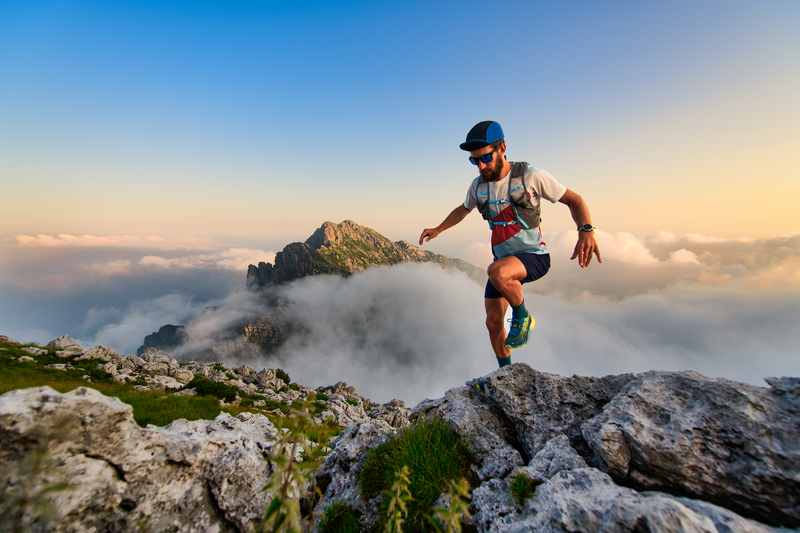
95% of researchers rate our articles as excellent or good
Learn more about the work of our research integrity team to safeguard the quality of each article we publish.
Find out more
MINI REVIEW article
Front. Neurosci. , 27 May 2015
Sec. Neuropharmacology
Volume 9 - 2015 | https://doi.org/10.3389/fnins.2015.00178
This article is part of the Research Topic Short- and long-term effects of methamphetamine exposure View all 8 articles
Parts of this article's content have been modified or rectified in:
Erratum on: Methamphetamine and the hypothalamic-pituitary-adrenal axis
Psychostimulants such as methamphetamine (MA) induce significant alterations in the function of the hypothalamic-pituitary-adrenal (HPA) axis. These changes in HPA axis function are associated with altered stress-related behaviors and might contribute to addictive processes such as relapse. In this mini-review we discuss acute and chronic effects of MA (adult and developmental exposure) on the HPA axis, including effects on HPA axis associated genes/proteins, brain regions, and behaviors such as anxiety and depression. A better understanding of the mechanisms through which MA affects the HPA axis may lead to more effective treatment strategies for MA addiction.
Abuse of amphetamine-like substances, including methamphetamine (MA), ranks second to cannabis in terms of worldwide illicit drug use (UNODC, 2011). A 2012 report indicates that in the United States MA is abused by approximately 439,000 people (SAMHSA, 2012), costing the United States approximately $23.4 billion annually (Nicosia et al., 2009). MA abuse is associated with high rates of relapse. A recent study indicated that 61% of MA users relapse to MA abuse within a year of release from treatment programs (Brecht and Herbeck, 2014). Furthermore, only 13% of individuals maintained abstinence from MA over the 5 year duration of the study (Brecht and Herbeck, 2014). Despite widespread need for improved MA addiction treatment options, current psychological and particularly pharmacological treatments for MA abuse have had limited success (Carson and Taylor, 2014).
Stress and the hypothalamic-pituitary-adrenal (HPA) axis are key components in psychostimulant addiction. Repeated drug use can induce functional and morphological changes in the HPA axis including alterations in stress hormone release as well as genes and proteins [e.g., corticotropin-releasing factor (CRF), arginine vasopressin (AVP), and glucocorticoid receptor (GR)] within brain regions pertinent to HPA axis function [e.g., extended amygdala, paraventricular hypothalamus (PVN); (Mantsch et al., 2007; Nawata et al., 2012)]. These changes contribute to a shift to the anti-reward pathway of drug abuse which is hypothesized to be activated secondarily to the reward pathway. This involves a change in the motivation from abusing drugs for their “pleasurable” effects to a desire to reduce internal distress (Koob et al., 2014). Emerging studies in humans indicate that the HPA axis is altered by repeated MA use, with individuals showing altered levels of stress hormones during withdrawal (Carson et al., 2012; Li et al., 2013). These alterations may contribute to adverse effects of MA, such as depression and anxiety, which in turn perpetuate further MA use. In this mini-review we summarize existing literature related to MA effects on the HPA axis and associated stress-related behaviors.
Numerous studies have demonstrated that MA activates the HPA axis (Morimasa et al., 1987; Williams et al., 2000; Zuloaga et al., 2014). Similar to stress- induced activation of the HPA axis, increased production of adrenocorticotropic hormone (ACTH) secretagogues (CRF and AVP) in neurons of the PVN is essential. When stimulated, AVP-expressing cells of the parvocellular PVN act in conjunction with CRF-expressing cells to activate cells in the anterior pituitary gland. This, in turn, stimulates cleavage of proopiomelanocortin (POMC) to produce ACTH in corticotropic cells. ACTH then enters the systemic blood stream and stimulates the adrenal cortex to release glucocorticoids (e.g., corticosterone and cortisol). Glucocorticoids circulate throughout the body and exert effects on numerous organs including the brain. This response is terminated through activation of GRs in the brain and pituitary which, via negative feedback, decrease further release of CRF and ACTH.
Activation of the HPA is an adaptive response which prepares an organism for “fight or flight” associated situations. However, in instances of chronic activation of the HPA axis (e.g., glucocorticoid treatments, chronic stress, or abuse of certain illicit drugs) glucocorticoids can be harmful. Excessive glucocorticoid exposure can exert negative effects on the brain including: increasing cell death (Zuloaga et al., 2011), altering cell proliferation patterns (Kim et al., 2004), and inducing dendritic remodeling (Kim et al., 2014). Repeated exposure to elevated glucocorticoids can also alter brain expression of genes and proteins associated with the HPA axis including CRF, AVP, GR, and mineralocorticoid receptor (MR; Imaki et al., 1991; Reul et al., 1987; Herman et al., 1989; Sawchenko et al., 1993). These alterations contribute to lasting effects on HPA axis function. Disrupted HPA axis function, including altered glucocorticoid release, are hallmarks of depressive and anxiety spectrum disorders in humans as well as related symptomology in rodents (Carroll et al., 1968; Landgraf et al., 1999). These affective mood states have been linked to both the onset of drug use as well as relapse (Haass-Koffler et al., 2014). See Figure 1 for a hypothetical mechanism through which MA use, via hyper-activation of the HPA axis, may induce changes in HPA axis associated genes/proteins within pertinent brain regions. Disruptions in the HPA axis and related behaviors such as anxiety and depression may potentially contribute to subsequent MA use.
Figure 1. Proposed scenario for MA effects on the HPA axis and behavior. MA activation of the HPA axis involves release of CRF from neurosecretory cells of the paraventricular hypothalamic nucleus and subsequent release of ACTH and glucocorticoids from the anterior pituitary and adrenal cortex, respectively. Release of glucocorticoids is greater in females than males. High levels of glucocorticoids may contribute to alterations in HPA axis associated genes/proteins (CRF, AVP, GR). These effects in the brain may subsequently alter HPA axis function (release of stress hormones) and in turn affect anxiety- and depressive-related behaviors often observed during withdrawal. Altered HPA axis function and mood behaviors may provide bi-directional positive feedback, thereby exacerbating these effects. Altered mood behaviors can lead to a need to alleviate an aversive mood state, thereby increasing the risk of further MA use as a means to reduce internal distress. The relationship between the elevated glucocorticoid response to MA in females and brain and behavioral consequences are currently unknown.
MA administration, ranging from 1 to 40 mg/kg, induces robust increases in corticosterone in rodents (Herring et al., 2008a; Zuloaga et al., 2014) and cortisol in nonhuman primates (Madden et al., 2005). A study in humans also reported elevated plasma cortisol in MA users that were intravenously administered a 0.5 mg/kg dose of MA (Harris et al., 2003). Recent studies in humans also indicate that chronic MA abuse can alter HPA axis function (Carson et al., 2012; Li et al., 2013). MA-dependent individuals show elevated ACTH and decreased cortisol levels at 96 h of MA abstinence compared to matched controls Li et al., 2013. These same MA-dependent individuals displayed elevated depressive symptomology (Li et al., 2013), indicating that both the HPA axis and associated behaviors are affected in recently abstinent MA abusers. Withdrawal from MA is commonly associated with elevated states of anxiety and depression (London et al., 2004; Cruickshank and Dyer, 2009), although whether these mood states are directly linked to altered HPA axis function is unknown.
In rats, chronic MA self-administration also increases anxiety and depressive-like behaviors during withdrawal from MA (Nawata et al., 2012; Jang et al., 2013). A single high dose injection of MA can also induce lasting depressive-like behavior in mice (Silva et al., 2014). Studies investigating effects of adult chronic MA on glucocorticoid release are few in number and have yielded mixed results, which may depend on timing, dose and method of administration. For example, Nawata et al. (2012) reported no alterations in blood corticosterone levels at 10 days of withdrawal from MA self-administration. However, other studies have reported lasting effects of MA on glucocorticoid levels. A neurotoxic dose of MA elevated basal corticosterone levels compared to controls at 24 h, 72 h, and 47 days after administration (Herring et al., 2008a,b; Grace et al., 2010b). Furthermore, chronic MA administration in drinking water alters the circadian rhythm of corticosterone 1 week post-withdrawal (Morimasa et al., 1987). Further studies specifically directed at investigating the HPA axis are needed to clarify the role of chronic MA on subsequent glucocorticoid release.
Because the rate of MA abuse is high in women of childbearing age, there is increased interest in the developmental effects on infants prenatally exposed to MA. However, there are few investigations of either short- or long-term effects of early developmental MA exposure, particularly in the context of alterations in the HPA axis function. A 2-year follow up study of children prenatally exposed to MA found that stress-induced cortisol levels were significantly higher in MA-exposed infants (Kirlic et al., 2013). Behavioral effects that may be associated with HPA axis alterations in prenatal MA exposed human offspring include altered arousal in infants (Smith et al., 2012; Kiblawi et al., 2014) and increased emotional reactivity and anxiety/depressed behavior in 3–5 year olds (LaGasse et al., 2012; Abar et al., 2013). Polydrug abuse, domestic violence, and emotional neglect are highly associated with parental MA abuse. These confounds make it difficult to dissociate effects of MA from other variables, necessitating use of animal models to explore specific effects of MA.
Extensive brain, particularly hippocampal, development occurs during the postnatal period in the rodent, while in humans analogous development occurs prenatally (Workman et al., 2013). Due to these differences in developmental trajectory, the majority of studies involving developmental MA and the HPA axis in rodents have utilized neonatal administration. Similar to adult MA exposure, neonatal administration also induces robust elevations in blood corticosterone (Acevedo et al., 2008; Grace et al., 2008). These elevations in corticosterone are greater than those induced by severe psychological stressors such as forced swim (Grace et al., 2008). An important consideration in developmental MA models is the timing of treatment which can cause differential effects on glucocorticoid release and behavior (Williams et al., 2003; Schaefer et al., 2008). Specifically, treatment that coincides with the neonatal stress hyporesponsive period (SHRP), during which basal and stress/drug induced glucocorticoid release are naturally blunted, results in the greatest long-term deficits in learning and memory (Acevedo et al., 2007; Vorhees et al., 2009).
Although long-term deficits on learning and memory following neonatal MA exposure are well documented, lasting effects on HPA axis function and stress-related behaviors are more subtle and findings are mixed. MA exposure near the neonatal SHRP results in subtle effects on adult HPA axis responses, including a more rapid rise in plasma corticosterone after forced swim (Grace et al., 2012). However, no long term effects in plasma corticosterone were found in similarly exposed rats following adult forced confinement or challenge with MA (Grace et al., 2012). Prenatal MA exposure in rats failed to induce any effects on adult basal plasma ACTH and corticosterone levels (Cabrera et al., 1993). Furthermore, several reports indicate that neonatal MA exposure does not alter anxiety-like behaviors in adolescent or adult mice (Siegel et al., 2011; Eastwood et al., 2012). However, in rats, several neonatal MA treatment regimens result in subtle decreases in anxiety-like behaviors in adulthood (Williams et al., 2003; Grace et al., 2010a). On the contrary, postnatal MA exposure via breast milk induced increased anxiety behavior in adult rats while prenatal MA induced no behavior effects (Hruba et al., 2012). Little is known about developmental effects of MA on depression-associated behavior, although juvenile exposure increases adult depressive behavior in mice (Joca et al., 2014). Together these findings indicate that developmental effects on glucocorticoids and stress-related behaviors are subtle and highly dependent on species examined, dose, and timing of treatment.
Numerous studies indicate CRF regulates stress-related behaviors. Administration of CRF and genetic overexpression of CRF increase anxiety-related behaviors in rodents (Stenzel-Poore et al., 1994; Spiga et al., 2006). Conversely, administration of CRF antagonists has opposite effects, decreasing anxiety behaviors (Rassnick et al., 1993). CRF and CRF receptors (CRF-R1/CRF-R2) have also been implicated in MA abuse. CRF mRNA is elevated in the nucleus accumbens following injection with MA (Martin et al., 2012). Furthermore, exposure to a high dose of MA (10 mg/kg) enhances mRNA levels of CRF to a subsequent lesser dose of MA (2.5 mg/kg) in the rat nucleus accumbens (Cadet et al., 2014). This indicates that prior exposure to MA can induce greater elevations in CRF. Interestingly, rats that had previously self-administered MA showed elevated levels of CRF in the amygdala and plasma at 10 days of MA withdrawal (Nawata et al., 2012). These elevations in CRF were associated with increased anxiety behaviors and stress-induced reinstatement of MA-seeking (Nawata et al., 2012). The non-selective CRF receptor antagonist (α-helical CRF9–41) decreases MA-seeking behavior induced by stress (Nawata et al., 2012). Furthermore, selective CRF-R1 antagonists attenuate both cue-and MA-induced reinstatement of MA-seeking (Moffett and Goeders, 2007). These studies indicate that CRF antagonists may be effective treatments for decreasing symptoms such as anxiety, depression, and craving that are associated with MA withdrawal. Recent evidence also indicates AVP as a potential target for MA effects. Similar to CRF, prior exposure to MA can elevate subsequent MA-induced AVP mRNA in the rat nucleus accumbens (Cadet et al., 2014). Another study indicates that antagonism of an AVP receptor subtype (AVPR1b) contributes to MA-induced conditioned place preference (Subiah et al., 2012). Developmental studies indicate AVP is particularly vulnerable to MA. Exposure during neonatal and adolescent periods causes lasting reductions in AVP-expressing cells in the PVN (Zuloaga et al., 2013; Joca et al., 2014).
Other HPA axis associated proteins GR and MR are also affected by MA administration. MA exposure decreases GR, and to a lesser extent MR, in the hippocampus and hypothalamus of rodents (Lowy, 1990; Lowy and Novotney, 1994; Kabbaj et al., 2003). Within the PVN and central amygdala (CEA), there is also a robust activation of glucocorticoid receptor (GR) positive cells, identified by a significant increase in c-Fos/GR dual-labeled cells, following MA treatment. This indicates MA targets this cell phenotype in the brain and as a result may exert lasting effects on the function of these neurons. Studies involving pharmacological blockade of GR indicate that activation of GR is involved in increasing locomotor activity following MA treatment (Ago et al., 2009), although its role in other MA processes such as MA-seeking and reinstatement is unknown. See Table 1 for a summary of main findings regarding MA effects on glucocorticoids, HPA axis associated genes/proteins, and stress-related behaviors.
Table 1. MA effects on glucocorticoids, HPA axis associated genes/proteins, and stress-associated behaviors.
Blockade of MA-induced glucocorticoid synthesis using metyrapone has been utilized to study the effects of glucocorticoids on MA-associated behaviors, particularly in the context of stress and cued reinstatement of MA use. Metyrapone administration failed to block stress induced MA seeking behavior in mice previously exposed to MA (Nawata et al., 2012). However, a combined treatment of metyrapone with the benzodiazepine oxazepam attenuated MA seeking induced by the presentation of a conditioned reinforcer (Keller et al., 2013), indicating at least some involvement of glucocorticoids in this response. In cocaine models, metyrapone reduces cocaine-induced locomotion and relapse of cocaine self-administration (Piazza et al., 1994; Marinelli et al., 1997). Little is known about corticosterone synthesis blockade on many MA-induced behaviors as it has largely been studied in the context of withdrawal.
Psychostimulant effects on the HPA axis and the anti-reward pathway of addiction are commonly associated with extended amygdala circuitry (Koob and Volkow, 2010). However, studies utilizing immediate early genes as markers of neural activation have been useful in identifying other candidate regions involved in MA regulation of the HPA axis. HPA axis associated brain areas including hypothalamic, hippocampal, cortical, and extended amygdala sub-regions show extensive immediate early gene responses following MA administration (Giardino et al., 2011; Tomita et al., 2013; Zuloaga et al., 2014). Even a relatively low dose of MA (1 mg/kg) induces a 4–5 fold increase in the number of c-Fos positive cells within the PVN and CEA, two regions central to regulation of the HPA axis. Less is known about potential alterations in neural activation patterns in HPA axis-associated brain regions following chronic MA exposure. The majority of studies involving repeated psychostimulant exposure on immediate early gene responses have focused on the neostriatum and nucleus accumbens (McCoy et al., 2011). In these regions, repeated MA blunts neural activation (McCoy et al., 2011). Another study involving MA self-administration reported that repeated MA decreases c-Fos in the CEA and BNST (Cornish et al., 2012). This finding indicates that neural activation patterns in HPA axis associated brain regions are affected by chronic MA exposure. Further understanding of the effects of repeated MA use on neural activation within these and other brain areas that regulate the HPA axis may reveal specific brain circuits associated with MA addiction.
MA activation of the HPA axis is sex- dependent in neonatal and adult mice. In postnatal day 20 mice, MA induces a significant increase in corticosterone in females but not males (Acevedo et al., 2008). In adult mice, a 1 mg/kg dose of MA induces a protracted elevation in corticosterone in females compared to males (Zuloaga et al., 2014). This sex difference may be related to HPA axis negative feedback. Male mice display greater numbers of c-Fos positive and dual-labeled c-Fos/GR positive cells in two regions that regulate HPA axis negative feedback (CA3 and cingulate cortex) following the same dose of MA. Given the role of GR in regulating negative feedback (Sapolsky et al., 1985), this indicates a potential mechanism through which males confer a more rapid shutdown of the HPA axis than females. Potential sex differences in the effects of repeated MA exposure on HPA axis function are currently unknown but are likely critical in understanding sex differences in addiction associated behaviors reported in rodents and humans. These include greater MA seeking and reinstatement to MA use following a period of withdrawal in female rodents (Roth and Carroll, 2004; Reichel et al., 2012). In humans, there are numerous sex differences in MA abuse; women begin using at an earlier age than men, have a shorter duration from first use to regular use, and are more dependent on and committed to MA (Brecht et al., 2004; Dluzen and Liu, 2008; SAMHSA, 2010). Sex differences in MA-induced alterations in the HPA axis may contribute to these sexually dimorphic abuse patterns. Of particular interest is the role of gonadal steroid hormones estrogen, progesterone, and testosterone in regulating these effects. Extensive literature indicates that estrogens enhance, whereas androgens suppress, glucocorticoid release via activation of estrogen and androgen receptor subtypes (Zuloaga et al., 2008, 2011; Handa and Weiser, 2014). These same hormones regulate behavioral effects of psychostimulants such as cocaine self-administration, reinstatement, and conditioned place preference (Cooper et al., 2013; Ramoa et al., 2013; Bobzean et al., 2014). A more comprehensive understanding of the interactions between psychostimulant abuse processes, stress hormones, and gonadal steroid hormones will provide insight into the underlying causes of addiction.
MA is a potent activator of the HPA axis during both development and adulthood. Prolonged release in plasma corticosterone may contribute to a variety of effects reported after MA administration. In particular, the altered expression of genes/proteins associated with the HPA axis including CRF, AVP, and GR which have been reported in a variety of HPA axis associated brain regions. These neural effects of MA have been linked to both elevated anxiety/despair states as well as increased stress-induced reinstatement to MA use. MA addiction has proven incredibly difficult to treat, particularly in terms of psychotherapeutic interventions (Carson and Taylor, 2014). Compounds that alleviate HPA axis dysfunction such as metyrapone and drugs that interact with receptors for CRF, AVP, and glucocorticoids offer alternative mechanisms of treatment. At present, only metyrapone has been assessed in the context of human MA use although pre-clinical studies on other HPA axis-associated compounds are emerging and may yield clinical trials. For these compounds, it is essential to consider sex-specific effects since both MA abuse patterns and HPA axis activation differ between the sexes.
The authors declare that the research was conducted in the absence of any commercial or financial relationships that could be construed as a potential conflict of interest.
Abar, B., LaGasse, L. L., Derauf, C., Newman, E., Shah, R., Smith, L. M., et al. (2013). Examining the relationships between prenatal methamphetamine exposure, early adversity, and child neurobehavioral disinhibition. Psychol. Addict. Behav. 27, 662–673. doi: 10.1037/a0030157
Acevedo, S. F., de Esch, I. J., and Raber, J. (2007). Sex- and histamine-dependent long-term cognitive effects of methamphetamine exposure. Neuropsychopharmacology 32, 665–672. doi: 10.1038/sj.npp.1301091
Acevedo, S. F., Pfankuch, T., van Meer, P., and Raber, J. (2008). Role of histamine in short- and long-term effects of methamphetamine on the developing mouse brain. J. Neurochem. 107, 976–986. doi: 10.1111/j.1471-4159.2008.05673.x
Ago, Y., Arikawa, S., Yata, M., Yano, K., Abe, M., Takuma, K., et al. (2009). Role of prefrontal dopaminergic neurotransmission in glucocorticoid receptor-mediated modulation of methamphetamine-induced hyperactivity. Synapse 63, 7–14. doi: 10.1002/syn.20575
Bobzean, S. A., Dennis, T. S., and Perrotti, L. I. (2014). Acute estradiol treatment affects the expression of cocaine-induced conditioned place preference in ovariectomized female rats. Brain Res. Bull. 103, 49–53. doi: 10.1016/j.brainresbull.2014.02.002
Brecht, M. L., and Herbeck, D. (2014). Time to relapse following treatment for methamphetamine use: a long-term perspective on patterns and predictors. Drug Alcohol Depend. 139, 18–25. doi: 10.1016/j.drugalcdep.2014.02.702
Brecht, M. L., O'Brien, A., von Mayrhauser, C., and Anglin, M. D. (2004). Methamphetamine use behaviors and gender differences. Addict. Behav. 29, 89–106. doi: 10.1016/S0306-4603(03)00082-0
Cabrera, T. M., Levy, A. D., Li, Q., van de Kar, L. D., and Battaglia, G. (1993). Prenatal methamphetamine attenuates serotonin mediated renin secretion in male and female rat progeny: evidence for selective long-term dysfunction of serotonin pathways in brain. Synapse 15, 198–208. doi: 10.1002/syn.890150305
Cadet, J. L., Brannock, C., Ladenheim, B., McCoy, M. T., Krasnova, I. N., Lehrmann, E., et al. (2014). Enhanced upregulation of CRH mRNA expression in the nucleus accumbens of male rats after a second injection of methamphetamine given thirty days later. PLoS ONE 9:e84665. doi: 10.1371/journal.pone.0084665
Carroll, B. J., Martin, F. I., and Davies, B. (1968). Resistance to suppression by dexamethasone of plasma 11-O.H.C.S. levels in severe depressive illness. Br. Med. J. 3, 285–287. doi: 10.1136/bmj.3.5613.285
Carson, D. S., Bosanquet, D. P., Carter, C. S., Pournajafi-Nazarloo, H., Blaszczynski, A., and McGregor, I. S. (2012). Preliminary evidence for lowered basal cortisol in a naturalistic sample of methamphetamine polydrug users. Exp. Clin. Psychopharmacol. 20, 497–503. doi: 10.1037/a0029976
Carson, D. S., and Taylor, E. R. (2014). Commentary on Heinzerling et al. (2014): a growing methamphetamine dependence therapeutics graveyard. Addiction 109, 1887–1888. doi: 10.1111/add.12709
Cooper, Z. D., Foltin, R. W., and Evans, S. M. (2013). Effects of menstrual cycle phase on cocaine self-administration in rhesus macaques. Horm. Behav. 63, 105–113. doi: 10.1016/j.yhbeh.2012.10.008
Cornish, J. L., Hunt, G. E., Robins, L., and McGregor, I. S. (2012). Regional c-Fos and FosB/DeltaFosB expression associated with chronic methamphetamine self-administration and methamphetamine-seeking behavior in rats. Neuroscience 206, 100–114. doi: 10.1016/j.neuroscience.2012.01.004
Cruickshank, C. C., and Dyer, K. R. (2009). A review of the clinical pharmacology of methamphetamine. Addiction 104, 1085–1099. doi: 10.1111/j.1360-0443.2009.02564.x
Dluzen, D. E., and Liu, B. (2008). Gender differences in methamphetamine use and responses: a review. Gend. Med. 5, 24–35. doi: 10.1016/S1550-8579(08)80005-8
Eastwood, E., Allen, C. N., and Raber, J. (2012). Effects of neonatal methamphetamine and thioperamide exposure on spatial memory retention and circadian activity later in life. Behav. Brain Res. 230, 229–236. doi: 10.1016/j.bbr.2012.02.003
Giardino, W. J., Pastor, R., Anacker, A. M., Spangler, E., Cote, D. M., Li, J., et al. (2011). Dissection of corticotropin-releasing factor system involvement in locomotor sensitivity to methamphetamine. Genes Brain Behav. 10, 78–89. doi: 10.1111/j.1601-183X.2010.00641.x
Grace, C. E., Schaefer, T. L., Graham, D. L., Skelton, M. R., Williams, M. T., and Vorhees, C. V. (2010a). Effects of inhibiting neonatal methamphetamine-induced corticosterone release in rats by adrenal autotransplantation on later learning, memory, and plasma corticosterone levels. Int. J. Dev. Neurosci. 28, 331–342. doi: 10.1016/j.ijdevneu.2010.02.005
Grace, C. E., Schaefer, T. L., Herring, N. R., Graham, D. L., Skelton, M. R., Gudelsky, G. A., et al. (2010b). Effect of a neurotoxic dose regimen of (+)-methamphetamine on behavior, plasma corticosterone, and brain monoamines in adult C57BL/6 mice. Neurotoxicol. Teratol. 32, 346–355. doi: 10.1016/j.ntt.2010.01.006
Grace, C. E., Schaefer, T. L., Herring, N. R., Skelton, M. R., McCrea, A. E., Vorhees, C. V., et al. (2008). (+)-Methamphetamine increases corticosterone in plasma and BDNF in brain more than forced swim or isolation in neonatal rats. Synapse 62, 110–121. doi: 10.1002/syn.20470
Grace, C. E., Schaefer, T. L., Herring, N. R., Williams, M. T., and Vorhees, C. V. (2012). Effects of neonatal methamphetamine treatment on adult stress-induced corticosterone release in rats. Neurotoxicol. Teratol. 34, 136–142. doi: 10.1016/j.ntt.2011.08.004
Haass-Koffler, C. L., Leggio, L., and Kenna, G. A. (2014). Pharmacological approaches to reducing craving in patients with alcohol use disorders. CNS Drugs 28, 343–360. doi: 10.1007/s40263-014-0149-3
Handa, R. J., and Weiser, M. J. (2014). Gonadal steroid hormones and the hypothalamo-pituitary-adrenal axis. Front. Neuroendocrinol. 35, 197–220. doi: 10.1016/j.yfrne.2013.11.001
Harris, D. S., Reus, V. I., Wolkowitz, O. M., Mendelson, J. E., and Jones, R. T. (2003). Altering cortisol level does not change the pleasurable effects of methamphetamine in humans. Neuropsychopharmacology 28, 1677–1684. doi: 10.1038/sj.npp.1300223
Herman, J. P., Patel, P. D., Akil, H., and Watson, S. J. (1989). Localization and regulation of glucocorticoid and mineralocorticoid receptor messenger RNAs in the hippocampal formation of the rat. Mol. Endocrinol. 3, 1886–1894. doi: 10.1210/mend-3-11-1886
Herring, N. R., Schaefer, T. L., Gudelsky, G. A., Vorhees, C. V., and Williams, M. T. (2008a). Effect of +-methamphetamine on path integration learning, novel object recognition, and neurotoxicity in rats. Psychopharmacology (Berl) 199, 637–650. doi: 10.1007/s00213-008-1183-y
Herring, N. R., Schaefer, T. L., Tang, P. H., Skelton, M. R., Lucot, J. P., Gudelsky, G. A., et al. (2008b). Comparison of time-dependent effects of (+)-methamphetamine or forced swim on monoamines, corticosterone, glucose, creatine, and creatinine in rats. BMC Neurosci. 9:49. doi: 10.1186/1471-2202-9-49
Hruba, L., Schutova, B., and Slamberova, R. (2012). Sex differences in anxiety-like behavior and locomotor activity following prenatal and postnatal methamphetamine exposure in adult rats. Physiol. Behav. 105, 364–370. doi: 10.1016/j.physbeh.2011.08.016
Imaki, T., Nahan, J. L., Rivier, C., Sawchenko, P. E., and Vale, W. (1991). Differential regulation of corticotropin-releasing factor mRNA in rat brain regions by glucocorticoids and stress. J. Neurosci. 11, 585–599.
Jang, C. G., Whitfield, T., Schulteis, G., Koob, G. F., and Wee, S. (2013). A dysphoric-like state during early withdrawal from extended access to methamphetamine self-administration in rats. Psychopharmacology (Berl) 225, 753–763. doi: 10.1007/s00213-012-2864-0
Joca, L., Zuloaga, D. G., Raber, J., and Siegel, J. A. (2014). Long-term effects of early adolescent methamphetamine exposure on depression-like behavior and the hypothalamic vasopressin system in mice. Dev. Neurosci. 36, 108–118. doi: 10.1159/000360001
Kabbaj, M., Yoshida, S., Numachi, Y., Matsuoka, H., Devine, D. P., and Sato, M. (2003). Methamphetamine differentially regulates hippocampal glucocorticoid and mineralocorticoid receptor mRNAs in Fischer and Lewis rats. Brain Res. Mol. Brain Res. 117, 8–14. doi: 10.1016/S0169-328X(03)00257-2
Keller, C. M., Cornett, E. M., Guerin, G. F., and Goeders, N. E. (2013). Combinations of oxazepam and metyrapone attenuate cocaine and methamphetamine cue reactivity. Drug Alcohol Depend. 133, 405–412. doi: 10.1016/j.drugalcdep.2013.06.025
Kiblawi, Z. N., Smith, L. M., Diaz, S. D., LaGasse, L. L., Derauf, C., Newman, E., et al. (2014). Prenatal methamphetamine exposure and neonatal and infant neurobehavioral outcome: results from the IDEAL study. Subst. Abus. 35, 68–73. doi: 10.1080/08897077.2013.814614
Kim, H., Yi, J. H., Choi, K., Hong, S., Shin, K. S., and Kang, S. J. (2014). Regional differences in acute corticosterone-induced dendritic remodeling in the rat brain and their behavioral consequences. BMC Neurosci. 15:65. doi: 10.1186/1471-2202-15-65
Kim, J. B., Ju, J. Y., Kim, J. H., Kim, T. Y., Yang, B. H., Lee, Y. S., et al. (2004). Dexamethasone inhibits proliferation of adult hippocampal neurogenesis in vivo and in vitro. Brain Res. 1027, 1–10. doi: 10.1016/j.brainres.2004.07.093
Kirlic, N., Newman, E., Lagasse, L. L., Derauf, C., Shah, R., Smith, L. M., et al. (2013). Cortisol reactivity in two-year-old children prenatally exposed to methamphetamine. J. Stud. Alcohol Drugs 74, 447–451. doi: 10.15288/jsad.2013.74.447
Koob, G. F., Buck, C. L., Cohen, A., Edwards, S., Park, P. E., Schlosburg, J. E., et al. (2014). Addiction as a stress surfeit disorder. Neuropharmacology 76(Pt B), 370–382. doi: 10.1016/j.neuropharm.2013.05.024
Koob, G. F., and Volkow, N. D. (2010). Neurocircuitry of addiction. Neuropsychopharmacology 35, 217–238. doi: 10.1038/npp.2009.110
LaGasse, L. L., Derauf, C., Smith, L. M., Newman, E., Shah, R., Neal, C., et al. (2012). Prenatal methamphetamine exposure and childhood behavior problems at 3 and 5 years of age. Pediatrics 129, 681–688. doi: 10.1542/peds.2011-2209
Landgraf, R., Wigger, A., Holsboer, F., and Neumann, I. D. (1999). Hyper-reactive hypothalamo-pituitary-adrenocortical axis in rats bred for high anxiety-related behaviour. J. Neuroendocrinol. 11, 405–407. doi: 10.1046/j.1365-2826.1999.00342.x
Li, S. X., Yan, S. Y., Bao, Y. P., Lian, Z., Qu, Z., Wu, Y. P., et al. (2013). Depression and alterations in hypothalamic-pituitary-adrenal and hypothalamic-pituitary-thyroid axis function in male abstinent methamphetamine abusers. Hum. Psychopharmacol. 28, 477–483. doi: 10.1002/hup.2335
London, E. D., Simon, S. L., Berman, S. M., Mandelkern, M. A., Lichtman, A. M., Bramen, J., et al. (2004). Mood disturbances and regional cerebral metabolic abnormalities in recently abstinent methamphetamine abusers. Arch. Gen. Psychiatry 61, 73–84. doi: 10.1001/archpsyc.61.1.73
Lowy, M. T. (1990). MK-801 antagonizes methamphetamine-induced decreases in hippocampal and striatal corticosteroid receptors. Brain Res. 533, 348–352. doi: 10.1016/0006-8993(90)91362-K
Lowy, M. T., and Novotney, S. (1994). Methamphetamine-induced decrease in neural glucocorticoid receptors: relationship to monoamine levels. Brain Res. 638, 175–181. doi: 10.1016/0006-8993(94)90647-5
Madden, L. J., Flynn, C. T., Zandonatti, M. A., May, M., Parsons, L. H., Katner, S. N., et al. (2005). Modeling human methamphetamine exposure in nonhuman primates: chronic dosing in the rhesus macaque leads to behavioral and physiological abnormalities. Neuropsychopharmacology 30, 350–359. doi: 10.1038/sj.npp.1300575
Mantsch, J. R., Taves, S., Khan, T., Katz, E. S., Sajan, T., Tang, L. C., et al. (2007). Restraint-induced corticosterone secretion and hypothalamic CRH mRNA expression are augmented during acute withdrawal from chronic cocaine administration. Neurosci. Lett. 415, 269–273. doi: 10.1016/j.neulet.2007.01.036
Marinelli, M., Rouge-Pont, F., De Jesus-Oliveira, C., Le Moal, M., and Piazza, P. V. (1997). Acute blockade of corticosterone secretion decreases the psychomotor stimulant effects of cocaine. Neuropsychopharmacology 16, 156–161. doi: 10.1016/S0893-133X(96)00169-8
Martin, T. A., Jayanthi, S., McCoy, M. T., Brannock, C., Ladenheim, B., Garrett, T., et al. (2012). Methamphetamine causes differential alterations in gene expression and patterns of histone acetylation/hypoacetylation in the rat nucleus accumbens. PLoS ONE 7:e34236. doi: 10.1371/journal.pone.0034236
McCoy, M. T., Jayanthi, S., Wulu, J. A., Beauvais, G., Ladenheim, B., Martin, T. A., et al. (2011). Chronic methamphetamine exposure suppresses the striatal expression of members of multiple families of immediate early genes (IEGs) in the rat: normalization by an acute methamphetamine injection. Psychopharmacology (Berl) 215, 353–365. doi: 10.1007/s00213-010-2146-7
Moffett, M. C., and Goeders, N. E. (2007). CP-154,526, a CRF type-1 receptor antagonist, attenuates the cue-and methamphetamine-induced reinstatement of extinguished methamphetamine-seeking behavior in rats. Psychopharmacology (Berl) 190, 171–180. doi: 10.1007/s00213-006-0625-7
Morimasa, T., Wirz-Justice, A., Kraeuchi, K., Arendt, J., Baumann, J., Haeusler, A., et al. (1987). Chronic methamphetamine and its withdrawal modify behavioral and neuroendocrine circadian rhythms. Physiol. Behav. 39, 699–705. doi: 10.1016/0031-9384(87)90253-8
Nawata, Y., Kitaichi, K., and Yamamoto, T. (2012). Increases of CRF in the amygdala are responsible for reinstatement of methamphetamine-seeking behavior induced by footshock. Pharmacol. Biochem. Behav. 101, 297–302. doi: 10.1016/j.pbb.2012.01.003
Nicosia, N., Pacula, R. L., Kilmer, B., Lundberg, R., and Chiesa, J. (2009). The Economic Cost of Methamphetamine Use in the United States, 2005, 169. Available online at: http://www.rand.org/pubs/monographs/MG829/
Piazza, P. V., Marinelli, M., Jodogne, C., Deroche, V., Rouge-Pont, F., Maccari, S., et al. (1994). Inhibition of corticosterone synthesis by Metyrapone decreases cocaine-induced locomotion and relapse of cocaine self-administration. Brain Res. 658, 259–264. doi: 10.1016/S0006-8993(09)90034-8
Ramoa, C. P., Doyle, S. E., Naim, D. W., and Lynch, W. J. (2013). Estradiol as a mechanism for sex differences in the development of an addicted phenotype following extended access cocaine self-administration. Neuropsychopharmacology 38, 1698–1705. doi: 10.1038/npp.2013.68
Rassnick, S., Heinrichs, S. C., Britton, K. T., and Koob, G. F. (1993). Microinjection of a corticotropin-releasing factor antagonist into the central nucleus of the amygdala reverses anxiogenic-like effects of ethanol withdrawal. Brain Res. 605, 25–32. doi: 10.1016/0006-8993(93)91352-S
Reichel, C. M., Chan, C. H., Ghee, S. M., and See, R. E. (2012). Sex differences in escalation of methamphetamine self-administration: cognitive and motivational consequences in rats. Psychopharmacology (Berl) 223, 371–380. doi: 10.1007/s00213-012-2727-8
Reul, J. M., van den Bosch, F. R., and de Kloet, E. R. (1987). Differential response of type I and type II corticosteroid receptors to changes in plasma steroid level and circadian rhythmicity. Neuroendocrinology 45, 407–412. doi: 10.1159/000124766
Roth, M. E., and Carroll, M. E. (2004). Sex differences in the acquisition of IV methamphetamine self-administration and subsequent maintenance under a progressive ratio schedule in rats. Psychopharmacology (Berl) 172, 443–449. doi: 10.1007/s00213-003-1670-0
Substance Abuse Mental Health Services Administration (SAMHSA). (2010). Gender Differences among American Indian Treatment Admissions Aged 18 to 25. The TEDS Report, Rockville, MD.
Substance Abuse Mental Health Services Administration (SAMHSA). (2012). “Results from the 2011 National Survey on Drug Use and Health: Summary of National Findings,” in NSDUH Series H-44, HHS Publication No. (SMA) 12-4713. Rockville, MD: Substance Abuse and Mental Health Services Administration.
Sapolsky, R. M., Meaney, M. J., and McEwen, B. S. (1985). The development of the glucocorticoid receptor system in the rat limbic brain. III. Negative-feedback regulation. Brain Res. 350, 169–173. doi: 10.1016/0165-3806(85)90261-5
Sawchenko, P. E., Arias, C. A., and Mortrud, M. T. (1993). Local tetrodotoxin blocks chronic stress effects on corticotropin-releasing factor and vasopressin messenger ribonucleic acids in hypophysiotropic neurons. J. Neuroendocrinol. 5, 341–348. doi: 10.1111/j.1365-2826.1993.tb00493.x
Schaefer, T. L., Skelton, M. R., Herring, N. R., Gudelsky, G. A., Vorhees, C. V., and Williams, M. T. (2008). Short- and long-term effects of (+)-methamphetamine and (±)-3,4-methylenedioxymethamphetamine on monoamine and corticosterone levels in the neonatal rat following multiple days of treatment. J. Neurochem. 104, 1674–1685. doi: 10.1111/j.1471-4159.2007.05112.x
Siegel, J. A., Park, B. S., and Raber, J. (2011). Long-term effects of neonatal methamphetamine exposure on cognitive function in adolescent mice. Behav. Brain Res. 219, 159–164. doi: 10.1016/j.bbr.2011.01.015
Silva, C. D., Neves, A. F., Dias, A. I., Freitas, H. J., Mendes, S. M., Pita, I., et al. (2014). A single neurotoxic dose of methamphetamine induces a long-lasting depressive-like behaviour in mice. Neurotox. Res. 25, 295–304. doi: 10.1007/s12640-013-9423-2
Smith, L. M., Paz, M. S., LaGasse, L. L., Derauf, C., Newman, E., Shah, R., et al. (2012). Maternal depression and prenatal exposure to methamphetamine: neurodevelopmental findings from the infant development, environment, and lifestyle (ideal) study. Depress. Anxiety 29, 515–522. doi: 10.1002/da.21956
Spiga, F., Lightman, S. L., Shekhar, A., and Lowry, C. A. (2006). Injections of urocortin 1 into the basolateral amygdala induce anxiety-like behavior and c-Fos expression in brainstem serotonergic neurons. Neuroscience 138, 1265–1276. doi: 10.1016/j.neuroscience.2005.12.051
Stenzel-Poore, M. P., Heinrichs, S. C., Rivest, S., Koob, G. F., and Vale, W. W. (1994). Overproduction of corticotropin-releasing factor in transgenic mice: a genetic model of anxiogenic behavior. J. Neurosci. 14, 2579–2584.
Subiah, C. O., Mabandla, M. V., Phulukdaree, A., Chuturgoon, A. A., and Daniels, W. M. (2012). The effects of vasopressin and oxytocin on methamphetamine-induced place preference behaviour in rats. Metab. Brain Dis. 27, 341–350. doi: 10.1007/s11011-012-9297-7
Tomita, M., Katsuyama, H., Watanabe, Y., Shibaike, Y., Yoshinari, H., Tee, J. W., et al. (2013). c-Fos immunoreactivity of neural cells in intoxication due to high-dose methamphetamine. J. Toxicol. Sci. 38, 671–678. doi: 10.2131/jts.38.671
Vorhees, C. V., Skelton, M. R., Grace, C. E., Schaefer, T. L., Graham, D. L., Braun, A. A., et al. (2009). Effects of (+)-methamphetamine on path integration and spatial learning, but not locomotor activity or acoustic startle, align with the stress hyporesponsive period in rats. Int. J. Dev. Neurosci. 27, 289–298. doi: 10.1016/j.ijdevneu.2008.12.003
Williams, M. T., Inman-Wood, S. L., Morford, L. L., McCrea, A. E., Ruttle, A. M., Moran, M. S., et al. (2000). Preweaning treatment with methamphetamine induces increases in both corticosterone and ACTH in rats. Neurotoxicol. Teratol. 22, 751–759. doi: 10.1016/S0892-0362(00)00091-X
Williams, M. T., Moran, M. S., and Vorhees, C. V. (2003). Refining the critical period for methamphetamine-induced spatial deficits in the Morris water maze. Psychopharmacology (Berl) 168, 329–338. doi: 10.1007/s00213-003-1433-y
Workman, A. D., Charvet, C. J., Clancy, B., Darlington, R. B., and Finlay, B. L. (2013). Modeling transformations of neurodevelopmental sequences across mammalian species. J. Neurosci. 33, 7368–7383. doi: 10.1523/JNEUROSCI.5746-12.2013
Zuloaga, D. G., Johnson, L. A., Agam, M., and Raber, J. (2014). Sex differences in activation of the hypothalamic-pituitary-adrenal axis by methamphetamine. J. Neurochem. 129, 495–508. doi: 10.1111/jnc.12651
Zuloaga, D. G., Morris, J. A., Jordan, C. L., and Breedlove, S. M. (2008). Mice with the testicular feminization mutation demonstrate a role for androgen receptors in the regulation of anxiety-related behaviors and the hypothalamic-pituitary-adrenal axis. Horm. Behav. 54, 758–766. doi: 10.1016/j.yhbeh.2008.08.004
Zuloaga, D. G., Poort, J. E., Jordan, C. L., and Breedlove, S. M. (2011). Male rats with the testicular feminization mutation of the androgen receptor display elevated anxiety-related behavior and corticosterone response to mild stress. Horm. Behav. 60, 380–388. doi: 10.1016/j.yhbeh.2011.07.008
Keywords: methamphetamine, stress, anxiety, substance abuse, glucocorticoids, HPA axis
Citation: Zuloaga DG, Jacobskind JS and Raber J (2015) Methamphetamine and the hypothalamic-pituitary-adrenal axis. Front. Neurosci. 9:178. doi: 10.3389/fnins.2015.00178
Received: 13 March 2015; Accepted: 03 May 2015;
Published: 27 May 2015.
Edited by:
Ashok Kumar, University of Florida, USAReviewed by:
Karthik Bodhinathan, Sanford Burnham Medical Research Institute, USACopyright © 2015 Zuloaga, Jacobskind and Raber. This is an open-access article distributed under the terms of the Creative Commons Attribution License (CC BY). The use, distribution or reproduction in other forums is permitted, provided the original author(s) or licensor are credited and that the original publication in this journal is cited, in accordance with accepted academic practice. No use, distribution or reproduction is permitted which does not comply with these terms.
*Correspondence: Damian G. Zuloaga, Department of Psychology, University at Albany, 1400 Washington Ave., Albany, NY 12222, USA,ZHp1bG9hZ2FAYWxiYW55LmVkdQ==
Disclaimer: All claims expressed in this article are solely those of the authors and do not necessarily represent those of their affiliated organizations, or those of the publisher, the editors and the reviewers. Any product that may be evaluated in this article or claim that may be made by its manufacturer is not guaranteed or endorsed by the publisher.
Research integrity at Frontiers
Learn more about the work of our research integrity team to safeguard the quality of each article we publish.