2012). In vivo magnetic resonance imaging of ferritin-based reporter visualizes native neuroblast migration. Neuroimage 59, 1004–1012. doi: 10.1016/j.neuroimage.2011.08.068
James, R., Kim, Y., Hockberger, P. E., and Szele, F. G. (2011). Subventricular zone cell migration: lessons from quantitative two-photon microscopy. Front. Neurosci. 5:30. doi: 10.3389/fnins.2011.00030
Kamada, M., Li, R. Y., Hashimoto, M., Kakuda, M., Okada, H., Koyanagi, Y., et al. (2004). Intrinsic and spontaneous neurogenesis in the postnatal slice culture of rat hippocampus. Eur. J. Neurosci. 20, 2499–2508. doi: 10.1111/j.1460-9568.2004.03721.x
Kempermann, G., Kuhn, H. G., and Gage, F. H. (1997). More hippocampal neurons in adult mice living in an enriched environment. Nature 386, 493–495. doi: 10.1038/386493a0
Kempermann, G., Song, H., and Gage, F. H. (2015). Neurogenesis in the adult hippocampus. Cold Spring Harb. Perspect. Med. 5:a018812. doi: 10.1101/cshperspect.a018812
Kiyokage, E., Pan, Y. Z., Shao, Z., Kobayashi, K., Szabo, G., Yanagawa, Y., et al. (2010). Molecular identity of periglomerular and short axon cells. J. Neurosci. 30, 1185–1196. doi: 10.1523/JNEUROSCI.3497-09.2010
Kokovay, E., Wang, Y., Kusek, G., Wurster, R., Lederman, P., Lowry, N., et al. (2012). VCAM1 is essential to maintain the structure of the SVZ niche and acts as an environmental sensor to regulate SVZ lineage progression. Cell Stem Cell 11, 220–230. doi: 10.1016/j.stem.2012.06.016
Kosaka, T., and Kosaka, K. (2008). Tyrosine hydroxylase-positive GABAergic juxtaglomerular neurons are the main source of the interglomerular connections in the mouse main olfactory bulb. Neurosci. Res. 60, 349–354. doi: 10.1016/j.neures.2007.11.012
Kovalchuk, Y., Homma, R., Liang, Y., Maslyukov, A., Hermes, M., Thestrup, T., et al. (2015). In vivo odourant response properties of migrating adult-born neurons in the mouse olfactory bulb. Nat. Commun. 6, 6349. doi: 10.1038/ncomms7349
Kretzschmar, K., and Watt, F. M. (2012). Lineage tracing. Cell 148, 33–45. doi: 10.1016/j.cell.2012.01.002
Kriegstein, A., and Alvarez-Buylla, A. (2009). The glial nature of embryonic and adult neural stem cells. Annu. Rev. Neurosci. 32, 149–184. doi: 10.1146/annurev.neuro.051508.135600
Kuhn, H. G., Winkler, J., Kempermann, G., Thal, L. J., and Gage, F. H. (1997). Epidermal growth factor and fibroblast growth factor-2 have different effects on neural progenitors in the adult rat brain. J. Neurosci. 17, 5820–5829.
Lacar, B., Herman, P., Platel, J. C., Kubera, C., Hyder, F., and Bordey, A. (2012). Neural progenitor cells regulate capillary blood flow in the postnatal subventricular zone. J. Neurosci. 32, 16435–16448. doi: 10.1523/JNEUROSCI.1457-12.2012
Lendvai, B., Stern, E. A., Chen, B., and Svoboda, K. (2000). Experience-dependent plasticity of dendritic spines in the developing rat barrel cortex in vivo. Nature 404, 876–881. doi: 10.1038/35009107
Lim, D. A., and Alvarez-Buylla, A. (1999). Interaction between astrocytes and adult subventricular zone precursors stimulates neurogenesis. Proc. Natl. Acad. Sci. U.S.A. 96, 7526–7531. doi: 10.1073/pnas.96.13.7526
Liu, Z., and Martin, L. J. (2003). Olfactory bulb core is a rich source of neural progenitor and stem cells in adult rodent and human. J. Comp. Neurol. 459, 368–391. doi: 10.1002/cne.10664
Livesey, F. J., and Cepko, C. L. (2001). Vertebrate neural cell-fate determination: lessons from the retina. Nat. Rev. Neurosci. 2, 109–118. doi: 10.1038/35053522
Lledo, P. M., Alonso, M., and Grubb, M. S. (2006). Adult neurogenesis and functional plasticity in neuronal circuits. Nat. Rev. Neurosci. 7, 179–193. doi: 10.1038/nrn1867
Lois, C., and Alvarez-Buylla, A. (1993). Proliferating subventricular zone cells in the adult mammalian forebrain can differentiate into neurons and glia. Proc. Natl. Acad. Sci. U.S.A. 90, 2074–2077. doi: 10.1073/pnas.90.5.2074
Lois, C., Garcia-Verdugo, J. M., and Alvarez-Buylla, A. (1996). Chain migration of neuronal precursors. Science 271, 978–981. doi: 10.1126/science.271.5251.978
Luo, Y., Coskun, V., Liang, A., Yu, J., Cheng, L., Ge, W., et al. (2015). Single-cell transcriptome analyses reveal signals to activate dormant neural stem cells. Cell 161, 1175–1186. doi: 10.1016/j.cell.2015.04.001
Manganas, L. N., Zhang, X., Li, Y., Hazel, R. D., Smith, S. D., Wagshul, M. E., et al. (2007). Magnetic resonance spectroscopy identifies neural progenitor cells in the live human brain. Science 318, 980–985. doi: 10.1126/science.1147851
Marinaro, C., Pannese, M., Weinandy, F., Sessa, A., Bergamaschi, A., Taketo, M. M., et al. (2012). Wnt signaling has opposing roles in the developing and the adult brain that are modulated by Hipk1. Cereb. Cortex 22, 2415–2427. doi: 10.1093/cercor/bhr320
Marmur, R., Mabie, P. C., Gokhan, S., Song, Q., Kessler, J. A., and Mehler, M. F. (1998). Isolation and developmental characterization of cerebral cortical multipotent progenitors. Dev. Biol. 204, 577–591. doi: 10.1006/dbio.1998.9099
Menn, B., Garcia-Verdugo, J. M., Yaschine, C., Gonzalez-Perez, O., Rowitch, D., and Alvarez-Buylla, A. (2006). Origin of oligodendrocytes in the subventricular zone of the adult brain. J. Neurosci. 26, 7907–7918. doi: 10.1523/JNEUROSCI.1299-06.2006
Merkle, F. T., Fuentealba, L. C., Sanders, T. A., Magno, L., Kessaris, N., and Alvarez-Buylla, A. (2014). Adult neural stem cells in distinct microdomains generate previously unknown interneuron types. Nat. Neurosci. 17, 207–214. doi: 10.1038/nn.3610
Merkle, F. T., Mirzadeh, Z., and Alvarez-Buylla, A. (2007). Mosaic organization of neural stem cells in the adult brain. Science 317, 381–384. doi: 10.1126/science.1144914
Merkle, F. T., Tramontin, A. D., Garcia-Verdugo, J. M., and Alvarez-Buylla, A. (2004). Radial glia give rise to adult neural stem cells in the subventricular zone. Proc. Natl. Acad. Sci. U.S.A. 101, 17528–17532. doi: 10.1073/pnas.0407893101
Ming, G. L., and Song, H. (2011). Adult neurogenesis in the mammalian brain: significant answers and significant questions. Neuron 70, 687–702. doi: 10.1016/j.neuron.2011.05.001
Mirzadeh, Z., Merkle, F. T., Soriano-Navarro, M., Garcia-Verdugo, J. M., and Alvarez-Buylla, A. (2008). Neural stem cells confer unique pinwheel architecture to the ventricular surface in neurogenic regions of the adult brain. Cell Stem Cell 3, 265–278. doi: 10.1016/j.stem.2008.07.004
Mizrahi, A., Lu, J., Irving, R., Feng, G., and Katz, L. C. (2006). In vivo imaging of juxtaglomerular neuron turnover in the mouse olfactory bulb. Proc. Natl. Acad. Sci. U.S.A. 103, 1912–1917. doi: 10.1073/pnas.0506297103
Mongiat, L. A., and Schinder, A. F. (2011). Adult neurogenesis and the plasticity of the dentate gyrus network. Eur. J. Neurosci. 33, 1055–1061. doi: 10.1111/j.1460-9568.2011.07603.x
Moreno-Estelles, M., Gonzalez-Gomez, P., Hortiguela, R., Diaz-Moreno, M., San Emeterio, J., Carvalho, A. L., et al. (2012). Symmetric expansion of neural stem cells from the adult olfactory bulb is driven by astrocytes via WNT7A. Stem Cells 30, 2796–2809. doi: 10.1002/stem.1243
Nam, S. C., Kim, Y., Dryanovski, D., Walker, A., Goings, G., Woolfrey, K., et al. (2007). Dynamic features of postnatal subventricular zone cell motility: a two-photon time-lapse study. J. Comp. Neurol. 505, 190–208. doi: 10.1002/cne.21473
Namba, T., Mochizuki, H., Onodera, M., Namiki, H., and Seki, T. (2007). Postnatal neurogenesis in hippocampal slice cultures: early in vitro labeling of neural precursor cells leads to efficient neuronal production. J. Neurosci. Res. 85, 1704–1712. doi: 10.1002/jnr.21295
Noctor, S. C., Martinez-Cerdeno, V., Ivic, L., and Kriegstein, A. R. (2004). Cortical neurons arise in symmetric and asymmetric division zones and migrate through specific phases. Nat. Neurosci. 7, 136–144. doi: 10.1038/nn1172
Ohira, K., Furuta, T., Hioki, H., Nakamura, K. C., Kuramoto, E., Tanaka, Y., et al. (2010). Ischemia-induced neurogenesis of neocortical layer 1 progenitor cells. Nat. Neurosci. 13, 173–179. doi: 10.1038/nn.2473
Ortega, F., Costa, M. R., Simon-Ebert, T., Schroeder, T., Gotz, M., and Berninger, B. (2011). Using an adherent cell culture of the mouse subependymal zone to study the behavior of adult neural stem cells on a single-cell level. Nat. Protoc. 6, 1847–1859. doi: 10.1038/nprot.2011.404
Ortega, F., Gascon, S., Masserdotti, G., Deshpande, A., Simon, C., Fischer, J., et al. (2013). Oligodendrogliogenic and neurogenic adult subependymal zone neural stem cells constitute distinct lineages and exhibit differential responsiveness to Wnt signalling. Nat. Cell Biol. 15, 602–613. doi: 10.1038/ncb2736
Pagano, S. F., Impagnatiello, F., Girelli, M., Cova, L., Grioni, E., Onofri, M., et al. (2000). Isolation and characterization of neural stem cells from the adult human olfactory bulb. Stem Cells 18, 295–300. doi: 10.1634/stemcells.18-4-295
Panizzo, R. A., Kyrtatos, P. G., Price, A. N., Gadian, D. G., Ferretti, P., and Lythgoe, M. F. (2009). In vivo magnetic resonance imaging of endogenous neuroblasts labelled with a ferumoxide-polycation complex. Neuroimage 44, 1239–1246. doi: 10.1016/j.neuroimage.2008.10.062
Pastrana, E., Cheng, L. C., and Doetsch, F. (2009). Simultaneous prospective purification of adult subventricular zone neural stem cells and their progeny. Proc. Natl. Acad. Sci. U.S.A. 106, 6387–6392. doi: 10.1073/pnas.0810407106
Pastrana, E., Silva-Vargas, V., and Doetsch, F. (2011). Eyes wide open: a critical review of sphere-formation as an assay for stem cells. Cell Stem Cell 8, 486–498. doi: 10.1016/j.stem.2011.04.007
Picard-Riera, N., Decker, L., Delarasse, C., Goude, K., Nait-Oumesmar, B., Liblau, R., et al. (2002). Experimental autoimmune encephalomyelitis mobilizes neural progenitors from the subventricular zone to undergo oligodendrogenesis in adult mice. Proc. Natl. Acad. Sci. U.S.A. 99, 13211–13216. doi: 10.1073/pnas.192314199
Platel, J. C., Gordon, V., Heintz, T., and Bordey, A. (2009). GFAP-GFP neural progenitors are antigenically homogeneous and anchored in their enclosed mosaic niche. Glia 57, 66–78. doi: 10.1002/glia.20735
Ponti, G., Obernier, K., Guinto, C., Jose, L., Bonfanti, L., and Alvarez-Buylla, A. (2013). Cell cycle and lineage progression of neural progenitors in the ventricular-subventricular zones of adult mice. Proc. Natl. Acad. Sci. U.S.A. 110, E1045–E1054. doi: 10.1073/pnas.1219563110
Qian, X., Goderie, S. K., Shen, Q., Stern, J. H., and Temple, S. (1998). Intrinsic programs of patterned cell lineages in isolated vertebrate CNS ventricular zone cells. Development 125, 3143–3152.
Qian, X., Shen, Q., Goderie, S. K., He, W., Capela, A., Davis, A. A., et al. (2000). Timing of CNS cell generation: a programmed sequence of neuron and glial cell production from isolated murine cortical stem cells. Neuron 28, 69–80. doi: 10.1016/S0896-6273(00)00086-6
Raineteau, O., Rietschin, L., Gradwohl, G., Guillemot, F., and Gahwiler, B. H. (2004). Neurogenesis in hippocampal slice cultures. Mol. Cell. Neurosci. 26, 241–250. doi: 10.1016/j.mcn.2004.01.003
Ramm, P., Couillard-Despres, S., Plotz, S., Rivera, F. J., Krampert, M., Lehner, B., et al. (2009). A nuclear magnetic resonance biomarker for neural progenitor cells: is it all neurogenesis? Stem Cells 27, 420–423. doi: 10.1634/stemcells.2008-0816
Ravin, R., Hoeppner, D. J., Munno, D. M., Carmel, L., Sullivan, J., Levitt, D. L., et al. (2008). Potency and fate specification in CNS stem cell populations in vitro. Cell Stem Cell 3, 670–680. doi: 10.1016/j.stem.2008.09.012
Reynolds, B. A., and Rietze, R. L. (2005). Neural stem cells and neurospheres–re-evaluating the relationship. Nat. Methods 2, 333–336. doi: 10.1038/nmeth758
Reynolds, B. A., and Weiss, S. (1996). Clonal and population analyses demonstrate that an EGF-responsive mammalian embryonic CNS precursor is a stem cell. Dev. Biol. 175, 1–13. doi: 10.1006/dbio.1996.0090
Rieger, M. A., Hoppe, P. S., Smejkal, B. M., Eitelhuber, A. C., and Schroeder, T. (2009). Hematopoietic cytokines can instruct lineage choice. Science 325, 217–218. doi: 10.1126/science.1171461
Riesenberg, A. N., Liu, Z., Kopan, R., and Brown, N. L. (2009). Rbpj cell autonomous regulation of retinal ganglion cell and cone photoreceptor fates in the mouse retina. J. Neurosci. 29, 12865–12877. doi: 10.1523/JNEUROSCI.3382-09.2009
Robel, S., Berninger, B., and Gotz, M. (2011). The stem cell potential of glia: lessons from reactive gliosis. Nat. Rev. Neurosci. 12, 88–104. doi: 10.1038/nrn2978
Rueger, M. A., Backes, H., Walberer, M., Neumaier, B., Ullrich, R., Simard, M. L., et al. (2010). Noninvasive imaging of endogenous neural stem cell mobilization in vivo using positron emission tomography. J. Neurosci. 30, 6454–6460. doi: 10.1523/JNEUROSCI.6092-09.2010
Saha, B., Peron, S., Murray, K., Jaber, M., and Gaillard, A. (2013). Cortical lesion stimulates adult subventricular zone neural progenitor cell proliferation and migration to the site of injury. Stem Cell Res. 11, 965–977. doi: 10.1016/j.scr.2013.06.006
Sanai, N., Tramontin, A. D., Quinones-Hinojosa, A., Barbaro, N. M., Gupta, N., Kunwar, S., et al. (2004). Unique astrocyte ribbon in adult human brain contains neural stem cells but lacks chain migration. Nature 427, 740–744. doi: 10.1038/nature02301
Scheffler, B., Walton, N. M., Lin, D. D., Goetz, A. K., Enikolopov, G., Roper, S. N., et al. (2005). Phenotypic and functional characterization of adult brain neuropoiesis. Proc. Natl. Acad. Sci. U.S.A. 102, 9353–9358. doi: 10.1073/pnas.0503965102
Schroeder, T. (2011). Long-term single-cell imaging of mammalian stem cells. Nat. Methods 8, S30–S35. doi: 10.1038/nmeth.1577
Seaberg, R. M., Smukler, S. R., and Van Der Kooy, D. (2005). Intrinsic differences distinguish transiently neurogenic progenitors from neural stem cells in the early postnatal brain. Dev. Biol. 278, 71–85. doi: 10.1016/j.ydbio.2004.10.017
Sequerra, E. B., Costa, M. R., Menezes, J. R., and Hedin-Pereira, C. (2013). Adult neural stem cells: plastic or restricted neuronal fates? Development 140, 3303–3309. doi: 10.1242/dev.093096
Sequerra, E. B., Miyakoshi, L. M., Froes, M. M., Menezes, J. R., and Hedin-Pereira, C. (2010). Generation of glutamatergic neurons from postnatal and adult subventricular zone with pyramidal-like morphology. Cereb. Cortex 20, 2583–2591. doi: 10.1093/cercor/bhq006
Seri, B., Garcia-Verdugo, J. M., Mcewen, B. S., and Alvarez-Buylla, A. (2001). Astrocytes give rise to new neurons in the adult mammalian hippocampus. J. Neurosci. 21, 7153–7160.
Shen, Q., Wang, Y., Kokovay, E., Lin, G., Chuang, S. M., Goderie, S. K., et al. (2008). Adult SVZ stem cells lie in a vascular niche: a quantitative analysis of niche cell-cell interactions. Cell Stem Cell 3, 289–300. doi: 10.1016/j.stem.2008.07.026
Shors, T. J., Townsend, D. A., Zhao, M., Kozorovitskiy, Y., and Gould, E. (2002). Neurogenesis may relate to some but not all types of hippocampal-dependent learning. Hippocampus 12, 578–584. doi: 10.1002/hipo.10103
Singec, I., Knoth, R., Meyer, R. P., Maciaczyk, J., Volk, B., Nikkhah, G., et al. (2006). Defining the actual sensitivity and specificity of the neurosphere assay in stem cell biology. Nat. Methods 3, 801–806. doi: 10.1038/nmeth926
Sirko, S., Behrendt, G., Johansson, P. A., Tripathi, P., Costa, M., Bek, S., et al. (2013). Reactive glia in the injured brain acquire stem cell properties in response to sonic hedgehog. [corrected]. Cell Stem Cell 12, 426–439. doi: 10.1016/j.stem.2013.01.019
Sobottka, B., Ziegler, U., Kaech, A., Becher, B., and Goebels, N. (2011). CNS live imaging reveals a new mechanism of myelination: the liquid croissant model. Glia 59, 1841–1849. doi: 10.1002/glia.21228
Sohn, J., Orosco, L., Guo, F., Chung, S. H., Bannerman, P., Mills Ko, E., et al. (2015). The subventricular zone continues to generate corpus callosum and rostral migratory stream astroglia in normal adult mice. J. Neurosci. 35, 3756–3763. doi: 10.1523/JNEUROSCI.3454-14.2015
Suh, H., Deng, W., and Gage, F. H. (2009). Signaling in adult neurogenesis. Annu. Rev. Cell Dev. Biol. 25, 253–275. doi: 10.1146/annurev.cellbio.042308.113256
Sulston, J. E., Schierenberg, E., White, J. G., and Thomson, J. N. (1983). The embryonic cell lineage of the nematode Caenorhabditis elegans. Dev. Biol. 100, 64–119. doi: 10.1016/0012-1606(83)90201-4
Tavazoie, M., Van Der Veken, L., Silva-Vargas, V., Louissaint, M., Colonna, L., Zaidi, B., et al. (2008). A specialized vascular niche for adult neural stem cells. Cell Stem Cell 3, 279–288. doi: 10.1016/j.stem.2008.07.025
Tong, C. K., Chen, J., Cebrian-Silla, A., Mirzadeh, Z., Obernier, K., Guinto, C. D., et al. (2014). Axonal control of the adult neural stem cell niche. Cell Stem Cell 14, 500–511. doi: 10.1016/j.stem.2014.01.014
Tong, C. K., Fuentealba, L. C., Shah, J. K., Lindquist, R. A., Ihrie, R. A., Guinto, C. D., et al. (2015). A dorsal SHH-dependent domain in the V-SVZ produces large numbers of oligodendroglial lineage cells in the postnatal brain. Stem Cell Rep. 5, 461–470. doi: 10.1016/j.stemcr.2015.08.013
Trachtenberg, J. T., Chen, B. E., Knott, G. W., Feng, G., Sanes, J. R., Welker, E., et al. (2002). Long-term in vivo imaging of experience-dependent synaptic plasticity in adult cortex. Nature 420, 788–794. doi: 10.1038/nature01273
Vergano-Vera, E., Mendez-Gomez, H. R., Hurtado-Chong, A., Cigudosa, J. C., and Vicario-Abejon, C. (2009). Fibroblast growth factor-2 increases the expression of neurogenic genes and promotes the migration and differentiation of neurons derived from transplanted neural stem/progenitor cells. Neuroscience 162, 39–54. doi: 10.1016/j.neuroscience.2009.03.033
Vreys, R., Vande Velde, G., Krylychkina, O., Vellema, M., Verhoye, M., Timmermans, J. P., et al. (2010). MRI visualization of endogenous neural progenitor cell migration along the RMS in the adult mouse brain: validation of various MPIO labeling strategies. Neuroimage 49, 2094–2103. doi: 10.1016/j.neuroimage.2009.10.034
Walton, N. M., Snyder, G. E., Park, D., Kobeissy, F., Scheffler, B., and Steindler, D. A. (2009). Gliotypic neural stem cells transiently adopt tumorigenic properties during normal differentiation. Stem Cells 27, 280–289. doi: 10.1634/stemcells.2008-0842
Weber, T., Vroemen, M., Behr, V., Neuberger, T., Jakob, P., Haase, A., et al. (2006). In vivo high-resolution MR imaging of neuropathologic changes in the injured rat spinal cord. AJNR Am. J. Neuroradiol. 27, 598–604.
Winter, M., Wait, E., Roysam, B., Goderie, S. K., Ali, R. A., Kokovay, E., et al. (2011). Vertebrate neural stem cell segmentation, tracking and lineaging with validation and editing. Nat. Protoc. 6, 1942–1952. doi: 10.1038/nprot.2011.422
Winter, M. R., Liu, M., Monteleone, D., Melunis, J., Hershberg, U., Goderie, S. K., et al. (2015). Computational image analysis reveals intrinsic multigenerational differences between anterior and posterior cerebral cortex neural progenitor cells. Stem Cell Rep. 5, 609–620. doi: 10.1016/j.stemcr.2015.08.002
Yokose, J., Ishizuka, T., Yoshida, T., Aoki, J., Koyanagi, Y., and Yawo, H. (2011). Lineage analysis of newly generated neurons in organotypic culture of rat hippocampus. Neurosci. Res. 69, 223–233. doi: 10.1016/j.neures.2010.11.010
Keywords: live imaging, aNSCs, neurogenic niches, timelapse videomicroscopy, lineage tracing
Citation: Ortega F and Costa MR (2016) Live Imaging of Adult Neural Stem Cells in Rodents. Front. Neurosci. 10:78. doi: 10.3389/fnins.2016.00078
Received: 14 December 2015; Accepted: 18 February 2016;
Published: 07 March 2016.
Edited by:
Laura López-Mascaraque, Instituto Cajal- Consejo Superior de Investigaciones Científicas, SpainReviewed by:
Olivier Raineteau, Institut National de la Santé et de la Recherche Médicale - Université de Lyon, FranceCarlos Vicario-Abejón, Instituto Cajal, Consejo Superior de Investigaciones Científicas, Spain
Copyright © 2016 Ortega and Costa. This is an open-access article distributed under the terms of the Creative Commons Attribution License (CC BY). The use, distribution or reproduction in other forums is permitted, provided the original author(s) or licensor are credited and that the original publication in this journal is cited, in accordance with accepted academic practice. No use, distribution or reproduction is permitted which does not comply with these terms.
*Correspondence: Felipe Ortega, Zm9ydGVnYW9AdWNtLmVz