- Department of Human Kinetics, St. Francis Xavier University, Antigonish, NS, Canada
Lactate, the conjugate base of lactic acid occurring in aqueous biological fluids, has been derided as a “dead-end” waste product of anaerobic metabolism. Catalyzed by the near-equilibrium enzyme lactate dehydrogenase (LDH), the reduction of pyruvate to lactate is thought to serve to regenerate the NAD+ necessary for continued glycolytic flux. Reaction kinetics for LDH imply that lactate oxidation is rarely favored in the tissues of its own production. However, a substantial body of research directly contradicts any notion that LDH invariably operates unidirectionally in vivo. In the current Perspective, a model is forwarded in which the continuous formation and oxidation of lactate serves as a mitochondrial electron shuttle, whereby lactate generated in the cytosol of the cell is oxidized at the mitochondria of the same cell. From this perspective, an intracellular lactate shuttle operates much like the malate-aspartate shuttle (MAS); it is also proposed that the two shuttles are necessarily interconnected in a lactate-MAS. Among the requisite features of such a model, significant compartmentalization of LDH, much like the creatine kinase of the phosphocreatine shuttle, would facilitate net cellular lactate oxidation in a variety of cell types.
Introduction: Lactate Dehydrogenase Reaction
The reduction of pyruvate to lactate, catalyzed by lactate dehydrogenase (LDH; Pyruvate + NADH + H+ ⇌ Lactate + NAD+) in the cytosol of many cells, has been regarded as a metabolic “dead-end” (Luft, 2001; Quistorff and Grunnet, 2011a), or perhaps more aptly, a metabolic cul de sac (Barros, 2013), because lactate can only rejoin the metabolic network via pyruvate. In mammals, the LDH reaction is also considered to be “near-equilibrium” (Spriet et al., 2000; Quistorff and Grunnet, 2011a,b), meaning that the reaction is regulated chiefly by the concentrations of its reactants and products, rather than by more sophisticated means of allosteric regulation or covalent modification (Crabtree and Newsholme, 1978). Because the equilibrium for the LDH reaction lies far to the right (i.e., lactate formation favored) (Williamson et al., 1967), regardless of LDH isoform (Quistorff and Grunnet, 2011a,b), the implication might be that LDH rarely favors the reverse reaction (i.e., lactate oxidation) in vivo. Indeed, the mass action ratio ([lactate][NAD+]/[pyruvate][NADH][H+]) necessary for appreciable lactate oxidation would need to exceed the equilibrium constant for LDH. However, experimental evidence increasingly belies any notion that LDH operates unidirectionally in vivo, and supports that lactate serves as an important metabolic fuel for many tissues, including skeletal (Brooks et al., 1991; Bergman et al., 1999; Donovan and Pagliassotti, 2000) and cardiac muscle (Gertz et al., 1988; Chatham et al., 2001), liver (Skilleter and Kun, 1972; Kline et al., 1986), and brain (Schurr et al., 1988; Bouzier-Sore et al., 2006; Wyss et al., 2011; Funfschilling et al., 2012; reviewed in Barros, 2013). The purpose of the current Perspective is to forward a model in which lactate is central to the shuttling of energetic substrate between the cytosol (glycolysis) and the mitochondria (oxidative phosphorylation). Components of such a concept have been demonstrated in heart (Safer et al., 1971) and skeletal muscle (Schantz, 1986), were later expanded to a lactate shuttle perspective (Stainsby and Brooks, 1990; Brooks et al., 1999) and comprehensively reviewed (Gladden, 2004) and again commented upon (Gladden, 2007). The concept is particularly supported by recent research in neuronal cells (Gellerich et al., 2012, 2013; Rueda et al., 2014). While the concept outlined in the current Perspective is not new, per se (Safer et al., 1971), an apparent lack of conventional recognition or acceptance of its theoretical underpinnings, warrants further attention.
The Malate-Aspartate Shuttle
Due to the impermeability of the inner mitochondrial membrane to NAD+ and NADH, NADH generated by glycolysis under aerobic conditions depends on the indirect transfer of reducing equivalents into the mitochondria via the malate-aspartate shuttle (MAS) and glycerol-phosphate shuttle. These shuttles are also thought to regenerate cytosolic NAD+ necessary to support glycolytic flux at the NAD+-requiring glyceraldehyde 3-phosphate dehydrogenase reaction. The MAS has been demonstrated to be the predominant means by which this occurs in most oxidative tissues, and appears to constitute the principal NADH shuttle in mature neurons (Kauppinen et al., 1987; Ramos et al., 2003; Contreras and Satrustegui, 2009; Gellerich et al., 2012). It is also well established that during conditions of increased cellular energy demand and/or increased glycolytic flux (e.g., during strenuous exercise), as well as hypoxia, that the concentration of lactate will increase as the LDH reaction facilitates increased rates of cytosolic NAD+ regeneration (Robergs et al., 2004). In the brain, however, increasing the concentration of lactate in circulation (e.g., as during exercise) results in an increase in lactate disposal in the brain (Quistorff et al., 2008; van Hall et al., 2009; Boumezbeur et al., 2010; Dienel, 2012). It has also been suggested that the increased LDH activity (and, in turn, lactate production) simply compensates for the inability of the MAS to keep pace with cytosolic NAD+ demand (Schantz, 1986). In neurons, Ca+2 activation of the MAS and TCA cycle are competitive, such that lower levels of Ca+2 stimulates MAS activity by activating the glutamate/aspartate carrier (Contreras and Satrustegui, 2009), while higher concentrations of Ca+2 activate α-ketoglutarate dehydrogenase in the mitochondrial matrix, limiting the α-ketoglutarate available for the MAS (Contreras and Satrustegui, 2009). It is also possible that lactate is formed continuously in the cytosol, regardless of metabolic state, and that lactate oxidized at the mitochondria is coupled to the MAS. In isolated cardiac mitochondria, for example, the MAS exhibits an excess capacity, suggesting that the MAS activity alone is sufficient to maintain cytosolic NAD+ regeneration (Digerness and Reddy, 1976). Why, then, at rest and under fully aerobic conditions, would lactate be produced during glycolysis, if all the pyruvate should be going to the mitochondria for oxidative phosphorylation, and the MAS should be regenerating sufficient NAD+?
Conventional (An)aerobic Glycolysis
The appearance and disappearance of lactate during varying metabolic states has been a topic of much historical conjecture, controversy, and intrigue. There have been many reviews of the literature examining lactate metabolism, to which readers may be directed. Some of the more recent include (Cruz et al., 2012; Dienel, 2012; Kitaoka et al., 2012; Doherty and Cleveland, 2013; Newington et al., 2013; Brooks, 2014; Schurr, 2014; Todd, 2014). Unfortunately, many contemporary textbooks still use the metabolic fate of pyruvate to distinguish two types of glycolysis: aerobic (i.e., requiring oxygen) and anaerobic (i.e., without oxygen). In the presence of oxygen, it has been said, pyruvate will proceed to the mitochondria to meet its metabolic demise via oxidative phosphorylation, the net result of which is mitochondrial ATP resynthesis and oxygen consumption (i.e., respiration) (Voet et al., 2011). Conversely, when oxygen is limiting, the pyruvate is reduced to lactate in the cytosol by LDH, oxidizing its cofactor NADH in the process (Voet et al., 2011). A problem with this traditional construct is that it does not reconcile well with some recurring scientific observations. For example, it is well established that lactate is produced, and consumed, under fully aerobic conditions. Indeed, in healthy, normoxic individuals at rest in the postabsorptive state, it can be expected that approximately 50 μmol·min−1 of lactate are released from the brain alone (van Hall et al., 2009; van Hall, 2010). Clearly, lactate is more than a dead-end waste metabolite of anaerobic glycolysis; rather, shuttling of lactate throughout the organism provides useful perspective in which to interpret experimental observation.
The Lactate Shuttle Concept
Two lactate shuttle concepts have been forwarded which describe the movement and utilization of lactate within and between cells (Brooks, 1998). The intracellular lactate shuttle hypothesis posits that lactate formed during glycolysis can be continuously used as an energy source within the same cell (Brooks, 1998). The intercellular, or cell-cell lactate shuttle involves lactate generated and exported from a cell to be taken up and utilized by another cell (Brooks, 1998). The cell-cell lactate shuttle has gained general acceptance; the finer details of the intracellular lactate shuttle continue to be investigated, however. Recently, we demonstrated both a physical, as well as functional association of LDH with mitochondria in skeletal muscle (Elustondo et al., 2013). Using laser-scanning confocal microscopy, we confirmed the colocalization of LDH with mitochondrial membrane proteins in rat skeletal muscle. We found that mitochondria in saponin-permeabilized skeletal muscle fibers from rats oxidized lactate in the presence of NAD+, malate, and ADP (Elustondo et al., 2013); this was found similarly by another group in human fibers (Jacobs et al., 2013). The pyruvate was then transported into the mitochondria where it was further oxidized by pyruvate dehydrogenase (PDH), then the TCA cycle, with reducing equivalents stimulating respiration (Elustondo et al., 2013; Jacobs et al., 2013). We were able to inhibit respiration with just 5μM alpha-cyano-hydroxycinnamate, an inhibitor of mitochondrial pyruvate transport, further supporting that pyruvate, but not lactate is transported into the mitochondrial matrix. These findings support that LDH is strategically positioned to functionally interact with mitochondria, and suggest that lactate oxidation occurs near the outer surface of the inner mitochondrial membrane. How might an intracellular lactate shuttle operate in an intact cell? Let us return to the MAS.
In vivo, cytosolic NAD+ could, in theory, be regenerated by malate dehydrogenase outside of the mitochondrial matrix, as part of the MAS. The literature gives some insight into different tissues and their mitochondrial shuttling activities. In the brain, the MAS has been considered the most important shuttle system for getting cytosolic NADH into the mitochondria (McKenna et al., 2006, and references therein); conversely, the glycerol-phosphate shuttle appears to be of lesser importance (Nguyen et al., 2003). Indeed, the intimate association of the MAS and the biosynthesis of neurotransmitter glutamate has been reported (Palaiologos et al., 1988). The published activities of the MAS measured in mitochondria isolated from rat brain are in the order of 26.7 nmol·min−1·mg−1 (Pardo et al., 2006). In synaptosomes, pharmacological inhibition of the MAS results in a pronounced (i.e. 50%) decrease in lactate oxidation (McKenna et al., 1993), supporting the model illustrated in Figure 1. Intracerebral production of lactate from 13C labeled glucose further supports the notion that lactate is an important fuel for neurons (Sampol et al., 2013).
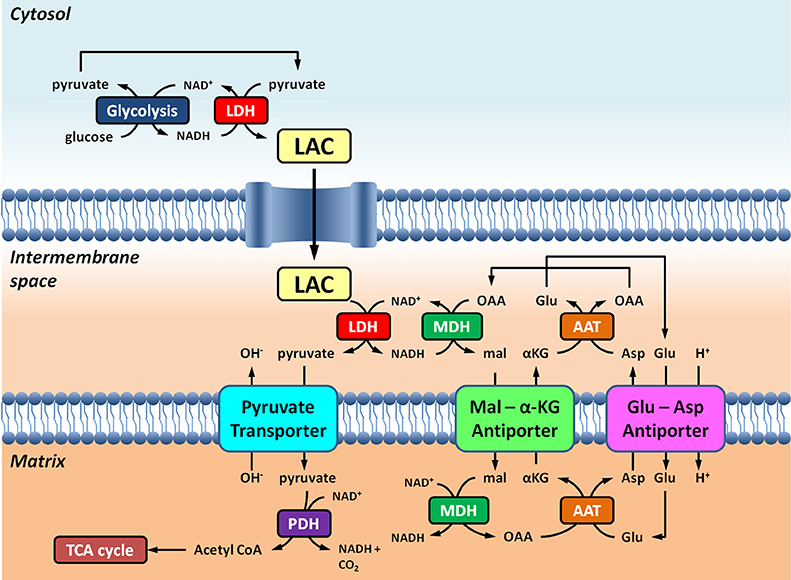
Figure 1. Schematic representation of the link between glycolysis and lactate oxidation at the mitochondria outlined in this Perspective. Overall, glycolysis yields pyruvate and NADH, in addition to ATP. NAD+ can be regenerated for glycolysis by the reduction of pyruvate to lactate (LAC) by lactate dehydrogenase (LDH). LAC can diffuse to the mitochondria where it is oxidized to pyruvate by LDH. NAD+ is regenerated by extra-matrix malate dehydrogenase (MDH) of the malate-aspartate shuttle. Pyruvate is subsequently transported across the inner mitochondrial membrane into the matrix, where it is then oxidized by pyruvate dehydrogenase (PDH) to acetyl CoA. Abbreviations: α-KG, alpha-ketoglutarate; Glu, glutamate; AAT, aspartate-aminotransferase; OAA, oxaloacetate; Mal, malate.
It should be noted that the MAS may have its limits. At high cardiac workloads, it has been shown that the α-ketoglutarate/malate transporter of the inner mitochondrial membrane cannot compete with matrix α-ketoglutarate dehydrogenase for their shared substrate, α-ketoglutarate (O'Donnell et al., 2004). This results in a limiting effect to the MAS and its shuttling of NADH into the mitochondria. The net effect of this limitation to the MAS would be a rise in cytosolic lactate concentration as NAD+ regeneration via the LDH reaction would help to preserve homeostatic NAD+/NADH, even in the presence of adequate oxygen. Indeed, this is the classic phenomenon observed during especially strenuous exercise where lactate can accumulate in the blood, despite adequate oxygen availability.
Intracellular Compartmentalization of LDH: Lessons from the Phosphocreatine Shuttle
The notion of shuttling compounds between the mitochondria and cytosol to meet the energetic demands of the cell using near-equilibrium enzymes is certainly not new. The phosphocreatine (PCr) shuttle involves distinct mitochondrial and cytosolic creatine kinase (CK) isoforms to essentially shuttle “high energy” phosphate from the mitochondria to the cytosol. Like the LDH reaction, the CK reaction (phosphocreatine + ADP + H+ ⇌ creatine + ATP), is considered to be “near-equilibrium,” favoring ATP resynthesis. However, experimental evidence demonstrates that in myocardial cells, only cytosolic CK is actually at, or near, equilibrium (Reviewed in Joubert et al., 2004). Mitochondrial CK, on the other hand, localized to the intermembrane space, is displaced from equilibrium, favoring net PCr resynthesis. By way of analogy, two distinct LDH populations are thought to be involved in the intracellular lactate shuttle: cytosolic and mitochondrial. The cytosolic LDH would be at or near equilibrium, whereas the mitochondrial LDH would be displaced from equilibrium. The cytosolic LDH would favor net lactate production, while the mitochondrial LDH would favor lactate oxidation. And much like the adenine nucleotide translocase (ANT), which transports ADP into the matrix across the inner mitochondrial membrane in exchange for ATP, facilitates the displacement from equilibrium for mitochondrial CK in the intermembrane space, so too would the pyruvate transporter continuously transport pyruvate, displacing the mitochondrial LDH reaction from equilibrium (Figure 1). Such a lactate shuttle would benefit from LDH localization in the intermembrane space near the inner mitochondrial membrane, bound to the outside of the outer mitochondrial membrane at contact sites of the outer and inner mitochondrial membrane, or both. If intracellular lactate oxidation is to occur at the mitochondria via compartmentalization, as with the PCr shuttle, the cellular localization of LDH in, at, or about the mitochondria would be a salient feature.
Intercellular Compartmentalization of Lactate Metabolism: the Astrocyte-Neuron Lactate Shuttle
A rich and growing body of neuroenergetic research also supports the existence of compartmentalized lactate metabolism among neighboring brain cells—namely, astrocytes and neurons (Reviewed in Pellerin and Magistretti, 2012). A variant of the intercellular lactate shuttle generally (Brooks, 2009), the astrocyte-neuron lactate shuttle (Pellerin and Magistretti, 2012) is based upon the idea that astrocytes are predominantly glycolytic, whereas neurons are more oxidative (Bouzier-Sore and Pellerin, 2013 and references therein). Using a metabolic modeling approach, it was argued that greater metabolic flux through PDH and the mitochondrial NADH shuttles in neurons compared to astrocytes necessarily dictates net lactate release by astrocytes and oxidation by neurons (Neves et al., 2012), supporting many experimental observations (reviewed in Bouzier-Sore and Pellerin, 2013). As mentioned previously, the MAS constitutes the major mitochondrial NADH shuttle in mature neurons (Kauppinen et al., 1987; Ramos et al., 2003; Contreras and Satrustegui, 2009; Gellerich et al., 2012). Hence, an important feature of the lactate-consuming neuron may well be its high MAS activity (Neves et al., 2012). Is it time for a lactate-malate-aspartate shuttle? Is there additional theoretical support for such a model in which lactate serves as a reducing equivalent?
Regeneration of Cytosolic NAD+
Lactate oxidation at the mitochondrion further makes sense of aerobic glycolysis by permitting cytosolic NAD+ regeneration by cytosolic LDH. Indeed, evidence in cultured cells points to a highly labile lactate/pyruvate ratio which varies to preserve homeostatic NAD+/NADH (Sun et al., 2012). This would be advantageous for the cell for a number of reasons. Firstly, it would provide an immediate means by which to regenerate NAD+ locally (i.e., in the cytosol, where glycolysis occurs); the greater relative diffusability of lactate (molecular weight = 89.07 g/mol) vs. NAD+ (molecular weight = 663.43 g/mol) means lactate can readily diffuse from the cell under conditions of increased glycolytic flux (e.g., intense exercise, hypoxic stress), while also being directed toward the mitochondria. During times of reduced cellular energy demand, continued lactate production during much lower rates of glycolytic flux would still be used to maintain homeostatic NAD+/NADH within the cell, as well as for continued coupling of intracellular lactate shuttling to the MAS.
Proton Shuttling and Mitochondrial Substrate Transport
Lactate oxidation at the mitochondria makes sense of aerobic glycolysis because lactate production in the cytosol effectively consumes a proton (Robergs et al., 2004), which is thought to help mitigate the metabolic acidosis associated with increased ATP turnover and high rates of glycolysis (Robergs et al., 2004). The cytosolic concentration of lactate typically exceeds that of pyruvate by at least 10-fold, meaning that lactate, and not pyruvate is the predominant monocarboxylate entering the mitochondria intermembrane space (Brooks et al., 1999). By oxidizing lactate in the mitochondrial intermembrane space, protons would be released where they could contribute to the ΔpH component of the mitochondrial proton motive force across the inner membrane (Santo-Domingo and Demaurex, 2012), and/or be transported indirectly into the mitochondria by the MAS. As with the transport of inorganic phosphate and some other substrates and ions (Santo-Domingo and Demaurex, 2012), carrier-mediated transport of pyruvate across the inner mitochondrial membrane in rat liver mitochondria appears to be directly coupled to proton symport (or OH− antiport) (Papa et al., 1971; Halestrap, 1975). Oxidation of lactate near the outer surface of the inner mitochondrial membrane, which releases a proton, would contribute to the ΔpH, and in turn, pyruvate transport into the matrix. Adjacent to the mitochondrial inner membrane, the LDH mass action ratio (i.e., concentrations of products/concentrations of reactions) could be largely facilitated by the “bleeding off” of pyruvate as it is continuously transported across the lactate-impermeable mitochondrial inner membrane, as well as a generous regeneration of NAD+ by the extra-matrix malate dehydrogenase of the MAS. In this model, the transport of pyruvate across in the inner mitochondrial membrane would directly influence the rate of lactate oxidation just outside the matrix. Lactate oxidation at the mitochondria would therefore be expected to be regulated indirectly at the PDH reaction in the matrix. This would be advantageous because unlike LDH, PDH is highly regulated via allostery and covalent modification. As mentioned, modeling predicts high PDH activity to dictate neuronal lactate consumption in vivo (Neves et al., 2012); and high PDH activity also characterizes lactate-consuming neurons in culture (Halim et al., 2010).
Methodological Considerations
If mitochondrial lactate oxidation is functionally linked to the activity of the MAS, then it would be important to include components of the MAS in in vitro analyses of mitochondrial lactate oxidation, such as malate itself or oxaloacetate. Malate is the likely choice, as it is routinely included to stimulate respiration in vitro, where it is transported into the matrix and oxidized by mitochondrial malate dehydrogenase to oxaloacetate. This oxaloacetate can then condense with acetyl coA formed, for example, when pyruvate is added. Including glutamate in addition to malate, allows full operation of the MAS at the level of mitochondrial respiration. An important, but sometimes overlooked aspect of appropriate mitochondrial lactate oxidation assessment is the inclusion of NAD+ as the requisite cofactor for the LDH reaction, and ADP as the phosphate acceptor to stimulate oxidative phosphorylation (i.e., state 3 respiration). Also, the extra-matrix component of the MAS involves the malate dehydrogenase reaction: oxaloacetate + NADH + H+ ⇌ malate + NAD+. Experimental protocols examining respiratory oxygen consumption in isolated mitochondria from muscle using high malate concentrations (e.g., 4 mM; Rasmussen et al., 2002), may favor the malate dehydrogenase reaction in the reverse direction (i.e., malate oxidation and NADH + H+ production) when added to the mitochondrial sample in combination with NAD+. Indeed, reversibility of the MAS has been observed in isolated hepatocytes (Berry, 1971) and mitochondria with reconstituted MAS (Kunz and Davis, 1991). The net effect of this MAS reversal on respiration would be to reduce malate from entering the mitochondria, forming oxaloacetate. More importantly, the reversal would prevent lactate oxidation to pyruvate, and subsequent transport and oxidation of the pyruvate in the matrix. Solutions to these methodological barriers to observing mitochondrial lactate oxidation in vitro involve including at least one component of the MAS. If adding malate, the appropriate concentration should be determined experimentally. Including ADP and NAD+ or NADH (recall, the MAS will generate NAD+ for the LDH reaction) is necessary also to observe appreciable mitochondrial lactate oxidation.
Summary
A lactate-MAS is the interaction between the lactate and malate-aspartate shuttles to translocate reducing power to the mitochondria, particularly within oxidative, metabolically active cells.
Conflict of Interest Statement
The author declares that the research was conducted in the absence of any commercial or financial relationships that could be construed as a potential conflict of interest.
Acknowledgments
Many thanks to Dr. Erich Gnaiger (Medical University of Innsbruck), and Dr. George Brooks (University of California, Berkeley) for each critiquing earlier drafts of this work.
References
Barros, L. F. (2013). Metabolic signaling by lactate in the brain. Trends Neurosci. 36, 396–404. doi: 10.1016/j.tins.2013.04.002
Pubmed Abstract | Pubmed Full Text | CrossRef Full Text | Google Scholar
Bergman, B. C., Wolfel, E. E., Butterfield, G. E., Lopaschuk, G. D., Casazza, G. A., Horning, M. A., et al. (1999). Active muscle and whole body lactate kinetics after endurance training in men. J. Appl. Physiol. (1985) 87, 1684–1696.
Berry, M. N. (1971). Energy-dependent reduction of pyruvate to lactate by intact isolated parenchymal cells from rat liver. Biochem. Biophys. Res. Commun. 44, 1449–1456. doi: 10.1016/S0006-291X(71)80248-6
Pubmed Abstract | Pubmed Full Text | CrossRef Full Text | Google Scholar
Boumezbeur, F., Petersen, K. F., Cline, G. W., Mason, G. F., Behar, K. L., Shulman, G. I., et al. (2010). The contribution of blood lactate to brain energy metabolism in humans measured by dynamic 13C nuclear magnetic resonance spectroscopy. J. Neurosci. 30, 13983–13991. doi: 10.1523/JNEUROSCI.2040-10.2010
Pubmed Abstract | Pubmed Full Text | CrossRef Full Text | Google Scholar
Bouzier-Sore, A. K., and Pellerin, L. (2013). Unraveling the complex metabolic nature of astrocytes. Front. Cell. Neurosci. 7:179. doi: 10.3389/fncel.2013.00179
Pubmed Abstract | Pubmed Full Text | CrossRef Full Text | Google Scholar
Bouzier-Sore, A. K., Voisin, P., Bouchaud, V., Bezancon, E., Franconi, J. M., and Pellerin, L. (2006). Competition between glucose and lactate as oxidative energy substrates in both neurons and astrocytes: a comparative NMR study. Eur. J. Neurosci. 24, 1687–1694. doi: 10.1111/j.1460-9568.2006.05056.x
Pubmed Abstract | Pubmed Full Text | CrossRef Full Text | Google Scholar
Brooks, G. A. (1998). Mammalian fuel utilization during sustained exercise. Comp. Biochem. Physiol. B Biochem. Mol. Biol. 120, 89–107. doi: 10.1016/S0305-0491(98)00025-X
Pubmed Abstract | Pubmed Full Text | CrossRef Full Text | Google Scholar
Brooks, G. A. (2009). Cell-cell and intracellular lactate shuttles. J. Physiol. 587, 5591–5600. doi: 10.1113/jphysiol.2009.178350
Pubmed Abstract | Pubmed Full Text | CrossRef Full Text | Google Scholar
Brooks, G. A. (2014). “Metabolic systems: the formation and utilization of lactate,” in History of Exercise Physiology. Human Kinetics, ed C. M. Tipton (Champaign, IL: Human Kinetics), 447–475.
Brooks, G. A., Butterfield, G. E., Wolfe, R. R., Groves, B. M., Mazzeo, R. S., Sutton, J. R., et al. (1991). Decreased reliance on lactate during exercise after acclimatization to 4,300 m. J. Appl. Physiol. (1985). 71, 333–341.
Brooks, G. A., Dubouchaud, H., Brown, M., Sicurello, J. P., and Butz, C. E. (1999). Role of mitochondrial lactate dehydrogenase and lactate oxidation in the intracellular lactate shuttle. Proc. Natl. Acad. Sci. U.S.A. 96, 1129–1134. doi: 10.1073/pnas.96.3.1129
Pubmed Abstract | Pubmed Full Text | CrossRef Full Text | Google Scholar
Chatham, J. C., Des Rosiers, C., and Forder, J. R. (2001). Evidence of separate pathways for lactate uptake and release by the perfused rat heart. Am. J. Physiol. Endocrinol. Metab. 281, E794–E802.
Contreras, L., and Satrustegui, J. (2009). Calcium signaling in brain mitochondria: interplay of malate aspartate NADH shuttle and calcium uniporter/mitochondrial dehydrogenase pathways. J. Biol. Chem. 284, 7091–7099. doi: 10.1074/jbc.M808066200
Pubmed Abstract | Pubmed Full Text | CrossRef Full Text | Google Scholar
Crabtree, B., and Newsholme, E. A. (1978). Sensitivity of a near-equilibrium reaction in a metabolic pathway to changes in substrate concentration. Eur. J. Biochem. 89, 19–22. doi: 10.1111/j.1432-1033.1978.tb20891.x
Pubmed Abstract | Pubmed Full Text | CrossRef Full Text | Google Scholar
Cruz, R. S., de Aguiar, R. A., Turnes, T., Penteado Dos Santos, R., de Oliveira, M. F., and Caputo, F. (2012). Intracellular shuttle: the lactate aerobic metabolism. ScientificWorldJournal 2012:420984. doi: 10.1100/2012/420984
Pubmed Abstract | Pubmed Full Text | CrossRef Full Text | Google Scholar
Dienel, G. A. (2012). Brain lactate metabolism: the discoveries and the controversies. J. Cereb. Blood Flow Metab. 32, 1107–1138. doi: 10.1038/jcbfm.2011.175
Pubmed Abstract | Pubmed Full Text | CrossRef Full Text | Google Scholar
Digerness, S. B., and Reddy, W. J. (1976). The malate-aspartate shuttle in heart mitochondria. J. Mol. Cell. Cardiol. 8, 779–785. doi: 10.1016/0022-2828(76)90084-5
Pubmed Abstract | Pubmed Full Text | CrossRef Full Text | Google Scholar
Doherty, J. R., and Cleveland, J. L. (2013). Targeting lactate metabolism for cancer therapeutics. J. Clin. Invest. 123, 3685–3692. doi: 10.1172/JCI69741
Pubmed Abstract | Pubmed Full Text | CrossRef Full Text | Google Scholar
Donovan, C. M., and Pagliassotti, M. J. (2000). Quantitative assessment of pathways for lactate disposal in skeletal muscle fiber types. Med. Sci. Sports Exerc. 32, 772–777. doi: 10.1097/00005768-200004000-00009
Pubmed Abstract | Pubmed Full Text | CrossRef Full Text | Google Scholar
Elustondo, P. A., White, A. E., Hughes, M. E., Brebner, K., Pavlov, E., and Kane, D. A. (2013). Physical and functional association of lactate dehydrogenase (LDH) with skeletal muscle mitochondria. J. Biol. Chem. 288, 25309–25317. doi: 10.1074/jbc.M113.476648
Pubmed Abstract | Pubmed Full Text | CrossRef Full Text | Google Scholar
Funfschilling, U., Supplie, L. M., Mahad, D., Boretius, S., Saab, A. S., Edgar, J., et al. (2012). Glycolytic oligodendrocytes maintain myelin and long-term axonal integrity. Nature 485, 517–521. doi: 10.1038/nature11007
Pubmed Abstract | Pubmed Full Text | CrossRef Full Text | Google Scholar
Gellerich, F. N., Gizatullina, Z., Gainutdinov, T., Muth, K., Seppet, E., Orynbayeva, Z., et al. (2013). The control of brain mitochondrial energization by cytosolic calcium: the mitochondrial gas pedal. IUBMB Life 65, 180–190. doi: 10.1002/iub.1131
Pubmed Abstract | Pubmed Full Text | CrossRef Full Text | Google Scholar
Gellerich, F. N., Gizatullina, Z., Trumbekaite, S., Korzeniewski, B., Gaynutdinov, T., Seppet, E., et al. (2012). Cytosolic Ca2+ regulates the energization of isolated brain mitochondria by formation of pyruvate through the malate-aspartate shuttle. Biochem. J. 443, 747–755. doi: 10.1042/BJ20110765
Pubmed Abstract | Pubmed Full Text | CrossRef Full Text | Google Scholar
Gertz, E. W., Wisneski, J. A., Stanley, W. C., and Neese, R. A. (1988). Myocardial substrate utilization during exercise in humans. Dual carbon-labeled carbohydrate isotope experiments. J. Clin. Invest. 82, 2017–2025. doi: 10.1172/JCI113822
Pubmed Abstract | Pubmed Full Text | CrossRef Full Text | Google Scholar
Gladden, L. B. (2004). Lactate metabolism: a new paradigm for the third millennium. J. Physiol. 558, 5–30. doi: 10.1113/jphysiol.2003.058701
Pubmed Abstract | Pubmed Full Text | CrossRef Full Text | Google Scholar
Gladden, L. B. (2007). Is there an intracellular lactate shuttle in skeletal muscle? J. Physiol. 582, 899. doi: 10.1113/jphysiol.2007.138487
Pubmed Abstract | Pubmed Full Text | CrossRef Full Text | Google Scholar
Halestrap, A. P. (1975). The mitochondrial pyruvate carrier. Kinetics and specificity for substrates and inhibitors. Biochem. J. 148, 85–96.
Halim, N. D., McFate, T., Mohyeldin, A., Okagaki, P., Korotchkina, L. G., Patel, M. S., et al. (2010). Phosphorylation status of pyruvate dehydrogenase distinguishes metabolic phenotypes of cultured rat brain astrocytes and neurons. Glia 58, 1168–1176. doi: 10.1002/glia.20996
Pubmed Abstract | Pubmed Full Text | CrossRef Full Text | Google Scholar
Jacobs, R. A., Meinild, A. K., Nordsborg, N. B., and Lundby, C. (2013). Lactate oxidation in human skeletal muscle mitochondria. Am. J. Physiol. Endocrinol. Metab. 304, E686–E689. doi: 10.1152/ajpendo.00476.2012
Pubmed Abstract | Pubmed Full Text | CrossRef Full Text | Google Scholar
Joubert, F., Mateo, P., Gillet, B., Beloeil, J. C., Mazet, J. L., and Hoerter, J. A. (2004). CK flux or direct ATP transfer: versatility of energy transfer pathways evidenced by NMR in the perfused heart. Mol. Cell. Biochem. 256–257, 43–58. doi: 10.1023/B:MCBI.0000009858.41434.fc
Pubmed Abstract | Pubmed Full Text | CrossRef Full Text | Google Scholar
Kauppinen, R. A., Sihra, T. S., and Nicholls, D. G. (1987). Aminooxyacetic acid inhibits the malate-aspartate shuttle in isolated nerve terminals and prevents the mitochondria from utilizing glycolytic substrates. Biochim. Biophys. Acta 930, 173–178. doi: 10.1016/0167-4889(87)90029-2
Pubmed Abstract | Pubmed Full Text | CrossRef Full Text | Google Scholar
Kitaoka, Y., Hoshino, D., and Hatta, H. (2012). Monocarboxylate transporter and lactate metabolism. J. Phys. Fitness Sports Med. 1, 247–252. doi: 10.7600/jpfsm.1.247
Kline, E. S., Brandt, R. B., Laux, J. E., Spainhour, S. E., Higgins, E. S., Rogers, K. S., et al. (1986). Localization of L-lactate dehydrogenase in mitochondria. Arch. Biochem. Biophys. 246, 673–680. doi: 10.1016/0003-9861(86)90323-1
Pubmed Abstract | Pubmed Full Text | CrossRef Full Text | Google Scholar
Kunz, W. S., and Davis, E. J. (1991). Control of reversible intracellular transfer of reducing potential. Arch. Biochem. Biophys. 284, 40–46. doi: 10.1016/0003-9861(91)90260-P
Pubmed Abstract | Pubmed Full Text | CrossRef Full Text | Google Scholar
Luft, F. C. (2001). Lactic acidosis update for critical care clinicians. J. Am. Soc. Nephrol. 12 Suppl 17: S15–S19.
McKenna, M. C., Tildon, J. T., Stevenson, J. H., Boatright, R., and Huang, S. (1993). Regulation of energy metabolism in synaptic terminals and cultured rat brain astrocytes: differences revealed using aminooxyacetate. Dev. Neurosci. 15, 320–329. doi: 10.1159/000111351
Pubmed Abstract | Pubmed Full Text | CrossRef Full Text | Google Scholar
McKenna, M. C., Waagepetersen, H. S., Schousboe, A., and Sonnewald, U. (2006). Neuronal and astrocytic shuttle mechanisms for cytosolic-mitochondrial transfer of reducing equivalents: current evidence and pharmacological tools. Biochem. Pharmacol. 71, 399–407. doi: 10.1016/j.bcp.2005.10.011
Pubmed Abstract | Pubmed Full Text | CrossRef Full Text | Google Scholar
Neves, A., Costalat, R., and Pellerin, L. (2012). Determinants of brain cell metabolic phenotypes and energy substrate utilization unraveled with a modeling approach. PLoS Comput. Biol. 8:e1002686. doi: 10.1371/journal.pcbi.1002686
Pubmed Abstract | Pubmed Full Text | CrossRef Full Text | Google Scholar
Newington, J. T., Harris, R. A., and Cumming, R. C. (2013). Reevaluating metabolism in alzheimer's disease from the perspective of the astrocyte-neuron lactate shuttle model. J. Neurodegener. Dis. 2013, 13. doi: 10.1155/2013/234572
Nguyen, N. H., Brathe, A., and Hassel, B. (2003). Neuronal uptake and metabolism of glycerol and the neuronal expression of mitochondrial glycerol-3-phosphate dehydrogenase. J. Neurochem. 85, 831–842. doi: 10.1046/j.1471-4159.2003.01762.x
Pubmed Abstract | Pubmed Full Text | CrossRef Full Text | Google Scholar
O'Donnell, J. M., Kudej, R. K., LaNoue, K. F., Vatner, S. F., and Lewandowski, E. D. (2004). Limited transfer of cytosolic NADH into mitochondria at high cardiac workload. Am. J. Physiol. Heart Circ. Physiol. 286, H2237–H2242. doi: 10.1152/ajpheart.01113.2003
Pubmed Abstract | Pubmed Full Text | CrossRef Full Text | Google Scholar
Palaiologos, G., Hertz, L., and Schousboe, A. (1988). Evidence that aspartate aminotransferase activity and ketodicarboxylate carrier function are essential for biosynthesis of transmitter glutamate. J. Neurochem. 51, 317–320. doi: 10.1111/j.1471-4159.1988.tb04872.x
Pubmed Abstract | Pubmed Full Text | CrossRef Full Text | Google Scholar
Papa, S., Francavilla, A., Paradies, G., and Meduri, B. (1971). The transport of pyruvate in rat liver mitochondria. FEBS Lett. 12, 285–288. doi: 10.1016/0014-5793(71)80200-4
Pubmed Abstract | Pubmed Full Text | CrossRef Full Text | Google Scholar
Pardo, B., Contreras, L., Serrano, A., Ramos, M., Kobayashi, K., Iijima, M., et al. (2006). Essential role of aralar in the transduction of small Ca2+ signals to neuronal mitochondria. J. Biol. Chem. 281, 1039–1047. doi: 10.1074/jbc.M507270200
Pubmed Abstract | Pubmed Full Text | CrossRef Full Text | Google Scholar
Pellerin, L., and Magistretti, P. J. (2012). Sweet sixteen for ANLS. J. Cereb. Blood Flow Metab. 32, 1152–1166. doi: 10.1038/jcbfm.2011.149
Pubmed Abstract | Pubmed Full Text | CrossRef Full Text | Google Scholar
Quistorff, B., and Grunnet, N. (2011a). High brain lactate is not caused by a shift in the lactate dehydrogenase A/B ratio. Proc. Natl. Acad. Sci. U.S.A. 108, E21. author reply: E22. doi: 10.1073/pnas.1017750108
Pubmed Abstract | Pubmed Full Text | CrossRef Full Text | Google Scholar
Quistorff, B., and Grunnet, N. (2011b). The isoenzyme pattern of LDH does not play a physiological role; except perhaps during fast transitions in energy metabolism. Aging (Albany, NY) 3, 457–460.
Quistorff, B., Secher, N. H., and Van Lieshout, J. J. (2008). Lactate fuels the human brain during exercise. FASEB J. 22, 3443–3449. doi: 10.1096/fj.08-106104
Pubmed Abstract | Pubmed Full Text | CrossRef Full Text | Google Scholar
Ramos, M., del Arco, A., Pardo, B., Martinez-Serrano, A., Martinez-Morales, J. R., Kobayashi, K., et al. (2003). Developmental changes in the Ca2+-regulated mitochondrial aspartate-glutamate carrier aralar1 in brain and prominent expression in the spinal cord. Brain Res. Dev. Brain Res. 143, 33–46. doi: 10.1016/S0165-3806(03)00097-X
Pubmed Abstract | Pubmed Full Text | CrossRef Full Text | Google Scholar
Rasmussen, H. N., van Hall, G., and Rasmussen, U. F. (2002). Lactate dehydrogenase is not a mitochondrial enzyme in human and mouse vastus lateralis muscle. J. Physiol. 541, 575–580. doi: 10.1113/jphysiol.2002.019216
Pubmed Abstract | Pubmed Full Text | CrossRef Full Text | Google Scholar
Robergs, R. A., Ghiasvand, F., and Parker, D. (2004). Biochemistry of exercise-induced metabolic acidosis. Am. J. Physiol. Regul. Integr. Comp. Physiol. 287, R502–R516. doi: 10.1152/ajpregu.00114.2004
Pubmed Abstract | Pubmed Full Text | CrossRef Full Text | Google Scholar
Rueda, C. B., Llorente-Folch, I., Amigo, I., Contreras, L., Gonzalez-Sanchez, P., Martinez-Valero, P., et al. (2014). Ca2+ regulation of mitochondrial function in neurons. Biochim. Biophys. Acta 1837, 1617–1624. doi: 10.1016/j.bbabio.2014.04.010
Safer, B., Smith, C. M., and Williamson, J. R. (1971). Control of the transport of reducing equivalents across the mitochondrial membrane in perfused rat heart. J. Mol. Cell. Cardiol. 2, 111–124. doi: 10.1016/0022-2828(71)90065-4
Pubmed Abstract | Pubmed Full Text | CrossRef Full Text | Google Scholar
Sampol, D., Ostrofet, E., Jobin, M. L., Raffard, G., Sanchez, S., Bouchaud, V., et al. (2013). Glucose and lactate metabolism in the awake and stimulated rat: a (13)C-NMR study. Front. Neuroenergetics 5:5. doi: 10.3389/fnene.2013.00005
Pubmed Abstract | Pubmed Full Text | CrossRef Full Text | Google Scholar
Santo-Domingo, J., and Demaurex, N. (2012). Perspectives on: SGP symposium on mitochondrial physiology and medicine: the renaissance of mitochondrial pH. J. Gen. Physiol. 139, 415–423. doi: 10.1085/jgp.201110767
Pubmed Abstract | Pubmed Full Text | CrossRef Full Text | Google Scholar
Schantz, P. G. (1986). Plasticity of human skeletal muscle with special reference to effects of physical training on enzyme levels of the NADH shuttles and phenotypic expression of slow and fast myofibrillar proteins. Acta Physiol. Scand. Suppl. 558, 1–62.
Schurr, A. (2014). Cerebral glycolysis: a century of persistent misunderstanding and misconception. Front. Neurosci. 8:360. doi: 10.3389/fnins.2014.00360
Schurr, A., West, C. A., and Rigor, B. M. (1988). Lactate-supported synaptic function in the rat hippocampal slice preparation. Science 240, 1326–1328. doi: 10.1126/science.3375817
Pubmed Abstract | Pubmed Full Text | CrossRef Full Text | Google Scholar
Skilleter, D. N., and Kun, E. (1972). The oxidation of L-lactate by liver mitochondria. Arch. Biochem. Biophys. 152, 92–104. doi: 10.1016/0003-9861(72)90197-X
Pubmed Abstract | Pubmed Full Text | CrossRef Full Text | Google Scholar
Spriet, L. L., Howlett, R. A., and Heigenhauser, G. J. (2000). An enzymatic approach to lactate production in human skeletal muscle during exercise. Med. Sci. Sports Exerc. 32, 756–763. doi: 10.1097/00005768-200004000-00007
Pubmed Abstract | Pubmed Full Text | CrossRef Full Text | Google Scholar
Stainsby, W. N., and Brooks, G. A. (1990). Control of lactic acid metabolism in contracting muscles and during exercise. Exerc. Sport Sci. Rev. 18, 29–63. doi: 10.1249/00003677-199001000-00005
Pubmed Abstract | Pubmed Full Text | CrossRef Full Text | Google Scholar
Sun, F., Dai, C., Xie, J., and Hu, X. (2012). Biochemical issues in estimation of cytosolic free NAD/NADH ratio. PLoS ONE 7:e34525. doi: 10.1371/journal.pone.0034525
Pubmed Abstract | Pubmed Full Text | CrossRef Full Text | Google Scholar
Todd, J. J. (2014). Lactate: valuable for physical performance and maintenance of brain function during exercise. Biosci. Horiz. 7, 1–7. doi: 10.1093/biohorizons/hzu001
van Hall, G. (2010). Lactate kinetics in human tissues at rest and during exercise. Acta Physiol. (Oxf.) 199, 499–508. doi: 10.1111/j.1748-1716.2010.02122.x
Pubmed Abstract | Pubmed Full Text | CrossRef Full Text | Google Scholar
van Hall, G., Stromstad, M., Rasmussen, P., Jans, O., Zaar, M., Gam, C., et al. (2009). Blood lactate is an important energy source for the human brain. J. Cereb. Blood Flow Metab. 29, 1121–1129. doi: 10.1038/jcbfm.2009.35
Pubmed Abstract | Pubmed Full Text | CrossRef Full Text | Google Scholar
Voet, D., Voet, J. G., and Pratt, C. W. (2011). Fundamentals of Biochemistry: Life at the Molecular Level. Hoboken, NJ: Wiley.
Williamson, D. H., Lund, P., and Krebs, H. A. (1967). The redox state of free nicotinamide-adenine dinucleotide in the cytoplasm and mitochondria of rat liver. Biochem. J. 103, 514–527.
Wyss, M. T., Jolivet, R., Buck, A., Magistretti, P. J., and Weber, B. (2011). In vivo evidence for lactate as a neuronal energy source. J. Neurosci. 31, 7477–7485. doi: 10.1523/JNEUROSCI.0415-11.2011
Pubmed Abstract | Pubmed Full Text | CrossRef Full Text | Google Scholar
Keywords: lactate, lactate dehydrogenases, mitochondria, pyruvates, malate aspartate
Citation: Kane DA (2014) Lactate oxidation at the mitochondria: a lactate-malate-aspartate shuttle at work. Front. Neurosci. 8:366. doi: 10.3389/fnins.2014.00366
Received: 11 September 2014; Accepted: 25 October 2014;
Published online: 25 November 2014.
Edited by:
Avital Schurr, University of Louisville, USAEvelyne Gozal, University of Louisville, USA
Reviewed by:
Tibor Kristian, University of Maryland School of Medicine, USADaniela Calvetti, Case Western Reserve University, USA
Copyright © 2014 Kane. This is an open-access article distributed under the terms of the Creative Commons Attribution License (CC BY). The use, distribution or reproduction in other forums is permitted, provided the original author(s) or licensor are credited and that the original publication in this journal is cited, in accordance with accepted academic practice. No use, distribution or reproduction is permitted which does not comply with these terms.
*Correspondence: Daniel A. Kane, Department of Human Kinetics, St. Francis Xavier University, 1 West Street, PO Box 5000, Antigonish, NS B2G 2W5, Canada e-mail: dkane@stfx.ca