The neural substrates of subjective time dilation
- 1 Cognitive Neuroimaging Unit, Institut National de la Santé et de la Recherche Médicale, U992, Gif/Yvette, France
- 2 CEA, DSV/I2BM, NeuroSpin Center, Gif/Yvette, France
- 3 Cognitive Neuroimaging Unit, Université Paris-Sud, Gif/Yvette, France
- 4 Department of Empirical and Analytical Psychophysics, Institute for Frontier Areas of Psychology and Mental Health, Freiburg, Germany
- 5 Department of Psychiatry, University of California San Diego, La Jolla, CA, USA
- 6 Veterans Affairs San Diego Healthcare System, San Diego, CA, USA
- 7 Atkinson Research Laboratory, Division of Neurosurgery, Barrow Neurological Institute, Phoenix, AZ, USA
For a given physical duration, certain events can be experienced as subjectively longer in duration than others. Try this for yourself: take a quick glance at the second hand of a clock. Immediately, the tick will pause momentarily and appear to be longer than the subsequent ticks. Yet, they all last exactly 1 s. By and large, a deviant or an unexpected stimulus in a series of similar events (same duration, same features) can elicit a relative overestimation of subjective time (or “time dilation”) but, as is shown here, this is not always the case. We conducted an event-related functional magnetic neuroimaging study on the time dilation effect. Participants were presented with a series of five visual discs, all static and of equal duration (standards) except for the fourth one, a looming or a receding target. The duration of the target was systematically varied and participants judged whether it was shorter or longer than all other standards in the sequence. Subjective time dilation was observed for the looming stimulus but not for the receding one, which was estimated to be of equal duration to the standards. The neural activation for targets (looming and receding) contrasted with the standards revealed an increased activation of the anterior insula and of the anterior cingulate cortex. Contrasting the looming with the receding targets (i.e., capturing the time dilation effect proper) revealed a specific activation of cortical midline structures. The implication of midline structures in the time dilation illusion is here interpreted in the context of self-referential processes.
Introduction
The experience of subjective time flow changes continuously. A classic dichotomy can illustrate this point: a given duration, say a minute, is not experientially invariant. Specifically, when awaiting for something, or feeling bored or blue, time drags; when entertained or absorbed in skillful performance, time flies (i.e., we are hardly aware of the minutes passing by).
The allocation of attention to time constitutes one major factor influencing whether the subjective flow of time will “speed up” or “slow down:” when attention is focused on time, perceived time slows down and experienced duration expands; when distracted away from it, it speeds up and duration contracts. Traditionally, cognitive models of time perception account for the speeding up or slowing down of time passing by positing the existence of an internal “clock” composed of a pacemaker (internal pacing of time units) and an accumulator (collection of time units) whose combined function is to represent subjective duration (Treisman, 1963; Gibbon et al., 1984). In the “attentional gate clock models,” the produced time units or pulses are only registered when attention is directed to time (Zakay and Block, 1997) leading to the opening of the gate and feeding of pulses into a counter.
Yet, two mechanisms can lead to alterations of subjective duration: (1) an increased (decreased) attention to time leads to an accumulation of more (less) pulses over a given time span or (2) an arousal-related increase (decrease) of the rate of pulses emitted by the pacemaker (faster or slower clock rate, respectively), leads to a faster (slower) accumulation of temporal units over time (Burle and Casini, 2001; Droit-Volet and Meck, 2007; Wittmann and Paulus, 2008). For instance, heightened emotional states are physiologically accompanied by an increase in arousal, which would lead to a higher pacemaker rate (Droit-Volet and Gil, 2009): indeed, subjects tend to overestimate the duration of highly arousing pictures with emotional valence (e.g., angry faces or accidents) (Angrilli et al., 1997; Droit-Volet et al., 2004). Similarly, an increase in body temperature is also associated with an overestimation of duration (Wearden and Penton-Voak, 1995) and in dangerous or life-threatening situations, people often report that events are prolonged in time and that everything occurs in slow motion (for such a personal report see Popper and Eccles, 1986, p. 529). During those moments, one experiences strong emotional distress and needs to react as quickly as possible. For these reasons, all bodily resources are recruited in a “fight or flight mode” enabled by heightened arousal levels (Stetson et al., 2007; Wittmann, 2009a). If mental processing speeds up considerably, external events seem to slow down accordingly as is popularly portrayed in “The matrix” combat scenes.
All together, the rate of the pacemaker in clock models determines the perceived duration of an event whether due to arousal or attention: as a rule of thumb, the faster the rate, the more units accumulated and the longer the perceived duration. However, there is presently no real consensus on the how, when and where of such clock components in the brain (Wittmann and van Wassenhove, 2009) albeit specific functional implementations have been proposed, notably as a centralized and dedicated system for time (Buhusi and Meck, 2005).
In controlled laboratory settings, subjective alterations of time (“temporal illusions”) can be systematically elicited. We now highlight a few cases which remain challenging for a general clock model framework and which could be parsimoniously accounted for by recent alternative proposals. One class of subjective duration effects are observed right before saccadic eye movement: in chronostasis, a backdating mechanism for temporal labeling of the saccadic onset depends on the duration of the preceding eye movement and leads to subjective dilation of duration (Yarrow et al., 2001); analogous effects have been reported in the tactile domain during action (Yarrow and Rothwell, 2003). During saccadic movement, the perceived order of events can be also inverted (Morrone et al., 2005; Binda et al., 2009). Such phenomena have been suggested to be tied to a neural remapping of temporal events compensating for the delay in producing a movement. However, subjective dilation of time can be seen without voluntary action for salient auditory events for instance (Alexander et al., 2005); more generally, the subjective duration of unexpected or infrequent stimuli is overestimated as compared to expected and frequent stimuli (Rose and Summers, 1995; Tse et al., 2004; Ulrich et al., 2006). Conversely, stimulus repetition can lead to a subjective temporal compression of events thereby eliciting an apparent subjective dilation in the non-repeated stimulus (Pariyadath and Eagleman, 2007; van Wassenhove et al., 2008; Eagleman and Pariyadath, 2009). These effects have been accounted for by an energy efficiency model relying on well described neural suppression effects (Eagleman and Pariyadath, 2009) – i.e., an automatic low-level encoding of temporal features. Additionally, visual adaptation paradigms can be used to locally distort the apparent duration of events (Johnston et al., 2006; Bruno et al., 2010) further suggesting that these effects do not necessitate attention and can be specific to stimulus feature or low-level properties of a given stimulus (see for instance Johnston (2010) for a local “content-dependent clock”). In line with this, a recent computational model has also been put forward in which no dedicated system is necessary and which relies on the natural statistics of events (Ahrens and Sahani, 2011). Consistent with local adaptation mechanisms and content-related timing, whereas a looming (expanding) disc embedded within a series of standard discs leads to subjective time dilation (Tse et al. 2004; van Wassenhove et al., 2008), a standard disc embedded within a series of looming discs leads to subjective time compression (van Wassenhove et al., 2008). An attention allocation mechanism would predict temporal dilation not only in the looming condition but also in the receding condition; an adaptation mechanism would rather predict such perceptual inversion (van Wassenhove et al., 2008). Hence, several explanations are currently debated: (1) the attentional gate model (in line with clock models): the subjective dilation of duration results from an increase of attention directed to the deviant stimulus (Tse et al., 2004; New and Scholl, 2009); (2) the energy efficiency coding: a greater amount of energy expenditure for the encoding of a deviant stimulus leads to subjective duration dilation in comparison to higher “coding efficiency” and less energy expenditure for standard stimuli (Eagleman and Pariyadath, 2009); (3) the local neural computations attuned to inherent stimuli properties: temporal effects can be driven by the intrinsic dynamics of the stimulus, namely, faster moving stimuli or stimuli with higher flicker frequency last subjectively longer (Kanai et al., 2006; New and Scholl, 2009). New computational schemes for time estimation are thus emerging albeit with different neural implementations (Karmakar and Buonomano, 2007; Johnston, 2010; Ahrens and Sahani, 2011).
Surprisingly little to no neuroimaging data are currently available that would provide insights on the neural mechanisms mediating such temporal illusions. Here, we report an event-related functional magnetic resonance imaging (fMRI) study (Wittmann et al., 2010b) using a previously demonstrated illusory effect. In this study, subjects viewed a stream of five visual events, all of which were static and of identical duration except for the fourth one, which was a deviant target consisting of a looming or a receding disc (van Wassenhove et al., 2008). The use of an experimental paradigm using a looming signal as a (deviant) target in a stream of steady (standard) events allowed us to test (i) the specificity of temporal dilation and (ii) the neural underpinnings of time perception with respect to self. Looming signals are not only salient and attention-drawing events (Yantis and Egeth, 1999; Franconeri et al., 2005; for a refined hypothesis see Skarratt et al., 2009) they also simulate approaching objects and constitute an intrinsic threat cue. For instance, rhesus monkeys show a persistent avoidance response to looming stimuli (Schiff et al., 1962) and brain responses specific to the dynamics of this stimulus develop in the first year of life in humans (van der Weel and van der Meer, 2009). Looming signals are natural self-referential events: the time-to-(self) contact of such stimuli needs to be computed rapidly for planning an adequate escape behavior.
Our hypothesis was that looming signals engage brain structures involved in the processing of time in self-referential coordinates. This bears particular relevance in the context of a recent proposal pertaining to the experience of time as a self-referential process (Craig, 2008, 2009a,b). One predicted implicated neural structure was the insular cortex which is functionally involved in interoception and is a key area for the integration of information originating within the body and for a meta-representation of homeostatic feelings. The insular cortex would naturally be at the core of the experienced self at one moment in time, providing a continuity of subjective awareness across time through a series of elementary emotional moments (Craig, 2009a). It is noteworthy that although activation of the insular cortex has been repeatedly shown in neuroimaging studies on time perception (e.g., Pouthas et al., 2005; Livesey et al., 2007; Gutyrchik et al., 2010), it is only recently that the insular cortex has been discussed as causally relating to the processing of duration and the experience of time (Craig, 2008, 2009a; Wittmann, 2009b; Kosillo and Smith, 2010; Wittmann et al., 2010a).
Materials and Methods
Psychophysical and fMRI data of 15 right-handed participants (seven female; mean age: 26 years) were analyzed in this study. First, participants underwent a psychophysical test outside the scanner (pre-fMRI) followed by a similar psychophysical test during fMRI. In the pre-fMRI session, participants were presented with a stream of five visual events consisting of three consecutive standard discs (STANDARD), one looming, receding or steady target (LOOM, RECEDE, STEADY) and one last STANDARD disc (Figure 1). In the fMRI session, the target stimulus consisted of either LOOM or RECEDE (i.e., the target was never STEADY). The target was systematically varied in duration and always the fourth event in the stream of ∼500 ms STANDARD discs (fMRI condition: 494.2 ms). The stimuli consisted of gray disks centered on the monitor screen displayed on a black background. Participants had to judge the duration of the target compared to all STANDARD discs. Participants judged in a two-alternative forced choice whether the target was “shorter” or “longer” than all other STANDARD in the trial (i.e., the first, second, third, and fifth stimuli). Due to hardware constraints (refresh rate) slight differences in standard and target durations were chosen in the pre-fMRI and the fMRI sessions: in the pre-fMRI session, STANDARD was 500 ms and targets were ±23.3, ±10, or ±3.3% of this duration; in the fMRI session, STANDARD was 494.2 ms and targets were ±23.8, ±7.1, or ±2.4% of the standard duration.
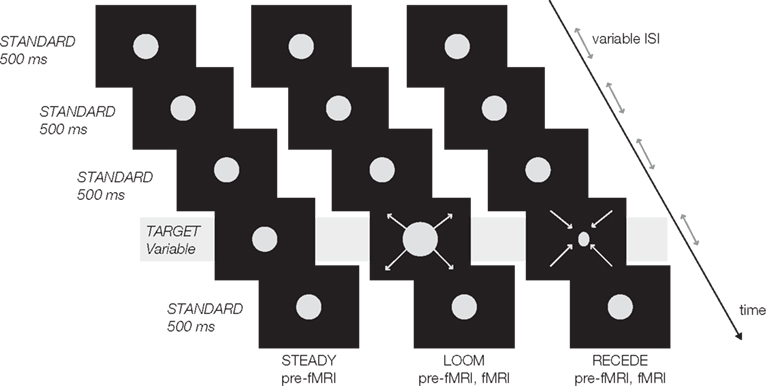
Figure 1. Experimental design: a trial consisted of a stream of five visual events, four standards (in first, second, third and fifth position) and one target (fourth position). All standards were static discs of ∼500 ms duration (STANDARD); all targets were presented in fourth position in the sequence and varied in duration. In the pre-fMRI session, a target could be static (STEADY), looming (LOOM) or receding (RECEDE). In the fMRI session, a target was either LOOM or RECEDE. The inter-stimulus intervals (ISI) and the inter-trial intervals (ITI) were pseudo-randomly chosen from 500 to 1000 ms (ISI), 2 to 4 s (ITI pre-fMRI), and 12 to 14 s (ITI fMRI).
The pre-fMRI session was conducted to (1) train the subjects in the task, (2) evaluate the perceived duration of a steady target to control for the compression of duration due to adaptation to repetition (Eagleman and Pariyadath, 2009), and (3) replicate prior findings with respect to time dilation observed in deviant looming but not receding stimulus presentation (van Wassenhove et al. 2008). To preserve a dynamic control (size, motion, integrated luminance, etc.), the receding signal was the control for the looming condition in the fMRI task, pending replication of the perceptual effects. Participants were scanned in a 3T GE Sigma scanner. Data were analyzed with the Analysis of Functional NeuroImages (AFNI) software package. Brain activations corresponding to each stimulus were contrasted (LOOM vs. STANDARD, RECEDE vs. STANDARD, and LOOM vs. RECEDE) using the estimated voxel-wise response amplitude and function for each regressor of interest covering the specific stimulus duration. t-Tests were used to probe for activation differences between the selected stimulus contrasts. A threshold adjustment method using Monte-Carlo simulations provided significant regions of interest which were corrected both on the voxel and cluster level (P < 0.001 and P < 0.05) if a minimum volume of 512 μl (1440 μl) and a connectivity radius of 4.0 mm was considered. For methodological details concerning the fMRI analysis, see Wittmann et al. (2010b).
Results
Pre-fMRI Psychophysics
The psychophysical results replicated prior findings (van Wassenhove et al., 2008): a temporal dilation effect was observed for LOOM but not for RECEDE targets as can be seen in Figure 2A (percent of “longer” responses for the target as a function of target duration). The points of subjective equality (PSE; Figure 2C) significantly differed in the LOOM vs. the STEADY conditions [t(1,10) = −3.846, P < 0.003), in the LOOM vs. the RECEDE conditions [t(1,11) = −5.269, P < 0.0001], but not in the RECEDE vs. the STEADY conditions [t(1,12) = −1.291, P = 0.221]. On average, a looming target of 419 ms was perceived as having the same duration as a standard event of 500 ms (Figure 2C). Note that the STEADY [PSE: 524 ms, t(1,12) = 1.905, P = 0.081] and the RECEDE [PSE: 498 ms, t(1,13) = 0.316, P = 0.757] stimuli were not significantly perceived as shorter than the standard duration of 500 ms suggesting that the dilation effect does not result from a compression of the standard durations.
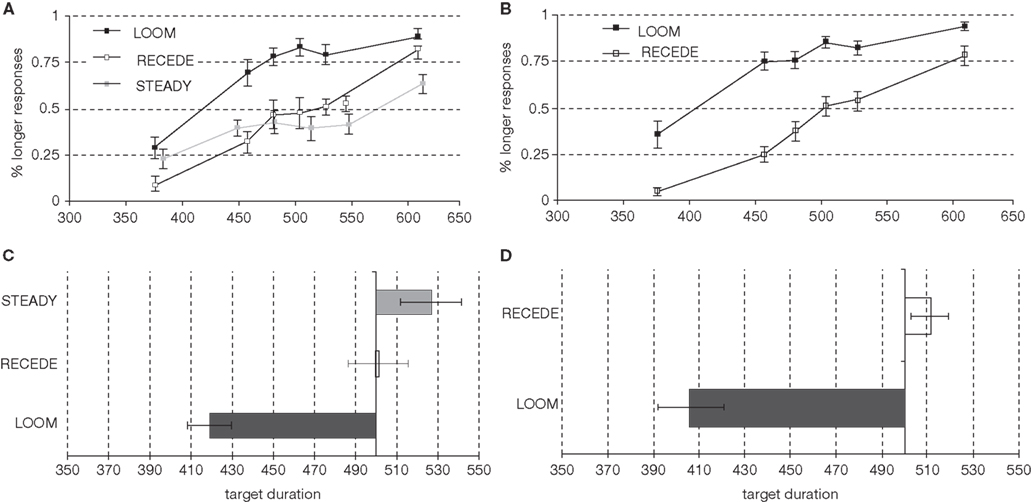
Figure 2. Psychophysical results in the pre-fMRI session (left column) and in the fMRI session (right column): Percent longer responses [(A) pre-fMRI; (B) fMRI], points of subjective equality [PSE; (C) pre-fMRI; (D) fMRI].
fMRI – Psychophysics Results
On the basis of the pre-fMRI behavioral results, we selected the main contrast for the fMRI to be the LOOM (illusory duration) vs. RECEDE (no illusory duration) targets. In the fMRI session, the PSE obtained in the LOOM and the RECEDE conditions differed significantly [t(1,13) = −7.365, P < 0.0001] (Figures 2B,D). As in the pre-fMRI session, a time dilation effect was observed for LOOM but not for RECEDE. On average, a 406 ms looming event was subjectively equal to the 494 ms STANDARD event (Figure 2D).
fMRI
We first contrasted responses to LOOM vs. STANDARD and RECEDE vs. STANDARD. These contrasts should reveal activations in regions related to the temporal evaluation of dynamic visual stimuli. For both contrasts, similar brain activations were detected (P < 0.001, corrected; Figure 3, Tables 1 and 2), namely a stronger activation was observed for the dynamic targets (LOOM, RECEDE) when compared with the STANDARD in (i) the left insula and the adjacent claustrum and basal ganglia, (ii) the anterior cingulate cortex, (iii) the right middle and superior frontal cortex, and (iv) the left superior frontal regions. Additionally, activation was visible for RECEDE as compared to STANDARD in the right insula and the anterior cingulate cortex bilaterally. Next, we contrasted brain activations between LOOM and RECEDE, which should detect neural correlates for the time dilation effect proper. In Figure 2B, the large area between the curves for LOOM and RECEDE condition reflects the magnitude of the subjective looming effect, indicating a large perceptual difference with respect to duration between these two conditions. Accordingly, a contrast between fMRI signals for LOOM and RECEDE was obtained (P < 0.05, corrected; Figure 4, Table 3). Significantly stronger activation for LOOM vs. RECEDE was found in the left medial frontal (including the left mid-cingulate), the posterior cingulate and precuneus regions, together with small portions of the left superior frontal and middle frontal areas. By contrast, stronger activity for RECEDE versus LOOM was observed in the left anterior insula, the left dorsal thalamus and the anterior cerebellum.
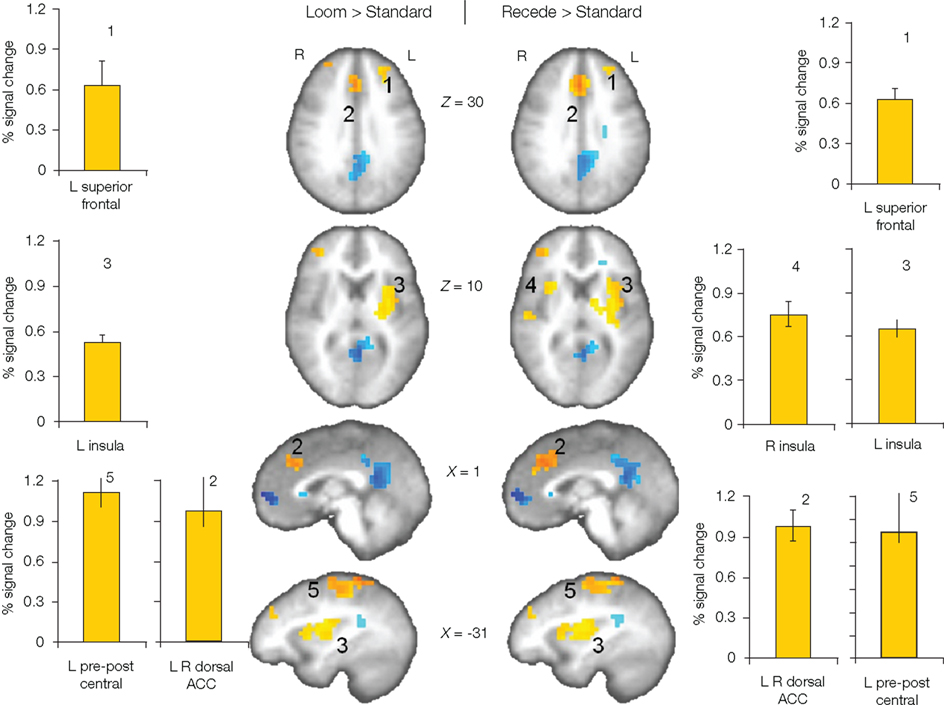
Figure 3. Brain regions with significantly greater activation (yellow to red voxels) for LOOM vs. STANDARD (left) and RECEDE vs. STANDARD (right) contrasts. Activation is depicted in axial (brain slices in row 1 and 2) and sagittal views (row 3 and 4); percent signal change is plotted (mean; SEM) as obtained through t-tests (P < 0.001, corrected). Numbers in slices correspond to numbers in bar charts representing the average percent signal change in specific regions of interest.
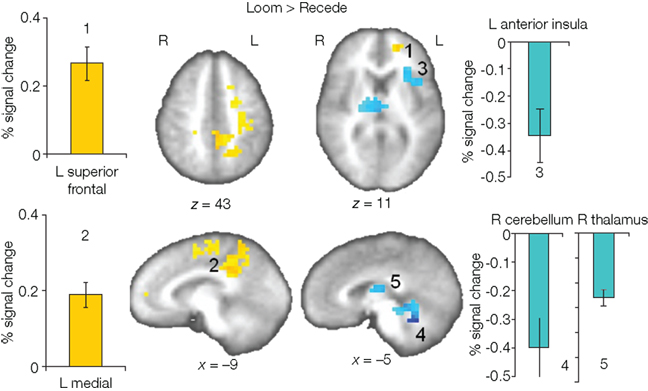
Figure 4. Brain regions with significantly greater activation for LOOM versus RECEDE (yellow to red voxels) and RECEDE versus LOOM (blue voxels) contrasts. Activation is depicted in axial (upper row) and sagittal views (lower rows); percent signal change is plotted (mean; SEM) as obtained through t-tests (P < 0.05, corrected). Numbers in the slices correspond to numbers in bar charts representing average percent signal change in specific regions of interest.
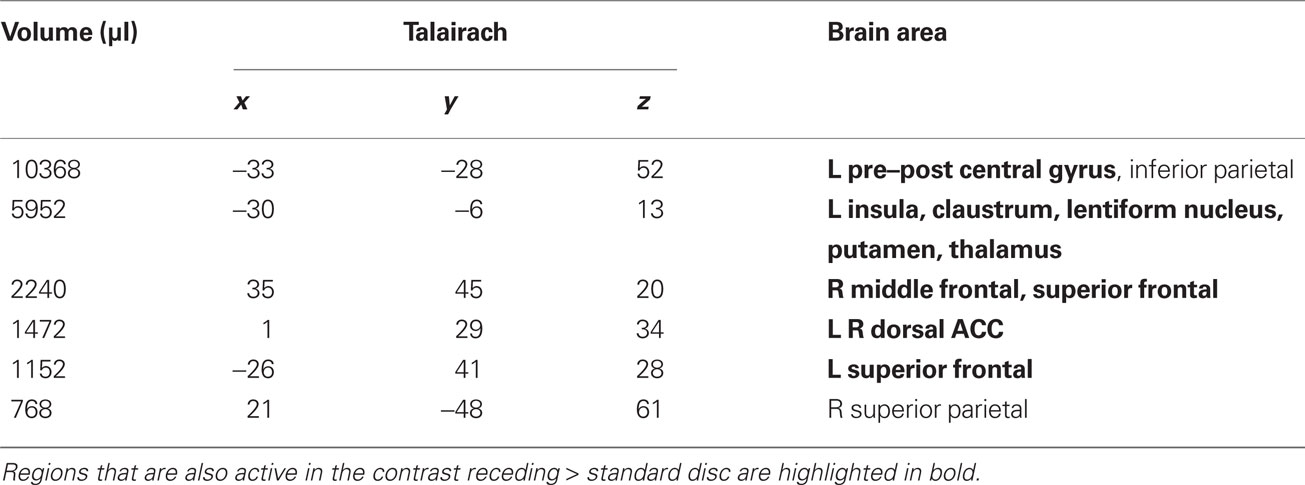
Table 1. LOOM > STANDARD. Regions of significant activation (P < 0.001, corrected) for the contrast looming > standard disc.
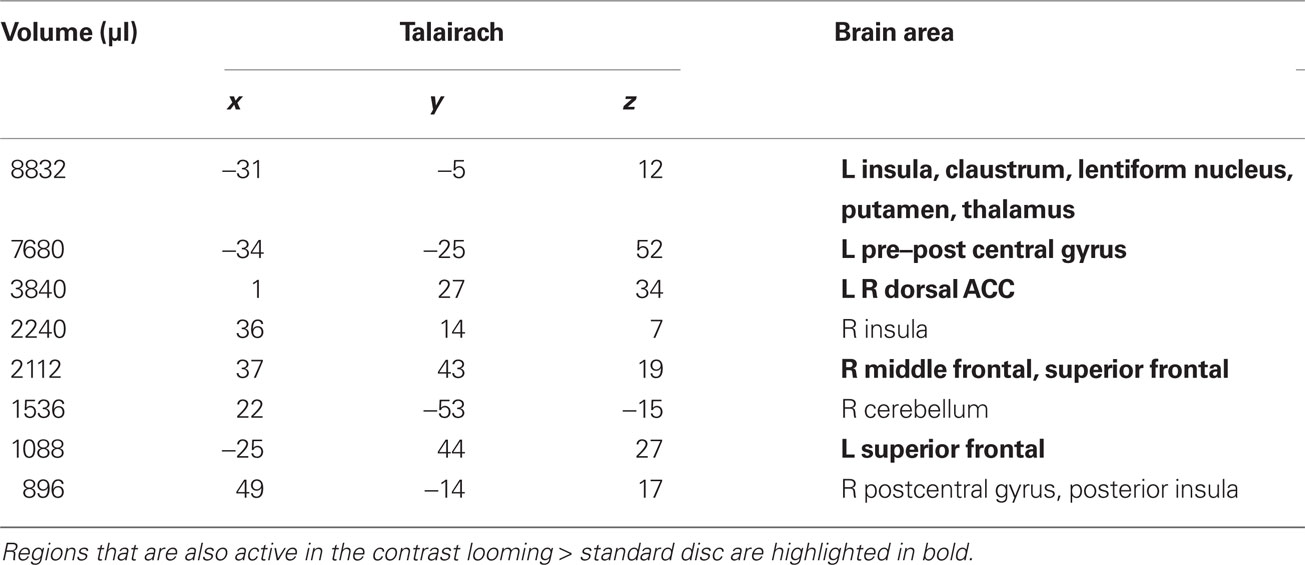
Table 2. RECEDE > STANDARD. Regions of significant activation (P < 0.001, corrected) for the contrast receding > standard disc.
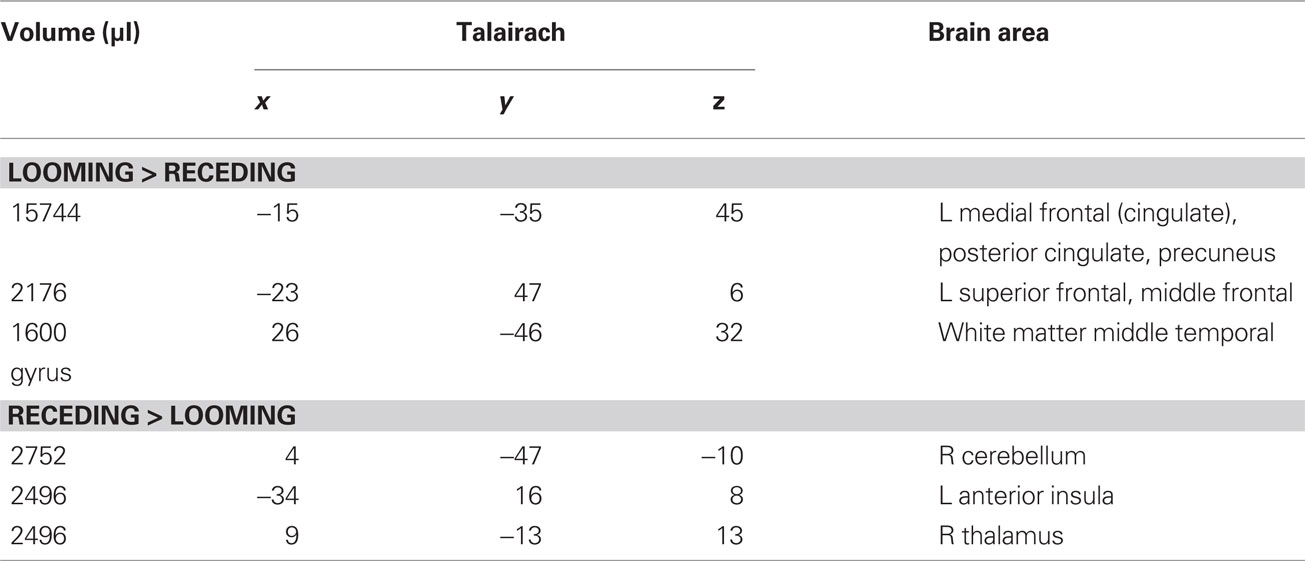
Table 3. LOOM > RECEDE, RECEDE > LOOM. Regions of significant activation (P < 0.05, corrected) for the contrast looming > receding disc and receding > looming disc.
Discussion
This is the first fMRI attempt at delineating brain regions involved in a visual temporal illusion, namely subjective time dilation with looming signals. The behavioral results replicated earlier findings (Tse et al., 2004; van Wassenhove et al., 2008) and showed a profound temporal dilation effect. One goal was to use a predictable temporal locus for the targets (in each trial the target was consistently the fourth in the sequence of five events) in order to avoid any temporal expectation effect due to a sudden allocation of attention to the target: specifically, the overestimation of duration observed here depends on the nature of the stimulus as no effect was found with a receding signal (for a thorough discussion, see van Wassenhove et al., 2008).
In this context, one possible interpretation of the dilation illusion fits the cognitive pacemaker-accumulator model of time perception: an emotional response accompanied by increased arousal (looming) leads to an increased pacemaker rate and a larger accumulation of pacemaker “ticks” (Droit-Volet and Meck, 2007; Wittmann and Paulus, 2008). However, the fMRI analysis shows that similar brain regions were activated in LOOM and RECEDE conditions when contrasted with STANDARD. The outcome of these contrasts can be interpreted as indicating regions of interest involved in the estimation of duration for dynamic or salient targets versus static standards. The insular cortex, the anterior cingulate cortex, the basal ganglia as well as the dorsolateral prefrontal cortex were activated in both contrasts. This is consistent with prior neuroimaging findings on time perception (Lewis and Miall, 2003; Rubia and Smith, 2004; Wittmann, 2009b,c). For instance, the basal ganglia (sp. the striatum) has been ascribed a role as coincidence detector of oscillatory phases (Mattell and Meck, 2004; Buhusi and Meck, 2005); dorsolateral frontal regions have been associated with working memory related integration, and anterior cingulate activity has been related to attention processes in time perception (Lewis and Miall, 2006). This network may thus highlight the comparison process between the duration stored in memory (the standard duration or “reference duration;” Gallistel and Gibbon, 2000) with the target duration to be compared irrespective of its content. It is noteworthy that activation of the insular cortex for both targets in the stream of standards is consistent with the insular cortex mediating alertness in the presence of salient stimulation (Sterzer and Kleinschmidt, 2010). The regions found in this contrast is thus consistent with the implications of neural structures classically implicated in the clock model but such contrast does not offer an explanatory account with regards to the specificity of the illusory percept found with looming versus receding signals.
One hypothesis considered in this study was that the experience of time is fundamentally self-referential (Craig, 2008, 2009a; Wittmann, 2009b), i.e., uses the internal representation of self as a frame of reference (van de Grind, 2002; Northoff et al., 2011). In this context, the use of looming stimuli provides an explicit means to test the effect of a self-referential stimulus. In the decisive LOOM vs. RECEDE contrast, differential activation was found in the mid- and posterior cingulate regions of the left hemisphere. A growing number of empirical studies indicate that mid- and posterior midline cortical structures are associated with the “default brain network” which is active when an individual is in a resting state and presumably engaged in self-relevant thoughts and beliefs (Raichle et al., 2001; Wicker et al., 2003). A recent study addressing the issue of mentalizing about self vs. others (Lombardo et al., 2010) reported the activation of medial prefrontal and posterior cingulate cortices as key structures. In another fMRI study, direct (one’s own self-beliefs) and reflected appraisals (one’s perception of how others view him or her) recruited the mid and posterior cingulate cortex (Ochsner et al., 2005). In the context of the task used here, the involvement of midline structures in the temporal perception of looming (but not receding) signals might be related to the “potential threat” carried by these signals to the perceiver (Schiff et al., 1962). Future studies will have to delineate more carefully subregions within the cingulate cortex and their respective functions (Northoff et al., 2011). As can be seen in Figure 3, a retrospenial region of the posterior cingulate cortex is also active during the presentation of the standards as compared to the dynamic targets, whereas more anterior and dorsal regions are also active in the looming versus receding contrast.
The left lateralized activation of the anterior insula found in RECEDE vs. LOOM is in line with an asymmetric representation of emotional feelings in the anterior insular cortex (Craig, 2008). In primates, the insular cortex functions as the primary receptive area for homeostasis. Re-representations of homeostatic afferent activity have been suggested to form the basis for the subjective awareness of emotional states (Craig, 2009b). The anterior insular and cingulate cortex are conjointly engaged during task performance as complementary limbic sensory and motor regions. Recent evidence suggests that the anterior insula and the anterior cingulate are part of the “core control network.” a system for task-dependent control of sensory information and goal-directed behavior (Cole and Schneider, 2007; Craig, 2009b). This control network is also engaged in the perception of time: the anterior insula has been shown to be related to dysfunctions in timing behavior (Rubia et al., 2009) and specifically involved in multisensory time synchronization (Bushara et al., 2001). The homeostatic awareness model (Craig, 2008, 2009b) provides a neuroanatomical framework for forebrain emotional asymmetry in which the left forebrain is predominantly related to parasympathetic activity (with approach, safety, positive affect), and the right forebrain is predominantly related to sympathetic activity (with arousal, danger, negative affect). Thus, activation in the left anterior insula for the RECEDE condition could be related to the feelings evoked with the receding (and thus non-threatening) stimulus, which is virtually moving away from the perceiver. However, this interpretation is weakened by the fact that no right-sided anterior insula activation (related to negative affect) was found in the LOOM condition, which would have been predicted by this model.
Research in the field of time perception is far from reaching a consensus on the two main questions of how and where in the brain time is processed (Wittmann and van Wassenhove, 2009). This is likely due to the complexity of time research as “time” encompasses a large number of aspects including duration on different time scales, synchrony, order etc. (van Wassenhove, 2009). All together, this study is a first attempt at addressing the difficult issue of content and stimulus-specificity in temporal illusions. Our results provide some hints as to why loom signals last subjectively longer than recede signals and highlight how two simplistic, albeit surprisingly powerful stimuli, can engage differential mechanisms in representing time. One limitation concerning the choice of our receding stimulus may have weakened our findings: an initial jump to a large size for the receding target, which then shrinks to a smaller size, could have led to a smaller effect size. Future experimental designs will likely use 3-D objects for more realistic stimulus rendering, which would be emotionally more salient. A better understanding of such temporal illusions will also largely benefit from temporally resolved neuroimaging techniques (magnetoencephalography, in particular). Nevertheless, the temporal illusion was robust across participants and we were thus able to explore one fundamental aspect of time perception. The findings presented here are noteworthy in offering a new view for the role of self-related processes in the perception of time.
Conflict of Interest Statement
The authors declare that the research was conducted in the absence of any commercial or financial relationships that could be construed as a potential conflict of interest.
Acknowledgments
This work was supported by grants to Martin Paulus and Marc Wittmann from the National Institutes of Health (NIH), National Institute of Drug Abuse (NIDA) (1R03DA020687-01A1) and by a grant from the Kavli Institute for Brain and Mind (KIBM 07-33).
Key Concepts
Time perception
Of all sensory experiences, “sensing time” is a peculiar one: our brains are not equipped with time receptors and time (abstracted out of the dynamics of the perceived environment) poses particularly challenging issues in perception. What is the object of attention when we attend to time? Are all senses sensitive to temporal aspects of the environment and if so, to which extent? We take it that time, as an experience, requires specific neural mechanisms allowing for the construction of a mental representation in the reference frame of the embodied and conscious self.
Temporal illusions
The time percept is fundamentally labile and variant. For instance, an auditory and a visual event of equal (objective) duration are not perceived as such: a sound is typically perceived as being longer than a visual event. Within a sensory modality, the first event in a sequence of identical stimuli, or an unexpected or surprising event can lead to a relative overestimation of its duration. Despite the highly subjective and non-invariant nature of time perception, temporal illusions can be systematically elicited, thereby allowing the assessment of natural (architectural and computational) constraints on the construction of a time percept.
Self-referential
A self-referential process implicates a form of recursion, namely, that the representation of self is incorporated in the encoding and analysis of a world event. Formally, a self-referential process functionally implicates the self, for instance as a reference frame or context of the event being analyzed. The neural structures likely implicated in such contextual representation of self are the cortical midline structures. It is noteworthy that not all events can be deemed as self-referential: the temporal properties of a bird’s song provide information about its identity as a bird within a species (not self-referential) whereas your hand tapping the rhythm of a popular song illustrates your self tuning to the song’s rhythm (a potential case of self-referential embodiment). The looming stimuli we used in this study are related to the self insofar as they can be interpreted as threatening events (an abstraction of an object approaching toward you with a potential impact).
References
Ahrens, M. B., and Sahani, M. (2011). Observers exploit stochastic model of sensory change to help judge the passage of time. Curr. Biol. 21, 1–7.
Alexander, I., Thilo, K. V., Cowey, A., and Walsh, V. (2005). Chronostasis without voluntary action. Exp. Brain Res. 161, 125–132.
Angrilli, A., Cherubini, P., Pavese, A., and Manfredini, S. (1997). The influence of affective factors on time perception. Percept. Psychophys. 59, 972–982.
Binda, P., Cicchini, G. M., Burr, D. C., and Morrone, M. C. (2009). Spatiotemporal distortions of visual perception at the time of saccades. J. Neurosci. 29, 13147–13157.
Bruno, A., Ayhan, I., and Johnston, A. (2010). Retinotopic adaptation-based visual duration compression. J. Vis. 10, 30.
Buhusi, C. V., and Meck, W. H. (2005). What makes us tick? Functional and neural mechanisms of interval timing. Nat. Neurosci. Rev. 6, 755–765.
Burle, B., and Casini, L. (2001). Dissociation between activation and attention effects in time estimation: implications for internal clock models. J. Exp. Psychol. Hum. Percept. Perform. 27, 195–205.
Bushara, K. O., Grafman, J., and Hallett, M. (2001). Neural correlates of auditory-visual stimulus onset asynchrony detection. J. Neurosci. 21, 300–304.
Cole, M. W., and Schneider, W. (2007). The cognitive control network: integrated cortical regions with dissociable functions. Neuroimage 37, 343–360.
Craig, A. D. (2008). “Interoception and emotion: a neuroanatomical perspective,” in Handbook of Emotion, eds M. Lewis, J. M. Haviland-Jones, and L. F. Barrett (New York: Guilford), 272–288.
Craig, A. D. (2009a). Emotional moments across time: a possible neural basis for time perception in the anterior insula. Philos. Trans. R. Soc. Lond. B 364, 1933–1942.
Craig, A. D. (2009b). How do you feel – now? The anterior insula and human awareness. Nat. Rev. Neurosci. 10, 59–70.
Droit-Volet, S., Brunot, S., and Niedenthal, P. M. (2004). Perception of the duration of emotional events. Cogn. Emot. 18, 849–858
Droit-Volet, S., and Gil, S. (2009). The time-emotion paradox. Philos. Trans. R. Soc. Lond. B 364, 1943–1953.
Droit-Volet, S., and Meck, W. H. (2007). How emotions colour our perception of time. Trends Cogn. Sci. 11, 504–513.
Eagleman, D., and Pariyadath, V. (2009). Is subjective duration a signature for coding efficiency? Philos. Trans. R. Soc. Lond. B 364, 1841–1851.
Franconeri, S. L., Hollingworth, A., and Simons, D. J. (2005). Do new objects capture attention? Psychol. Sci. 16, 275–281.
Gibbon, J., Church, R. M., and Meck, W. H. (1984). “Scalar timing in memory,” in Timing and Time Perception, eds J. Gibbon, and L. Allan (New York: Annals of the New York Academy of Sciences), 52–77.
Gutyrchik, E., Churan, J., Meindl, T., Bokde, A. L. W., von Bernewitz, H., Born, C., Reiser, M., Pöppel, E., and Wittmann, M. (2010). Functional neuroimaging of duration discrimination on two different time scales. Neurosci. Lett. 469, 411–415.
Johnston, A. (2010). “Modulation of time perception by visual adaptation,” in Attention and Time, eds K. Nobre, and J. Coull (Oxford: Oxford University Press), 187–200.
Johnston, A., Arnold, D. H., and Nishida, S. (2006). Spatially localized distortions of event time. Curr. Biol. 16, 472–479.
Kanai, R., Paffen, C. L. E., Hogendoorn, H., and Verstraten, F. A. J. (2006). Time dilation in dynamic visual display. J. Vis. 6, 1421–1430.
Karmakar, U. R., and Buonomano, D. V. (2007). Timing in the absence of clocks: encoding time in neural network states. Neuron 53, 427–438.
Kosillo, P., and Smith, A. T. (2010). The role of the human anterior insular cortex in time processing. Brain Struct. Funct. 214, 623–628.
Lewis, P. A., and Miall, R. C. (2003). Distinct systems for automatic and cognitively controlled time measurement: evidence from neuroimaging. Curr. Opin. Neurobiol. 13, 250–255.
Lewis, P. A., and Miall, R. C. (2006). A right hemispheric prefrontal system for cognitive time measurement. Behav. Processes 71, 226–234.
Livesey, A. C., Wall, M. B., and Smith, A. T. (2007). Time perception: manipulation of task difficulty dissociates clock functions from other cognitive demands. Neuropsychologia 45, 321–331.
Lombardo, M. V., Chakrabarti, B., Bullmore, E. T., Wheelwright, S. J., Sadek, S. A., Suckling, J., MRC AIMS Consortiumand Baron-Cohen, S. (2010). Shared neural circuits for mentalizing about the self and others. J. Cogn. Neurosci. 22, 1623–1635.
Mattell, M. S., and Meck, W. H. (2004). Cortico-striatal circuits and interval timing: coincidence detection of oscillatory processes. Cogn. Brain Res. 21, 139–170.
Morrone, M. C., Ross, J., and Burr, D. (2005). Saccadic eye movements cause compression of time as well as space. Nat. Neurosci. 8, 950–954.
New, J. J., and Scholl, B. J. (2009). Subjective time dilation: spatially local, object-based, or a global visual experience. J. Vis. 9, 1–11.
Northoff, G., Qin, P., and Feinberg, T. E. (2011). Brain imaging of the self – Conceptual, anatomical and methodological issues. Conscious. Cogn. 20, 52–63.
Ochsner, K. N., Beer, J. S., Robertson, E. R., Cooper, J. C., Gabrieli, J. D., Kihsltrom, J. K., and D’Esposito, M. (2005). The neural correlates of direct and reflected self-knowledge. Neuroimage 28, 797–814.
Pariyadath, V., and Eagleman, D. (2007). The effect of predictability on subjective duration. PLoS ONE 2, e1264.
Popper, K., and Eccles, J. C. (1986). The self and its brain. An argument for interactionism. London, NY: Routledge.
Pouthas, V., George, N., Poline, J. B., Pfeuty, M., Vandemoorteele, P. F., Hugueville, L., Ferrandez, A. M., Lehericy, S., Lebihan, D., and Renault, B. (2005). Neural network involved in time perception: an fMRI study comparing long and short interval estimation. Hum. Brain Mapp. 25, 433–441.
Raichle, M. E., MacLeod, A. M., Snyder, A. Z., Powers, W. J., Gusnard, D. A., and Shulman, G. L. (2001). A default mode of brain function. Proc. Natl. Acad. Sci. U.S.A. 98, 676–682.
Rose, D., and Summers, J. (1995). Duration illusions in a train of visual stimuli. Perception 24, 1177–1187.
Rubia, K., Halari, R., Christakou, A., and Taylor, E. (2009). Impulsiveness as a timing disturbance: neurocognitive abnormalities in attention-deficit hyperactivity disorder during temporal processes and normalization with methylphenidate. Philos. Trans. R. Soc. Lond. B 364, 1919–1931.
Rubia, K., and Smith, A. (2004). The neural correlates of cognitive time management: a review. Acta Neurobiol. Exp. 64, 329–340.
Schiff, W., Caviness, J. A., and Gibson, J. J. (1962). Persistent fear responses in rhesus monkeys to the optical stimulus of “looming”. Science 136, 982–983.
Skarratt, P. A., Cole, G. G., and Gellatly, A. R. (2009). Prioritization of looming and receding objects: equal slopes, different intercepts. Atten. Percept. Psychophys. 71, 964–970.
Sterzer, P., and Kleinschmidt, A. (2010). Anterior insula activations in perceptual paradigms: often observed but barely understood. Brain Struct. Funct. 214, 611–622.
Stetson, C., Fiesta, M. P., and Eagleman, D. M. (2007). Does time really slow down during a frightening event? PLoS ONE 2, e1295.
Treisman, M. (1963). Temporal discrimination and the difference interval: implications for a model of the “internal clock”. Psychol. Monogr. 77, 1–31.
Tse, P. U., Intriligator, J., Rivest, J., and Cavanagh, P. (2004). Attention and the subjective expansion of time. Percept. Psychophys. 66, 1171–1189.
Ulrich, R., Nitschke, J., and Rammsayer, T. (2006). Perceived duration of expected and unexpected stimuli. Psychol. Res. 70, 77–87.
van der Weel, F. R., and van der Meer, A. L. (2009). Seeing it coming: infants’ brain responses to looming danger. Naturwissenschaften 96, 1385–1391.
van Wassenhove, V. (2009). Minding time – an amodel representational space for time perception. Philos. Trans. R. Soc. Lond. B 364, 1815–1830.
van Wassenhove, V., Buonomano, D. V., Shimojo, S., and Shams, L. (2008). Distortions of subjective time perception within and across senses. PLoS ONE 3, e1437.
Wearden, J. H., and Penton-Voak, I. S. (1995). Feeling the heat: body temperature and the rate of subjective time, revisited. Q. J. Exp. Psychol. B 48, 129–141.
Wicker, B., Ruby, P., Royet, J. P., and Fonlupt, P. (2003). A relation between rest and the self in the brain? Brain Res. Rev. 43, 224–230.
Wittmann, M. (2009a). “The subjective flow of time,” in The Encyclopedia of Time, ed. H. J. Birks (Thousand Oaks: SAGE Publications), 1322–1324.
Wittmann, M. (2009c). Die Neuropsychologie der Zeit – Kognitive und emotionale Modulatoren der zeitlichen Erfahrung. Z. Med. Psychol. 18, 28–39.
Wittmann, M., and Paulus, M. P. (2008). Decision making, impulsivity and time perception. Trends Cogn. Sci. 12, 7–12.
Wittmann, M., Simmons, A. N., Aron, J., and Paulus, M. P. (2010a). Accumulation of neural activity in the posterior insula encodes the passage of time. Neuropsychologia 48, 3110–3120.
Wittmann, M., van Wassenhove, V., Craig, A. D., and Paulus, M. P. (2010b). The neural substrates of subjective time dilation. Front. Hum. Neurosci. 4:2.
Wittmann, M., and van Wassenhove, V. (2009). The experience of time: neural mechanisms and the interplay of emotion, cognition and embodiment. Philos. Trans. R. Soc. Lond. B 364, 1809–1813.
Yantis, S., and Egeth, H. E. (1999). On the distinction between visual salience and stimulus-driven attentional capture. J. Exp. Psychol. Hum. Percept. Perform. 25, 661–676.
Yarrow, K., Haggard, P., Heal, R., Brown, P., and Rothwell, J. C. E. (2001). Illusory perceptions of space and time preserve cross-saccadic perceptual continuity. Nature 414, 302–305.
Yarrow, K., and Rothwell, J. C. E. (2003). Manual chronostasis: tactile perception precedes physical contact. Curr. Biol. 13, 1134–1139.
Keywords: time perception, visual illusion, looming, insular cortex, cingulate cortex, midline structures, self, fMRI
Citation: van Wassenhove V, Wittmann M, Craig AD and Paulus MP (2011) Psychological and neural mechanisms of subjective time dilation. Front. Neurosci. 5:56. doi: 10.3389/fnins.2011.00056
Received: 18 November 2010; Accepted: 01 April 2011;
Published online: 26 April 2011.
Edited by:
Srikantan S. Nagarajan, University of California, San Francisco, USAReviewed by:
David T. Blake, Medical College of Georgia, USACorby L. Dale, University of California San Francisco, USA
Copyright: © 2011 van Wassenhove, Wittmann, Craig and Paulus. This is an open-access article subject to a non-exclusive license between the authors and Frontiers Media SA, which permits use, distribution and reproduction in other forums, provided the original authors and source are credited and other Frontiers conditions are complied with.
*Correspondence: Institute for Frontier Areas of Psychology and Mental Health, Department of Empirical and Analytical Psychophysics, Wilhelmstrasse 3a, Freiburg, 79098, Germany, wittmann@igpp.de