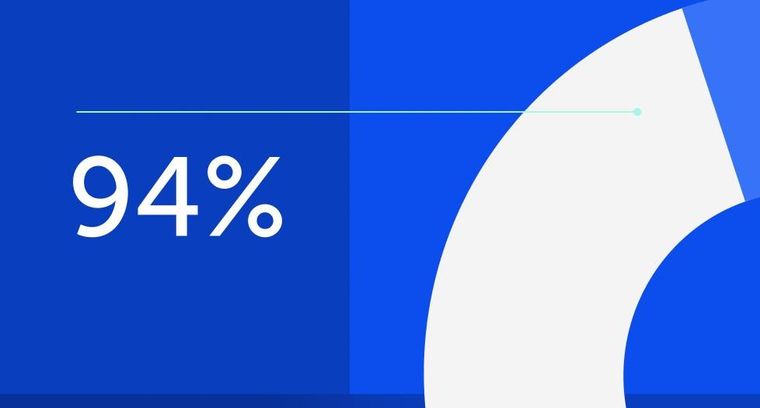
94% of researchers rate our articles as excellent or good
Learn more about the work of our research integrity team to safeguard the quality of each article we publish.
Find out more
REVIEW article
Front. Neurosci., 23 February 2016
Sec. Neurogenesis
Volume 10 - 2016 | https://doi.org/10.3389/fnins.2016.00052
This article is part of the Research Topic50th Anniversary of Adult Neurogenesis: Olfaction, hippocampus and beyondView all 23 articles
The generation of neurons in the adult mammalian brain requires the activation of quiescent neural stem cells (NSCs). This activation and the sequential steps of neuron formation from NSCs are regulated by a number of stimuli, which include growth factors. Insulin-like growth factor-I (IGF-I) exert pleiotropic effects, regulating multiple cellular processes depending on their concentration, cell type, and the developmental stage of the animal. Although IGF-I expression is relatively high in the embryonic brain its levels drop sharply in the adult brain except in neurogenic regions, i.e., the hippocampus (HP) and the subventricular zone-olfactory bulb (SVZ-OB). By contrast, the expression of IGF-IR remains relatively high in the brain irrespective of the age of the animal. Evidence indicates that IGF-I influences NSC proliferation and differentiation into neurons and glia as well as neuronal maturation including synapse formation. Furthermore, recent studies have shown that IGF-I not only promote adult neurogenesis by regulating NSC number and differentiation but also by influencing neuronal positioning and migration as described during SVZ-OB neurogenesis. In this article we will revise and discuss the actions reported for IGF-I signaling in a variety of in vitro and in vivo models, focusing on the maintenance and proliferation of NSCs/progenitors, neurogenesis, and neuron integration in synaptic circuits.
IGF-I belongs to the insulin family which is divided in two groups of peptides: one includes insulin and the IGFs and the other relaxin and insulin-like hormones. In the insulin group, each peptide binds to a specific receptor with high affinity, although it can also bind to the other receptor with low affinity (Table 1). Furthermore, the insulin receptor and the IGF-I receptor (IGF-IR) can form heterodimers with similar affinity for both growth factors (Hernández-Sánchez et al., 2008). The IGF-IR has the higher affinity for IGF-I but its affinity is 10 times lower for IGF-II and 250 times lower for insulin (Versteyhe et al., 2013). In addition, there are at least seven IGF-binding proteins (IGFBPs) that increase the half-life of the peptide by preventing its proteolysis and modulating the interaction with the receptor (Table 1; Ocrant et al., 1990; Hwa et al., 1999; Bondy and Cheng, 2004; Agis-Balboa et al., 2011; Fernandez and Torres-Alemán, 2012; Agis-Balboa and Fischer, 2014).
The mature IGF-I is a single polypeptide chain of 70 amino acids (7.5 kDa) with 57 amino acids being identical in mammals, birds, and amphibians (Liu et al., 1993; Russo et al., 2005; Annunziata et al., 2011). The IGF-IR is a tyrosine kinase receptor characterized by tetramers, which are composed of two α subunits and two β subunits (Russo et al., 2005; Annunziata et al., 2011; Vogel, 2013).
IGF-I is abundantly produced during embryonic development in many tissues, but its expression is markedly reduced during postnatal life. In the adult individual, IGF-I is mainly synthesized in the liver via a process regulated by the growth hormone (GH). Furthermore, there is a small local production in tissues including brain regions such as the SVZ, the OB, the HP, and the cerebellum (CB; Rotwein et al., 1988; Ye et al., 1997). In the brain, IGF-I can be synthesized by neurons independently of GH action (Bartlett et al., 1991, 1992; Bondy and Cheng, 2004; Russo et al., 2005; Fernandez and Torres-Alemán, 2012). Systemic IGF-I can pass from the blood to the cerebrospinal fluid through the lipoprotein receptor-related protein 2 (LRP2). In addition, IGF-I can cross the blood-brain-barrier by binding to the IGF-IR present on endothelial cells and later it is picked up either by astrocytes to be transferred to neurons or directly by neurons (Nishijima et al., 2010; Fernandez and Torres-Alemán, 2012). Therefore, IGF-I can act in the brain in an endocrine, paracrine or autocrine manner.
IGF-IR expression begins early during embryonic development in regions that include the cortex, OB, HP, CB, hypothalamus (HT), and spinal cord (Bondy et al., 1990). Postnatally, IGF-IR levels are slightly reduced and in the adult, its expression is clearly detected in the SVZ, OB, HP, CB, and the choroid plexus (Bondy and Cheng, 2004; Russo et al., 2005; Fernandez and Torres-Alemán, 2012).
The specific IGF-I binding to IGF-IR triggers the auto phosphorylation of the receptor and the activation of the insulin receptor substrates (IRS). These activated IRSs are auto-phosphorylated and in turn activate the intracellular signaling pathways including PI3K and MAP kinase pathways (Liu et al., 1993; Bondy and Cheng, 2004; Bateman and McNeill, 2006; Fernandez and Torres-Alemán, 2012; Puche and Castilla-Cortázar, 2012).
The phosphatidylinositol 3-kinase (PI3K) phophorylates the serine/threonine protein kinase (Akt) through the phosphoinositide-dependent protein kinase (PDK). Phospho-Akt promotes the translocation of the glucose transporters to the plasma membrane affecting cell metabolism (Bondy and Cheng, 2004; Fernandez and Torres-Alemán, 2012). Another Akt substrate is the mammalian target of rapamycin (mTOR). mTOR1 activates p70S6K, regulating protein synthesis while mTOR2 activates a series of kinases (including Akt), affecting proliferation, cell migration, and positioning (Hurtado-Chong et al., 2009; Iwanami et al., 2009; Onuma et al., 2011; Fernandez and Torres-Alemán, 2012; Paliouras et al., 2012; Pun et al., 2012). Akt also promotes the activation of fork head transcription factor (FOXO), which regulates cell proliferation, oxidative stress and apoptosis (Bateman and McNeill, 2006; Fernandez and Torres-Alemán, 2012; O'Kusky and Ye, 2012). Moreover, Akt can activate the cAMP responsive element binding protein (CREB) regulating the transcription of genes involved in cell cycle progression, survival, and differentiation. The binding of IGF-I to the IGF-IR can also promote the activation of Son of sevenless (SOS) triggering the phosphorylation of RAS, which in turn promotes the activation of MAPK. Later, MAPK produces the phosphorylation of ERK inducing proliferation of multiple cell types (Baltensperger et al., 1993; Skolnik et al., 1993; Bateman and McNeill, 2006; Cundiff et al., 2009; Fernandez and Torres-Alemán, 2012).
IGF-I is a pleiotropic factor involved in multiple processes, so its actions are different depending on its concentration, the cell type, and the developmental stage of the animal.
IGF-I is necessary very early during pregnancy because it promotes embryo implantation in the uterus (O'Kusky and Ye, 2012). Later, IGF-I is important for the proper prenatal growth and postnatal survival of the animal. This fact is reflected in the smaller size of the global Igf-I Knockout (KO) mice and Igf-Ir KO mice compared to their control littermates after birth. The liver-specific Igf-I-deficient (LID) mice have a similar body size compared to the control animals, suggesting that IGF-I affects tissue growth in an autocrine or paracrine manner (Yakar et al., 2002). Interestingly, exogenously administrated IGF-I can compensate for most autocrine/paracrine actions of this growth factor (Wu et al., 2009). The large majority of global Igf-I KO mice die soon after birth due to insufficient lung maturation, although the death rate depends on the mouse strains (Liu et al., 1993; Moreno-Barriuso et al., 2006; Kappeler et al., 2008; Hurtado-Chong et al., 2009; Pais et al., 2013). The muscles, brain, bones and skin are affected by the lack of IGF-I, as reflected by the muscle hypoplasia and the reduced brain size, ossification, and skin thickness found in the KO mice (Baker et al., 1993; Liu et al., 1993; Powell-Braxton et al., 1993; Beck et al., 1995; Pichel et al., 2003). This phenotype is also observed in the few surviving postnatal KO mice which show a reduction in body and brain size, lower development of ossification centers, infertility, and deafness (Baker et al., 1993; Wang et al., 1999; Yakar et al., 2002; Fernández-Moreno et al., 2004; Cediel et al., 2006; Stratikopoulos et al., 2008; Hurtado-Chong et al., 2009; Wu et al., 2009; O'Kusky and Ye, 2012; Rodríguez-de la Rosa et al., 2015).
In humans, mutations in the IGF-I and IGF-IR genes cause growth retardation including microcephaly (Roback et al., 1991; Woods et al., 1996; Walenkamp et al., 2005, 2013; van Duyvenvoorde et al., 2010; Burkhardt et al., 2015). Furthermore, the congenital deficiency of IGF-I or IGF-IR are features of the Laron syndrome, which also includes growth hormone receptor (GHR) deficiency and/or the alteration of molecules of the GH and IGF-I signaling pathways. Patients with this syndrome experience less growth after birth and this becomes more severe with age, leading to smaller brain size, smaller heart, less muscle development, among other deficits (Puche and Castilla-Cortázar, 2012). Although short stature is a common feature of the individuals bearing IGF-I and/or IGF-IR mutations, a recent study has described intragenic deletions of the IGF-IR associated to a developmental delay and intellectual disability of five people that do not have a significant short stature (Witsch et al., 2013).
During central nervous system (CNS) development and adult neurogenesis, the IGF-I/IGF-IR system regulates the proliferation and survival of neural progenitors, as well as the generation, differentiation, and maturation of neurons in multiple ways (Beck et al., 1995; Cheng et al., 2001; Pichel et al., 2003; Russo et al., 2005; Hurtado-Chong et al., 2009; Fernandez and Torres-Alemán, 2012; O'Kusky and Ye, 2012; Chaker et al., 2015). These aspects are discussed in depth below (Figure 1).
Figure 1. A schematic summary of the role of IGF-I during postnatal-adult neurogenesis. The IGF-I is a pleiotropic factor that affect a variety of cellular processes. The administration of IGF-I enhances cell proliferation and neurogenesis in the OB, DG, and HT in vitro and in vivo. However, the use of transgenic mice that overexpress IGF-I or lack the Igf-I and Igf-IR genes has revealed contradictory effects of IGF-I signaling on these processes. IGF-I promotes cell migration in the SVZ-OB and the survival and axonal growth of neurons in both the SVZ-OB and DG. In contrast, the effects on dendritic spines and synapse formation may depend on the neuron type and developmental stage of the cell and animal. DG, dentate gyrus; Diff., differentiation; OB, olfactory bulb; RMS, rostral migratory stream; SVZ, subventricular zone; HT, hypothalamus; 3V, third ventricle.
IGF-I promotes proliferation of neural cells by interacting with the IGF-IR which may activate the PI3K/Akt or the MAP kinase pathways (Otaegi et al., 2006; Mairet-Coello et al., 2009; Vogel, 2013; Yuan et al., 2015). During embryonic development, IGF-I promotes the proliferation of neuroepithelial progenitor cells both in vitro (Hernández-Sánchez et al., 1995; Arsenijevic et al., 2001; Vicario-Abejón et al., 2003; Cui and Almazan, 2007; Magariños et al., 2010; Ziegler et al., 2012) and in vivo (Popken et al., 2004; Ye and D'Ercole, 2006; Hu et al., 2012). This positive effect of IGF-I on cell proliferation was also observed postnatally and in the adult brain (Aberg et al., 2000, 2003; Trejo et al., 2001; Gago et al., 2003; Popken et al., 2004; Kalluri et al., 2007; Kouroupi et al., 2010; Pérez-Martín et al., 2010; Yuan et al., 2015), although enhanced proliferation was not found in the adult HP of an astrocyte-conditional IGF-I overexpressing mouse (Carlson et al., 2014). In contrast, mice that overexpress IGF-I under the regulation of the Nestin promoter, active in neural progenitors, show an increase in brain size both at E18 and postnatally (Popken et al., 2004) due to a shorter cell cycle produce by the decrease in the G1 phase length (Hodge et al., 2004).
During OB development, IGF-I can stimulate the proliferation of stem and progenitor cells as observed in embryonic olfactory bulb stem cells (eOBSCs) cultures where the addition of IGF-I increases the number of proliferative cells and of neurospheres compared to untreated cultures. However, when eOBSCs were isolated from Igf-I KO embryos, there was no difference in the percentage of BrdU+ cells compared to wildtype (WT) cells (Vicario-Abejón et al., 2003). In contrast, a decrease in the number of cells in the M phase of cell cycle was observed in the SVZ of Igf-I adult KO mice (Hurtado-Chong et al., 2009; Figure 1).
IGF-I also affects cell proliferation in the dentate gyrus (DG) of the HP (Figure 1). In fact, in cultures of adult rat DG progenitor cells an increase in the number of dividing cells was found after IGF-I treatment (Aberg et al., 2003). In addition, when mice were administered with IGF-I peripherally, more BrdU+ cells in the DG were detected (Aberg et al., 2000). A similar effect was observed after physical exercise, a condition that enhances IGF-I entry into the brain (Trejo et al., 2001, 2008; Glasper et al., 2010; Fernandez and Torres-Alemán, 2012). However, in the Igf-I KO mice the lack of IGF-I produced an increase in the number of proliferative cells in DG (Cheng et al., 2001) whereas both GH/IGF-I deficiency and deleting the IGF-IR in neural progenitors did not specifically affect proliferation in the postnatal-adult DG (Lichtenwalner et al., 2006; Liu et al., 2009).
In sum, the majority of these findings have shown that an increase in the IGF-I levels promotes cell proliferation both in vitro and in vivo. However, deleting this growth factor and/or its receptor in KO mouse has produced contrasting effects that are not completely elucidated yet (Figure 1). The expression of insulin, IGF-II and of truncated IGF-I-related peptides in the KO mice might partially explain the discrepancy obtained in different studies. Although the majority of truncated peptides are thought to be non-functional, we cannot completely exclude that in certain KO mice lines they could affect the results described. The development of new technologies such as the CRISPR-Cas9 system, which allows the complete deletion of specific genes, and the generation of double or triple KO mouse lines could help to understand the effect of the deletion of IGF-I or its receptor in cell proliferation during adult neurogenesis.
Evidence indicates that IGF-I promotes cell survival by inhibiting apoptosis both in vivo and in vitro. These effects have been observed in neural progenitors and in multiple neuronal types such as cortical cells, motoneurons, Purkinje cells, or optic neural progenitor cells (Gago et al., 2003; Vicario-Abejón et al., 2004; Hodge et al., 2007; Croci et al., 2010; Aburto et al., 2012; Lunn et al., 2015). In the DG, the lack of IGF-I or IGF-IR causes a decrease in neuronal survival under basal conditions (Cheng et al., 2001; Lichtenwalner et al., 2006; Liu et al., 2009) or after ischemia (Liu et al., 2011) whereas IGF-I overexpression rescued neuronal survival in the lesioned HP (Carlson et al., 2014; Figure 1).
Moreover, IGF-I could prevent neuronal death in neurodegenerative diseases such as Alzheimer, regulating the accumulation of amyloid-β, and Tau proteins (Carro et al., 2002; Puche and Castilla-Cortázar, 2012). In fact, IGF-I enhances the transport of amyloid-β carrier proteins such as albumin and transthyretin, promoting its degradation (Carro et al., 2002). Moreover, this factor activates Akt which inhibits GSK3β, preventing Tau hyperphosporilation (Bondy and Cheng, 2004). In addition to the accumulation of amyloid-β and phosphorylated Tau proteins, the cognitive decline found in Alzheimer's patients might be attributable to decreased dentate gyrus neurogenesis. In contrast, an increase in IGF-I levels enhances neurogenesis (see below) and ameliorates the age-related cognitive malfunction in the brain. Therefore, restoring hippocampal neurogenesis by IGF-I may be a strategy for reversing age-related cerebral dysfunction. However, other studies have reported that IGF-I can promote the production of amyloid-β (Araki et al., 2009) and that knocking-out IGF-IR in neurons of a mouse model of Alzheimer's disease (AD) favors amyloid-β clearance probably by preserving autophagy and improves spatial memory (Gontier et al., 2015). This potential neuroprotective effect of reducing IGF-I/IGF-IR signaling has also been proposed for spinal muscular atrophy (SMA; Biondi et al., 2015). Therefore, the role of IGF-I in AD and motor neuron disease requires further investigation.
IGF-I can also prevent the gradual loss of other physiological functions associated with aging produced by oxidative stress and DNA damage, among others (Puche and Castilla-Cortázar, 2012). However, some Igf-I deficient mice, which have low levels of circulating IGF-I, exhibit an increased lifespan possibly due to alterations in energy metabolism and a transient enhancement in neurogenesis (Sun et al., 2005; Sun, 2006; Junnila et al., 2013; Chaker et al., 2015). These mice show an upregulation of local IGF-I levels in the hippocampus which could explain the increase in neurogenesis (Sun et al., 2005). All these data reveal that the effect of circulating and local IGF-I may be different but the full mechanisms have not been elucidated.
IGF-I is also involved in the regulation of the migration of certain cell types. In neuroblastoma cell line cultures, IGF-I stimulates cell migration (Puglianiello et al., 2000; Russo et al., 2005). The first demonstration that IGF-I regulates cell migration and positioning in vivo was described by Hurtado-Chong et al. through Igf-I KO mice and explant cultures (Figure 1). These studies showed that IGF-I is necessary for the exit of neuroblasts from the SVZ to the OB and for the radial neuronal migration in the OB (Hurtado-Chong et al., 2009). These effects were mediated by the activation of the PI3K pathway and by phosphorylation of the reelin signal transducer, homolog 1 (Dab1; Hurtado-Chong et al., 2009). These findings indicate that IGF-I promotes adult neurogenesis not only by regulating NSC number and differentiation but also by directing neuronal positioning and migration (Figure 1). Successively, IGF-I has been related to the migration of doublecortin+ immature neurons in the SVZ-RMS, dorsal root ganglion neurons and cerebellar neurons in rodents, and neural crest cells in the zebrafish (Onuma et al., 2011; Xiang et al., 2011; Li et al., 2012; Maucksch et al., 2013).
The IGF-I/IGF-IR system regulates the differentiation and maturation of neurons generated from NSCs and progenitors both during embryonic development and in the adult brain largely via the PI3K/Akt pathway (Aberg et al., 2000; Brooker et al., 2000; O'Kusky et al., 2000; Trejo et al., 2001; Vicario-Abejón et al., 2003; Otaegi et al., 2006; Carlson et al., 2014; Zhang et al., 2014; Yuan et al., 2015). Furthermore, IGF-I also influences the development of astrocytes and oligodendrocytes (Ye and D'Ercole, 2006; O'Kusky and Ye, 2012). Indeed, IGF-I promotes the differentiation of neural progenitors into mature oligodendrocytes that produce myelin (Carson et al., 1993; Ye et al., 1995; Gago et al., 2003; Hsieh et al., 2004) and stimulates the proliferation and differentiation of astrocytes under physiological conditions and after injury (Cao et al., 2003; Ye et al., 2004).
In Igf-I and Igf-IR KO mice, a reduction in the number of neurons during embryonic development and postnatal-adult neurogenesis in SVZ-OB and HP has been described (Baker et al., 1993; Liu et al., 1993, 2009; Powell-Braxton et al., 1993; Beck et al., 1995; Hurtado-Chong et al., 2009). When IGF-I was added to eOBSCs in culture, it produced an increase in the number of neurons, astrocytes and oligodendrocytes, whereas there was a decrease in the differentiation of eOBSCs isolated from the Igf-I KO mice (Vicario-Abejón et al., 2003; Otaegi et al., 2006). In the postnatal-adult OB of Igf-I KO animals, reductions in the number of interneuron populations were observed, possibly due to the altered neuroblast exit and migration from the SVZ, as mentioned above (Hurtado-Chong et al., 2009). By contrast, animals that overexpress Igf-I exhibit an increase in the number of neurons in the HP (O'Kusky et al., 2000; Popken et al., 2004; Carlson et al., 2014; Figure 1).
In addition to its role in the main neurogenic adult brain regions IGF-I also increases neurogenesis in the hypothalamus. After intra-cerebroventricular treatment with IGF-I, the number of neurons and astrocytes labeled with BrdU was significantly increased in the whole hypothalamus (Pérez-Martín et al., 2010). A similar effect of this growth factor was also found in hypothalamic cell cultures and explants (Torres-Aleman et al., 1990; Pérez-Martín et al., 2010).
IGF-I may regulate neuronal maturation, affecting axonal and dendritic growth, and establishing synapses in different brain areas independently of cell survival (O'Kusky et al., 2000; Cao et al., 2011; Figure 1). Thus, Igf-I KO animals have a lower development in the peripheral nerves (Gao et al., 1999), an altered innervation of the sensory cells of the organ of Corti (Camarero et al., 2001) and a lower density of spines in neurons of layers II-III of the cortex (Cheng et al., 2003). In the OB of Igf-I KO mice, the pattern of the axonal projections of sensory olfactory neurons is altered, because IGF-I acts as a chemoattractant for axonal growth cones (Scolnick et al., 2008). In the HP, IGF-I is involved in the establishment of neuronal polarity and the initial growth of the axonal cone, through the Akt pathway (Laurino et al., 2005; Sosa et al., 2006). Although the structure of the CB is preserved in E18.5 Igf-I KO mice (Vicario-Abejón et al., 2004), it has been shown that IGF-I promotes the establishment of cerebellar synapses whereas lack of IGF-I facilitates its removal during postnatal development (Kakizawa et al., 2003). Likewise, IGF-I overexpression in transgenic mouse promotes dendrite growth and synaptogenesis in the DG (O'Kusky et al., 2000; Carlson et al., 2014).
Exercise produces an increase in the IGF-I levels in adults which then stimulates an increase in the density of spines in the basal dendrites of CA1 pyramidal neurons but does not affect either the granule neurons in the GD or the CA3 pyramidal neurons (Glasper et al., 2010). Similarly, when IGF-I is administered by ventricular infusion no effect was observed in the number of synapses in CA3 (Poe et al., 2001). However, a decrease in the serum IGF-I levels causes a reduction of glutamatergic boutons in the HP (Trejo et al., 2007). This finding suggests that IGF-I entry to the HP can promote synapse formation and/or maintenance and as such can be beneficial for spatial learning and to reduce anxiety-like behavior (Llorens-Martín et al., 2010; Baldini et al., 2013). In contrast, it has been recently reported that the suppression of IGF-IR signaling in KO mice enhances olfactory function in aged males but not in females, adding new levels of complexity to the understanding of the role of IGF-I/IGF-IR in the regulation of neurogenesis, synaptogenesis and function in the adult brain (Chaker et al., 2015; Figure 1).
The IGF-I affects the proliferation of progenitor cells, the survival of both progenitors and neurons, differentiation, and maturation of neurons in the neurogenic areas of the adult brain (Figure 1). In addition it regulates neuronal positioning and migration in the SVZ-OB. However, the studies performed have reported that the action of IGF-I signaling could be different or even opposite depending on the experimental approach used, the point in development and/or cell type affected. The production of cell-specific transgenic mouse lines, double KO for IGF-I and IGF-IR in combination with new technologies such as CRISPR-Cas9, optogenetics, and pharmacogenetics might contribute to the deeper understanding of the role and mechanisms of action of IGF-I/IGF-IR signaling during postnatal-adult neurogenesis. They also could help to elucidate the role of local and systemic IGF-I in this process and to identify new molecules regulated by this growth factor.
VN Manuscript writing. ÇD Revision of the manuscript. CV Manuscript writing Financial support Final approval of the manuscript.
The authors declare that the research was conducted in the absence of any commercial or financial relationships that could be construed as a potential conflict of interest.
The work in Carlos Vicario laboratory was funded by grants from the Spanish Ministerio de Ciencia e Innovación and Ministerio de Economía y Competitividad (MICINN and MINECO; BFU2007-61230, BFU2010-1963 and SAF2013-4759R, the Instituto de Salud Carlos III (ISCIII; CIBERNED CB06/05/0065), and the Comunidad de Madrid (S2011/BMD-2336) to CV. VN and ÇD were supported by a FPI Fellowship from the MICINN and MINECO and grants from CIBERNED and the European Union in the 7th framework program.
Aberg, M. A., Aberg, N. D., Hedbäcker, H., Oscarsson, J., and Eriksson, P. S. (2000). Peripheral infusion of IGF-I selectively induces neurogenesis in the adult rat hippocampus. J. Neurosci. 20, 2896–2903.
Aberg, M. A., Aberg, N. D., Palmer, T. D., Alborn, A. M., Carlsson-Skwirut, C., Bang, P., et al. (2003). IGF-I has a direct proliferative effect in adult hippocampal progenitor cells. Mol. Cell. Neurosci. 24, 23–40. doi: 10.1016/S1044-7431(03)00082-4
Aburto, M. R., Magariños, M., Leon, Y., Varela-Nieto, I., and Sanchez-Calderon, H. (2012). AKT signaling mediates IGF-I survival actions on otic neural progenitors. PLoS ONE 7:e30790. doi: 10.1371/journal.pone.0030790
Agis-Balboa, R. C., Arcos-Diaz, D., Wittnam, J., Govindarajan, N., Blom, K., Burkhardt, S., et al. (2011). A hippocampal insulin-growth factor 2 pathway regulates the extinction of fear memories. EMBO J. 30, 4071–4083. doi: 10.1038/emboj.2011.293
Agis-Balboa, R. C., and Fischer, A. (2014). Generating new neurons to circumvent your fears: the role of IGF signaling. Cell. Mol. Life Sci. 71, 21–42. doi: 10.1007/s00018-013-1316-2
Annunziata, M., Granata, R., and Ghigo, E. (2011). The IGF system. Acta Diabetol. 48, 1–9. doi: 10.1007/s00592-010-0227-z
Araki, W., Kume, H., Oda, A., Tamaoka, A., and Kametani, F. (2009). IGF-1 promotes beta-amyloid production by a secretase-independent mechanism. Biochem. Biophys. Res. Commun. 380, 111–114. doi: 10.1016/j.bbrc.2009.01.044
Arsenijevic, Y., Weiss, S., Schneider, B., and Aebischer, P. (2001). Insulin-like growth factor-I is necessary for neural stem cell proliferation and demonstrates distinct actions of epidermal growth factor and fibroblast growth factor-2. J. Neurosci. 21, 7194–7202.
Baker, J., Liu, J. P., Robertson, E. J., and Efstratiadis, A. (1993). Role of insulin-like growth factors in embryonic and postnatal growth. Cell 75, b73–b82. doi: 10.1016/S0092-8674(05)80085-6
Baldini, S., Restani, L., Baroncelli, L., Coltelli, M., Franco, R., Cenni, M. C., et al. (2013). Enriched early life experiences reduce adult anxiety-like behavior in rats: a role for insulin-like growth factor 1. J. Neurosci. 33, 11715–11723. doi: 10.1523/JNEUROSCI.3541-12.2013
Baltensperger, K., Kozma, L. M., Cherniack, A. D., Klarlund, J. K., Chawla, A., Banerjee, U., et al. (1993). Binding of the Ras activator son of sevenless to insulin receptor substrate-1 signaling complexes. Science 260, 1950–1952. doi: 10.1126/science.8391166
Bartlett, W. P., Li, X. S., and Williams, M. (1992). Expression of IGF-1 mRNA in the murine subventricular zone during postnatal development. Brain Res. Mol. Brain Res. 12, 285–291. doi: 10.1016/0169-328X(92)90131-T
Bartlett, W. P., Li, X. S., Williams, M., and Benkovic, S. (1991). Localization of insulin-like growth factor-1 mRNA in murine central nervous system during postnatal development. Dev. Biol. 147, 239–250. doi: 10.1016/S0012-1606(05)80021-1
Bateman, J. M., and McNeill, H. (2006). Insulin/IGF signalling in neurogenesis. Cell. Mol. Life Sci. 63, 1701–1705. doi: 10.1007/s00018-006-6036-4
Beck, K. D., Powell-Braxton, L., Widmer, H. R., Valverde, J., and Hefti, F. (1995). Igf1 gene disruption results in reduced brain size, CNS hypomyelination, and loss of hippocampal granule and striatal parvalbumin-containing neurons. Neuron 14, 717–730. doi: 10.1016/0896-6273(95)90216-3
Biondi, O., Branchu, J., Ben Salah, A., Houdebine, L., Bertin, L., Chali, F., et al. (2015). IGF-1R reduction triggers neuroprotective signaling pathways in spinal muscular atrophy mice. J. Neurosci. 35, 12063–12079. doi: 10.1523/JNEUROSCI.0608-15.2015
Bondy, C. A., and Cheng, C. M. (2004). Signaling by insulin-like growth factor 1 in brain. Eur. J. Pharmacol. 490, 25–31. doi: 10.1016/j.ejphar.2004.02.042
Bondy, C. A., Werner, H., Roberts, C. T. Jr., and LeRoith, D. (1990). Cellular pattern of insulin-like growth factor-I (IGF-I) and type I IGF receptor gene expression in early organogenesis: comparison with IGF-II gene expression. Mol. Endocrinol. 4, 1386–1398. doi: 10.1210/mend-4-9-1386
Brooker, G. J., Kalloniatis, M., Russo, V. C., Murphy, M., Werther, G. A., and Bartlett, P. F. (2000). Endogenous IGF-1 regulates the neuronal differentiation of adult stem cells. J. Neurosci. Res. 59, 332–341. doi: 10.1002/(SICI)1097-4547(20000201)59:3<332::AID-JNR6>3.0.CO;2-2
Burkhardt, S., Gesing, J., Kapellen, T. M., Kovacs, P., Kratzsch, J., Schlicke, M., et al. (2015). Novel heterozygous IGF1R mutation in two brothers with developing impaired glucose tolerance. J. Pediatr. Endocrinol. Metab. 28, 217–225. doi: 10.1515/jpem-2014-0132
Camarero, G., Avendano, C., Fernandez-Moreno, C., Villar, A., Contreras, J., de Pablo, F., et al. (2001). Delayed inner ear maturation and neuronal loss in postnatal Igf-1-deficient mice. J. Neurosci. 21, 7630–7641.
Cao, P., Maximov, A., and Südhof, T. C. (2011). Activity-dependent IGF-1 exocytosis is controlled by the Ca(2+)-sensor synaptotagmin-10. Cell 145, 300–311. doi: 10.1016/j.cell.2011.03.034
Cao, Y., Gunn, A. J., Bennet, L., Wu, D., George, S., Gluckman, P. D., et al. (2003). Insulin-like growth factor (IGF)-1 suppresses oligodendrocyte caspase-3 activation and increases glial proliferation after ischemia in near-term fetal sheep. J. Cereb. Blood Flow Metab. 23, 739–747. doi: 10.1097/01.WCB.0000067720.12805.6F
Carlson, S. W., Madathil, S. K., Sama, D. M., Gao, X., Chen, J., and Saatman, K. E. (2014). Conditional overexpression of insulin-like growth factor-1 enhances hippocampal neurogenesis and restores immature neuron dendritic processes after traumatic brain injury. J. Neuropathol. Exp. Neurol. 73, 734–746. doi: 10.1097/NEN.0000000000000092
Carro, E., Trejo, J. L., Gomez-Isla, T., LeRoith, D., and Torres-Aleman, I. (2002). Serum insulin-like growth factor I regulates brain amyloid-beta levels. Nat. Med. 8, 1390–1397. doi: 10.1038/nm1202-793
Carson, M. J., Behringer, R. R., Brinster, R. L., and McMorris, F. A. (1993). Insulin-like growth factor I increases brain growth and central nervous system myelination in transgenic mice. Neuron 10, 729–740. doi: 10.1016/0896-6273(93)90173-O
Cediel, R., Riquelme, R., Contreras, J., Díaz, A., and Varela-Nieto, I. (2006). Sensorineural hearing loss in insulin-like growth factor I-null mice: a new model of human deafness. Eur. J. Neurosci. 23, 587–590. doi: 10.1111/j.1460-9568.2005.04584.x
Croci, L., Barili, V., Chia, D., Massimino, L., van Vugt, R., Masserdotti, G., et al. (2010). Local insulin-like growth factor I expression is essential for Purkinje neuron survival at birth. Cell Death Differ. 18, 48–59. doi: 10.1038/cdd.2010.78
Cui, Q. L., and Almazan, G. (2007). IGF-I-induced oligodendrocyte progenitor proliferation requires PI3K/Akt, MEK/ERK, and Src-like tyrosine kinases. J. Neurochem. 100, 1480–1493. doi: 10.1111/j.1471-4159.2006.04329.x
Cundiff, P., Liu, L., Wang, Y., Zou, J., Pan, Y. W., Abel, G., et al. (2009). ERK5 MAP kinase regulates neurogenin1 during cortical neurogenesis. PLoS ONE 4:e5204. doi: 10.1371/journal.pone.0005204
Chaker, Z., Aïd, S., Berry, H., and Holzenberger, M. (2015). Suppression of IGF-I signals in neural stem cells enhances neurogenesis and olfactory function during aging. Aging Cell 14, 847–856. doi: 10.1111/acel.12365
Cheng, C. M., Cohen, M., Tseng, V., and Bondy, C. A. (2001). Endogenous IGF1 enhances cell survival in the postnatal dentate gyrus. J. Neurosci. Res. 64, 341–347. doi: 10.1002/jnr.1084
Cheng, C. M., Mervis, R. F., Niu, S. L., Salem, N. Jr. Witters, L. A., Tseng, V., et al. (2003). Insulin-like growth factor 1 is essential for normal dendritic growth. J. Neurosci. Res. 73, 1–9. doi: 10.1002/jnr.10634
Fernández-Moreno, C., Pichel, J. G., Chesnokova, V., and De Pablo, F. (2004). Increased leptin and white adipose tissue hypoplasia are sexually dimorphic in Lif null/Igf-I haploinsufficient mice. FEBS Lett. 557, 64–68. doi: 10.1016/S0014-5793(03)01445-5
Fernandez, A. M., and Torres-Alemán, I. (2012). The many faces of insulin-like peptide signalling in the brain. Nat. Rev. Neurosci. 13, 225–239. doi: 10.1038/nrn3209
Gago, N., Avellana-Adalid, V., Baron-Van Evercooren, A., and Schumacher, M. (2003). Control of cell survival and proliferation of postnatal PSA-NCAM(+) progenitors. Mol. Cell. Neurosci. 22, 162–178. doi: 10.1016/S1044-7431(02)00030-1
Gao, W. Q., Shinsky, N., Ingle, G., Beck, K., Elias, K. A., and Powell-Braxton, L. (1999). IGF-I deficient mice show reduced peripheral nerve conduction velocities and decreased axonal diameters and respond to exogenous IGF-I treatment. J. Neurobiol. 39, 142–152.
Glasper, E. R., Llorens-Martin, M. V., Leuner, B., Gould, E., and Trejo, J. L. (2010). Blockade of insulin-like growth factor-I has complex effects on structural plasticity in the hippocampus. Hippocampus 20, 706–712. doi: 10.1002/hipo.20672
Gontier, G., George, C., Chaker, Z., Holzenberger, M., and Aïd, S. (2015). Blocking IGF signaling in adult neurons alleviates alzheimer's disease pathology through amyloid-beta clearance. J. Neurosci. 35, 11500–11513. doi: 10.1523/JNEUROSCI.0343-15.2015
Hernández-Sánchez, C., López-Carranza, A., Alarcón, C., de La Rosa, E. J., and de Pablo, F. (1995). Autocrine/paracrine role of insulin-related growth factors in neurogenesis: local expression and effects on cell proliferation and differentiation in retina. Proc. Natl. Acad. Sci. U.S.A. 92, 9834–9838. doi: 10.1073/pnas.92.21.9834
Hernández-Sánchez, C., Mansilla, A., de Pablo, F., and Zardoya, R. (2008). Evolution of the insulin receptor family and receptor isoform expression in vertebrates. Mol. Biol. Evol. 25, 1043–1053. doi: 10.1093/molbev/msn036
Hodge, R. D., D'Ercole, A. J., and O'Kusky, J. R. (2004). Insulin-like growth factor-I accelerates the cell cycle by decreasing G1 phase length and increases cell cycle reentry in the embryonic cerebral cortex. J. Neurosci. 24, 10201–10210. doi: 10.1523/JNEUROSCI.3246-04.2004
Hodge, R. D., D'Ercole, A. J., and O'Kusky, J. R. (2007). Insulin-like growth factor-I (IGF-I) inhibits neuronal apoptosis in the developing cerebral cortex in vivo. Int. J. Dev. Neurosci. 25, 233–241. doi: 10.1016/j.ijdevneu.2007.03.004
Hsieh, J., Aimone, J. B., Kaspar, B. K., Kuwabara, T., Nakashima, K., and Gage, F. H. (2004). IGF-I instructs multipotent adult neural progenitor cells to become oligodendrocytes. J. Cell Biol. 164, 111–122. doi: 10.1083/jcb.200308101
Hu, Q., Lee, S. Y., O'Kusky, J. R., and Ye, P. (2012). Signaling through the type 1 insulin-like Growth Factor Receptor (IGF1R) interacts with canonical Wnt signaling to promote neural proliferation in developing brain. ASN Neuro 4:e00092. doi: 10.1042/AN20120009
Hurtado-Chong, A., Yusta-Boyo, M. J., Vergaño-Vera, E., Bulfone, A., de Pablo, F., and Vicario-Abejón, C. (2009). IGF-I promotes neuronal migration and positioning in the olfactory bulb and the exit of neuroblasts from the subventricular zone. Eur. J. Neurosci. 30, 742–755. doi: 10.1111/j.1460-9568.2009.06870.x
Hwa, V., Oh, Y., and Rosenfeld, R. G. (1999). The insulin-like growth factor-binding protein (IGFBP) superfamily. Endocr. Rev. 20, 761–787. doi: 10.1210/er.20.6.761
Iwanami, A., Cloughesy, T. F., and Mischel, P. S. (2009). Striking the balance between PTEN and PDK1: it all depends on the cell context. Genes Dev. 23, 1699–1704. doi: 10.1101/gad.1832909
Junnila, R. K., List, E. O., Berryman, D. E., Murrey, J. W., and Kopchick, J. J. (2013). The GH/IGF-1 axis in ageing and longevity. Nat. Rev. Endocrinol. 9, 366–376. doi: 10.1038/nrendo.2013.67
Kakizawa, S., Yamada, K., Iino, M., Watanabe, M., and Kano, M. (2003). Effects of insulin-like growth factor I on climbing fibre synapse elimination during cerebellar development. Eur. J. Neurosci. 17, 545–554. doi: 10.1046/j.1460-9568.2003.02486.x
Kalluri, H. S., Vemuganti, R., and Dempsey, R. J. (2007). Mechanism of insulin-like growth factor I-mediated proliferation of adult neural progenitor cells: role of Akt. Eur. J. Neurosci. 25, 1041–1048. doi: 10.1111/j.1460-9568.2007.05336.x
Kappeler, L., De Magalhaes Filho, C., Dupont, J., Leneuve, P., Cervera, P., Périn, L., et al. (2008). Brain IGF-1 receptors control mammalian growth and lifespan through a neuroendocrine mechanism. PLoS Biol. 6:e254. doi: 10.1371/journal.pbio.0060254
Kouroupi, G., Lavdas, A. A., Gaitanou, M., Thomaidou, D., Stylianopoulou, F., and Matsas, R. (2010). Lentivirus-mediated expression of insulin-like growth factor-I promotes neural stem/precursor cell proliferation and enhances their potential to generate neurons. J. Neurochem. 115, 460–474. doi: 10.1111/j.1471-4159.2010.06939.x
Laurino, L., Wang, X. X., de la Houssaye, B. A., Sosa, L., Dupraz, S., Cáceres, A., et al. (2005). PI3K activation by IGF-1 is essential for the regulation of membrane expansion at the nerve growth cone. J. Cell Sci. 118, 3653–3662. doi: 10.1242/jcs.02490
Li, Y., Komuro, Y., Fahrion, J. K., Hu, T., Ohno, N., Fenner, K. B., et al. (2012). Light stimuli control neuronal migration by altering of insulin-like growth factor 1 (IGF-1) signaling. Proc. Natl. Acad. Sci. U.S.A. 109, 2630–2635. doi: 10.1073/pnas.1111326109
Lichtenwalner, R. J., Forbes, M. E., Sonntag, W. E., and Riddle, D. R. (2006). Adult-onset deficiency in growth hormone and insulin-like growth factor-I decreases survival of dentate granule neurons: insights into the regulation of adult hippocampal neurogenesis. J. Neurosci. Res. 83, 199–210. doi: 10.1002/jnr.20719
Liu, J. P., Baker, J., Perkins, A. S., Robertson, E. J., and Efstratiadis, A. (1993). Mice carrying null mutations of the genes encoding insulin-like growth factor I (Igf-1) and type 1 IGF receptor (Igf1r). Cell 75, 59–72. doi: 10.1016/s0092-8674(05)80084-4
Liu, W., D'Ercole, J. A., and Ye, P. (2011). Blunting type 1 insulin-like growth factor receptor expression exacerbates neuronal apoptosis following hypoxic/ischemic injury. BMC Neurosci. 12:64. doi: 10.1186/1471-2202-12-64
Liu, W., Ye, P., O'Kusky, J. R., and D'Ercole, A. J. (2009). Type 1 insulin-like growth factor receptor signaling is essential for the development of the hippocampal formation and dentate gyrus. J. Neurosci. Res. 87, 2821–2832. doi: 10.1002/jnr.22129
Lunn, J. S., Sakowski, S. A., McGinley, L. M., Pacut, C., Hazel, T. G., Johe, K., et al. (2015). Autocrine production of IGF-I increases stem cell-mediated neuroprotection. Stem Cells 33, 1480–1489. doi: 10.1002/stem.1933
Llorens-Martín, M., Torres-Aleman, I., and Trejo, J. L. (2010). Exercise modulates insulin-like growth factor 1-dependent and -independent effects on adult hippocampal neurogenesis and behaviour. Mol. Cell. Neurosci. 44, 109–117. doi: 10.1016/j.mcn.2010.02.006
Magariños, M., Aburto, M. R., Sánchez-Calderón, H., Muñoz-Agudo, C., Rapp, U. R., and Varela-Nieto, I. (2010). RAF kinase activity regulates neuroepithelial cell proliferation and neuronal progenitor cell differentiation during early inner ear development. PLoS ONE 5:e14435. doi: 10.1371/journal.pone.0014435
Mairet-Coello, G., Tury, A., and DiCicco-Bloom, E. (2009). Insulin-like growth factor-1 promotes G(1)/S cell cycle progression through bidirectional regulation of cyclins and cyclin-dependent kinase inhibitors via the phosphatidylinositol 3-kinase/Akt pathway in developing rat cerebral cortex. J. Neurosci. 29, 775–788. doi: 10.1523/JNEUROSCI.1700-08.2009
Maucksch, C., McGregor, A. L., Yang, M., Gordon, R. J., Yang, M., and Connor, B. (2013). IGF-I redirects doublecortin-positive cell migration in the normal adult rat brain. Neuroscience 241, 106–115. doi: 10.1016/j.neuroscience.2013.03.021
Moreno-Barriuso, N., López-Malpartida, A. V., de Pablo, F., and Pichel, J. G. (2006). Alterations in alveolar epithelium differentiation and vasculogenesis in lungs of LIF/IGF-I double deficient embryos. Dev. Dyn. 235, 2040–2050. doi: 10.1002/dvdy.20842
Nishijima, T., Piriz, J., Duflot, S., Fernandez, A. M., Gaitan, G., Gomez-Pinedo, U., et al. (2010). Neuronal activity drives localized blood-brain-barrier transport of serum insulin-like growth factor-I into the CNS. Neuron 67, 834–846. doi: 10.1016/j.neuron.2010.08.007
O'Kusky, J., and Ye, P. (2012). Neurodevelopmental effects of insulin-like growth factor signaling. Front. Neuroendocrinol. 33:2. doi: 10.1016/j.yfrne.2012.06.002
O'Kusky, J. R., Ye, P., and D'Ercole, A. J. (2000). Insulin-like growth factor-I promotes neurogenesis and synaptogenesis in the hippocampal dentate gyrus during postnatal development. J. Neurosci. 20, 8435–8442.
Ocrant, I., Fay, C. T., and Parmelee, J. T. (1990). Characterization of insulin-like growth factor binding proteins produced in the rat central nervous system. Endocrinology 127, 1260–1267. doi: 10.1210/endo-127-3-1260
Onuma, T. A., Ding, Y., Abraham, E., Zohar, Y., Ando, H., and Duan, C. (2011). Regulation of temporal and spatial organization of newborn GnRH neurons by IGF signaling in zebrafish. J. Neurosci. 31, 11814–11824. doi: 10.1523/JNEUROSCI.6804-10.2011
Otaegi, G., Yusta-Boyo, M. J., Vergaño-Vera, E., Méndez-Gómez, H. R., Carrera, A. C., Abad, J. L., et al. (2006). Modulation of the PI 3-kinase-Akt signalling pathway by IGF-I and PTEN regulates the differentiation of neural stem/precursor cells. J. Cell Sci. 119, 2739–2748. doi: 10.1242/jcs.03012
Pais, R. S., Moreno-Barriuso, N., Hernández-Porras, I., López, I. P., De Las Rivas, J., and Pichel, J. G. (2013). Transcriptome analysis in prenatal IGF1-deficient mice identifies molecular pathways and target genes involved in distal lung differentiation. PLoS ONE 8:e83028. doi: 10.1371/journal.pone.0083028
Paliouras, G. N., Hamilton, L. K., Aumont, A., Joppé, S. E., Barnabé-Heider, F., and Fernandes, K. J. (2012). Mammalian target of rapamycin signaling is a key regulator of the transit-amplifying progenitor pool in the adult and aging forebrain. J. Neurosci. 32, 15012–15026. doi: 10.1523/JNEUROSCI.2248-12.2012
Pérez-Martín, M., Cifuentes, M., Grondona, J. M., López-Avalos, M. D., Gómez-Pinedo, U., García-Verdugo, J. M., et al. (2010). IGF-I stimulates neurogenesis in the hypothalamus of adult rats. Eur. J. Neurosci. 31, 1533–1548. doi: 10.1111/j.1460-9568.2010.07220.x
Pichel, J. G., Fernández-Moreno, C., Vicario-Abejón, C., Testillano, P. S., Patterson, P. H., and de Pablo, F. (2003). Developmental cooperation of leukemia inhibitory factor and insulin-like growth factor I in mice is tissue-specific and essential for lung maturation involving the transcription factors Sp3 and TTF-1. Mech. Dev. 120, 349–361. doi: 10.1016/S0925-4773(02)00449-5
Poe, B. H., Linville, C., Riddle, D. R., Sonntag, W. E., and Brunso-Bechtold, J. K. (2001). Effects of age and insulin-like growth factor-1 on neuron and synapse numbers in area CA3 of hippocampus. Neuroscience 107, 231–238. doi: 10.1016/S0306-4522(01)00341-4
Popken, G. J., Hodge, R. D., Ye, P., Zhang, J., Ng, W., O'Kusky, J. R., et al. (2004). In vivo effects of insulin-like growth factor-I (IGF-I) on prenatal and early postnatal development of the central nervous system. Eur. J. Neurosci. 19, 2056–2068. doi: 10.1111/j.0953-816X.2004.03320.x
Powell-Braxton, L., Hollingshead, P., Warburton, C., Dowd, M., Pitts-Meek, S., Dalton, D., et al. (1993). IGF-I is required for normal embryonic growth in mice. Genes Dev. 7, 2609–2617. doi: 10.1101/gad.7.12b.2609
Puche, J. E., and Castilla-Cortázar, I. (2012). Human conditions of insulin-like growth factor-I (IGF-I) deficiency. J. Transl. Med. 10, 224. doi: 10.1186/1479-5876-10-224
Puglianiello, A., Germani, D., Rossi, P., and Cianfarani, S. (2000). IGF-I stimulates chemotaxis of human neuroblasts. Involvement of type 1 IGF receptor, IGF binding proteins, phosphatidylinositol-3 kinase pathway and plasmin system. J. Endocrinol. 165, 123–131. doi: 10.1677/joe.0.1650123
Pun, R. Y., Rolle, I. J., Lasarge, C. L., Hosford, B. E., Rosen, J. M., Uhl, J. D., et al. (2012). Excessive activation of mTOR in postnatally generated granule cells is sufficient to cause epilepsy. Neuron 75, 1022–1034. doi: 10.1016/j.neuron.2012.08.002
Roback, E. W., Barakat, A. J., Dev, V. G., Mbikay, M., Chrétien, M., and Butler, M. G. (1991). An infant with deletion of the distal long arm of chromosome 15 (q26.1—-qter) and loss of insulin-like growth factor 1 receptor gene. Am. J. Med. Genet. 38, 74–79. doi: 10.1002/ajmg.1320380117
Rodríguez-de la Rosa, L., Sánchez-Calderón, H., Contreras, J., Murillo-Cuesta, S., Falagan, S., Avendaño, C., et al. (2015). Comparative gene expression study of the vestibular organ of the Igf1 deficient mouse using whole-transcript arrays. Hear. Res. 330(Pt A), 62–77. doi: 10.1016/j.heares.2015.08.016
Rotwein, P., Burgess, S. K., Milbrandt, J. D., and Krause, J. E. (1988). Differential expression of insulin-like growth factor genes in rat central nervous system. Proc. Natl. Acad. Sci. U.S.A. 85, 265–269. doi: 10.1073/pnas.85.1.265
Russo, V. C., Gluckman, P. D., Feldman, E. L., and Werther, G. A. (2005). The insulin-like growth factor system and its pleiotropic functions in brain. Endocr. Rev. 26, 916–943. doi: 10.1210/er.2004-0024
Scolnick, J. A., Cui, K., Duggan, C. D., Xuan, S., Yuan, X.-B., Efstratiadis, A., et al. (2008). Role of IGF signaling in olfactory sensory map formation and axon guidance. Neuron 57, 847–857. doi: 10.1016/j.neuron.2008.01.027
Skolnik, E. Y., Lee, C. H., Batzer, A., Vicentini, L. M., Zhou, M., Daly, R., et al. (1993). The SH2/SH3 domain-containing protein GRB2 interacts with tyrosine-phosphorylated IRS1 and Shc: implications for insulin control of ras signalling. EMBO J. 12, 1929–1936.
Sosa, L., Dupraz, S., Laurino, L., Bollati, F., Bisbal, M., Cáceres, A., et al. (2006). IGF-1 receptor is essential for the establishment of hippocampal neuronal polarity. Nat. Neurosci. 9, 993–995. doi: 10.1038/nn1742
Stratikopoulos, E., Szabolcs, M., Dragatsis, I., Klinakis, A., and Efstratiadis, A. (2008). The hormonal action of IGF1 in postnatal mouse growth. Proc. Natl. Acad. Sci. U.S.A. 105, 19378–19383. doi: 10.1073/pnas.0809223105
Sun, L. Y. (2006). Hippocampal IGF-1 expression, neurogenesis and slowed aging: clues to longevity from mutant mice. Age (Dordr). 28, 181–189. doi: 10.1007/s11357-006-9009-5
Sun, L. Y., Evans, M. S., Hsieh, J., Panici, J., and Bartke, A. (2005). Increased neurogenesis in dentate gyrus of long-lived Ames dwarf mice. Endocrinology 146, 1138–1144. doi: 10.1210/en.2004-1115
Torres-Aleman, I., Naftolin, F., and Robbins, R. J. (1990). Trophic effects of basic fibroblast growth factor on fetal rat hypothalamic cells: interactions with insulin-like growth factor I. Brain Res. Dev. Brain Res. 52, 253–257. doi: 10.1016/0165-3806(90)90242-Q
Trejo, J. L., Carro, E., and Torres-Aleman, I. (2001). Circulating insulin-like growth factor I mediates exercise-induced increases in the number of new neurons in the adult hippocampus. J. Neurosci. 21, 1628–1634.
Trejo, J. L., Llorens-Martín, M. V., and Torres-Alemán, I. (2008). The effects of exercise on spatial learning and anxiety-like behavior are mediated by an IGF-I-dependent mechanism related to hippocampal neurogenesis. Mol. Cell. Neurosci. 37, 402–411. doi: 10.1016/j.mcn.2007.10.016
Trejo, J. L., Piriz, J., Llorens-Martin, M. V., Fernandez, A. M., Bolós, M., LeRoith, D., et al. (2007). Central actions of liver-derived insulin-like growth factor I underlying its pro-cognitive effects. Mol. Psychiatry 12, 1118–1128. doi: 10.1038/sj.mp.4002076
van Duyvenvoorde, H. A., van Setten, P. A., Walenkamp, M. J., van Doorn, J., Koenig, J., Gauguin, L., et al. (2010). Short stature associated with a novel heterozygous mutation in the insulin-like growth factor 1 gene. J. Clin. Endocrinol. Metab. 95, E363–E367. doi: 10.1210/jc.2010-0511
Versteyhe, S., Klaproth, B., Borup, R., Palsgaard, J., Jensen, M., Gray, S. G., et al. (2013). IGF-I, IGF-II, and insulin stimulate different gene expression responses through binding to the IGF-I Receptor. Front. Endocrinol. (Lausanne). 4:98. doi: 10.3389/fendo.2013.00098
Vicario-Abejón, C., Fernandez-Moreno, C., Pichel, J. G., and de Pablo, F. (2004). Mice lacking IGF-I and LIF have motoneuron deficits in brain stem nuclei. Neuroreport 15, 2769–2772.
Vicario-Abejón, C., Yusta-Boyo, M. J., Fernández-Moreno, C., and de Pablo, F. (2003). Locally born olfactory bulb stem cells proliferate in response to insulin-related factors and require endogenous insulin-like growth factor-I for differentiation into neurons and glia. J. Neurosci. 23, 895–906.
Vogel, T. (2013). “Insulin/IGF-signalling in embryonic and adult neural proliferation and differentiation in the mammalian central nervous system,” in Trends in Cell Signaling Pathways in Neuronal Fate Decision, ed S. Wislet-Gendebien (Rijeka: InTech), 37–73.
Walenkamp, M. J., Karperien, M., Pereira, A. M., Hilhorst-Hofstee, Y., van Doorn, J., Chen, J. W., et al. (2005). Homozygous and heterozygous expression of a novel insulin-like growth factor-I mutation. J. Clin. Endocrinol. Metab. 90, 2855–2864. doi: 10.1210/jc.2004-1254
Walenkamp, M. J., Losekoot, M., and Wit, J. M. (2013). Molecular IGF-1 and IGF-1 receptor defects: from genetics to clinical management. Endocr. Dev. 24, 128–137. doi: 10.1159/000342841
Wang, J., Zhou, J., Powell-Braxton, L., and Bondy, C. (1999). Effects of Igf1 gene deletion on postnatal growth patterns. Endocrinology 140, 3391–3394. doi: 10.1210/endo.140.7.7045
Witsch, J., Szafranski, P., Chen, C. A., Immken, L., Simpson Patel, G., Hixson, P., et al. (2013). Intragenic deletions of the IGF1 receptor gene in five individuals with psychiatric phenotypes and developmental delay. Eur. J. Hum. Genet. 21, 1304–1307. doi: 10.1038/ejhg.2013.42
Woods, K. A., Camacho-Hübner, C., Savage, M. O., and Clark, A. J. (1996). Intrauterine growth retardation and postnatal growth failure associated with deletion of the insulin-like growth factor I gene. N. Engl. J. Med. 335, 1363–1367. doi: 10.1056/NEJM199610313351805
Wu, Y., Sun, H., Yakar, S., and LeRoith, D. (2009). Elevated levels of insulin-like growth factor (IGF)-I in serum rescue the severe growth retardation of IGF-I null mice. Endocrinology 150, 4395–4403. doi: 10.1210/en.2009-0272
Xiang, Y., Ding, N., Xing, Z., Zhang, W., Liu, H., and Li, Z. (2011). Insulin-like growth factor-1 regulates neurite outgrowth and neuronal migration from organotypic cultured dorsal root ganglion. Int. J. Neurosci. 121, 101–106. doi: 10.3109/00207454.2010.535935
Yakar, S., Rosen, C. J., Beamer, W. G., Ackert-Bicknell, C. L., Wu, Y., Liu, J.-L., et al. (2002). Circulating levels of IGF-1 directly regulate bone growth and density. J. Clin. Invest. 110, 771–781. doi: 10.1172/JCI0215463
Ye, P., Carson, J., and D'Ercole, A. J. (1995). In vivo actions of insulin-like growth factor-I (IGF-I) on brain myelination: studies of IGF-I and IGF binding protein-1 (IGFBP-1) transgenic mice. J. Neurosci. 15, 7344–7356.
Ye, P., and D'Ercole, A. J. (2006). Insulin-like growth factor actions during development of neural stem cells and progenitors in the central nervous system. J. Neurosci. Res. 83, 1–6. doi: 10.1002/jnr.20688
Ye, P., Popken, G. J., Kemper, A., McCarthy, K., Popko, B., and D'Ercole, A. J. (2004). Astrocyte-specific overexpression of insulin-like growth factor-I promotes brain overgrowth and glial fibrillary acidic protein expression. J. Neurosci. Res. 78, 472–484. doi: 10.1002/jnr.20288
Ye, P., Umayahara, Y., Ritter, D., Bunting, T., Auman, H., Rotwein, P., et al. (1997). Regulation of insulin-like growth factor I (IGF-I) gene expression in brain of transgenic mice expressing an IGF-I-luciferase fusion gene. Endocrinology 138, 5466–5475. doi: 10.1210/en.138.12.5466
Yuan, H., Chen, R., Wu, L., Chen, Q., Hu, A., Zhang, T., et al. (2015). The regulatory mechanism of neurogenesis by IGF-1 in adult mice. Mol. Neurobiol. 51, 512–522. doi: 10.1007/s12035-014-8717-6
Zhang, X., Zhang, L., Cheng, X., Guo, Y., Sun, X., Chen, G., et al. (2014). IGF-1 promotes Brn-4 expression and neuronal differentiation of neural stem cells via the PI3K/Akt pathway. PLoS ONE 9:e113801. doi: 10.1371/journal.pone.0113801
Keywords: IGF-I, neurogenesis, proliferation, survival, differentiation, maturation, migration, IGF-IR
Citation: Nieto-Estévez V, Defterali Ç and Vicario-Abejón C (2016) IGF-I: A Key Growth Factor that Regulates Neurogenesis and Synaptogenesis from Embryonic to Adult Stages of the Brain. Front. Neurosci. 10:52. doi: 10.3389/fnins.2016.00052
Received: 07 December 2015; Accepted: 05 February 2016;
Published: 23 February 2016.
Edited by:
Alino Martinez-Marcos, Universidad de Castilla, SpainReviewed by:
Jacques Epelbaum, Centre de Psychiatrie & Neurosciences, Université Paris Descartes, FranceCopyright © 2016 Nieto-Estévez, Defterali and Vicario-Abejón. This is an open-access article distributed under the terms of the Creative Commons Attribution License (CC BY). The use, distribution or reproduction in other forums is permitted, provided the original author(s) or licensor are credited and that the original publication in this journal is cited, in accordance with accepted academic practice. No use, distribution or reproduction is permitted which does not comply with these terms.
*Correspondence: Carlos Vicario-Abejón, Y3ZpY2FyaW9AY2FqYWwuY3NpYy5lcw==
Disclaimer: All claims expressed in this article are solely those of the authors and do not necessarily represent those of their affiliated organizations, or those of the publisher, the editors and the reviewers. Any product that may be evaluated in this article or claim that may be made by its manufacturer is not guaranteed or endorsed by the publisher.
Research integrity at Frontiers
Learn more about the work of our research integrity team to safeguard the quality of each article we publish.