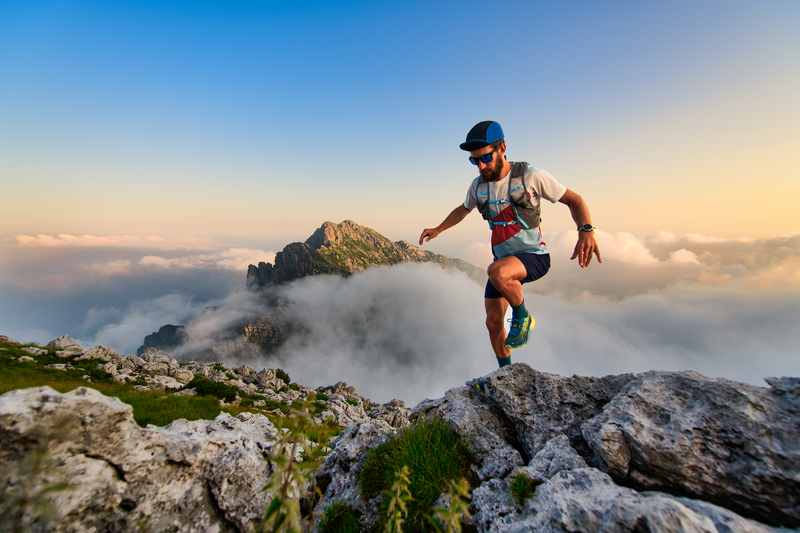
95% of researchers rate our articles as excellent or good
Learn more about the work of our research integrity team to safeguard the quality of each article we publish.
Find out more
ORIGINAL RESEARCH article
Front. Neurorobot. , 31 July 2023
Volume 17 - 2023 | https://doi.org/10.3389/fnbot.2023.1177201
This article is part of the Research Topic Wearable Robots and Sensorimotor Interfaces: Augmentation, Rehabilitation, Assistance or substitution of human sensorimotor function. Volume II View all 5 articles
Introduction: Stroke-related deficits often include motor impairments and gait dysfunction, leading to a limitation of social activities and consequently affecting the quality of life of stroke survivors. Neurorehabilitation takes advantage of the contribution of different techniques in order to achieve more benefits for patients. Robotic devices help to improve the outcomes of physical rehabilitation. Moreover, motor imagery seems to play a role in neurological rehabilitation since it leads to the activation of the same brain areas as actual movements. This study investigates the use of a combined physical and cognitive protocol for gait rehabilitation in stroke patients.
Methods: Specifically, we tested the efficacy of a 5-week training program using a robotic orthosis (P.I.G.R.O.) in conjunction with motor imagery training. Twelve chronic stroke patients participated in the study. We evaluated balance and gait performance before and after the training. Six of them underwent fMRI examination before and after the training to assess the effects of the protocol on brain plasticity mechanisms in motor and imagery tasks.
Results: Our results show that the rehabilitation protocol can effectively improve gait performance and balance and reduce the risk of falls in stroke patients. Furthermore, the fMRI results suggest that rehabilitation is associated with cerebral plastic changes in motor networks.
Discussion: The present findings, if confirmed by future research, have the potential to advance the development of new, more effective rehabilitation approaches for stroke patients, improving their quality of life and reducing the burden of stroke-related disability.
Stroke is a primary cause of disability worldwide, with approximately 15 million people experiencing a stroke each year (Feigin et al., 2017). One of the most debilitating consequences of a stroke is difficulty on walking, or gait dysfunction. Stroke occurs suddenly and may result in major deficits (Stokes, 2004), thus often drastically hampering patients' quality of lives, by leading to a loss of autonomy, and consequently, of social relationships. Stroke survivors are therefore often affected by a series of complex problems on a physical, psychological, and social level. One of the most common and greater needs of a stroke patient is to restore walking ability, since motor deficits often persist after discharge from the hospital (Pennycott et al., 2012) and greatly limit independence in carrying out the activities of daily and social life (Hesse, 2008). The main difficulties in walking for stroke patients are decreased strength, inability to produce voluntary muscle contractions, and inappropriate muscle activity (Perry et al., 1995). Furthermore, a few weeks following brain injury, two additional deficits may occur, such as spasticity and changes in the mechanical properties of the muscles that cause abnormal stretching of the muscle groups (Olney and Richards, 1996). Stroke patients may also present somatosensory and proprioceptive dysfunctions, that prevent them from receiving an adequate sensory feedback from their movements, thus impairing the normal gait cycle (Kessner et al., 2019).
Motor rehabilitation in the neurological field must take into consideration all these different aspects and must be implemented as a multidisciplinary approach to improve impaired functions, reduce symptoms, and increase the personal wellbeing of both the patient and their family, according to the World Health Organization guidelines (WHO). Recovery is a complex process that occurs through a combination of spontaneous and learning-dependent processes. These include restoration of the functionality of the damaged tissue, reorganization of the neural pathways to relearn the lost function, and compensatory mechanisms that help patients interact with the environment through new skills (Langhorne et al., 2011). Neuro-motor rehabilitation techniques are then based on the assumption that the brain can recover or compensate for lost functions through the phenomenon of cerebral plasticity. It has been demonstrated that, while acquiring new skills, cortical regions associated with sensorimotor functions of the body parts most involved in the task gradually start to be represented over larger cortical territories (Pascual-Leone et al., 1994; Kami et al., 1995). Thus, it is likely that, after a brain injury, the sensorimotor experiences of the individual can remodel the structure and function of undamaged parts of the brain, promoting the recovery process (de Diego et al., 2013; Chen and Shaw, 2014). One possibility to support this process is to passively induce lower limb movements. Such passive leg movement has been demonstrated to induce a proprioceptive and kinesthetic activation which provides an afferent input to the supra-spinal motor control centers, stimulating and re-activating these circuits (Rossini et al., 2003; Li et al., 2018).
In recent years, more and more robotic devices have been developed to help the patient regain confidence in gait movements in complete safety (Hobbs and Artemiadis, 2020). These devices can be used in combination with a Body Weight Support (BWS) system, allowing weight relief during patients' movement (Bruni et al., 2018). Several studies have been made to experiment the effectiveness of these devices and some of these seem to give positive results, in association with classical therapy (Hesse, 2008; Mehrholz et al., 2020).
Another factor that seems to play a crucial role in improving motor functions in both healthy subjects and patients during post-stroke rehabilitation is Motor Imagery (from now on, MI) (Sacco et al., 2006; Zimmermann-Schlatter et al., 2008; Li et al., 2018). MI is defined as “an active process during which the representation of an action is internally reproduced within working memory without any overt output” (Decety and Grèzes, 1999). MI seems to be effective for motor rehabilitation both from a behavioral perspective (Jackson et al., 2001), but also for promoting cerebral reorganization in motor rehabilitation of stroke patients (Sun et al., 2013).
Here, we implemented a protocol that combines the use of MI with a robotic exoskeleton, in the context of gait rehabilitation. The robotic orthosis used in this rehabilitation protocol is called Pneumatic Interactive Gait Rehabilitation Orthosis (P.I.G.R.O.) (Belforte et al., 2014; Sacco et al., 2018), it is specifically developed for mimicking natural walking movements, and it can be used in both passive and active modes in order to assist patients during the motor rehabilitation process. The objective of this study is thus to verify whether a combined MI+PIGRO protocol is effective in rehabilitating the gait of chronic stroke patients and whether it can actively support brain plasticity. With this aim, we conducted a 5-week training program combining the use of a robotic orthosis and MI training for gait rehabilitation in chronic stroke patients. We tested behavioral performance before and after the rehabilitation protocol. Following the training, we expected an improvement in gait and balance, assessed by behavioral scales. Furthermore, we expected that our combined rehabilitation protocol may promote brain plasticity. To verify this, before and after the rehabilitation training, we examined areas of cerebral activation while performing foot movement and MI task during fMRI scanning.
Twelve patients with outcomes of chronic ischemic and hemorrhagic stroke (M = 7; age range = 33–75), were recruited at least one year after the cerebral event. Six of them had a right hemisphere lesion, while the other six had a left hemisphere lesion (for a broad description of lesions see Table 1). They were preliminary tested by a cognitive screening to determine if exclusion criteria were present. They we excluded in case of cognitive deterioration, aphasia, psychiatric illness or severe behavioral changes, drug or alcohol abuse, severe deficits in one or more of the following areas: visual, auditory, attentional, reasoning, language comprehension, presence of neglect, apraxia. Physical inclusion criteria were hemiparesis in the lower limbs, unstable gait but preservation of the ability to stand independently or with assistance. Physical exclusion criteria were the presence of peripheral neuropathies of the lower limbs, spinal lesions, previous central nervous system disease, and severe orthopedic impairment. Seven subjects were recruited from the ASL of Fossano (CN) and five were recruited from the Puzzle center in Turin.
The research has been conducted in accordance with the World Medical Association's Declaration of Helsinki. It has been performed in accordance with national guidelines and with the prior approval of the Ethics committee of “AOU Città della Salute e della Scienza di Torino”. The patients provided their written informed consent to participate in this study.
The robotic orthosis used in this rehabilitation protocol is called P.I.G.R.O. and it was developed in collaboration between the Department of Psychology and the Department of Mechanical and Aerospace Engineering of the Polytechnic University of Turin. PIGRO consists of a modular and size-adaptable 6-DoF exoskeleton that can be adapted to patients with an anthropometric range between 10%ile woman and 95%ile man and is designed to reproduce the physiological movements of walking. It consists of two independent “legs” composed of three parts: Hip joint, femoral segment and tibial segment, which are adjustable in length according to the patient's measurements and involves six joints (two for the hip, knee and ankle) that can be monitored (Figure 1). Part of the orthosis structure uses spring steel so it is more comfortable and wearable (Belforte et al., 2014).
Figure 1. (A) P.I.G.R.O. orthosis used in the rehabilitation training. (B) P.I.G.R.O. operator's monitor screenshot with the six joints visualized. (C) Examples of biofeedback monitor. In particular, the thicker curve is the machine reference for the patient, while the thinner curve represents the patient's performance during the test.
It can be used by patients either being completely passive, which is typically the case at the beginning of rehabilitation training, and then gradually active, which means that patients must gradually exert more effort and strength to perform the movement. The orthosis was used in conjunction with a Body Weight Support (BWS) system, allowing for complete weight loss of the mass of the orthosis itself and of the patients' body. The BWS provided during training was initially set to fully support the subjects' weight and gradually adjusted based on the individual's abilities. Therefore, rehabilitation training could be performed in complete suspension to avoid spasticity of the lower limbs, allowing the passive leg movement that induce a proprioceptive and kinesthetic activation. To ensure that the movement of the machine was transmitted and perceived correctly by the patient, it was necessary for the exoskeleton and the subject to adhere well to each other. This was guaranteed by the presence of orthopedic splints and Velcro straps along each joint and by a fabric corset on the abdominal area. The corset was divided into two independent sections, each one connected to a leg. If necessary, pads could be added to the parts in contact with the patient to improve comfort during use in suspension (Figure 1A).
The operator has at his disposal a computer with two monitors to control the machine: from the screen of the main one he can set the speed of the step cycle and adjust the pressure independently in the two limbs (to allow passive or active movement of the patient), it can start, pause and stop the movement, as well as being able to enter all the information related to the patient and the session in progress; moreover, in this window it is possible to observe the motion curves set in the machine for each articulation (Figure 1B). Specifically, two motion curves were observed during the participant movement for each articulation: one represents the movement run by the machine and the other represents the movement performed by the patient (Figure 1C). Those two curves were superimposed to allow an online comparison and a possible correction by the operator. In addition, participants were shown the motion curve of one joint of one or both legs at a time on a monitor to make them constantly aware of their motion performance through visual biofeedback and to allow them to better associate the correct movement with the proprioceptive information.
The experimental protocol proposed consisted of two types of training: physical, which combined classic physiotherapeutic rehabilitation and rehabilitation with robotic devices; and cognitive, which included rehabilitation through motor imagination and focus of attention.
Patients underwent three sessions of physical and cognitive assessment. A first evaluation was made 5 weeks before the start of treatment (T0), one at the beginning of the treatment (T1), and one at the end of the treatment (T2). Concerning the physical evaluation the following tests were performed: Berg Balance Scale (BBS) (Berg et al., 1992) and Tinetti Balance and Gait Scale (TBGS) (Tinetti et al., 1986). Moreover, a neuropsychological evaluation was performed in order to check for the exclusion criteria and assess the preserved imagery ability. In T1 and T2 compatible subjects underwent a functional magnetic resonance examination (Figure 2).
We used two of the most common scales (i.e., BBS and TBGS) that can provide an objective measure of walking ability from a behavioral perspective. Both scales were performed by a physical therapist at each of the experimental time points (T0, T1, and T2). BBS is used to assess balance and fall risk in both elderly healthy subjects and patients with various diseases (stroke, Alzheimer's disease, dementia, multiple sclerosis, Parkinson's disease). It consists of 14 items that examine the patient's ability to hold a position, move from one position to another, and complete a task. BBS scores are categorized according to the performance of functional status and independence into three levels: (a) 0 to 20, available mobility although wheelchair bound; (b) 21 to 40, walking with assistance; and (c) 41 to 56, walking independently (Berg et al., 1992). The TBGS assesses balance and gait, key factors in predicting fall risk, by evaluating motor skills that are important for daily living, such as standing up and sitting down. It consists of two different scales: the first part, which evaluates balance functions, consists of 9 tests that examine static balance abilities in different positions and the execution of stance changes. The second part, on the other hand, evaluates walking functions based on gait characteristics such as symmetry, step length, and continuity, using 10 items.
Neuropsychological assessment includes evaluation of various cognitive domains and has been used to screen exclusion criteria. It includes the Mini Mental State Examination (MMSE) (Folstein et al., 1975), a neuropsychological test used to assess intellectual performance and the presence of cognitive impairment; the Token Test (short form of the Achen Aphasia Test) (De Bleser et al., 1986), used to assess some aspects of oral language comprehension and check for aphasia; Albert's Test (Fullerton et al., 1986), which is used to determine the presence of unilateral spatial neglect and it is specific for stroke patients; Frontal Assessment Battery (FAB) (Hurtado-Pomares et al., 2018), which is used to examine global executive functions at cognitive and behavioral levels. Finally, Short Form 36 Health Survey Questionnaire (SF-36) is a self-administered test that was used to capture the impact of a disease on various dimensions of quality of life such as physical functioning, limitations due to physical health, limitations due to emotional problems, energy and fatigue, emotional wellbeing, social activities, pain, and perception of general health. Moreover, the preserved imagery ability was evaluated through the use of Kinesthetic and Visual Imagery Questionnaire (KVIQ) (Malouin et al., 2007), a motor imagination questionnaire adapted for patients who are unable to stand or perform complex movements, in which both visual and kinesthetic dimensions of motor imagination are tested, and the Vividness of Visual Imagery Questionnaire (VVIQ) (McKelvie, 1995), which explores the vividness of visual imagination.
The rehabilitation protocol consisted of three sessions per week over a 5-week period. Each session lasted 1 hour and a half and was divided into three parts: The first took place on the robotic orthosis (30 min), in the second a motor imagery guided practice was performed, and in the last part the patients were asked to walk on the floor with the help of a physiotherapist. In addition, between sessions the subject was instructed to do motor imagery exercises at home, reinforcing the work done with the therapists. The detailed program of each session of the rehabilitation protocol are briefly described and summarized in Figure 3. The 15 sessions were divided into blocks: after getting used to the exoskeleton and the protocol in general, they focused on a specific joint (in the order hip-knee-ankle). The movement of each joint on the floor was presented and explained in its dedicated session. The movement curves in suspension were presented so that patients could perform them. When performing the movement, patients were asked to focus their attention first on the passive movement (i.e., when the pressure in the legs is high: 6 bar, the patient must not make efforts and must concentrate on the movement performed by the machine) and then on the active movement (i.e., when the pressure in the legs is low: 4 or 2 bar). In this active part, patients were asked to try to reproduce the movement, relying mainly on proprioception, but also on the verbal feedback from the psychologist, which becomes less and less as the rehabilitation progresses, and on visual feedback when needed. Even though the protocol consists of precise phases and tasks, sessions could vary according to the different needs of each patient. In particular, pressure could be changed from 4 to 2 bar, and a different amount of time, in a range between 1 and 3 min, could be dedicated to a specific joint, depending on patients' capabilities. We opted for a compromise between a reproducible protocol assuring methodological rigor and the respect for individual clinical needs: although it can be considered as a limitation of the study, tailorizing protocols represents a current gold standard in medicine and rehabilitation.
Figure 3. Aims and description of the rehabilitation protocol, organized in three sessions per week over a period of 5 weeks.
Eligible subjects (N = 6) underwent two sessions of fMRI examination in T1 and T2. All the subjects have a left hemisphere lesion. The task adopted in this study was inspired by the task used by Dobkin et al. (2004) for rehabilitation of ankle joint movement, as ankle movement appears to activate very similar brain networks to those activated during gait (Sahyoun et al., 2004).
The task was performed using a block design with 12 s of rest alternating with 12 s of active condition. Throughout the session, an image of two feet was projected: In the resting phase, the feet were white and the patients had to remain still, while in the active phase, the right or the left foot turned red and they had to move the corresponding foot in the first run, or imagine moving it in second run, performing plantar flexion and then dorsiflexion, like they were pressing a pedal.
Data acquisition was performed at the Koelliker hospital in Turin on a 1.5 T Intera scanner (Philips Medical Systems). Structural and functional images were acquired for each patient. In the first part of the exam a set of three-dimensional high-resolution T1-weighted structural images was acquired (FFE sequence, TR = 25 ms, TE = shortest, flip angle = 30°, acquisition matrix = 288 × 288, FoV = 288 mm). The set consisted of 107 sagittal contiguous images (slice thickness = 1,5 mm) covering the whole brain with an in-plane resolution of 0.99 mm × 0.99 mm. Functional T2-weighted images were acquired using echoplanar (EPI) sequences (TR = 3,000 ms, TE = 60 ms, flip angle = 90°, acquisition matrix = 64 × 64; FoV = 256 mm. For each task, a total of 206 volumes were acquired. Each volume consisted of 25 axial slices (slice thickness = 4 mm with a 0.5-mm gap), parallel to the anterior–posterior (AC–PC) commissure line and covering the whole brain.
Analyses for fMRI data were performed using the AFNI (Cox, 1996) and FSL (Jenkinson et al., 2012) software programs. Structural images were brain extracted, corrected for intensity bias, and spatially normalized to the Montreal Neurological Institute (MNI) space with non-linear registration. All functional volumes were slice timing corrected, spatially realigned to the first volume of the functional acquisition, and correct for scan motion. All functional volumes are then spatially smoothed with a 6 mm full-width half-maximum isotropic Gaussian kernel (FWHM) and the signal was normalized (center: 0; variations in %). Spatial parameters are computed for aligning average EPIs to high resolution T1w and then re-sample in the size of the functional acquisition (4 × 4 × 4.5 mm3) using a weighted sinc-interpolation method.
The fMRI responses of each subject were modeled using the General Linear Model (GLM). The design matrix of the GLM included the onset and duration of each experimental condition (12s) as well as the six predictors obtained from the motion correction parameters in the realignment process to account for the voxel intensity variations caused by the subjects' head movements. The conditions predictors were modeled as consecutive and convolved with a double gamma hemodynamic Hemodynamic Response Function (HRF).
As regard the group level analysis, results were transformed into standard space, a common brain mask was created (including all the standardized masks for each subject) and a paired-sample t-test was performed in order to compare activity before and after the rehabilitation protocol (T0 vs. T2) for each foot.
A repeated measure ANOVA was used to compare pre-training with post-training on BBS and TBGS scores. The BBS was used to assess balance and stability of walking, and the TBGS was used to measure movements, postural changes and walking aspects related to a safe and efficient execution of the activities of daily living. ANOVA results showed a main effect on time (T0 vs. T1 vs. T2) in both tests (BBS: F = 7.5, p < 0.05; TBGS: F = 5.8, p < 0.05) so a paired-sample t-test was performed. Results are shown in Figures 4, 5. Results of t-test show on both scales that the group of patients (N = 12) presents an absence of changes between T0 (baseline) (BBS mean = 32.2 ± 8.5; TBGS mean = 16.8 ± 3.4) and T1 (BBS mean= 32.2 ± 9; TBGS mean = 17 ± 3.8, p > 0.05), confirming the stability of their conditions. On the contrary, a statistically significant improvement emerged between T1 (pre-training) and T2 (post-training) (BBS mean = 36.89 ± 8.5; TBGS mean = 20.8 ± 2.8) showing that our training positively affected their balance (p < 0.05).
Figure 4. BBS results in T0 (mean = 32; SD = 8.5), T1 (mean = 32.2; SD = 9) and T2 (mean = 36.8; SD = 8.5). *p < 0.05.
Figure 5. TBGS results in T0 (mean = 16.8; SD = 3.4), T1 (mean = 17; SD = 3.8) and T2 (mean = 20.8; SD = 2.8). *p < 0.05.
Considering the size of fMRI sample (N = 6), the high variability of people's brain plasticity mechanism and of the lesions of participants in terms of their extent and location, we considered results with an uncorrected p value ≤ 0.05. This is a major limitation of the study. However, we still believe that the results obtained by fMRI analysis, represent a preliminary though promising finding, which may be an interesting starting point for future research.
A paired-samples t-test was performed to compare pretraining activity with post-training activity. The results of the paired-samples t-test on right foot motor activity comparing pre- and post-rehabilitation time at the group level (N = 6) show greater activation of activity after training compared to pre-training time in the bilateral precuneus, right angular gyrus (AG), and bilateral superior and inferior parietal lobule (SPL, IPL) (uncorrected p ≤ 0.05). The map of the threshold results is shown in Figure 6. Results are clustered for groups of voxels ≥10. In addition, the results are summarized in Table 2. No activation was found for the left foot using the same statistics.
Figure 6. Group level activation in motor activity in post- minus pre- rehabilitation of right foot. Each activation is fully described in Table 2 (Left=Left).
The paired-sample t-test results on imagery activity comparing pre- vs. post- training at group level reveal a greater activation of those areas involved in motor execution and motor planning, especially when considering the right foot activity. In particular, precentral and postcentral gyrus, paracentral lobule, Supplementary Motor Area (SMA), and parietal lobules. Imagery results are summarized in Tables 3, 4.
In the present study, we tested a combined (physical & cognitive) protocol, directed to rehabilitate the gait of chronic stroke patients, using an original robotic exoskeleton, called P.I.G.R.O. The results suggest a significant overall improvement in gait performance associated with possible brain reorganization.
From a behavioral point of view, although we do not have certainty of durability over the long term, the BBS and TBGS scores showed an improvement in balance and walking ability after the rehabilitation. As indicated in Figures 4, 5, the group of 12 patients demonstrated stability in their conditions between the baseline (T0) and pre-treatment (T1) assessments on both scales. The BBS mean scores in T0 and T1 indicated that patients needed mobility assistance (Neuls et al., 2011). Although the mean score at T2 is still below the threshold of good balance (41/56) (Blum and Korner-Bitensky, 2008), indicating the inability to move safely, a significant improvement was observed between the pre-training (T1) and post-training (T2) assessments. These findings show that our training had a positive impact on patients' balance. Moreover, prior to training, the patients scored below the cutoff value (cutoff = 18) on the TBGS scale. Since this scale is highly sensitive to fall risk, values over the cutoff reflect the patients' high risk of falling, indicating insufficient efficiency and stability in their motor activities (Scura and Munakomi, 2023). After the training, their score exceeded the cutoff value, showing that they had recovered mobility to the point of significantly reducing the risk of falling. Altogether, these behavioral results indicate that the combined use of MI associated with a motor rehabilitation plan can be an effective protocol in inducing a motor performance improvement.
The imagination of a movement may be realized from two different perspectives: through an external/allocentric perspective (in the third person), or internally, through an egocentric perspective (in the first person) (Malouin et al., 2007). When individuals intentionally focus their attention on imaging to perform a movement in the first person, as realized in the present protocol, the mental representation of such movement is activated, supporting the reconstruction of its motor schema (Jeannerod, 1994; Sacco et al., 2011). The focus of attention has been suggested to be a crucial aspect in inducing neuroplasticity (Li et al., 2018). Moreover, the visual feedback plays an important role helping the construction of the proprioceptive experience (Ernst and Bülthoff, 2004). Although visual feedback in 3D might have been more effective (Song et al., 2015), the 2D online feedback here proposed helps to better associate movement with proprioceptive sensation by providing visual support in processing somatosensory feedback. Future studies could explore this further, for example, with the help of Virtual Reality devices, which has proven useful in many aspects of rehabilitation to make settings as ecologic as possible (Semblantes et al., 2018; Kim et al., 2020).
Importantly, our results are in line with previous studies, showing that the use of MI in motor rehabilitation protocols is effective in supporting the rehabilitation of motor deficits (Jackson et al., 2001; Dunsky et al., 2008; Zhang et al., 2011). It has been shown that stroke patients undergoing MI associated with motor-based therapy showed significant improvements in gross motor function of upper limbs (Machado et al., 2019). Moreover, a similar protocol that combined sensory-motor training and MI tested in a study with two cranial trauma patients, provided promising results in restoring balance and gait as well as enhancing connectivity in the motor network (Sacco et al., 2011). Although we cannot isolate the contribution of MI because we do not have a specific control group, we suspect, in agreement with previous studies, that this is one of the factors that contribute to the results since it is an integral and important part of the rehabilitation protocol.
In line with previous studies, the present training program seems to be able to improve patients' functional abilities, reducing their risk of falls. This may allow them to perform activities of daily living more safely and efficiently increasing independence in daily and social activities. Accordingly, the employment of a combined MI+P.I.G.R.O. protocol may have important implications for the quality of life and overall wellbeing of patients and their families. However, it cannot be speculated whether these improvements remain constant in the long term, i.e., beyond the 5 weeks considered in our study.
From a neurophysiological perspective, the improved gait performance appeared to be supported by a brain reorganization in the lesioned hemisphere during the motor task, particularly in higher-level motor networks. fMRI results show greater activation in the bilateral pre-cuneus, right AG, and bilateral SPL and IPL, when compared to before training activation for the contralateral side (i.e., right foot). Given the connectivity between the pre-cuneus and M1 (Zhang et al., 2011), its increased activation may reflect a general enhancement of the cerebral network involved in movement-related activities. More specifically, previous studies demonstrated the pre-cuneus involvement in higher-order aspects of action and its function as an interface between cognition and action, as described by Wenderoth et al. (2005). Similarly, AG acts as an integrative hub for multisensory information due to its rich connectivity. Previous studies have reported the role of AG during the learning process (Seghier, 2013), including motor skills learning (Draganski et al., 2004; Amad et al., 2017). More specifically, AG is one of the brain areas showing greater plasticity when learning challenging visuomotor tasks (such as, juggling), and, as observed in a resting state connectivity study, it was demonstrated to be included in a neural network deeply involved in motor training skills (Draganski et al., 2004; Amad et al., 2017). Moreover, activation in the bilateral SPL may reflect the enhanced kinesthetic process. SPL appears to be involved in another high-level mechanism, that compares the spatial position and orientation of the stored representation of the motor plan with afferent signals (Wolbers et al., 2003). Remarkably, no cerebral activation was observed during left foot movement in the post- minus pre- contrast, suggesting that cerebral reorganization occurred only in the lesioned hemisphere. Taken together, this evidence, when compared with the current literature, seems to indicate that the brain changes associated with motor activity occur in areas involved in higher-level aspects of motor control, that is, motor integration and association.
Importantly, looking at the MI activity results for the right foot, it seems that MI training effectively stimulates the motor networks that we expected to be involved in rehabilitation. The brain activity during the imagery task seems to be enhanced by the training, especially for the lesioned hemisphere. Although the involvement of primary motor cortex in MI has been considered controversial by the literature (Dechent et al., 2004), recent studies exploiting transcranial magnetic stimulation indicated that MI is able to improve primary motor cortex plasticity in healthy subjects (Avanzino et al., 2015). Apparently, motor imagery leads to the activation of the same brain areas as actual movement both in healthy subjects and in neurological patients (Hallett et al., 1994; Gerardin et al., 2000; Hanakawa et al., 2003; Kimberley et al., 2006; Confalonieri et al., 2012). Importantly our results may be considered as supporting evidence to this hypothesis. In particular, we found activation of primary motor and sensory cortices, paracentral lobule, which controls motor and sensory innervation of the contralateral lower extremity, SMA, involved in planning motor sequence and movement execution, and parietal lobules, responsible for transforming motor information into motor commands. As suggested by previous works, a possible interpretation is that imagery training promotes the kinesthetic sensation of movement and allows better activation of processes involved in the motor task, serving as a primer that stimulates the actual motor network (Jackson et al., 2001). A recent TMS work provides further proofs toward the role of imagery in motor learning and plasticity assuming its actual occurrence at cortical level (Ruffino et al., 2019). In conclusion, although we do not have a control group to verify the actual specific contribution of MI, we suggest that imagery training integrated into the rehabilitation protocol could be one of the factors that affected motor rehabilitation in our sample.
Overall, our preliminary findings suggest that the described rehabilitation protocol, matching the employment of P.I.G.R.O. exoskeleton with the use of MI, it can be effective in rehabilitating gait performance in chronic stroke patients and in supporting changes in the cerebral network involved in movement. However, given the sample size of the present research, further studies are needed to confirm the results. Ultimately, this research has the potential to support the development of new multidisciplinary approaches for stroke patients, improving their quality of life, and reducing the burden of stroke-related disability. Future research should further investigate the role of lesion extent and location in the recovery and the use of motor imagery in the context of stroke patient rehabilitation.
The raw data supporting the conclusions of this article will be made available by the authors, without undue reservation.
The studies involving human participants were reviewed and approved by AOU Città della Salute e della Scienza di Torino. The patients/participants provided their written informed consent to participate in this study.
KS and GG conceptualized the work and the experimental design. KS, MZ, and SD contributed to patients' screening protocol and data acquisition. M-CV, KS, IR, and AC contributed to data analysis. M-CV, IR, and KS wrote the first draft of the manuscript. All authors contributed to manuscript revision, read, and approved the submitted version.
This research was supported by PNRR Innovation Ecosystems, Project NODES, Spoke 5 Industry for health and silver economy, Objective Development of innovative and digital strategies for the care of frail subjects, 2022–2025.
We thank Eng. Guido Belforte, Gabriella Eula, Silvia Appendino, and Silvia Sirolli (Polytechnic of Turin) for designing and developing the robotic orthosis; Gianfranco Lamberti (Former Director @Neurorehabilitation Unit, Fossano) for making available clinical infrastructures and patients' demographics; Dumitru Scafesi (Senior MRI Technician @Koelliker Hospital) for optimizing MR sequences; Roberta Virgilio (Master's degree Psychologist) for assisting patients while using the orthosis; and Elisabetta ML Geda and Marzia Schinello (Master's degree Psychologists) for screening and assessing patients'. We deeply thank all the participants and their caregivers.
The authors declare that the research was conducted in the absence of any commercial or financial relationships that could be construed as a potential conflict of interest.
All claims expressed in this article are solely those of the authors and do not necessarily represent those of their affiliated organizations, or those of the publisher, the editors and the reviewers. Any product that may be evaluated in this article, or claim that may be made by its manufacturer, is not guaranteed or endorsed by the publisher.
Amad, A., Seidman, J., Draper, S. B., Bruchhage, M. M. K., Lowry, R. G., Wheeler, J., et al. (2017). Motor learning induces plasticity in the resting brain—drumming up a connection. Cerebral Cortex 27, 2010–2021. doi: 10.1093/cercor/bhw048
Avanzino, L., Gueugneau, N., Bisio, A., Ruggeri, P., Papaxanthis, C., and Bove, M. (2015). Motor cortical plasticity induced by motor learning through mental practice. Front. Behav. Neurosci. 9, 105. doi: 10.3389/fnbeh.2015.00105
Belforte, G., Eula, G., Sirolli, S., Bois, P., Geda, E., D'Agata, F., et al. (2014). Bra.Di.P.O. and P.I.G.R.O.: innovative devices for motor learning programs. J. Robot. 2014, e656029. doi: 10.1155/2014/656029
Berg, K. O., Wood-Dauphinee, S. L., Williams, J. I., and Maki, B. (1992). Measuring balance in the elderly: Validation of an instrument. Revue Canadienne De Sante Publique. 83 Suppl 2, S7–11.
Blum, L., and Korner-Bitensky, N. (2008). Usefulness of the berg balance scale in stroke rehabilitation: a systematic review. Phys. Ther. 88, 559–566. doi: 10.2522/ptj.20070205
Bruni, M. F., Melegari, C., De Cola, M. C., Bramanti, A., Bramanti, P., and Calabr,ò, R. S. (2018). What does best evidence tell us about robotic gait rehabilitation in stroke patients: A systematic review and meta-analysis. J. Clin. Neurosci. 48, 11–17. doi: 10.1016/j.jocn.2017.10.048
Chen, J.-C., and Shaw, F.-Z. (2014). Progress in sensorimotor rehabilitative physical therapy programs for stroke patients. World J. Clin. Cases 2, 316–326. doi: 10.12998/wjcc.v2.i8.316
Confalonieri, L., Pagnoni, G., Barsalow, L., Rajendra, J., and Butler, A. J. (2012). Brain activation in primary motor and somatosensory cortices during motor imagery correlates with motor imagery ability in stroke patients. ISRN Neurol. 2012, 613595. doi: 10.5402/2012/613595
Cox, R. W. (1996). AFNI: software for analysis and visualization of functional magnetic resonance neuroimages. Comput. Biomed. Res. 29, 162–173. doi: 10.1006/cbmr.1996.0014
De Bleser, R., Denes, G. F., Luzzati, C., and Mazzucchi, A. (1986). L'Aachener Aphasie Test (AAT): I. Problemi e soluzioni per una versione italiana del Test e per uno studio crosslinguistico dei disturbi afasici. [The Aachen Aphasia Test: I. Problems and solutions for an Italian version of the test and for a cross-linguistic study of aphasic disturbances.]. Arch. Psicol. Neurol. Psichiatr. 47, 209–237.
de Diego, C., Puig, S., and Navarro, X. (2013). A sensorimotor stimulation program for rehabilitation of chronic stroke patients. Restor. Neurol. Neurosci. 31, 361–371. doi: 10.3233/RNN-120250
Decety, J., and Grèzes, J. (1999). Neural mechanisms subserving the perception of human actions. Trends Cogn. Sci. 3, 172–178. doi: 10.1016/S1364-6613(99)01312-1
Dechent, P., Merboldt, K.-D., and Frahm, J. (2004). Is the human primary motor cortex involved in motor imagery? Cogn. Brain Res. 19, 138–144. doi: 10.1016/j.cogbrainres.2003.11.012
Dobkin, B. H., Firestine, A., West, M., Saremi, K., and Woods, R. (2004). Ankle dorsiflexion as an fMRI paradigm to assay motor control for walking during rehabilitation. NeuroImage 23, 370–381. doi: 10.1016/j.neuroimage.2004.06.008
Draganski, B., Gaser, C., Busch, V., Schuierer, G., Bogdahn, U., and May, A. (2004). Neuroplasticity: Changes in grey matter induced by training. Nature 427, 311–313. doi: 10.1038/427311a
Dunsky, A., Dickstein, R., Marcovitz, E., Levy, S., and Deutsch, J. (2008). Home-based motor imagery training for gait rehabilitation of people with chronic poststroke hemiparesis. Arch. Phys. Med. Rehabil. 89, 1580–1588. doi: 10.1016/j.apmr.2007.12.039
Ernst, M. O., and Bülthoff, H. H. (2004). Merging the senses into a robust percept. Trends Cogn. Sci. 8, 162–169. doi: 10.1016/j.tics.2004.02.002
Feigin, V. L., Norrving, B., and Mensah, G. A. (2017). Global burden of stroke. Circ. Res. 120, 439–448. doi: 10.1161/CIRCRESAHA.116.308413
Folstein, M. F., Folstein, S. E., and McHugh, P. R. (1975). Mini-mental state. A practical method for grading the cognitive state of patients for the clinician. J. Psychiatr. Res. 12, 189–198. doi: 10.1016/0022-3956(75)90026-6
Fullerton, K. J., Mcsherry, D., and Stout, R. W. (1986). Albert's test: a neglected test of perceptual neglect. Lancet 327, 430–432. doi: 10.1016/S0140-6736(86)92381-0
Gerardin, E., Sirigu, A., Lehéricy, S., Poline, J.-B., Gaymard, B., Marsault, C., et al. (2000). Partially overlapping neural networks for real and imagined hand movements. Cerebral Cortex 10, 1093–1104. doi: 10.1093/cercor/10.11.1093
Hallett, M., Fieldman, J., Cohen, L. G., Sadato, N., and Pascual-Leone, A. (1994). Involvement of primary motor cortex in motor imagery and mental practice. Behav. Brain Sci. 17, 210–210. doi: 10.1017/S0140525X00034130
Hanakawa, T., Immisch, I., Toma, K., Dimyan, M. A., Van Gelderen, P., and Hallett, M. (2003). Functional properties of brain areas associated with motor execution and imagery. J. Neurophysiol. 89, 989–1002. doi: 10.1152/jn.00132.2002
Hesse, S. (2008). Treadmill training with partial body weight support after stroke: A review. NeuroRehabilitation 23, 55–65. doi: 10.3233/NRE-2008-23106
Hobbs, B., and Artemiadis, P. (2020). A review of robot-assisted lower-limb stroke therapy: unexplored paths and future directions in gait rehabilitation. Front. Neurorobot. 14, 19. doi: 10.3389/fnbot.2020.00019
Hurtado-Pomares, M., Carmen Terol-Cantero, M., Sánchez-Pérez, A., Peral-Gómez, P., Valera-Gran, D., and Navarrete-Muñoz, E. M. (2018). The frontal assessment battery in clinical practice: A systematic review. Int. J. Geriatr. Psychiat. 33, 237–251. doi: 10.1002/gps.4751
Jackson, P. L., Lafleur, M. F., Malouin, F., Richards, C., and Doyon, J. (2001). Potential role of mental practice using motor imagery in neurologic rehabilitation. Arch. Phys. Med. Rehabilit. 82, 1133–1141. doi: 10.1053/apmr.2001.24286
Jeannerod, M. (1994). The representing brain: Neural correlates of motor intention and imagery. Behav. Brain Sci. 17, 187–202. doi: 10.1017/S0140525X00034026
Jenkinson, M., Beckmann, C. F., Behrens, T. E. J., Woolrich, M. W., and Smith, S. M. (2012). FSL. NeuroImage 62, 782–790. doi: 10.1016/j.neuroimage.2011.09.015
Kami, A., Meyer, G., and Jezzard, P. (1995). Functional MRI evidence for adult motor cortex plasticity during motor skill learning. Nature. 377, 155–8. doi: 10.1038/377155a0
Kessner, S. S., Schlemm, E., Cheng, B., Bingel, U., Fiehler, J., Gerloff, C., et al. (2019). Somatosensory deficits after ischemic stroke. Stroke 50, 1116–1123. doi: 10.1161/STROKEAHA.118.023750
Kim, W.-S., Cho, S., Ku, J., Kim, Y., Lee, K., Hwang, H.-J., et al. (2020). Clinical application of virtual reality for upper limb motor rehabilitation in stroke: review of technologies and clinical evidence. J. Clin. Med. 9, 3369. doi: 10.3390/jcm9103369
Kimberley, T. J., Khandekar, G., Skraba, L. L., Spencer, J. A., Van Gorp, E. A., and Walker, S. R. (2006). Neural substrates for motor imagery in severe hemiparesis. Neurorehabilit. Neural Repair 20, 268–277. doi: 10.1177/1545968306286958
Langhorne, P., Bernhardt, J., and Kwakkel, G. (2011). Stroke rehabilitation. Lancet 377, 1693–1702. doi: 10.1016/S0140-6736(11)60325-5
Li, M., Xu, G., Xie, J., and Chen, C. (2018). “A review: Motor rehabilitation after stroke with control based on human intent,” in Proceedings of the Institution of Mechanical Engineers, Part H: Journal of Engineering in Medicine 344–360. doi: 10.1177/0954411918755828
Machado, T., Carregosa, A., Santos, M., da Silva Ribeiro, N. M., and Melo, A. (2019). Efficacy of motor imagery additional to motor-based therapy in the recovery of motor function of the upper limb in post-stroke individuals: A systematic review. Top Stroke Rehabil. 26, 548–553. doi: 10.1080/10749357.2019.1627716
Malouin, F., Richards, C. L., Jackson, P. L., Lafleur, M. F., Durand, A., and Doyon, J. (2007). The kinesthetic and visual imagery questionnaire (KVIQ) for assessing motor imagery in persons with physical disabilities: a reliability and construct validity study. J. Neurol. Phys. Ther. 31, 20. doi: 10.1097/01.NPT.0000260567.24122.64
McKelvie, S. J. (1995). The VVIQ as a psychometric test of individual differences in visual imagery vividness: A critical quantitative review and plea for direction. J. Mental Imagery 19, 1–106.
Mehrholz, J., Thomas, S., Kugler, J., Pohl, M., and Elsner, B. (2020). Electromechanical-assisted training for walking after stroke. Cochr. Datab. System. Rev. 10, CD006185. doi: 10.1002/14651858.CD006185.pub5
Neuls, P. D., Clark, T. L., Van Heuklon, N. C., Proctor, J. E., Kilker, B. J., Bieber, M. E., et al. (2011). Usefulness of the Berg Balance Scale to predict falls in the elderly. J. Geriatr. Phys. Ther. 34, 3–10. doi: 10.1097/JPT.0b013e3181ff2b0e
Olney, S. J., and Richards, C. (1996). Hemiparetic gait following stroke. Part I: Characteristics. Gait Posture 4, 136–148. doi: 10.1016/0966-6362(96)01063-6
Pascual-Leone, A., Grafman, J., and Hallett, M. (1994). Modulation of cortical motor output maps during development of implicit and explicit knowledge. Science 263, 1287–1289. doi: 10.1126/science.8122113
Pennycott, A., Wyss, D., Vallery, H., Klamroth-Marganska, V., and Riener, R. (2012). Towards more effective robotic gait training for stroke rehabilitation: A review. J. NeuroEng. Rehabilit. 9, 65. doi: 10.1186/1743-0003-9-65
Perry, J., Garrett, M., Gronley, J., and Mulroy, S. (1995). Classification of walking handicap in the stroke population. Stroke. 26, 982–9. doi: 10.1161/01.STR.26.6.982
Rossini, P. M., Calautti, C., Pauri, F., and Baron, J.-C. (2003). Post-stroke plastic reorganisation in the adult brain. Lancet Neurol. 2, 493–502. doi: 10.1016/S1474-4422(03)00485-X
Ruffino, C., Gaveau, J., Papaxanthis, C., and Lebon, F. (2019). An acute session of motor imagery training induces use-dependent plasticity. Sci. Rep. 9, 20002. doi: 10.1038/s41598-019-56628-z
Sacco, K., Belforte, G., Eula, G., Raparelli, T., Sirolli, S., Geda, E., et al. (2018). “PIGRO: An active exoskeleton for robotic neurorehabilitation training driven by an electro-pneumatic control,” in Advances in Service and Industrial Robotics: Proceedings of the 26th International Conference on Robotics in Alpe-Adria-Danube Region, RAAD 2017, 845–853. doi: 10.1007/978-3-319-61276-8_89
Sacco, K., Cauda, F., Cerliani, L., Mate, D., Duca, S., and Geminiani, G. C. (2006). Motor imagery of walking following training in locomotor attention. The effect of ‘the tango lesson'. NeuroImage 32, 1441–1449. doi: 10.1016/j.neuroimage.2006.05.018
Sacco, K., Cauda, F., D'Agata, F., Duca, S., Zettin, M., Virgilio, R., et al. (2011). A combined robotic and cognitive training for locomotor rehabilitation: evidences of cerebral functional reorganization in two chronic traumatic brain injured patients. Front. Hum. Neurosci. 5, 146. doi: 10.3389/fnhum.2011.00146
Sahyoun, C., Floyer-Lea, A., Johansen-Berg, H., and Matthews, P. M. (2004). Towards an understanding of gait control: Brain activation during the anticipation, preparation and execution of foot movements. NeuroImage 21, 568–575. doi: 10.1016/j.neuroimage.2003.09.065
Scura, D., and Munakomi, S. (2023). “Tinetti gait and balance test,” in StatPearls (StatPearls Publishing).
Seghier, M. L. (2013). The angular gyrus: multiple functions and multiple subdivisions. Neuroscientist 19, 43–61. doi: 10.1177/1073858412440596
Semblantes, P. A., Andaluz, V. H., Lagla, J., Chicaiza, F. A., and Acurio, A. (2018). Visual feedback framework for rehabilitation of stroke patients. Inform. Med. Unlocked 13, 41–50. doi: 10.1016/j.imu.2018.10.002
Song, W.-K., Cho, K.-H., Jeong, J. Y., Kim, Y., Kim, J. Y., and Shin, J.-H. (2015). “Reaching contralateral target by chronic hemiparetic stroke survivors using active-assisted/active exercise with 2D/3D visual feedback,” in 2015 IEEE International Conference on Rehabilitation Robotics (ICORR) 913–918. doi: 10.1109/ICORR.2015.7281320
Stokes, M. (2004). Physical Management in Neurological Rehabilitation. London: Elsevier Health Sciences.
Sun, L., Yin, D., Zhu, Y., Fan, M., Zang, L., Wu, Y., et al. (2013). Cortical reorganization after motor imagery training in chronic stroke patients with severe motor impairment: A longitudinal fMRI study. Neuroradiology 55, 913–925. doi: 10.1007/s00234-013-1188-z
Tinetti, M. E., Franklin Williams, T., and Mayewski, R. (1986). Fall risk index for elderly patients based on number of chronic disabilities. Am. J. Med. 80, 429–434. doi: 10.1016/0002-9343(86)90717-5
Wenderoth, N., Debaere, F., Sunaert, S., and Swinnen, S. P. (2005). The role of anterior cingulate cortex and precuneus in the coordination of motor behaviour. Eur. J. Neurosci. 22, 235–246. doi: 10.1111/j.1460-9568.2005.04176.x
Wolbers, T., Weiller, C., and Büchel, C. (2003). Contralateral coding of imagined body parts in the superior parietal lobe. Cerebral Cortex 13, 392–399. doi: 10.1093/cercor/13.4.392
Zhang, H., Xu, L., Wang, S., Xie, B., Guo, J., Long, Z., et al. (2011). Behavioral improvements and brain functional alterations by motor imagery training. Brain Res. 1407, 38–46. doi: 10.1016/j.brainres.2011.06.038
Keywords: robotic orthosis, cognitive training, functional magnetic resonance imaging (fMRI), walking, rehabilitation, stroke
Citation: Villa M-C, Geminiani GC, Zettin M, Cicerale A, Ronga I, Duca S and Sacco K (2023) Re-learning mental representation of walking after a brain lesion. Effects of a cognitive-motor training with a robotic orthosis. Front. Neurorobot. 17:1177201. doi: 10.3389/fnbot.2023.1177201
Received: 01 March 2023; Accepted: 17 July 2023;
Published: 31 July 2023.
Edited by:
Chad Gregory Rose, Auburn University, United StatesReviewed by:
Josefina Gutierrez, National Institute of Rehabilitation Luis Guillermo Ibarra Ibarra, MexicoCopyright © 2023 Villa, Geminiani, Zettin, Cicerale, Ronga, Duca and Sacco. This is an open-access article distributed under the terms of the Creative Commons Attribution License (CC BY). The use, distribution or reproduction in other forums is permitted, provided the original author(s) and the copyright owner(s) are credited and that the original publication in this journal is cited, in accordance with accepted academic practice. No use, distribution or reproduction is permitted which does not comply with these terms.
*Correspondence: Katiuscia Sacco, a2F0aXVzY2lhLnNhY2NvQHVuaXRvLml0
Disclaimer: All claims expressed in this article are solely those of the authors and do not necessarily represent those of their affiliated organizations, or those of the publisher, the editors and the reviewers. Any product that may be evaluated in this article or claim that may be made by its manufacturer is not guaranteed or endorsed by the publisher.
Research integrity at Frontiers
Learn more about the work of our research integrity team to safeguard the quality of each article we publish.