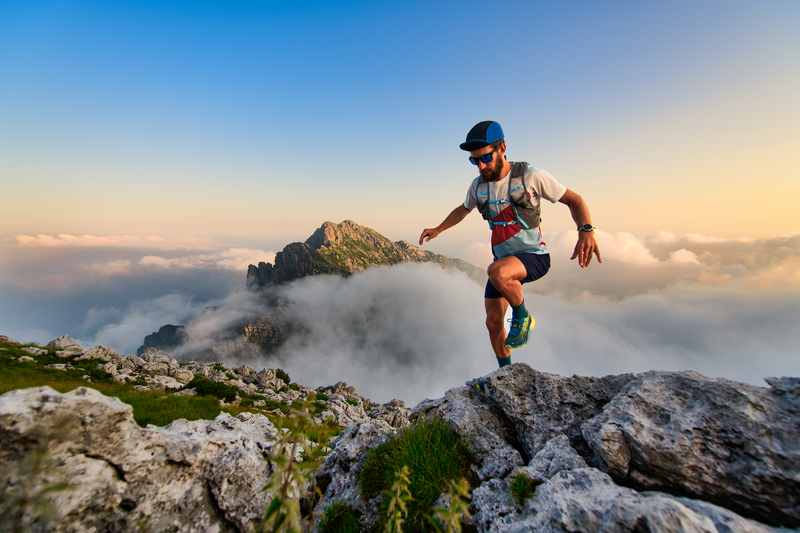
95% of researchers rate our articles as excellent or good
Learn more about the work of our research integrity team to safeguard the quality of each article we publish.
Find out more
REVIEW article
Front. Neurol. , 05 February 2025
Sec. Neurotrauma
Volume 16 - 2025 | https://doi.org/10.3389/fneur.2025.1472679
Traumatic brain injury (TBI) is a complex condition involving mechanisms that lead to brain dysfunction and nerve damage, resulting in significant morbidity and mortality globally. Affecting ~50 million people annually, TBI's impact includes a high death rate, exceeding that of heart disease and cancer. Complications arising from TBI encompass concussion, cerebral hemorrhage, tumors, encephalitis, delayed apoptosis, and necrosis. Current treatment methods, such as pharmacotherapy with dihydropyridines, high-pressure oxygen therapy, behavioral therapy, and non-invasive brain stimulation, have shown limited efficacy. A comprehensive understanding of vascular components is essential for developing new treatments to improve blood vessel-related brain damage. Recently, mesenchymal stem cells (MSCs) have shown promising results in repairing and mitigating brain damage. Studies indicate that MSCs can promote neurogenesis and angiogenesis through various mechanisms, including releasing bioactive molecules and extracellular vesicles (EVs), which help reduce neuroinflammation. In research, the distinctive characteristics of MSCs have positioned them as highly desirable cell sources. Extensive investigations have been conducted on the regulatory properties of MSCs and their manipulation, tagging, and transportation techniques for brain-related applications. This review explores the progress and prospects of MSC therapy in TBI, focusing on mechanisms of action, therapeutic benefits, and the challenges and potential limitations of using MSCs in treating neurological disorders.
Traumatic brain injury (TBI) is a disruption in normal brain function caused by an external force, leading to various degrees of neuronal damage and brain dysfunction (1–3). TBI is the most common cause of death and disability in people under 40 years of age in the United Kingdom and the third leading cause of death worldwide (1, 4). The mortality rate is 3.5 times higher than that of heart disease and cancer in industrialized countries (5). TBI involves both primary and secondary injuries. Mechanical trauma initiates primary damage, which subsequently triggers a cascade of pathological changes, leading to secondary injury (6). TBI contributes to various complications, such as cerebral hemorrhage and concussion. Additionally, TBI-related processes can lead to delayed apoptosis and necrosis, affecting autophagy and neuronal count (5, 7). Intracerebral hemorrhage (ICH), referred to as cerebral bleeding, intraparenchymal bleeding, or hemorrhagic stroke, is sudden bleeding into the tissues of the mind or bleeding the mind tissue into its ventricles, or both, that can cause brain harm and be life-threatening (8). Bleeding happens in 46% of all TBIs and is progressively predominant in moderate and severe injuries. Brain damage brings about hemorrhage that can steadily advance over the initial 24–48 h. Sometimes, the spilling blood gathers outside the vessel, forming a hematoma (9, 10). One less investigated potential objective has been mined vasculature and its effect on TBI results. A significant result of TBI is immediate harm to the cerebral vasculature. We still need a complete understanding of vascular components that could improve new vascular therapies for TBI and vascular-related brain injuries (11).
At present, there is no safe drug treatment for moderate and severe TBI (12, 13). Among the pharmacological agents evaluated, nimodipine and nifedipine, both dihydropyridines, demonstrated efficacy in rodent and mammalian models. However, these agents showed limited effectiveness in promoting recovery in patients with TBI (14, 15). So far, high-pressure oxygen therapy, non-invasive brain stimulation, drug therapy, and behavioral therapy have been used to treat TBI. Recent advances in stem cell research have opened new avenues for treating TBI (16, 17). In recent years, progress in the study of stem cell biology, especially mesenchymal stem cells (MSCs), has been very effective in improving and repairing brain injuries, such as TBI, and therapeutic strategies for stroke treatment (Table 1) (6, 18). These cells can, by migrating to that area and replacing the damaged tissue or by differentiating into cells that secrete growth factors and anti-inflammatory cytokines, in addition to decreasing brain edema and neuroinflammation and, on the other hand, improving function. Movement increases the proliferation and differentiation of neural stem cells, neurogenesis, and angiogenesis (16, 19). Exosomes play a role in the recovery of perinatal brain damage and reduce neuroinflammation caused by microglia (20, 21). Based on studies, MSCs produce extracellular vesicles (EVs) that prevent microglia activation, and then exosomes are used to reduce neuroinflammation caused by TBI (Figure 1) (22, 23).
Figure 1. The origins, differentiation ability, therapeutic mechanisms, and delivery methods of MSCs and EVs secreted by MSCs for TBI. MSCs can differentiate into chondrocytes, adipocytes, neurons, osteoblasts, and glial cells.
This article aims to investigate the familiarity with MSCs and their types, EVs derived from MSCs, the regenerative and therapeutic role of MSCs and EVs in TBI, and the challenges and potential limitations of MSCs used in treating neurological disorders.
MSCs are multipotent cells that can self-renew and differentiate into various cell types. They can be sourced from multiple human tissues and organs, including bone marrow (BM), adipose tissue, lung, brain, synovial fusion, pancreas, synovium, and peripheral blood (24–30). Friedenstein was the first to develop guinea pig bone-forming cells, and Owen revitalized this research by extending this technique to rats (31, 32). The isolation and in vitro expansion of MSCs derived from human BM were first documented in 1992, with subsequent reports of their therapeutic administration to patients commencing as early as 1993, as noted in a publication from 1995 (33, 34). Stem cells are characterized primarily by their multi-differentiation and self-renewal capabilities, as well as their various origins. Additionally, MSCs aid in tissue regeneration by producing cytokines and growth factors that draw other cells to the injured area (35–37). These cytokines and growth factors also encourage the development of new blood vessels, which are essential for tissue healing. Stem cell treatment is a viable alternative for tissue regeneration and repair since MSCs can control immune system activity, lower inflammation, and inhibit immunological responses. They are therefore excellent candidates for cellular treatments for a range of illnesses because of this characteristic (38–40). Stem cell transplantation has been shown in numerous studies to be beneficial for several diseases, including diabetic foot ulcers, congenital cataracts, ocular surface burns, severe skin burns, myocardial infarction, Parkinson's disease, Huntington's disease, and TBI (41–50). MSCs derived from human or rat donors specifically target damaged brain tissue (homing) following injections and aid in functional recovery (51, 52).
MSCs demonstrate therapeutic benefits in various medical conditions due to their diverse mechanisms of action. These cells are recognized for their regenerative properties, which encompass facilitating tissue regeneration and enhancing the healing process of wounds (45, 53, 54). The functions of MSCs in the management of neurological disorders and diseases encompass mitigating inflammation via immunomodulation, discharging trophic factors to facilitate therapeutic outcomes, fostering neurogenesis, being antibacterial, stimulating angiogenesis, diminishing infarct volume, substituting damaged cells, and emitting EVs, all of which contribute to their therapeutic efficacy (55–58). MSCs demonstrate immunomodulatory properties through the inhibition of inflammatory reactions and the facilitation of anti-inflammatory pathways (59, 60). MSCs have the potential to enhance the production of anti-inflammatory cytokines, including interleukin-4 (IL-4), interleukin-10 (IL-10), and tumor necrosis factor β (TNF-β). Conversely, MSCs have the potential to decrease the production of inflammatory cytokines such as interleukin-1 (IL-1), interferon γ (IFN-γ), tumor necrosis factor α (TNF-α), and membrane cofactor protein-1 (MCP-1) (61–64). MSCs modulated various pathways related to immune cells and immune responses by controlling the levels of cytokines, thereby mitigating inflammation (65–67). Studies showed that TNF-α and IFN-γ were the central pro-inflammatory cytokines. Prostaglandin E2 (PGE2) has been identified as a significant mediator of MSCs, playing a crucial role in modulating the immune response and inflammation. It achieves this by regulating immunity, suppressing T-cell proliferation, and influencing T-cell differentiation (68). Following a stroke, there was a noted decrease in the level of PGE2, which subsequently increased after the transplantation of MSCs. This was accompanied by a reduction in the secretion of TNF-α in dendritic cells (DCs) and a reduction in the secretion of IFN-γ in T helper one cells and natural killer (NK) cells. Consequently, there was a notable decrease in the density of TNF-α, suggesting that MSCs mitigated the neuroinflammation induced by stroke (69, 70).
Studies conducted in laboratory settings have shown that MSCs play a role in stimulating the growth of neurogenesis and angiogenesis. MSCs release growth factors like vascular endothelial growth factor (VEGF) and brain-derived neurotrophic factor (BDNF), which contribute to the development of neurons and the creation of new vascular structures (71–73). These properties enable MSCs to mitigate tissue damage and enhance functional recovery in conditions such as TBI (6, 74).
MSCs are a kind of immunodeficient cell that is easily obtained. Allogeneic gene transplantation often does not result in immunological rejection. Human leukocyte antigen (HLA) class I is represented at modest levels in the majority of stem cells, according to earlier research. HLA class II is not expressed by them, nor are co-stimulator factors (CD40, CD80, and CD86) or surface markers of hematopoietic cells (CD34, CD45, CD79, and CD14) expressed by them (75–77). This characteristic allows stem cells to have immune privilege without provoking the host and transplanted cells' immune systems to clash (78). HLA class I is essential because modest protein concentrations can shield cells from the cytotoxic effects of NK cells (79). It has been documented that exposure to the pro-inflammatory milieu of injured tissues causes MSCs to express HLA class II (78). It has been shown that MSCs are extremely immunogenic after they are transplanted into the host (80). Over 90% of undifferentiated MSCs demonstrate the expression of HLA class II upon exposure to IFN-γ (81). Furthermore, to evade immune monitoring, hair follicle stem cells downregulate major histocompatibility complex (MHC) class I in the static state, according to research by Agudo et al. (82). Numerous variables, such as the microenvironment and cell state, might affect how immunogenic MSCs become. Consequently, more research on the specifics of MSC immunogenicity is required to increase the effectiveness of MSC transplantation (83).
MSCs exhibit various cell surface immune markers, leading the International Society for Cellular Therapy (ISCT) to establish identification criteria for MSCs in 2006. These criteria include characteristics such as plasticity and adherence, as well as the presence of CD73, CD90, and CD105 markers while lacking CD14, CD34, CD45, CD11b, CD79α, CD19, and HLA-DR markers. Additionally, MSCs should demonstrate the ability to differentiate into chondrocytes, osteoblasts, and adipocytes (84). The goal of the ISCT recommendations is to encourage cooperation among researchers and standardize MSC research. The common immunophenotypes of MSCs may generally be expressed by MSCs derived from various tissue origins, while the expression of the other immunophenotypes varies somewhat. As research develops and new information becomes available, this standard will likely be changed in the future (83).
Various origins of MSCs have been identified in the literature. Contemporary studies indicate stem cells can be isolated from diverse tissue sources (Figure 1). Bone marrow-derived mesenchymal stem cells (BM-MSCs), Human umbilical cord mesenchymal stem cells (HUC-MSCs), Adipose tissue-derived mesenchymal stem cells (ADSCs), and placenta-derived mesenchymal stem cells (PD-MSCs) are among the more researched MSC types. BM-MSCs are a diverse population of cells comprising pluripotent adult stem cells that can differentiate into many lineages, such as chondrocytic, adipocytic, or osteocytic (85, 86). This cell type constitutes ~0.001%−0.01% of bone marrow mononuclear cells (BMMNCs). It is characterized by the presence of CD73, CD90, and CD105 markers while lacking the expression of CD14, CD45, CD34, CD11b, CD79α, CD19, and HLA-DR surface molecules (87). Owing to its low abundance, large-scale in vitro culture and amplification are needed to provide enough samples for study or therapeutic application (88). BM-MSC collection is frequently an expensive and intrusive procedure. Additionally, when donor age increased, the quality of the BM-MSCs' cells declined noticeably (89, 90).
HUC-MSCs were isolated from Wharton's Jelly, a gelatinous connective tissue surrounding the umbilical cord blood vessels (91). Since it is often abandoned at birthing, collecting it is non-intrusive and presents few moral dilemmas (92). Its traits include a lengthy survival duration, a low doubling time, and potent anti-inflammatory properties. Prolonged in vitro culture has minimal effect on its genetic stability and phenotype (93–96). In contrast to BM-MSCs, HUC-MSCs exhibit enhanced proliferative capacity and reduced HLA-ABC and HLA-DR expression (97).
ADSCs are abundant in tissue reservoirs and can be acquired through minimally invasive procedures involving extracting subcutaneous white adipose tissue from the abdominal region, thighs, or buttocks of both animals and humans (98). ADSCs are easy to isolate and have a high yield-1,000 mL of adipose tissue can generate about 100 mL of ADSCs (99). It can differentiate into several lineages, such as hepatic, neurogenic, chondrogenesis, osteogenesis, cardiomyocyte, and adipogenesis (100, 101). In low-passage cultures, ADSCs frequently express CD34; however, this diminishes with ongoing cell passage (102, 103). In contrast to BM-MSCs, ASCs lack the expression of the sialoglycoprotein podocalyxin (PODXL) and the adhesion marker CD106 (104, 105).
The amniotic membrane, amniotic fluid, chorionic villi, chorionic plate, decidua basalis, entire placenta, and complete placenta are among the tissue sources of placenta-derived MSCs (PD-MSCs) (106). Compared to other tissue-derived MSCs, the placenta's stem cell-like cells have a greater capacity for differentiation and self-renewal (107). Furthermore, research conducted in vivo and in vitro has demonstrated its low immune qualities (108). Additionally, it has been shown that PD-MSCs promote monocyte differentiation from inflammatory M1 macrophages to M2-like macrophages, indicating that PD-MSCs may help treat inflammatory disorders (109). Nonetheless, MSCS that are separated from various placental regions have modest variations in characteristics. The placental tissue, for instance, comprises two distinct individual tissues: the fetal and the maternal placental tissues. Compared to MSCs produced from maternal placental tissues, those derived from fetal placental tissues exhibit substantially greater proliferation ability (110).
Gingival MSCs (GMSCs) are derived from various sources, such as periodontal tissue, dental pulp, and gingival ligaments. GMSCs exhibit MSC-related cell surface markers such as CD73, CD90, CD105, and stromal cell antigen 1 (STRO-1), just as MSCs from other sources (111). Research has also revealed that GMSCs may transdifferentiate into ectoderm and endoderm cell lineages, including keratinocytes, endothelial cells, and nerve cells, in addition to having the capacity to develop into the three mesoderm lines of adipocytes, osteocytes, and chondrocytes (112, 113). Furthermore, GMSCs can modulate the immune system, have an anti-inflammatory effect, and stimulate macrophage development (114–116). Moreover, GMSCS have constant morphological and functional properties under increased passage, are homogeneous, multiply quickly, and are not carcinogenic (114).
From normal human labial minor salivary glands, scientists have isolated heterogeneous cell populations with mesenchymal and epithelial characteristics (117). The existence of human labial gland-derived MSCs (LGMSCs) in the oral mucosa's lamina propria was later verified (118). Wang and colleagues effectively recovered MSCs from mature female salivary gland cysts and used flow cytometry to detect the distinctive MSC expression markers, such as CD29, CD44, CD73, CD90, and CD105. Notably, the salivary gland epithelial markers (CD49f) and CD34, CD45, CD106, and CD117 were negative (119). Compared to other MSCs, LGMSCs show a better capacity for differentiating into salivary gland epithelioid-like cells. They also possess the capability for osteogenic and lipogenic differentiation. Its capacity for adipogenic differentiation is, however, inferior to that of ADSCs (120, 121). LGMSCs can also modulate immunological function, have a shallow glandular site, and are simple to acquire and grow in vitro (122–124).
Furthermore, MSCs obtained from organs including the liver and pancreas are being studied, opening up possibilities for MSC multi-source routes. Noteworthy, MSCs derived from donors with type 1 diabetes mellitus (T1DM) have phenotypic and functional similarities with those of donors in good health. They can continue to perform secretory or immunomodulatory tasks typically (125). Nonetheless, MSCs derived from donors with type 2 diabetes mellitus (T2DM) frequently exhibit elevated apoptosis and senescence to reduced angiogenesis capacity (126).
The therapeutic benefits of MSCs may be attributed to the production and dissemination of EVs, although other substances released by MSCs are also linked to their therapeutic properties (Figure 1) (127–130). Owing to the lack of agreement over specific EV subtype markers, it is advised to use physical EV features like size (131–133). Consequently, EVs with <200 nm are called tiny EVs. Considering that microvesicles range in diameter from 100 to 1,000 nm, some of them may also be tiny EVs (131). Therefore, it won't be entirely proper to use the word “exosome.” The International Society for Extracellular Vesicles supports the term “EVs” to refer to any naturally occurring particles that are discharged from cells and are surrounded by a lipid bilayer but are unable to multiply due to the lack of a functioning nucleus (132, 133). Nearly all cells can release EVs, which are composed of many functional components, including proteins, lipids, enzymes, cytokines, receptors on the cell surface, and nucleic acids such as DNA, messenger RNAs (mRNAs), and microRNAs (miRNAs) (133, 134). EVs are essential for intercellular communication because, in their capacity as regenerative medicine, they deliver their payload to recipient cells (135, 136).
The issues with MSC use, particularly in human treatments, have made it possible to do related research on vesicles. Many of the therapeutic actions of MSCs were recapitulated by MSC-EVs, with notable enhancements. Recent research has demonstrated that MSC-EV therapies offer comparable or even greater efficacy than MSCs in treating a wide range of illnesses, all while lowering hazards significantly. Therefore, EVs took the place of their parent cells for several therapies. These demonstrations have created opportunities for a novel therapeutic approach based on the usage of MSC-EVs, which is commonly referred to as cell-free treatment (137). There are several benefits to this advancement, including quicker tissue penetration and more excellent safety. Additionally, the limited potential of MSC-EVs to trigger the immune system prevents disappointments even during allo- and xeno-grafts; the ease of transport and storage makes the potential of EV therapy optimal when compared to standard cell-based approaches; and the inability of MSC-EVs to self-replicate dramatically reduces the risk of tumors and expansions, typical of MSCs (137–141). Some clinical trials have developed as a result of these findings. Since MSC-derived EVs eliminate many of the hazards associated with MSC-based treatment, their applications are gaining popularity (142). The heterogeneity of MSC-EVs is an important characteristic. Their parent cells are produced in the stroma of tissues, which might vary, as was previously indicated (142).
Stroke is the leading cause of permanent disability and the second leading cause of death worldwide, with ~5.5 million deaths per year (143). About 80% of stroke events include ischemic stroke (144). While TBI is often caused by motor vehicle or sports accidents and is the leading cause of death and disability in adolescents and young men, stroke mainly affects the elderly (145). Neonatal/perinatal ischemic stroke is a devastating disease that occurs once in every 3,500 births per year in the United States (146, 147). The consequences of perinatal stroke include spasticity, cognitive impairment, and death (148). Angiopathy and thromboembolism caused by intracranial or extracranial vessels are among the causes of ischemic stroke, and the most common cause in children under 15 years old is cerebral arteriopathy, which includes half of the cases (146, 148, 149). In stroke, rapid activation of innate immunity is the cause of inflammation (150). Breakdown of transcellular ion gradients due to the reduction of oxygen and energy supply, cytotoxic edema, production of toxic free radicals, and progressive thrombus formation in cerebral microvessels due to endothelial dysfunction are among the first pathological events after cerebral stroke (151). Studies have shown that CD4+ CD28-T cells increase in clinical conditions of acute ischemic stroke. T cells producing high amounts of γ-interferon and TNF-α probably have a direct pathogenic role in nerve damage (152). There are few treatments for ischemic stroke. In adults, the only FDA-approved drug for the treatment of ischemic stroke is tissue plasminogen activator (tPA), a thrombolytic agent. In comparison, the efficacy and safety of tPA in children are unknown (148). The paraspinal administration of etanercept in a study that included 629 patients with chronic neurological, mental, and clinical disorders after a stroke caused a partial improvement of the disease (153). The hypothesis that inhibiting the production of pro-inflammatory cytokines may be a therapeutic approach to treating brain injury is also proposed (154). Cytokines TNF-α, IL-1, and interleukin-6 modulate tissue damage in ischemic stroke (155). For the treatment of increased intracranial pressure (ICP) resulting from various causes, particularly ischemic and traumatic brain injuries, decompressive craniectomy has been employed. Given the rigid structure of the skull, brain swelling induced by stroke or TBI can lead to compartment syndrome and elevated ICP, necessitating this surgical intervention (156). Due to the little-known treatment methods, there is an urgent clinical demand for new treatment options. The therapeutic strategy can be successful in this situation if it targets several pathophysiological mechanisms that occur in different stages of brain damage (Figure 1) (143).
MSC may play a role in the recovery of nerve damage by regulating various mechanisms such as immune system function and nutritional factor secretion (157–160). The mechanisms of action of MSCs have two levels: the peripheral level and the central level. The peripheral level includes the reduction of inflammation and immune modulation, and the central level is affected by angiogenesis, astrocytes, neurogenesis, axons, and oligodendrocytes (Figure 1) (18, 161). These cells will probably be able to create an environment that stimulates angiogenesis and neurogenesis, and on the other hand, they will increase the secretion of growth factors (162). Transplantation of MSCs into animal models of infants suffering from ischemic stroke improves performance. This mechanism works by stimulating neurogenesis, oligodendrogenesis, and axon regeneration. Infants are believed to benefit more from cell therapy than adults because infants have more flexible brains and different injury pathophysiologies. Also, microglial activation is more evident in infants because microglial activation is also present during the physiological development of the brain (153). The role of EVs derived from MSC is to prevent microglia activation (22).
An increasing body of preclinical research indicates that stem cell therapy shows promise in the treatment of ischemic brain injury and in mitigating its enduring consequences. The positive outcomes observed in phase I clinical trials of stem cell therapy for stroke have bolstered the confidence of researchers and clinicians in the potential clinical utility of this therapeutic approach (163, 164). Further improvement is necessary regarding the clinical applicability of these treatments and to validate their effectiveness and safety (165, 166). Additionally, the National Institutes of Health Consortium's “Stem Cell Therapies as an Emerging Paradigm for Stroke (STEPS)” has established the fundamental guidelines supporting the use of MSCs in clinical trials for stroke patients to guarantee the resolution of ethical, technical, and medical issues before clinical translation (167, 168). According to STEPS, human trials might involve either an immediate infusion of stem cells to reduce the chance of ischemia harm occurring later on or a late intervention to promote neuronal regeneration during the chronic phase of the stroke (164). Furthermore, additional data from earlier clinical studies has shown the necessity of enhancing critical elements, such as the proper selection of appropriate cells and the mode of delivery, to successfully convert preclinical findings into practical clinical practice (169).
After confirming that stem cell transplantation in stroke patients is a safe and well-tolerated treatment, higher-stage clinical trials sought to determine whether stem cells may offer quantifiable advantages (170). A randomized controlled trial (RCT) was conducted to examine alterations in neuroimaging metrics following the administration of stem cell-based therapy in individuals diagnosed with ischemic stroke. The participants were segregated into groups receiving MSC treatment and control groups. The neuroimaging assessment encompassed 31 patients who received MSC treatment and 13 control patients. Motor function was assessed through the Fugl-Meyer assessment scale. At the same time, neuroimaging techniques were employed to analyze fractional anisotropy in the corticospinal tract and posterior limb of the internal capsule, as well as connectivity within the motor network. The group receiving MSC treatment demonstrated a notable enhancement in motor function and preservation of corticospinal tract integrity, in contrast to the control group, which showed a deterioration in these aspects. Moreover, the MSC group exhibited heightened interhemispheric and ipsilesional connectivity, demonstrating notable variations in interhemispheric connectivity alterations compared to the control group. These results imply that stem cell-based treatment can promote network reconfiguration and prevent degeneration of the corticospinal tract, promoting motor recovery following a stroke (NCT01716481) (171).
A study conducted at a single medical facility, known as ISIS-HERMES, utilized an RCT design with an open-label approach to investigate the safety, feasibility, and effectiveness of intravenous (IV) autologous BM-MSCs in individuals aged 18–70 who had experienced moderate to severe ischemic carotid stroke within 2 weeks of its onset. The study had a follow-up period of 2 years. Participants were assigned randomly in a 2:1 ratio to receive MSCs or standard care. The main objectives of the study were to evaluate the feasibility and safety of the intervention, with an additional focus on secondary outcomes, such as overall improvement and motor recovery, assessed through fMRI during passive wrist movements. Out of 31 participants, 16 were administered MSCs, demonstrating a treatment feasibility rate of 80%. The cohort that received MSC treatment exhibited notable enhancements in motor-NIHSS (p = 0.004), motor-Fugl-Meyer scores (p = 0.028), and task-related fMRI activity within the primary motor cortex regions MI-4a and MI-4p (p = 0.031 and p = 0.002, respectively). The findings suggest that administering IV autologous MSC therapy following a stroke is both safe and viable, and it contributes to improved motor function recovery by promoting sensorimotor neuroplasticity (NCT00875654) (172).
A phase 2 single-center, assessor-blinded RCT investigated the safety and efficacy of IV autologous BM-MSCs in 17 patients aged 30–75 with severe ischemic stroke in the middle cerebral artery territory. Participants were randomly assigned to receive either BM-MSCs or conventional treatment. The primary endpoints evaluated after 12 months were the National Institutes of Health Stroke Scale (NIHSS), modified Rankin Scale (mRS), Barthel Index (BI), and MRI infarct volume. The findings indicated no notable variations in NIHSS, mRS, or BI among the groups; however, a marked decrease in median infarct volume was observed in the BM-MSC group. The treatment was deemed safe and well-received, indicating promising advantages in diminishing infarct volume (NCT01461720) (173).
Chung et al. (174) conducted an RCT to investigate the potential benefits of autologous-modified MSCs in enhancing recovery among individuals with chronic stroke. The study's findings indicated that the IV administration of preconditioned autologous MSCs, along with autologous serum, was both feasible and safe for patients with chronic severe stroke. Additionally, the researchers noted improvements in foot movement through detailed functional assessments (NCT01716481) (174). These clinical trials collectively emphasize the therapeutic potential of MSCs in improving stroke outcomes.
Spontaneous ICH is one of the most detrimental cerebrovascular diseases globally, causes immoderate morbidity and mortality, and is a kind of stroke. Cerebral hemorrhage is frequently categorized based totally on the same region in the brain where it happens (175). Bleeding within the brain itself is called ICH. Bleeding can also occur between the lining of the brain and the brain tissue itself. That's known as a subarachnoid hemorrhage. If a blood clot occurs between the cranium and the brain, it's known as a subdural or epidural hematoma, depending on whether it's far underneath or over the difficult overlaying (dura) of the brain. Subdural and epidural hematoma most likely occur due to stressful brain damage or after a fall (176). The pooled blood that creates a hematoma within the brain can cause extended intracranial stress, which in turn damages the mind's parenchyma and may result in everlasting nerve damage or loss of life. The most common cause of cerebral hemorrhage is high blood pressure. Over time, excessive blood strain can weaken the arterial walls and lead to rupture, which is set at 13% of strokes, hemorrhagic strokes, or spontaneous bleeding within the brain (176). The reasons for cerebral hemorrhage consist of high blood stress (high blood pressure) or cerebral amyloid angiopathy (CAA), which is one of the most common and important reasons for cerebral hemorrhage. With the passage of time and age, blood pressure will increase and may harm the walls of cerebral arteries. Weaken and expand (aneurysm) abnormally and lead to rupture (177–179).
MSCs have shown promising results in preclinical models for the treatment of ICH. MSC treatment has improved neural network reconstruction, neurological functioning, and ICH-induced neuronal abnormalities through anti-inflammatory, neurogenesis, angiogenesis, and anti-apoptotic effects (180, 181). By modulating immune responses and releasing anti-inflammatory cytokines, MSCs lessen the inflammatory cascade set off by brain hemorrhage. This reduces the possibility of further harm to brain tissue (182, 183). A study conducted by Azevedo et al. (184) in a preclinical setting aimed to examine the possible effects of MSCs on CD4 T cells. The findings indicated that MSCs prompted the differentiation of CD4 T cells into regulatory T cell (Treg)-like cells through the activation of TGF-β and, or programmed death-1 (PD-1)/and programmed death ligand 1 (PD-L1) signaling pathways. Experimental evidence has confirmed that PD-L1 downregulates the migration of CD4+ T cells to the brain, leading to the upregulation of Th2 and Treg cells while simultaneously downregulating Th1 and Th17 cells. This regulatory effect is mediated through the mTOR pathway, as demonstrated in both in vitro and in vivo studies (185). Additionally, other research has shown comparable findings in the ICH rodent model, a specific B10.D2 [H-2(d)) donor to BALB/c (H-2(d)] recipient mice model, and the experimental autoimmune neuritis (EAN) rat model (185–187). It is widely recognized that neuroinflammation exacerbates the advancement of brain damage resulting from ICH. Therefore, interventions to modulate the immune response can potentially mitigate ICH-induced brain injury. The notable characteristics of anti-inflammatory and immunomodulatory effects render MSC transplantation a suitable therapeutic option for addressing inflammatory conditions such as ICH. This is achieved by modulating microglia and neutrophils, augmenting the defensive role of anti-inflammatory cytokines, and mitigating the adverse effects of pro-inflammatory cytokines (181, 188, 189). Kim et al. (190) discovered that transplanting ADMSCs into rats with an ICH model resulted in a reduction of acute inflammation and chronic brain deterioration, leading to enhanced long-term functional recovery (190).
BMSCs are commonly employed in treating brain injuries due to their convenient procurement from the host and ability to penetrate the blood-brain barrier (BBB) without causing structural disruption. This allows them to differentiate into neurons or neuron-like cells, facilitating tissue repair (191–195). Several research studies have shown that BMSCs have the potential to reduce neurological impairments and maintain the integrity of the BBB in rats with ICH (182, 196). In their study, Chen et al. (197) observed that using rat ADSCs in treating rats with ICH resulted in the development of cells resembling neurons and astrocytes near the injury site. Additionally, this treatment led to enhanced levels of VEGF, contributing to the restoration of neurological function in the affected rats (197). Yang et al. (198) utilized ADSCs obtained from the fat tissue of a 65-year-old male donor and administered them via injection into the right femoral vein of rats with ICH-induced stroke. Their findings indicated that transplantation of ADSCs may enhance the functional recovery of the test subjects (198).
MSCs reduce brain damage after ICH through the Hippo signaling pathway, which could promote neurogenesis and decrease the facet outcomes of intellectual injuries (199, 200). The Hippo signaling pathway is regulated by kinase activity, specifically involving mammalian sterile 20-like kinase 1 (MST1) and its associated protein, Yes-associated protein (YAP), which protects astrocytes from apoptosis. This pathway induces nuclear translocation of YAP by suppressing MST1 using small interfering RNA (siRNA). Furthermore, studies suggest that astrocytes adopt an astroglial-mesenchymal phenotype after BM-MSC transplantation, potentially enhancing their reparative capacity through the Hippo pathway. These findings position the Hippo signaling pathway as a promising therapeutic target for advancing the treatment and management of ICH (199, 201, 202).
A concussion is a “temporary disturbance in brain function as a result of trauma” and is a subset of neurological accidents referred to as annoying brain accidents. Concussions are technically a subset of mild traumatic brain injury (mTBI) (203). Concussions arise as a result of direct or oblique trauma to the head. However, indirect effects from forces someplace else inside the body can bring about acute acceleration or deceleration harm to the brain, which can also result in concussions (204). A complex web of biological processes, including structural modifications, neurochemical shifts, and functional deficits, interact to cause concussions. Cellular and metabolic alterations may result from the first impact's ability to stretch and damage axons. These consist of inflammatory reactions, disruption of the BBB, and excitatory neurotransmitter release. All of these mechanisms have a role in the acute and long-term symptoms that people with concussions experience. The discharge of electrolytes through ion channel depolarization results in the release of neurotransmitters and subsequent neuronal dysfunction. Adjustments in glucose metabolism lower cerebral blood flow, and mitochondrial dysfunction additionally occurs (205, 206).
MSCs and their EVs have emerged as promising therapeutic agents for addressing the complex pathophysiology of concussions (207, 208). A 2013 experiment that included 97 TBI patients who received autologous BM-MSCs by lumbar puncture lends support to the safety and effectiveness of this cell treatment in the context of clinical investigations of MSC therapy for TBI. After receiving a transplant, over 40% of patients showed better neurological function. Twenty-seven of the 73 individuals initially presented with motor problems showed improved motor abilities. The study found that patient age and the administrative window following injury all impacted the result, with younger patients responding better to the advantages of a cell transplant (209). Because of the features of multi-capacity and self-renewal and their availability and occasional immunogenicity and ability after freezing, their use turns them into a promising treatment for injuries and strokes. MSCs are multipotent stem cells with the potential for self-renewal and more than one differentiation (210–214). Inside the mouse TBI model, IV-injected BM-MSCs can penetrate the BBB and introduce dietary elements into the mind enhancer. They can also selectively switch them to the damaged areas of the brain tissue and differentiate them into neurons and astrocytes (215, 216). Advertising of axonal regeneration within the mind and angiogenesis and increasing glial cells on the site of harm can boost the internal restoration procedure. Additionally, MSC-derived EVs, which carry bioactive molecules such as mRNA, miRNA, and anti-inflammatory cytokines, play a crucial role in intercellular communication and neuroprotection (217). EVs derived from BM-MSCs prompt T cells by freeing anti-inflammatory cytokines and affecting apoptosis (218, 219). Research displays that EVs decreased using MSCs in hypoxic situations can put off neuronal degeneration and cause neurological recovery (220). Therefore, research is needed to determine the quality of MSCs to treat TBI. Thinking about the prevalence of mitochondrial dysfunction in TBI, enhancing mitochondrial features has been a practical therapeutic aim for acute brain harm in recent years. Mitochondrial switching from MSCs can lessen the rate of apoptosis in recipient cells and enhance cellular survival by regulating the Bcl-2-associated protein X (Bax)/Bcl-2 ratio (221). MSCs can also boost the expression of the antiapoptotic gene Bcl-2 and decrease the extent of superoxide anion, thereby shielding brain tissue (222). This mitochondrial transfer occurs among MSCs and target cells via tunneling nanotubes (TNTs), microvesicles, EVs, hole junctions, and cytoplasmic fusion (223–226). Many studies have shown that MSCs can shield brain tissue from excessive harm by inhibiting oxidative pressure. In a TBI mouse model, overexpression of specific genes, including that for superoxide dismutase 2, in vitro can enhance the antioxidant impact of MSCs and improve their therapeutic effects (227). In conclusion, while concussions pose significant clinical challenges, MSCs and their EVs offer innovative therapeutic strategies. Much medical research is underway to decide the most advantageous course and timing of management and dosage of MSCs and EVs, which can be famous directions for future studies.
Tumors that form in the brain can have debilitating effects, even if the tumor is benign (228). Glioblastoma multiforme (GBM) is the most common and aggressive brain tumor and a complex and resistant cancer. GBM can originate from normal brain cells or low-grade astrocytes (229, 230). Although the use of surgery, radiotherapy, and chemotherapy is suggested to increase life expectancy and quality of life, there is currently no cure for this cancer (231). One of the ways that has created new hope for the treatment and reducing the complications of GBM is mesenchymal stem cell therapy (232).
Studies have shown that MSCs are toxic to tumor cells. Also, new research shows that these cells induce apoptosis through the phosphatidyl-3-kinase/protein kinase B (AKT/PI3K) pathway and suppress the growth and proliferation of glioma cells (233). MSCs have anti-angiogenic properties, which has made them sound like anti-tumors; observations show that these cells can inhibit tumor blood vessels (234). Without forming a new ship and access to blood, tumors cannot grow more than 2–3 cubic millimeters (235). The process that controls and inhibits angiogenesis by MSCs in GBM is the reduction of focal adhesion kinase (FAK) and integrin β2α expression. FAK is a cytoplasmic tyrosine kinase involved in integrin activation, regulation of cell migration, proliferation, persistence, and aggression play a role. Research shows that MSC reduces FAK activity and reduces the formation of new blood vessels in the tumor (236). MSCs can induce apoptosis by inducing glioma cell death by downregulating X protein-associated inhibitor of apoptosis (XIPA). This protein is a member of the family of apoptosis inhibitor proteins, which has a positive effect on most malignancies, including GBM (237). MSC prevents the increase of glioma cell lines, accompanied by a 50% increase in cytotoxicity and apoptosis. The data obtained from the western blot shows a significant reduction of XIPA and alpha-serine/threonine kinase, which is associated with cell death. Further studies show that huc-MSC MSCs express genes encoding pro-proteins. Belonging to the pro-apoptotic Bcl-2 family, including Bax Bad, these studies confirmed the successful induction of apoptosis by MSC, huc-MSC, in glioma cells (238). An additional potential anti-tumor mechanism associated with MSCs is their influence on epidermal growth factor receptor (EGFR) signaling (239).
A few of these tactics have been taken to the clinic, where at least two ongoing clinical trials assess the potential benefits of MSC-based GBM therapies (240). The initial study, conducted at the M.D. Anderson Cancer Center in Texas, United States, is an extension of their findings from preclinical experiments involving GBM. In these experiments, allogeneic MSCs carrying oncolytic viruses (OVs) were introduced into the carotid artery, demonstrating encouraging outcomes (NCT03896568) (241). In this phase I clinical trial, an open-label approach is employed to investigate the utilization of the conditionally replicating oncolytic adenovirus Delta24-RGD in conjunction with MSCs. This strategy aims to capitalize on the inherent tumor-tropism of MSCs, with the potential benefit of restricting the dissemination of the virus to non-target organs. Additionally, this enables MSCs to penetrate the BBB and disperse broadly throughout the tumor (NCT03896568). Patients with recurrent glioblastoma are being treated in another research at CHA University in South Korea by transplanting MSCs that express the suicide gene cytosine deaminase (CD). This phase I/II clinical trial is open-label and aims to assess safety, efficacy, and maximum tolerated dosage (NCT04657315).
There is a similarity between macrophages and MSCs, such as the ability to switch between pro- and anti-inflammatory phenotypes. Stefani and colleagues tested the effect of low-dose MSCs on GBM. The GL261 glioma cell line implanted in the right striatum of mice treated with irradiated BM-MSCs increased mouse lifespan and reduced tumor volume by ~67%, and histochemical staining of the vessels of the tumor mass also showed that the blood vessels inside the tumor had decreased density (238). One of the most essential characteristics of malignant gliomas is the immunosuppression that an active tumor creates in the existing tissue (242). Therefore, one of the anti-cancer treatment strategies based on MSCs is dependent on intra-tumor immunomodulating cytokines, such as ILs. Momeh et al. researched that intra-tumor administration of genetically modified MSCs increased expression. IL-7 and IL-2 control pro-inflammatory intracranial tumors. Recent studies have proven that MSCs that express IL-24 secret immunomodulatory cytokines suppress tumor growth and induce apoptosis of glioma cells (139). Other cytokines delivered by MSC-dependent strategies include interferon-beta (IFN-β) secretion (243). MSCs represent a groundbreaking approach to GBM treatment by targeting tumor growth, angiogenesis, and the immunosuppressive microenvironment.
Encephalitis means brain inflammation. This disease is mainly caused by viruses and, in some cases, due to the immune system. The most common viral encephalitis is caused by herpes simplex infection, and other causes are viruses such as rabies, polio, and measles (244–246). Adults with encephalitis manifest with the onset of high fever, headache, confusion, and sometimes seizures. Younger children or infants may present with irritability, loss of appetite, and fever (247).
Currently, the application of MSCs in treating encephalitis demonstrates significant potential (248–250). MSCs have characteristics such as the ability to regulate immunity, the ability to migrate to the site of injury, and the ability to differentiate into different types of cells such as fat cells, osteocytes, chondrocytes, and neuron-like cells (95, 251, 252). Research shows that MSC transplantation can regulate the expression of BDNF and nerve growth factor (NGF) and also can improve nerves in many central nervous system (CNS) diseases (253–256). By regulating inflammation and other processes, MSCs have a therapeutic effect on most CNS diseases. In recent years, many studies have been conducted on the therapeutic effect of MSCs on CNS and viral diseases (257–261). In the research, they found that MSC transplantation improved the life span and also reduced the neurological symptoms in the mouse model that was infected with encephalitis and also in the mice that are suffering from neuroinflammation and treated with MSC, based on the changes in Pathological tissue, their neuroinflammation decreases. Research in the laboratory shows that IV or intraspinal administration of MSC improves the autoimmune encephalitis (EAE) mouse model and causes the disease to decrease significantly. Mice infected with JEV without MSC treatment showed clinical signs of encephalitis, which starts with piloerection and physical limitations, followed by paralysis and stiffness, and finally leads to severe neurological symptoms such as paralysis, seizures, and even death. The group treated with MSC had a faster recovery in terms of weight and behavioral conditions. Also, the lifespan in this group increased significantly compared to the group that was not treated, and studies have shown that treatment with MSC also reduces pain. Severe meningitis decreased significantly in JEV-infected mice treated with MSCs, and the levels of inflammatory cytokines and chemokines were also reduced in this group compared to the untreated group (261). Experiments show that after JEV infection, either in vivo or in vitro, a significant amount of TNF-α is produced, and MSCs can produce TSG-6 (TNF-α-stimulated gene/protein 6); by inducing TNF-α, it moderates inflammatory responses, controls BBB destruction, and also improves tissue damage. Through the experiments they conducted, researchers found that the expression of cytokines transforming growth factor TGF-β and TSG-6 in MSCs that were Cultured with Neuro2a cells that were infected with JEV was increased, which has an anti-inflammatory role (262, 263).
MSCs, in addition to their role in regulating inflammation, possess a protective immune function against various injuries caused by bacteria and viruses (259, 260, 264–268). Research indicates that MSCs exhibit an antiviral role. Also, the titer of JEV decreased in Neuro2a cells cultured simultaneously with MSC. One of the reasons for this is that MSCs can improve innate and adaptive immune responses by modulating immunity and helping eliminate the virus (259, 269, 270). Neuro 2A (N2a) is a cell line derived from mouse neural crest cells that is widely utilized in research focused on neuronal differentiation, axonal growth, and various signaling pathways. A notable feature of these cells is their capacity to undergo differentiation into neurons within a matter of days (261).
Critical to the efficacy of MSC therapy for neurological disorders are the dosage, duration, and route of administration of MSCs. Various studies have utilized dosages ranging from 1 × 106 to 5 × 106 for each kilogram of body weight across different animal models and types of injuries. Furthermore, the optimal dosage remains to be determined, as variations in dosage may lead to differing therapeutic outcomes. Consequently, further investigation is essential to refine dosing strategies and maximize the therapeutic potential of MSCs (162, 253, 280).
The route of administration is another critical factor that affects the bio-distribution, retention, and therapeutic efficiency of MSCs. Different routes, including IV, IA, intrathecal, intraperitoneal, and localized injections, have been employed in numerous studies (271, 281–283). IV injection is frequently used in instances of extensive injury, including conditions such as stroke, TBI, and Parkinson's disease, to facilitate the widespread dissemination of cells throughout the body, encompassing the affected brain tissue (280, 284, 285). IA administration represents a promising route for delivering MSCs in the treatment of neurological disorders, particularly ischemic stroke and brain injuries (286, 287). Compared to intracerebroventricular, intraparenchymal, and IV stem cell administration, IA stem cell distribution after ischemic stroke is less invasive and permits better diffusion and distribution of more significant stem cells inside and outside the infarct area (288). Moreover, it mitigates the risk of MSC entrapment in the lungs and liver, a standard limitation of IV administration (289). For instance, Zhang et al. (290) demonstrated that IA delivery of bone marrow MSCs resulted in the most significant neurological recovery compared to IV and intracerebral routes in a rat model of cerebral ischemia (290). Thus, a thorough understanding of MSC dosage and administration routes is critical for optimizing therapeutic outcomes and advancing MSC therapy from preclinical studies to clinical applications.
MSC-derived EVs have been shown to have neurorestorative capacity and have emerged as an innovative TBI therapy (Figures 1, 2). MSC-derived EVs have been demonstrated to enhance functional recovery in a rat model of TBI with postponed IV injection in a broad range of efficacious dosages (50–200 μg protein/rat) for TBI therapy with a prolonged therapeutic window from 1 day to 7 days post injury (291). In addition, EVs generated from monkey BM-MSCs that are given 24 h after an injury can improve fine motor function recovery in a monkey cortical injury model (292). The protective benefits of MSC-derived EVs in rats with TBI are mediated via endogenous angiogenesis and neurogenesis, as well as inflammation reduction (291). Following TBI, endogenous neurovascular plasticity, such as neurogenesis, angiogenesis, axonal sprouting, and synaptogenesis, occurs. This may aid in the brain damage's natural healing process (293). Post-brain damage, spontaneous healing is not always possible. They are developing innovative treatments to increase neurovascular plasticity and promote functional recovery following TBI, which is urgently needed. In the dentate gyrus of the damaged hippocampal brain, there is an increased endogenous neurogenic response in the subventricular and subgranular zones. This response is linked to the recovery of cognitive function following TBI (294). Neural stem cells located in the subventricular zone and subgranular zone exhibit the capacity to continually produce new neurons in adult mammals, which subsequently differentiate into fully developed neurons. The ability of adult-born dentate gyrus granule cells to integrate functionally into the current circuitry is well known (295). Neurogenesis and angiogenesis are markedly increased in the wounded brain following TBI when treated with MSC-derived EVs (beginning 24 h after injury), which may partially account for functional recovery following TBI (296). Normal brain vasculature is quiescent, but following an injury, it becomes active. Growth factors that support neurorestorative processes like neurogenesis and synaptogenesis may be secreted by activated vasculature, which might promote functional recovery following brain damage (297).
Figure 2. Mechanisms of MSC application in TBI. Communication between MSCs and tissue environments is facilitated through two primary mechanisms: direct cell-to-cell interactions and communication via EVs. MSCs engage in communicate with neighboring cells such as immune cells, nerve cells, glial cells, and endothelial cells, enabling regenerative healing and the architectural reorganization of injured tissue. MSCs produce EVs that encapsulate proteins, lipids, microRNAs, and cytokines, facilitating the transfer of functional molecules among cells. Positive modulation enhances biological functions such as autophagy, apoptosis, pyroptosis, inflammation, angiogenesis, cell plasticity, cell migration, and oxidative stress. These interactions induce the differentiation of MSCs into specific cell lineages and regulate immune cell reactions (181).
A thorough analysis has been done on the possible impacts of EVs produced from MSCs on neuroinflammation, neurogenesis, and, particularly, functional recovery in TBI (298, 299). According to a recent study, in elderly rats with a stroke, BM-MSCs-EVs boost post-stroke neurogenesis next to the subventricular zone and support functional neurological recovery (300). Through endogenous angiogenesis and neurogenesis promotion, MSC-derived EVs from human BM-MSCs (100 μg protein, IV) markedly enhance functional recovery following ICH in rats (301). Therefore, MSC-derived EVs without cells might be a potential treatment for ICH. The introduction of cell-free EVs derived from MSCs via IV administration following a stroke has been shown to enhance functional recovery and promote neurite restructuring, neurogenesis, and angiogenesis. This approach presents a novel therapeutic strategy for treating stroke (302–305). By defending the BBB, preventing apoptosis, reducing inflammation, and controlling autophagy in brain lesions through various chemicals and mechanisms, including miRNA, EVs may enhance cognitive function (306). In vitro neural progenitor cell neural development is promoted by EVs produced from adipose-derived MSCs (307). Through the transfer of miR-25 from EVs generated from adipose-derived MSCs, autophagy is decreased in stroke mice (308). In a stroke-prone mouse model, EVs derived from human BM-MSCs enhance neurodegeneration and avert post-ischemic immunosuppression (309). EVs from human BM-MSCs reduce neuroinflammation in rats with focal brain damage (310). Mice exposed to ischemia brain injury are protected against MSC-derived EV-enclosed microRNA-93 (311). EVs derived from HUC-MSCs, when administered intranasal, demonstrate neuroprotective properties and enhance functional recovery following perinatal brain injury in rat models (312). The above research indicates that EV therapy enhances functional recovery by affecting the immune system, neurogenesis, neurorestoration, angiogenesis, and neuroprotection.
Neuroinflammation is a distinguishing feature of both acute and chronic TBI. An emerging mechanism facilitating cell-cell communication in regulating immune responses involves EVs (313). After being injected IV, green fluorescent protein-tagged (GFP+) MSC-EVs can be absorbed by microglia, astrocytes, and neurons in the TBI rat brain as early as 30 min later. More EVs are found in the injured hemisphere, and GFP+ EVs are co-localized with CD68+ macrophages in the liver, spleen, and thymus (314). These findings imply that IV injection of EVs may play not only central functions in promoting neurovascular remodeling and controlling neuroinflammation but also non-central effects in modulating peripheral immune responses. If there are any non-CNS effects, they would be complimentary and would not lessen the therapeutic benefits of EVs on neurovascular remodeling and functional recovery (315, 316).
Giving MSC-EVs to mice as soon as possible (15 min after the injury) reduces the size of the lesion and enhances their functional ability. This is achieved by modifying the polarization of macroglia and microglia, boosting the expression of the anti-apoptotic protein Bcl-2, but decreasing the expression of the pro-apoptotic protein Bcl-2-associated X protein and pro-inflammatory cytokines, IL-1β and TNF-α (317). The neuroprotective effectiveness of MSC-derived EVs has been studied in large animal models in translational research. Early (1-h post-injury) single-dose treatment of MSC-derived EVs reduces brain swelling, lesion size, and BBB breach, providing neuroprotection in a combined swine model of TBI and hemorrhagic shock (318). These findings promote further research into EVs as a cutting-edge TBI treatment by showing that cell-free EVs have neuroprotective and neurorestorative benefits for enhancing TBI functional recovery. Utilizing MSCs from human BM as the source of EVs might guarantee the most significant translational potential for TBI research in big animals. Multiple citations regarding the utilization of human BM-MSCs-derived EVs in extensive animal models of TBI, specifically in monkeys and swine, are outlined in Supplementary (Table 2) (130, 292, 318–320).
While we concentrated on the therapeutic benefits of MSC-derived EVs in TBI, EVs produced from a wide range of other cells, such as astrocytes, microglia, and neural stem cells, can also improve functional recovery in TBI (207). Adipose tissue is commonly regarded as refuse and disposed of; however, it represents a valuable reservoir of cells (321, 322). It has been demonstrated that adipose tissue is a rich, readily available, and plentiful source of adult stem cells with multipotent qualities appropriate for tissue engineering and regenerative medicine applications (321). Treating TBI using MSC-derived EVs has shown to be a promising approach (323, 324).
Given their ease of separation, minimal immunogenicity, and capacity to develop into a variety of tissue lineages, including brain cells, MSCs provide the most therapeutic promise (281). Nonetheless, there are still several restrictions on MSC transplantation. MSCs are likely to be contaminated during their cultivation and treatment, and cells cultivated in vitro are susceptible to mutation. Foreign infections may potentially spread as a result of cell transplantation. Furthermore, MSC transplantation may provide cancer cells vitality and encourage the development and spread of tumors. It is unclear how MSCs initiate and control mitochondrial translocation. Furthermore, it is impossible to overlook the possibility of allogeneic immunological rejection. Therefore, enhancing MSC therapy's safety is very crucial (21).
In the following, we discuss some challenges and limitations of using MSCs in nervous system disorders and TBI.
It's interesting to note that MSCs can have proinflammatory and immunosuppressive effects. These effects are contingent upon the degree to which the cell is stimulated by chemokines (e.g., PGE2, TGF-B, IL-6, IL-10, HLAG5), metalloproteinases, nitric oxide (NO), indoleamine-2,3-dioxygenase (IDO1), and inflammatory cytokines. Therefore, MSCs' immunosuppressive activity can be used to avoid instances of allograft rejection as well as an aberrant inflammatory or autoimmune response (333). MSCs are known to exhibit immunosuppressive effects in the presence of NO on a molecular level (334, 335). In contrast, in regions deficient in NO, specifically in areas where the activity of inducible nitric oxide synthase (iNOS) is suppressed, MSCs promote the proliferation of immune cells. Additionally, Qin et al. (336) have found that MSCs cannot stop T-cell proliferation in rat models when the NOS inhibitor L-NMMA is present (336). These results strongly suggest that NO generation or increased NOS2 activity is necessary for starting MSC-mediated immunosuppression. In MSC-mediated immunomodulation, indoleamine 2,3-dioxygenase is a switch similar to NO (337, 338). While some studies indicate that MSCs may play a role in cancer development, others show that they have a suppressive impact on the growth of tumors (339). The processes that underlie these suppressive effects include the induction of cell cycle arrest, suppression of proliferation-related signaling pathways PI3K/AKT, and, ultimately, a decrease in cancer development (340). On the contrary, alternative research has demonstrated that MSCs can differentiate into cancer-associated fibroblasts (CAFs) and consequently play a role in facilitating cancer advancement (341–343).
Numerous factors, including the technique used for separation, the individual diversity of the source tissue, the donor's health, and the specific cell culture's history, might influence the stemness qualities of MSCs (344). Roughly 10% of the cells in the dental pulp are mesenchymal stem cells (DP-MSCs). Compared to BM-MSCs and AT-MSCs, DP-MSCs generate fewer proangiogenic factors in vitro, although having more excellent proliferation rates (345). However, additional research has demonstrated that the chemokines and neurotrophins that DP-MSCs release are essential for neuroprotection and the body's reaction to nervous system damage (346, 347). Remarkably, MSCs display donor-related differences as well. These may result from the patient's age, underlying illnesses, gender, body mass index (BMI), and donor place (344). In a rat model, MSCs extracted from female donors are more effective than MSCs from male donors in lowering lung inflammation, according to a 2016 research by Sammour et al. (348). On the other hand, the osteogenic capacity of local stem cells is diminished by the hormonal fluctuations that women experience, particularly after menopause (349). Additionally, Ogawa et al. (350) confirmed that there is gender variability in AT-MSCs by observing that cells produced from female mice had greater levels of the adipogenesis marker Peroxisome Proliferator-Activated Receptor-⋎2 (PPAR-⋎2) (348–350). Age-related alterations in MSCs have also been documented in several studies (98, 351, 352). For instance, MSCs derived from older individuals exhibit reduced superoxide dismutase activity, along with elevated concentrations of reactive nitrogen species (RNS) and reactive oxygen species (ROS) (353). As a result, MSCs suffer oxidative damage, which triggers apoptosis. Furthermore, aged MSCs have elevated expression of p53 and p21, which are known for their pro-apoptotic activities, and downregulated expression of the Notch1 receptor, which is linked to bone formation (353).
For therapies utilizing MSCs to achieve notable efficacy in addressing neurological disorders, it is imperative to consider the impact of these diseases on the regenerative capabilities of the cells. For instance, via downregulating pro-angiogenic factors, Diabetes Mellitus (type 2 diabetes) negatively affects MSC function and decreases their capacity to form new blood vessels (354). Furthermore, BM-MSCs obtained from diabetes patients show an increased tendency to grow into adipocytes and reduced paracrine secretion (355). Additionally, it has been noted that BMI affects adipocytes' capacity for differentiation and proliferation (356). Therefore, impaired DNA telomere length, cell proliferation, and differentiation are features of overweight persons. Together with this, cells have a reduced capacity for self-renewal and an early beginning of apoptosis. Furthermore, elevated BMI negatively impacts adipogenic and osteogenic differentiation in AT- and BM-MSCs, as demonstrated by significantly reduced cell division, increased senescence, and lessened adipogenic differentiation (357). It's noteworthy to notice that with a significant drop in weight, there is less damage to DNA and an improvement in both cell viability and replicative lifespan (358).
Lastly, pharmacological substances and treatment modalities such as immunosuppressive medications, anticancer medications, and radiation therapy also affect the characteristics of MSCs (359–361). Like this, long-term morphine usage reduces endothelial progenitor cell activation and angiogenesis (362). Furthermore, it hurts MSC differentiation and proliferation, changing their secretory capacities and impeding wound healing (362).
MSCs from diverse origins have dramatically variable features, even though several studies have demonstrated the intriguing advantages of MSCs in tissue regeneration, making them an appealing study topic in regenerative medicine (363). For instance, compared to cells isolated from adult tissues, MSCs derived from fetal tissues exhibit faster cell proliferation and the capacity to go through many in vitro passages before senescence (364). Conversely, adult-isolated BM-MSCs and AT-MSCs have a greater stemness, demonstrated by their capacity to form more fibroblast colonies (CFU-F) (365, 366). It's interesting to note that MSCs derived from specific donors may have distinct variations. Studies on BM-MSCs isolated from donors of various ages and sexes revealed notable variations in the cells' proliferation rates, osteogenesis, and activity levels of the marker for bone remodeling (alkaline phosphatase, or ALP) (367). It's interesting to note that there was no reported relationship between these and the donors' age or sex. However, other research has demonstrated that the age of the donor has a significant impact on the characteristics of BM-MSCs. For instance, cells taken from older adults showed reduced proliferation, higher apoptosis, and a lower ability to differentiate into osteoblasts (368). Heo et al. (366) also showed that MSCs generated from various tissues exhibit significant inter donor heterogeneity in distal-less homeobox 5 (DLX5) gene expression (366). To facilitate the identification of specific molecular and functional phenotypes associated with harvesting techniques and tissue sources, Colter et al. (369) categorized MSCs into three subpopulations based on their morphology: spindle-shaped proliferating cells resembling fibroblasts (Type I); large, flat cells characterized by prominent cytoskeletal structure containing numerous granules (Type II); and small, round cells exhibiting a high capacity for self-renewal (Type III) (369, 370).
Further study is necessary to resolve the numerous issues and disagreements surrounding the use of MSCs in the human cell niche despite the positive results that MSC therapy offers. Thus, some of the primary possible risks associated with MSC therapy are as follows: (1) the possibility of pro-tumorigenic activity and undesirable cell type differentiation; (2) an uncontrolled immune response; (3) a brief survival period following implantation; (4) a lack of sufficient research on the differentiation capacities of MSCs; and (5) an inability to determine the best doses and mode of cell administration. By promoting tumor invasion by releasing CCL5 and preventing apoptosis by releasing pro-survival molecules like VEGF and bFGF, MSCs may demonstrate pro-tumorous activity (371–374). Therefore, introducing MSCs may cause an uncontrollable immunological response at the local or global level since they can explain both immunosuppressive and immunomodulatory effects (375). Regarding MSCs' brief lifetime after implantation, several studies have shown that, soon after transplantation, MSCs undergo massive mortality due to the activation of hypoxia signaling pathways and Caspase 3-mediated apoptosis. Remarkably, research by Deschepper et al. (376) revealed that ischemia circumstances (low pO2 and glucose depletion) caused the widespread mortality of MSCs at day 6. Still, hypoxic settings (low O2) allowed cells to survive until day 12 (376).
There are still several uncertainties about the differentiation ability and use of MSCs despite all the advantages of potential therapy approaches. These consist of their precise mode of action, safety during standard clinical usage, and tissue migration patterns (377). As a result, a large body of research indicates that various clinical indications and illnesses require distinct administration techniques for optimal therapeutic success (378, 379).
Despite the ability of MSCs to migrate toward tumor locations, numerous studies caution against their pro-tumor effects, which include immunosuppression, stimulation of blood vessel formation, transformation into cancer-associated fibroblasts, prevention of cell death in cancer cells, enhancement of metastasis and tumor growth, initiation of epithelial-mesenchymal transition (EMT), and facilitation of resistance to drugs (334, 380–393). While the previously described research examined and measured the impact of local MSCs on tumor development and related activities, it is essential to consider MSCs' capacity to promote tumor growth while developing novel treatment strategies utilizing this cell population (394). MSCs play a crucial role in inhibiting the innate and adaptive immune responses. They achieve this by secreting several substances such as TGF α, TNF β, IFN γ, PGE2, NO, HLA-G, HGF, IL-1b, IL-1a, LPS, and IL-6 (375, 394). Consequently, these elements lessen the maturation of DC, the production of IgG, the activity of natural killer cells (NKCs), and the proliferation of effector T- and B-cells. The net effect is a decrease in anti-tumor immunity. Additionally, it has been demonstrated that MSCs stimulate tumor angiogenesis by releasing bFGF, VEGF, FGF-2, IL-8, IL-6, IGF-1, TNF, and TGF β. They also induce the development of new tumor arteries and change into smooth muscle cells and pericytes. MSCs can differentiate into cancer-associated fibroblasts (CAFs) and smooth muscle cells (56, 389, 394, 395).
The use of MSCs for therapeutic reasons necessitates highly competent specialists to prevent cell contamination and ease the deployment of a highly standardized technique. A consistent methodology detailing the proper processes for isolating and maintaining MSC cultures still does not exist, even though several clinical trials are now in progress (396). The rationale for the significance of this standardized protocol is its ability to simplify the process of comparing several experimental studies and clinical trials side by side to identify the best distribution strategy and concentration. The public's interest in stem cell treatment has grown, leading to a growth in biobanking in recent years. While these establishments offer their clients the ability to obtain versatile stem cells as needed, they are also vulnerable to potential exploitation (56, 396). This is especially noticeable in biobanks run by private companies, where there is a chance of privacy violations and health data being sold (397). Furthermore, users of these biobanking services seem to belong to a particular social group: well-educated, white, middle-class people. As a result, those who don't match these stereotypes—namely, those who are lower class, indigenous, or from culturally varied communities—are inadvertently left out (398). This prejudice not only prevents marginalized people from accessing biobanks but also has a detrimental effect on scientific research because biobank samples and data are used in many different types of studies. However, understanding also brings the power to address these problems with representativeness and inclusion, which we should actively work to address in the following years (56, 398).
Simultaneously, researchers have demonstrated that while stem cell therapy is utilized for treating brain damage, MSC therapy has potential disadvantages, such as the risk of tumor formation. However, this risk can be mitigated using exosomes derived from MSCs, which offer a safer alternative. Research has shown that MSCs can increase and decrease tumor growth and tumorigenesis in different conditions (Figures 1, 2). The tumor in its microenvironment tries not to be recognized by the immune system and creates a stable state by secreting inflammatory mediators. There is a lot of focus on the interaction between cancer cells, normal cells, and the matrix in the microenvironment because this interaction contributes to the milestones of cancer progression, such as angiogenesis, immune modulation, metastasis, and invasion, as well as resistance to apoptosis (399–401). Some studies have shown that MSCs migrate to the cancer microenvironment. This place supports the development of the tumor vascular system and influences immune reactions, thus modulating the tumor's response to antitumor therapy (Figure 2). MSCs have immunosuppressive solid properties that cause tumor cells to escape immune surveillance (341, 402–405). MSCs in the tumor microenvironment by pro-inflammatory cytokines IFN-γ, TNF-α, or IL-1β can be activated and secreted by macrophages and tumor cells (371, 406–408). IFN-γ produced by Th1 decreases, and IL-4 secretion increases through Th2, which minimizes antitumor immunity and immune response. Monocyte differentiation is controlled by IL-6 secreted by MSC toward DCs, and they reduce the ability of DCs to stimulate T cells (380, 409). Exosomes derived from MSCs have shown significant promise in the field of regenerative medicine, including the treatment of TBI. The three-dimensional culture of MSCs enhances the production of exosomes, thereby increasing their therapeutic efficacy. Exosomes offer inherent safety advantages over the administration of living cells. They reduce the risk of blockage in small vessels or irregular growth of transplanted cells. Unlike exogenous neural progenitor stem cell transplantation, MSC-derived exosomes stimulate endogenous neural progenitor stem cells to repair the damaged brain. The use of exosomes has several significant advantages, such as:
• There is no ethical problem with embryonic cells.
• They are less invasive.
• There is little or no tumorigenesis.
Exosomes are promising therapeutic agents because their complex cargo of protein and genetic material has different biochemical potential to participate in many biochemical and cellular processes, which is an essential feature in treating complex diseases with multiple secondary injury mechanisms such as TBI (394).
Future research should focus on optimizing MSC delivery methods and exploring the long-term effects of MSC therapy on neuroplasticity and cognitive function. Additionally, understanding the molecular mechanisms underlying MSC-mediated neuroprotection could pave the way for targeted therapies.
MSCs and their EVs represent innovative and promising therapeutic strategies for treating neurological disorders, particularly TBI, ischemic strokes, concussions, tumors, encephalitis, and brain hemorrhages. Through their multifaceted mechanisms, including immunomodulation, angiogenesis, neurogenesis, anti-inflammatory properties, and apoptosis regulation, MSCs and EVs address the complex pathophysiology of neurological injuries effectively. The key insights of the article highlight the ability of MSCs to target injured brain regions, thereby reducing neuroinflammation and promoting recovery. Similarly, cell-free therapeutic agents derived from MSC-derived EVs present enhanced safety profiles and circumvent challenges associated with using MSCs, such as immunogenicity and tumorigenicity. A review of preclinical and clinical evidence strongly suggests that MSCs and EVs are promising candidates for addressing unmet therapeutic needs in brain injuries due to their regenerative potential.
These advances, however, present several challenges that must be addressed. The sources of MSCs exhibit variability, recipient responses are heterogeneous, and concerns regarding immunocompatibility and potential tumorigenic risks warrant further investigation. Additionally, there is a need for standardization and thorough validation of optimal dosing, administration routes, and long-term safety profiles.
AY: Conceptualization, Investigation, Software, Writing – original draft. MDG: Conceptualization, Supervision, Project administration, Investigation, Visualization, Writing – review & editing. RH: Investigation, Writing – original draft. AR: Writing – review & editing. NM: Writing – review & editing. HA: Methodology, Project administration, Supervision, Validation, Writing – original draft, Writing – review & editing. AF: Conceptualization, Supervision, Project administration, Investigation, Visualization, Writing – review & editing.
The author(s) declare that no financial support was received for the research, authorship, and/or publication of this article.
The authors declare that the research was conducted in the absence of any commercial or financial relationships that could be construed as a potential conflict of interest.
All claims expressed in this article are solely those of the authors and do not necessarily represent those of their affiliated organizations, or those of the publisher, the editors and the reviewers. Any product that may be evaluated in this article, or claim that may be made by its manufacturer, is not guaranteed or endorsed by the publisher.
1. Khellaf A, Khan DZ, Helmy A. Recent advances in traumatic brain injury. J Neurol. (2019) 266:2878–89. doi: 10.1007/s00415-019-09541-4
2. Maas AIR, Menon DK, Manley GT, Abrams M, Åkerlund C, Andelic N, et al. Traumatic brain injury: progress and challenges in prevention, clinical care, and research. Lancet Neurol. (2022) 21:1004–60. doi: 10.1016/S1474-4422(22)00309-X
3. Howlett JR, Nelson LD, Stein MBJ. Mental health consequences of traumatic brain injury. Biol Psychiatry. (2022) 91:413–20. doi: 10.1016/j.biopsych.2021.09.024
4. Streubel-Gallasch L, Zyśk M, Beretta C, Erlandsson A. Traumatic brain injury in the presence of Aβ pathology affects neuronal survival, glial activation and autophagy. Sci Rep. (2021) 11:1–19. doi: 10.1038/s41598-021-02371-3
5. Rostami E. Traumatic Brain Injury in Humans and Animal Models. Sweden: Karolinska Institutet (2012).
6. Dehghanian F, Soltani Z, Khaksari M. Can mesenchymal stem cells act multipotential in traumatic brain injury? J Mol Neurosci. (2020) 70:677–88. doi: 10.1007/s12031-019-01475-w
7. Dehghan F, Shahrokhi N, Khaksari M, Soltani Z, Asadikorom G, Najafi A, et al. Does the administration of melatonin during post-traumatic brain injury affect cytokine levels? Inflammopharmacology. (2018) 26:1017–23. doi: 10.1007/s10787-017-0417-1
8. Kutty SA. Intracerebral Hematoma. In: Hemorrhagic Stroke-An Update. IntechOpen. (2017). doi: 10.5772/66867
9. Perel P, Roberts I, Bouamra O, Woodford M, Mooney J, Lecky F. Intracranial bleeding in patients with traumatic brain injury: a prognostic study. BMC Emerg Med. (2009) 9:15. doi: 10.1186/1471-227X-9-15
10. Hochstadter E, Stewart TC, Alharfi IM, Ranger A, Fraser DD. Subarachnoid hemorrhage prevalence and its association with short-term outcome in pediatric severe traumatic brain injury. Neurocrit Care. (2014) 21:505–13. doi: 10.1007/s12028-014-9986-7
11. Salehi A, Zhang JH, Obenaus A. Response of the cerebral vasculature following traumatic brain injury. J Cereb Blood Flow Metabol. (2017) 37:2320–39. doi: 10.1177/0271678X17701460
12. Kota DJ, Prabhakara KS, Toledano-Furman N, Bhattarai D, Chen Q, DiCarlo B, et al. Prostaglandin E2 indicates therapeutic efficacy of mesenchymal stem cells in experimental traumatic brain injury. Stem Cells. (2017) 35:1416–30. doi: 10.1002/stem.2603
13. Kalra S, Malik R, Singh G, Bhatia S, Al-Harrasi A, Mohan S, et al. Pathogenesis and management of traumatic brain injury (TBI): role of neuroinflammation and anti-inflammatory drugs. Inflammopharmacology. (2022) 30:1153–66. doi: 10.1007/s10787-022-01017-8
14. Gurkoff G, Shahlaie K, Lyeth B, Berman R. Voltage-gated calcium channel antagonists and traumatic brain injury. Pharmaceuticals. (2013) 6:788–812. doi: 10.3390/ph6070788
15. Wells AJ, Viaroli E, Hutchinson PJ. The management of traumatic brain injury. Surgery. (2024) 42:543–52. doi: 10.1016/j.mpsur.2024.05.004
16. Dang B, Chen W, He W, Chen G. Rehabilitation treatment and progress of traumatic brain injury dysfunction. Neural Plast. (2017). 2017:1582182. doi: 10.1155/2017/1582182
17. Russo MV, McGavern DB. Inflammatory neuroprotection following traumatic brain injury. Science. (2016) 353:783–5. doi: 10.1126/science.aaf6260
18. Wang F, Tang H, Zhu J, Zhang JH. Transplanting mesenchymal stem cells for treatment of ischemic stroke. Cell Transplant. (2018) 27:1825–34. doi: 10.1177/0963689718795424
19. Cozene B, Sadanandan N, Farooq J, Kingsbury C, Park YJ, Wang Z-J, et al. Mesenchymal stem cell-induced anti-neuroinflammation against traumatic brain injury. Cell Transplant. (2021) 30:09636897211035715. doi: 10.1177/09636897211035715
20. Thomi G, Surbek D, Haesler V, Joerger-Messerli M, Schoeberlein A. Correction: exosomes derived from umbilical cord mesenchymal stem cells reduce microglia-mediated neuroinflammation in perinatal brain injury. Stem Cell Res Ther. (2022) 13:1–2. doi: 10.1186/s13287-022-03079-5
21. Zhang K, Jiang Y, Wang B, Li T, Shang D, Zhang X. Mesenchymal stem cell therapy: a potential treatment targeting pathological manifestations of traumatic brain injury. Oxid Med Cell Longev. (2022) 2022:4645021. doi: 10.1155/2022/4645021
22. Jaimes Y, Naaldijk Y, Wenk K, Leovsky C, Emmrich F. Mesenchymal stem cell-derived microvesicles modulate lipopolysaccharides-induced inflammatory responses to microglia cells. Stem Cells. (2017) 35:812–23. doi: 10.1002/stem.2541
23. Li Y, Yang Y-Y, Ren J-L, Xu F, Chen F-M, Li A. Exosomes secreted by stem cells from human exfoliated deciduous teeth contribute to functional recovery after traumatic brain injury by shifting microglia M1/M2 polarization in rats. Stem Cell Res Ther. (2017) 8:1–11. doi: 10.1186/s13287-017-0648-5
24. Turajane T, Chaveewanakorn U, Fongsarun W, Aojanepong J, Papadopoulos KI. Avoidance of total knee arthroplasty in early osteoarthritis of the knee with intra-articular implantation of autologous activated peripheral blood stem cells versus hyaluronic acid: a randomized controlled trial with differential effects of growth factor addition. Stem Cells Int. (2017). 2017:8925132. doi: 10.1155/2017/8925132
25. Wu X, Wang W, Meng C, Yang S, Duan D, Xu W, et al. Regulation of differentiation in trabecular bone-derived mesenchymal stem cells by T cell activation and inflammation. Oncol Rep. (2013) 30:2211–9. doi: 10.3892/or.2013.2687
26. Neybecker P, Henrionnet C, Pape E, Mainard D, Galois L, Loeuille D, et al. In vitro and in vivo potentialities for cartilage repair from human advanced knee osteoarthritis synovial fluid-derived mesenchymal stem cells. Stem Cell Res Ther. (2018) 9:329. doi: 10.1186/s13287-018-1071-2
27. Lee WS, Kim HJ, Kim KI, Kim GB, Jin W. Intra-articular injection of autologous adipose tissue-derived mesenchymal stem cells for the treatment of knee osteoarthritis: a phase IIb, randomized, placebo-controlled clinical trial. Stem Cells Transl Med. (2019) 8:504–11. doi: 10.1002/sctm.18-0122
28. Ilas DC, Churchman SM, Baboolal T, Giannoudis PV, Aderinto J, McGonagle D, et al. The simultaneous analysis of mesenchymal stem cells and early osteocytes accumulation in osteoarthritic femoral head sclerotic bone. Rheumatology (Oxford). (2019) 58:1777–83. doi: 10.1093/rheumatology/kez130
29. Greif DN, Kouroupis D, Murdock CJ, Griswold AJ, Kaplan LD, Best TM, et al. Infrapatellar fat pad/synovium complex in early-stage knee osteoarthritis: potential new target and source of therapeutic mesenchymal stem/stromal cells. Front Bioeng Biotechnol. (2020) 8:860. doi: 10.3389/fbioe.2020.00860
30. Cai H, Guo H. Mesenchymal stem cells and their exocytotic vesicles. Int J Mol Sci. (2023) 24:2085. doi: 10.3390/ijms24032085
31. Friedenstein A, Chailakhjan R, Lalykina KS. The development of fibroblast colonies in monolayer cultures of guinea-pig bone marrow and spleen cells. Cell Tissue Kinet. (1970) 3:393–403. doi: 10.1111/j.1365-2184.1970.tb00347.x
32. Owen M, Friedenstein A. Stromal stem cells: marrow-derived osteogenic precursors. in Ciba Foundation Symposium 136-Cell and Molecular Biology of Vertebrate Hard Tissues: Cell and Molecular Biology of Vertebrate Hard Tissues: Ciba Foundation Symposium. Wiley Online Library. (2007). doi: 10.1002/9780470513637.ch4
33. Haynesworth SE, Goshima J, Goldberg VM, Caplan AI. Characterization of cells with osteogenic potential from human marrow. Bone. (1992) 13:81–8. doi: 10.1016/8756-3282(92)90364-3
34. Lazarus HM, Haynesworth SE, Gerson SL, Rosenthal NS, Caplan AI. Ex vivo expansion and subsequent infusion of human bone marrow-derived stromal progenitor cells (mesenchymal progenitor cells): implications for therapeutic use. Bone Marrow Transplant. (1995) 16:557–64.
35. Guillamat-Prats R. The role of MSC in wound healing, scarring and regeneration. Cells. (2021) 10:1729. doi: 10.3390/cells10071729
36. Aliniay-Sharafshadehi S, Yousefi MH, Ghodratie M, Kashfi M, Afkhami H, Ghoreyshiamiri SM. Exploring the therapeutic potential of different sources of mesenchymal stem cells: novel approach to combat burn wound infections. Front Microbiol. (2024) 15:1495011. doi: 10.3389/fmicb.2024.1495011
37. Andalib E, Kashfi M, Mahmoudvand G, Rezaei E, Mahjoor M, Torki A, et al. Application of hypoxia-mesenchymal stem cells in treatment of anaerobic bacterial wound infection: wound healing and infection recovery. Front. Microbiol. (2023) 14:1251956. doi: 10.3389/fmicb.2023.1251956
38. Ma S, Xie N, Li W, Yuan B, Shi Y, Wang Y. Immunobiology of mesenchymal stem cells. Cell Death Differ. (2014) 21:216–25. doi: 10.1038/cdd.2013.158
39. Fakouri A, Razavi Z-S, Mohammed AT, Hussein AHA, Afkhami H, Hooshiar MH. Applications of mesenchymal stem cell-exosome components in wound infection healing: new insights. Burns Trauma. (2024) 12:tkae021. doi: 10.1093/burnst/tkae021
40. Farokhi S, Razavi Z-S, Mavaei M, Shadab A. New perspectives on arteriosclerosis treatment using nanoparticles and mesenchymal stem cells. Discover Appl Sci. (2024) 6:1–40. doi: 10.1007/s42452-024-06113-8
41. Jackson CJ, Tonseth KA, Utheim TP. Cultured epidermal stem cells in regenerative medicine. Stem Cell Res Ther. (2017) 8:1–7. doi: 10.1186/s13287-017-0587-1
42. Rodgers K, Jadhav S. The application of mesenchymal stem cells to treat thermal and radiation burns. Adv Drug Deliv Rev. (2018) 123:75–81. doi: 10.1016/j.addr.2017.10.003
43. Miao C, Lei M, Hu W, Han S, Wang Q. A brief review: the therapeutic potential of bone marrow mesenchymal stem cells in myocardial infarction. Stem Cell Res Ther. (2017) 8:242. doi: 10.1186/s13287-017-0697-9
44. Parmar M. Towards stem cell based therapies for Parkinson's disease. Development. (2018) 145:dev156117. doi: 10.1242/dev.156117
45. Andrzejewska A, Dabrowska S, Lukomska B, Janowski M. Mesenchymal stem cells for neurological disorders. Adv Sci (Weinh). (2021) 8:2002944. doi: 10.1002/advs.202002944
46. Kerkis I, Haddad MS, Valverde CW, Glosman S. Neural and mesenchymal stem cells in animal models of Huntington's disease: past experiences and future challenges. Stem Cell Res Ther. (2015) 6:232. doi: 10.1186/s13287-015-0248-1
47. Berlanga-Acosta JA, Guillén-Nieto GE, Rodríguez-Rodríguez N, Mendoza-Mari Y, Bringas-Vega ML, Berlanga-Saez JO, et al. Cellular senescence as the pathogenic hub of diabetes-related wound chronicity. Front Endocrinol (Lausanne). (2020) 11:573032. doi: 10.3389/fendo.2020.573032
48. Weston NM, Sun D. The potential of stem cells in treatment of traumatic brain injury. Curr Neurol Neurosci Rep. (2018) 18:1. doi: 10.1007/s11910-018-0812-z
49. Basu S, Ali H, Sangwan V. Clinical outcomes of repeat autologous cultivated limbal epithelial transplantation for ocular surface burns. Am J Ophthalmol. (2012) 153:643–50.e2. doi: 10.1016/j.ajo.2011.09.016
50. Shiels A, Hejtmancik JF. Biology of inherited cataracts and opportunities for treatment. Annu Rev Vis Sci. (2019) 5:123–49. doi: 10.1146/annurev-vision-091517-034346
51. Mahmood A, Lu D, Wang L, Li Y, Lu M, Chopp M. Treatment of traumatic brain injury in female rats with intravenous administration of bone marrow stromal cells. Neurosurgery. (2001) 49:1196–204. doi: 10.1227/00006123-200111000-00031
52. Zanier ER, Montinaro M, Vigano M, Villa P, Fumagalli S, Pischiutta F, et al. Human umbilical cord blood mesenchymal stem cells protect mice brain after trauma. Crit Care Med. (2011) 39:2501–10. doi: 10.1097/CCM.0b013e31822629ba
53. Han Y, Yang J, Fang J, Zhou Y, Candi E, Wang J, et al. The secretion profile of mesenchymal stem cells and potential applications in treating human diseases. Signal Transduct Target Ther. (2022) 7:92. doi: 10.1038/s41392-022-00932-0
54. Wang M, Xu X, Lei X, Tan J, Xie H. Mesenchymal stem cell-based therapy for burn wound healing. Burns Trauma. (2021) 9:tkab002. doi: 10.1093/burnst/tkab002
55. Herman S, Fishel I. Intranasal delivery of mesenchymal stem cells-derived extracellular vesicles for the treatment of neurological diseases. Stem Cells. (2021) 39:1589–600. doi: 10.1002/stem.3456
56. Isaković J, Chin BD, Oberwinter M, Rance HK. Mesenchymal stem cell therapy for neurological disorders: the light or the dark side of the force? Front Bioeng Biotechnol. (2023) 11:1139359. doi: 10.3389/fbioe.2023.1139359
57. Li X, Guan Y, Li C, Zhang T, Meng F, Zhang J, et al. Immunomodulatory effects of mesenchymal stem cells in peripheral nerve injury. Stem Cell Res Ther. (2022) 13:18. doi: 10.1186/s13287-021-02690-2
58. Zhou T, Yuan Z, Weng J, Pei D, Du X, He C, et al. Challenges and advances in clinical applications of mesenchymal stromal cells. J Hematol Oncol. (2021) 14:24. doi: 10.1186/s13045-021-01037-x
59. Yang G, Fan X, Liu Y, Jie P, Mazhar M, Liu Y, et al. Immunomodulatory mechanisms and therapeutic potential of mesenchymal stem cells. Stem Cell Rev Rep. (2023) 19:1214–31. doi: 10.1007/s12015-023-10539-9
60. Afkhami H, Mahmoudvand G, Fakouri A, Shadab A, Mahjoor M, Movahhed TK. New insights in application of mesenchymal stem cells therapy in tumor microenvironment: Pros and cons. Front Cell Dev Biol. (2023) 11:1255697. doi: 10.3389/fcell.2023.1255697
61. Yang C, Hawkins KE, Doré S. Candelario-Jalil E. Vascular and cellular pathophysiology of stroke: neuroinflammatory mechanisms of blood-brain barrier damage in ischemic stroke. Am J Physiol Cell Physiol. (2019) 316:C135. doi: 10.1152/ajpcell.00136.2018
62. He J, Zhang N, Zhu Y, Jin R, Wu F. MSC spheroids-loaded collagen hydrogels simultaneously promote neuronal differentiation and suppress inflammatory reaction through PI3K-Akt signaling pathway. Biomaterials. (2021) 265:120448. doi: 10.1016/j.biomaterials.2020.120448
63. Mahjoor M, Afkhami H, Najafi M, Nasr A, Khorrami S. The role of microRNA-30c in targeting interleukin 6, as an inflammatory cytokine, in the mesenchymal stem cell: a therapeutic approach in colorectal cancer. J Cancer Res Clin Oncol. (2023) 149:3149–60. doi: 10.1007/s00432-022-04123-w
64. Mahjoor M, Fakouri A, Farokhi S, Nazari H, Afkhami H, Heidari F. Regenerative potential of mesenchymal stromal cells in wound healing: unveiling the influence of normoxic and hypoxic environments. Front Cell Dev Biol. (2023) 11:1245872. doi: 10.3389/fcell.2023.1245872
65. Zhang Z, Huang S, Wu S, Qi J, Li W, Liu S, et al. Clearance of apoptotic cells by mesenchymal stem cells contributes to immunosuppression via PGE2. EBioMedicine. (2019) 45:341–50. doi: 10.1016/j.ebiom.2019.06.016
66. Mahmoudvand G, Rouzbahani AK, Razavi ZS, Mahjoor M, Afkhami H. Mesenchymal stem cell therapy for non-healing diabetic foot ulcer infection: new insight. Front Bioeng Biotechnol. (2023) 11:1158484. doi: 10.3389/fbioe.2023.1158484
67. Mirshekar M, Afkhami H, Razavi S, Masjedian Jazi F, Darban-Sarokhalil D, Ohadi E, et al. Potential antibacterial activity and healing effect of topical administration of bone marrow and adipose mesenchymal stem cells encapsulated in collagen-fibrin hydrogel scaffold on full-thickness burn wound infection caused by Pseudomonas aeruginosa. Burns. (2023) 49:1944–57. doi: 10.1016/j.burns.2023.01.005
68. Rozenberg A, Rezk A, Boivin M-N, Darlington PJ, Nyirenda M, Li R, et al. Human mesenchymal stem cells impact Th17 and Th1 responses through a prostaglandin E2 and myeloid-dependent mechanism. Stem Cells Transl Med. (2016) 5:1506–14. doi: 10.5966/sctm.2015-0243
69. Lucas SM, Rothwell NJ, Gibson RM. The role of inflammation in CNS injury and disease. Br J Pharmacol. (2006) 147:S232–40. doi: 10.1038/sj.bjp.0706400
70. Aggarwal S, Pittenger MF. Human mesenchymal stem cells modulate allogeneic immune cell responses. Blood. (2005) 105:1815–22. doi: 10.1182/blood-2004-04-1559
71. Chen Y, Shao JZ, Xiang LX, Dong XJ, Zhang GR. Mesenchymal stem cells: a promising candidate in regenerative medicine. Int J Biochem Cell Biol. (2008) 40:815–20. doi: 10.1016/j.biocel.2008.01.007
72. Wei L, Fraser JL, Lu ZY, Hu X, Yu SP. Transplantation of hypoxia preconditioned bone marrow mesenchymal stem cells enhances angiogenesis and neurogenesis after cerebral ischemia in rats. Neurobiol Dis. (2012) 46:635–45. doi: 10.1016/j.nbd.2012.03.002
73. Abbasi-Kangevari M, Ghamari S-H, Safaeinejad F, Bahrami S, Niknejad H. Potential therapeutic features of human amniotic mesenchymal stem cells in multiple sclerosis: immunomodulation, inflammation suppression, angiogenesis promotion, oxidative stress inhibition, neurogenesis induction, MMPs regulation, and remyelination stimulation. Front Immunol. (2019) 10:238. doi: 10.3389/fimmu.2019.00238
74. Hasan A, Deeb G, Rahal R, Atwi K, Mondello S, Marei HE, et al. Mesenchymal stem cells in the treatment of traumatic brain injury. Front Neurol. (2017) 8:28. doi: 10.3389/fneur.2017.00028
75. Klyushnenkova E, Mosca JD, Zernetkina V, Majumdar MK, Beggs KJ, Simonetti DW, et al. T cell responses to allogeneic human mesenchymal stem cells: immunogenicity, tolerance, and suppression. J Biomed Sci. (2005) 12:47–57. doi: 10.1007/s11373-004-8183-7
76. Jacobs SA, Roobrouck VD, Verfaillie CM, Van Gool SW. Immunological characteristics of human mesenchymal stem cells and multipotent adult progenitor cells. Immunol Cell Biol. (2013) 91:32–9. doi: 10.1038/icb.2012.64
77. Abdal Dayem A, Lee SB, Kim K, Lim KM, Jeon TI, Seok J, et al. Production of mesenchymal stem cells through stem cell reprogramming. Int J Mol Sci. (2019) 20:1922. doi: 10.3390/ijms20081922
78. Kot M, Baj-Krzyworzeka M, Szatanek R, Musiał-Wysocka A, Suda-Szczurek M, Majka M. The importance of HLA assessment in “off-the-shelf” allogeneic mesenchymal stem cells based-therapies. Int J Mol Sci. (2019) 20:5680. doi: 10.3390/ijms20225680
79. Jhunjhunwala S, Hammer C, Delamarre L. Antigen presentation in cancer: insights into tumour immunogenicity and immune evasion. Nat Rev Cancer. (2021) 21:298–312. doi: 10.1038/s41568-021-00339-z
80. Yang XF, Chen T, Ren LW, Yang L, Qi H, Li FR. Immunogenicity of insulin-producing cells derived from human umbilical cord mesenchymal stem cells. Exp Ther Med. (2017) 13:1456–64. doi: 10.3892/etm.2017.4096
81. Le Blanc K, Tammik C, Rosendahl K, Zetterberg E, Ringdén O. HLA expression and immunologic propertiesof differentiated and undifferentiated mesenchymal stem cells. Exp Hematol. (2003) 31:890–6. doi: 10.1016/S0301-472X(03)00110-3
82. Agudo J, Park ES, Rose SA, Alibo E, Sweeney R, Dhainaut M, et al. Quiescent tissue stem cells evade immune surveillance. Immunity. (2018) 48:271–85.e5. doi: 10.1016/j.immuni.2018.02.001
83. Yu X, Liu P, Li Z, Zhang Z. Function and mechanism of mesenchymal stem cells in the healing of diabetic foot wounds. Front Endocrinol (Lausanne). (2023) 14:1099310. doi: 10.3389/fendo.2023.1099310
84. Galland S, Stamenkovic I. Mesenchymal stromal cells in cancer: a review of their immunomodulatory functions and dual effects on tumor progression. J Pathol. (2020) 250:555–72. doi: 10.1002/path.5357
85. Pittenger MF, Discher DE, Péault BM, Phinney DG, Hare JM, Caplan AI. Mesenchymal stem cell perspective: cell biology to clinical progress. NPJ Regen Med. (2019) 4:22. doi: 10.1038/s41536-019-0083-6
86. Pittenger MF, Mackay AM, Beck SC, Jaiswal RK, Douglas R, Mosca JD, et al. Multilineage potential of adult human mesenchymal stem cells. Science. (1999) 284:143–7. doi: 10.1126/science.284.5411.143
87. Gao Q, Wang L, Wang S, Huang B, Jing Y, Su J. Bone marrow mesenchymal stromal cells: identification, classification, and differentiation. Front Cell Dev Biol. (2022) 9:787118. doi: 10.3389/fcell.2021.787118
88. Bhat S, Viswanathan P, Chandanala S, Prasanna SJ, Seetharam RN. Expansion and characterization of bone marrow derived human mesenchymal stromal cells in serum-free conditions. Sci Rep. (2021) 11:3403. doi: 10.1038/s41598-021-83088-1
89. Charbord P. Bone marrow mesenchymal stem cells: historical overview and concepts. Hum Gene Ther. (2010) 21:1045–56. doi: 10.1089/hum.2010.115
90. Baghaei K, Hashemi SM, Tokhanbigli S, Ras AS. Isolation, differentiation, and characterization of mesenchymal stem cells from human bone marrow. Gastroenterol Hepatol Bed Bench. (2017) 10:208. doi: 10.22037/ghfbb.v0i0.1089
91. Kim DW, Staples M, Shinozuka K, Pantcheva P, Kang SD, Borlongan CV. Wharton's jelly-derived mesenchymal stem cells: phenotypic characterization and optimizing their therapeutic potential for clinical applications. Int J Mol Sci. (2013) 14:11692–712. doi: 10.3390/ijms140611692
92. Mebarki M, Abadie C, Larghero J, Cras A. Human umbilical cord-derived mesenchymal stem/stromal cells: a promising candidate for the development of advanced therapy medicinal products. Stem Cell Res Ther. (2021) 12:571. doi: 10.1186/s13287-021-02222-y
93. Bieback K, Kern S, Klüter H, Eichler H. Critical parameters for the isolation of mesenchymal stem cells from umbilical cord blood. Stem Cells. (2004) 22:625–34. doi: 10.1634/stemcells.22-4-625
94. Jin HJ, Bae YK, Kim M, Kwon SJ, Jeon HB, Choi SJ, et al. Comparative analysis of human mesenchymal stem cells from bone marrow, adipose tissue, and umbilical cord blood as sources of cell therapy. Int J Mol Sci. (2013) 14:17986–8001. doi: 10.3390/ijms140917986
95. Ryu H-H, Kang B-J, Park S-S, Kim Y, Sung G-J, Woo H-M, et al. Comparison of mesenchymal stem cells derived from fat, bone marrow, Wharton's jelly, and umbilical cord blood for treating spinal cord injuries in dogs. J Vet Med Sci. (2012) 2012:0065. doi: 10.1292/jvms.12-0065
96. Sabapathy V, Sundaram B, SV M, Mankuzhy P, Kumar S. Human Wharton's jelly mesenchymal stem cells plasticity augments scar-free skin wound healing with hair growth. PLoS ONE. (2014) 9:e93726. doi: 10.1371/journal.pone.0093726
97. Shang Y, Guan H, Zhou F. Biological characteristics of umbilical cord mesenchymal stem cells and its therapeutic potential for hematological disorders. Front Cell Dev Biol. (2021) 9:570179. doi: 10.3389/fcell.2021.570179
98. Mohamed-Ahmed S, Fristad I, Lie SA, Suliman S, Mustafa K, Vindenes H, et al. Adipose-derived and bone marrow mesenchymal stem cells: a donor-matched comparison. Stem Cell Res Ther. (2018) 9:168. doi: 10.1186/s13287-018-0914-1
99. Gruber HE, Somayaji S, Riley F, Hoelscher GL, Norton HJ, Ingram J, et al. Human adipose-derived mesenchymal stem cells: serial passaging, doubling time and cell senescence. Biotech Histochem. (2012) 87:303–11. doi: 10.3109/10520295.2011.649785
100. Huang SJ, Fu RH, Shyu WC, Liu SP, Jong GP, Chiu YW, et al. Adipose-derived stem cells: isolation, characterization, and differentiation potential. Cell Transplant. (2013) 22:701–9. doi: 10.3727/096368912X655127
101. Krawczenko A, Klimczak AJ. Adipose tissue-derived mesenchymal stem/stromal cells and their contribution to angiogenic processes in tissue regeneration. Int J Mol Sci. (2022) 23:2425. doi: 10.3390/ijms23052425
102. Suga H, Matsumoto D, Eto H, Inoue K, Aoi N, Kato H, et al. Functional implications of CD34 expression in human adipose–derived stem/progenitor cells. Stem Cells Dev. (2009) 18:1201–10. doi: 10.1089/scd.2009.0003
103. Mazini L, Ezzoubi M, Malka G. Overview of current adipose-derived stem cell (ADSCs) processing involved in therapeutic advancements: flow chart and regulation updates before and after COVID-19. Stem Cell Res Ther. (2021) 12:1. doi: 10.1186/s13287-020-02006-w
104. Bunnell BA. Adipose tissue-derived mesenchymal stem cells. MDPI. 2021, 3433. doi: 10.3390/cells10123433
105. Mohamed-Ahmed S, Yassin MA, Rashad A, Espedal H, Idris SB, Finne-Wistrand A, et al. Comparison of bone regenerative capacity of donor-matched human adipose–derived and bone marrow mesenchymal stem cells. Cell Tissue Res. (2021) 383:1061–75. doi: 10.1007/s00441-020-03315-5
106. Oliveira MS, Barreto-Filho JB. Placental-derived stem cells: Culture, differentiation and challenges. World J Stem Cells. (2015) 7:769. doi: 10.4252/wjsc.v7.i4.769
107. Macias MI, Grande J, Moreno A, Domínguez I, Bornstein R, Flores AI. Isolation and characterization of true mesenchymal stem cells derived from human term decidua capable of multilineage differentiation into all 3 embryonic layers. Am J Obstet Gynecol. (2010) 203:495.e9–495.e23. doi: 10.1016/j.ajog.2010.06.045
108. Siddesh SE, Gowda DM, Jain R, Gulati A, Patil GS, Anudeep N, et al. Placenta-derived mesenchymal stem cells (P-MSCs) for COVID-19 pneumonia—a regenerative dogma. Stem Cell Investig. (2021) 8:3. doi: 10.21037/sci-2020-034
109. Abumaree MH, Jumah MAA, Kalionis B, Jawdat D, Khaldi AA, Abomaray FM, et al. Human placental mesenchymal stem cells (pMSCs) play a role as immune suppressive cells by shifting macrophage differentiation from inflammatory M1 to anti-inflammatory M2 macrophages. Stem Cell Rev Rep. (2013) 9:620–41. doi: 10.1007/s12015-013-9455-2
110. Campagnoli C, Roberts IA, Kumar S, Bennett PR, Bellantuono I, Fisk NM. Identification of mesenchymal stem/progenitor cells in human first-trimester fetal blood, liver, and bone marrow. Blood. (2001) 98:2396–402. doi: 10.1182/blood.V98.8.2396
111. Zhang Q, Shi S, Liu Y, Uyanne J, Shi Y, Shi S, et al. Mesenchymal stem cells derived from human gingiva are capable of immunomodulatory functions and ameliorate inflammation-related tissue destruction in experimental colitis. J Immunol. (2009) 183:7787–98. doi: 10.4049/jimmunol.0902318
112. Li D, Zou X-Y, El-Ayachi I, Romero LO Yu Z, Iglesias-Linares A, et al. Human dental pulp stem cells and gingival mesenchymal stem cells display action potential capacity in vitro after neuronogenic differentiation. Stem Cell Rev Rep. (2019) 15:67–81. doi: 10.1007/s12015-018-9854-5
113. Girija DM, Kalachaveedu M, Rao SR, Subbarayan R. Transdifferentiation of human gingival mesenchymal stem cells into functional keratinocytes by Acalypha indica in three-dimensional microenvironment. J Cell Physiol. (2018) 233:8450–7. doi: 10.1002/jcp.26807
114. Luo Y, Wu W, Gu J, Zhang X, Dang J, Wang J, et al. Human gingival tissue-derived MSC suppress osteoclastogenesis and bone erosion via CD39-adenosine signal pathway in autoimmune arthritis. (2019) 43:620–31. doi: 10.1016/j.ebiom.2019.04.058
115. Rosa-Ruiz MDPD, Álvarez-Pérez MA, Cortés-Morales VA, Monroy-García A, Mayani H, Fragoso-González G, et al. Mesenchymal stem/stromal cells derived from dental tissues: a comparative in vitro evaluation of their immunoregulatory properties against T cells. Cells. (2019) 8:1491. doi: 10.3390/cells8121491
116. Zhang X, Huang F, Li W, Dang J-L, Yuan J, Wang J, et al. Human gingiva-derived mesenchymal stem cells modulate monocytes/macrophages and alleviate atherosclerosis. Front Immunol. (2018) 9:878. doi: 10.3389/fimmu.2018.00878
117. Andreadis D, Bakopoulou A, Leyhausen G, Epivatianos A, Volk J, Markopoulos A, et al. Minor salivary glands of the lips: a novel, easily accessible source of potential stem/progenitor cells. Clin Oral Investig. (2014) 18:847–56. doi: 10.1007/s00784-013-1056-6
118. Lu L, Li Y, Du MJ, Zhang C, Zhang XY, Tong HZ, et al. Characterization of a self-renewing and multi-potent cell population isolated from human minor salivary glands. Sci Rep. (2015) 5:10106. doi: 10.1038/srep10106
119. Wang S-Q, Wang Y-X, Hua H. Characteristics of labial gland mesenchymal stem cells of healthy individuals and patients with sjögren's syndrome: a preliminary study. Stem Cells Dev. (2017) 26:1171–85. doi: 10.1089/scd.2017.0045
120. Sato A, Okumura K, Matsumoto S, Hattori K, Hattori S, Shinohara M, et al. Isolation, tissue localization, and cellular characterization of progenitors derived from adult human salivary glands. Cloning Stem Cells. (2007) 9:191–205. doi: 10.1089/clo.2006.0054
121. Tatsuishi Y, Hirota M, Kishi T, Adachi M, Fukui T, Mitsudo K, et al. Human salivary gland stem/progenitor cells remain dormant even after irradiation. Int J Mol Med. (2009) 24:361–6. doi: 10.3892/ijmm_00000240
122. Xu J, Su Y, Hu L, Cain A, Gu Y, Liu B, et al. Effect of bone morphogenetic protein 6 on Immunomodulatory functions of salivary gland-derived Mesenchymal stem cells in Sjögren's syndrome. Stem Cells Dev. (2018) 27:1540–8. doi: 10.1089/scd.2017.0161
123. Li B, Xing Y, Gan Y, He J, Hua H. Labial gland-derived mesenchymal stem cells and their exosomes ameliorate murine Sjögren's syndrome by modulating the balance of Treg and Th17 cells. Stem Cell Res Ther. (2021) 12:478. doi: 10.1186/s13287-021-02541-0
124. McCoy SS, Giri J, Das R, Paul PK, Pennati A, Parker M, et al. Minor salivary gland mesenchymal stromal cells derived from patients with Sj?gren's syndrome deploy intact immune plasticity. Cytotherapy. (2021) 23:301–10. doi: 10.1016/j.jcyt.2020.09.008
125. Davies LC, Alm JJ, Heldring N, Moll G, Gavin C, Batsis I, et al. Type 1 diabetes mellitus donor mesenchymal stromal cells exhibit comparable potency to healthy controls in vitro. Stem Cells Transl Med. (2016) 5:1485–95. doi: 10.5966/sctm.2015-0272
126. Sávio-Silva C, Beyerstedt S, Soinski-Sousa PE, Casaro EB, Balby-Rocha MTA, Simplício-Filho A, et al. Mesenchymal stem cell therapy for diabetic kidney disease: a review of the studies using syngeneic, autologous, allogeneic, and xenogeneic cells. Stem Cells Int. (2020) 2020:8833725. doi: 10.1155/2020/8833725
127. Eleuteri S, Fierabracci AJ. Insights into the secretome of mesenchymal stem cells and its potential applications. Int J Mol Sci. (2019) 20:4597. doi: 10.3390/ijms20184597
128. Ahangar P, Mills SJ, Cowin AJ. Mesenchymal stem cell secretome as an emerging cell-free alternative for improving wound repair. Int J Mol Sci. (2020) 21:7038. doi: 10.3390/ijms21197038
129. Pinho AG, Cibrão JR, Silva NA, Monteiro S, Salgado AJ. Cell secretome: basic insights and therapeutic opportunities for CNS disorders. Pharmaceuticals (Basel). (2020) 13:31. doi: 10.3390/ph13020031
130. Muhammad SA, Abbas AY, Imam MU, Saidu Y, Bilbis LS. Efficacy of stem cell secretome in the treatment of traumatic brain injury: a systematic review and meta-analysis of preclinical studies. Mol Neurobiol. (2022) 59:2894–909. doi: 10.1007/s12035-022-02759-w
131. Gould SJ, Raposo GJ. As we wait: coping with an imperfect nomenclature for extracellular vesicles. J Extracell Vesicles. (2013) 2:20389. doi: 10.3402/jev.v2i0.20389
132. Théry C, Witwer KW, Aikawa E, Alcaraz MJ, Anderson JD, Andriantsitohaina R, et al. Minimal information for studies of extracellular vesicles 2018 (MISEV2018): a position statement of the International Society for Extracellular Vesicles and update of the MISEV2014 guidelines. J Extracell Vesicles. (2018) 7:1535750. doi: 10.1080/20013078.2018.1535750
133. Witwer KW, Théry CJ. Extracellular vesicles or exosomes? On primacy, precision, and popularity influencing a choice of nomenclature. J Extracell Vesicles. (2019) 18:1648167. doi: 10.1080/20013078.2019.1648167
134. Keerthikumar S, Chisanga D, Ariyaratne D, Al Saffar H, Anand S, Zhao K, et al. ExoCarta: a web-based compendium of exosomal cargo. J Mol Biol. (2016) 428:688–92. doi: 10.1016/j.jmb.2015.09.019
135. Zappulli V, Friis KP, Fitzpatrick Z, Maguire CA, Breakefield XO. Extracellular vesicles and intercellular communication within the nervous system. J Clin Invest. (2016) 126:1198–207. doi: 10.1172/JCI81134
136. Liu YJ, Wang CJ. A review of the regulatory mechanisms of extracellular vesicles-mediated intercellular communication. Cell Commun Signal. (2023) 21:77. doi: 10.1186/s12964-023-01103-6
137. Jafarinia M, Alsahebfosoul F, Salehi H, Eskandari N, Ganjalikhani-Hakemi M. Mesenchymal stem cell-derived extracellular vesicles: a novel cell-free therapy. Immunol Invest. (2020) 49:758–80. doi: 10.1080/08820139.2020.1712416
138. Zhang B, Yin Y, Lai RC, Tan SS, Choo AB, Lim SK. Mesenchymal stem cells secrete immunologically active exosomes. Stem Cells Dev. (2014) 23:1233–44. doi: 10.1089/scd.2013.0479
139. Zhuang W-Z, Lin Y-H, Su L-J, Wu M-S, Jeng H-Y, Chang H-C, et al. Mesenchymal stem/stromal cell-based therapy: mechanism, systemic safety and biodistribution for precision clinical applications. J Biomed Sci. (2021) 28:1–38. doi: 10.1186/s12929-021-00725-7
140. Watanabe Y, Tsuchiya A, Terai S. The development of mesenchymal stem cell therapy in the present, and the perspective of cell-free therapy in the future. Clin Mol Hepatol. (2021) 27:70. doi: 10.3350/cmh.2020.0194
141. Bazzoni R, Kamga PT, Tanasi I, Krampera M. Extracellular vesicle-dependent communication between mesenchymal stromal cells and immune effector cells. Front Cell Dev Biol. (2020) 8:596079. doi: 10.3389/fcell.2020.596079
142. Racchetti G, Meldolesi J. Extracellular vesicles of mesenchymal stem cells: therapeutic properties discovered with extraordinary success. Biomedicines. (2021) 9:667. doi: 10.3390/biomedicines9060667
143. Albert-Weißenberger C, Sirén AL, Kleinschnitz C. Ischemic stroke and traumatic brain injury: The role of the kallikrein–kinin system. Prog Neurobiol. (2013) 101:65–82. doi: 10.1016/j.pneurobio.2012.11.004
144. Lapchak PA, Zhang JH. The high cost of stroke and stroke cytoprotection research. Translat Stroke Res. (2017) 8:307–17. doi: 10.1007/s12975-016-0518-y
145. Tagliaferri F, Compagnone C, Korsic M, Servadei F, Kraus J. A systematic review of brain injury epidemiology in Europe. Acta Neurochir. (2006) 148:255–68. doi: 10.1007/s00701-005-0651-y
146. Go AS, Mozaffarian D, Roger VL, Benjamin EJ, Berry JD, Borden WB, et al. Heart disease and stroke statistics-−2013 update: a report from the American Heart Association. Circulation. (2013) 127:e6–245. doi: 10.1161/CIR.0b013e31828124ad
147. Agrawal N, Johnston SC, Wu YW, Sidney S, Fullerton HJ. Imaging data reveal a higher pediatric stroke incidence than prior US estimates. Stroke. (2009) 40:3415–21. doi: 10.1161/STROKEAHA.109.564633
148. Lynch JK, Hirtz DG, DeVeber G, Nelson KB. Report of the national institute of neurological disorders and stroke workshop on perinatal and childhood stroke. Pediatrics. (2002) 109:116–23. doi: 10.1542/peds.109.1.116
149. Ganesan V, Prengler M, McShane MA, Wade AM, Kirkham FJ. Investigation of risk factors in children with arterial ischemic stroke. Ann Neurol. (2003) 53:167–73. doi: 10.1002/ana.10423
150. Shi Y, Leak RK, Keep RF, Chen J. Translational stroke research on blood-brain barrier damage: challenges, perspectives, and goals. Transl Stroke Res. (2016) 7:89–92. doi: 10.1007/s12975-016-0447-9
151. Schuhmann MK, Stegner D, Berna-Erro A, Bittner S, Braun A, Kleinschnitz C, et al. Stromal interaction molecules 1 and 2 are key regulators of autoreactive T cell activation in murine autoimmune central nervous system inflammation. J Immunol. (2010) 184:1536–42. doi: 10.4049/jimmunol.0902161
152. Tuttolomondo A, Pecoraro R, Casuccio A, Di Raimondo D, Buttà C, Clemente G, et al. Peripheral frequency of CD4+ CD28– cells in acute ischemic stroke: Relationship with stroke subtype and severity markers. Medicine. (2015) 94:e813. doi: 10.1097/MD.0000000000000813
153. Hsuan YC-Y, Lin C-H, Chang C-P, Lin M-T. Mesenchymal stem cell-based treatments for stroke, neural trauma, and heat stroke. Brain Behav. (2016) 6:e00526. doi: 10.1002/brb3.526
154. Barone FC, Parsons AA. Therapeutic potential of anti-inflammatory drugs in focal stroke. Expert Opin Investig Drugs. (2000) 9:2281–306. doi: 10.1517/13543784.9.10.2281
155. Lambertsen KL, Biber K, Finsen B. Inflammatory cytokines in experimental and human stroke. J Cereb Blood Flow Metab. (2012) 32:1677–98. doi: 10.1038/jcbfm.2012.88
156. Kolias AG, Kirkpatrick PJ, Hutchinson PJ. Decompressive craniectomy: past, present and future. Nat Rev Neurol. (2013) 9:405–15. doi: 10.1038/nrneurol.2013.106
157. Kalladka D, Muir KW. Brain repair: cell therapy in stroke. Stem Cells Clon. (2014) 7:31. doi: 10.2147/SCCAA.S38003
158. Gholizadeh O, Yasamineh S, Amini P, Afkhami H, Delarampour A, Akbarzadeh S, et al. Therapeutic and diagnostic applications of nanoparticles in the management of COVID-19: a comprehensive overview. Virol J. (2022) 19:1–22. doi: 10.1186/s12985-022-01935-7
159. Yasamineh S, Kalajahi HG, Yasamineh P, Yazdani Y, Gholizadeh O, Tabatabaie R, et al. An overview on nanoparticle-based strategies to fight viral infections with a focus on COVID-19. J Nanobiotechnology. (2022) 20:1–26. doi: 10.1186/s12951-022-01625-0
160. Picard-Riera N, Decker L, Delarasse C, Goude K, Nait-Oumesmar B, Liblau R, et al. Experimental autoimmune encephalomyelitis mobilizes neural progenitors from the subventricular zone to undergo oligodendrogenesis in adult mice. Proc Natl Acad Sci USA. (2002) 99:13211–6. doi: 10.1073/pnas.192314199
161. Saberiyan M, Safi A, Kamel A, Movahhed-Abbasabad P, Miralimalek M, Afkhami H, et al. An overview on the common laboratory parameter alterations and their related molecular pathways in screening for COVID-19 patients. Clin Lab. (2020) 66:705. doi: 10.7754/Clin.Lab.2020.200705
162. Donega V, van Velthoven CTJ, Nijboer CH, van Bel F, Kas MJH, Kavelaars A, et al. Intranasal mesenchymal stem cell treatment for neonatal brain damage: long-term cognitive and sensorimotor improvement. PLoS ONE. (2013) 8:e51253. doi: 10.1371/journal.pone.0051253
163. Gervois P, Wolfs E, Ratajczak J, Dillen Y, Vangansewinkel T, Hilkens P, et al. Stem cell-based therapies for ischemic stroke: preclinical results and the potential of imaging-assisted evaluation of donor cell fate and mechanisms of brain regeneration. Med Res Rev. (2016) 36:1080–126. doi: 10.1002/med.21400
164. Zhang S, Lachance BB, Moiz B, Jia X. Optimizing stem cell therapy after ischemic brain injury. J Stroke. (2020) 22:286. doi: 10.5853/jos.2019.03048
165. Nagpal A, Choy FC, Howell S, Hillier S, Chan F, Hamilton-Bruce MA, et al. Safety and effectiveness of stem cell therapies in early-phase clinical trials in stroke: a systematic review and meta-analysis. Stem Cell Res Ther. (2017) 8:191. doi: 10.1186/s13287-017-0643-x
166. Levy ML, Crawford JR, Dib N, Verkh L, Tankovich N, Cramer SC. Phase I/II study of safety and preliminary efficacy of intravenous allogeneic mesenchymal stem cells in chronic stroke. Stroke. (2019) 50:2835–41. doi: 10.1161/STROKEAHA.119.026318
167. Dabrowska S, Andrzejewska A, Lukomska B, Janowski M. Neuroinflammation as a target for treatment of stroke using mesenchymal stem cells and extracellular vesicles. J Neuroinflammation. (2019) 16:178. doi: 10.1186/s12974-019-1571-8
168. Savitz SI, Misra V, Kasam M, Juneja H, Cox CS Jr, Alderman S, et al. Intravenous autologous bone marrow mononuclear cells for ischemic stroke. Ann Neurol. (2011) 70:59–69. doi: 10.1002/ana.22458
169. Lucia Maria Ferri A, Bersano A, Lisini D, Boncoraglio G, Frigerio S, Parati E. Mesenchymal stem cells for ischemic stroke: progress and possibilities. Curr Med Chem. (2016) 23:1598–608. doi: 10.2174/0929867323666160222113702
170. Qiao L, Huang FJ, Zhao M, Xie JH, Shi J, Wang J, et al. A two-year follow-up study of cotransplantation with neural stem/progenitor cells and mesenchymal stromal cells in ischemic stroke patients. Cell Transplant. (2014) 23:65–72. doi: 10.3727/096368914X684961
171. Lee J, Chang WH, Chung J-W, Kim SJ, Kim S-K, Lee JS, et al. Efficacy of intravenous mesenchymal stem cells for motor recovery after ischemic stroke: a neuroimaging study. Stroke. (2022) 53:20–8. doi: 10.1161/STROKEAHA.121.034505
172. Jaillard A, Hommel M, Moisan A, Zeffiro TA, Favre-Wiki IM, Barbieux-Guillot M, et al. Autologous mesenchymal stem cells improve motor recovery in subacute ischemic stroke: a randomized clinical trial. Transl Stroke Res. (2020) 11:910–23. doi: 10.1007/s12975-020-00787-z
173. Law ZK, Tan HJ, Chin SP, Wong CY, Wan Yahya WNN, Muda AS, et al. The effects of intravenous infusion of autologous mesenchymal stromal cells in patients with subacute middle cerebral artery infarct: a phase 2 randomized controlled trial on safety, tolerability and efficacy. Cytotherapy. (2021) 23:833–40. doi: 10.1016/j.jcyt.2021.03.005
174. Chung JW, Chang WH, Bang OY, Moon GJ, Kim SJ, Kim SK, et al. Efficacy and safety of intravenous mesenchymal stem cells for ischemic stroke. Neurology. (2021) 96:e1012–23. doi: 10.1212/WNL.0000000000011440
175. Chen C-Y, Lin P-T, Wang Y-H, Syu R-W, Hsu S-L, Chang L-H, et al. Etiology and risk factors of intracranial hemorrhage and ischemic stroke in young adults. J Chin Med Assoc. (2021) 84:930–6. doi: 10.1097/JCMA.0000000000000598
177. Banerjee G, Carare R, Cordonnier C, Greenberg SM, Schneider JA, Smith EE, et al. The increasing impact of cerebral amyloid angiopathy: essential new insights for clinical practice. J Neurol Neurosurg Psychiatry. (2017) 88:982–94. doi: 10.1136/jnnp-2016-314697
178. Hilkens NA, van Asch CJJ, Werring DJ, Wilson D, Rinkel GJE, Algra A, et al. Predicting the presence of macrovascular causes in non-traumatic intracerebral haemorrhage: the DIAGRAM prediction score. J Neurol Neurosurg Psychiatry. (2018) 89:674–9. doi: 10.1136/jnnp-2017-317262
179. Wilson D, Charidimou A, Werring DJ. Advances in understanding spontaneous intracerebral hemorrhage: insights from neuroimaging. Exp Rev Neurother. (2014) 14:661–78. doi: 10.1586/14737175.2014.918506
180. Zhu X, Yan T, Cheng C, Ma J, Xiang J, Lv Y, et al. Mesenchymal Stem Cells (MSCs) in targeted drug delivery: literature review and exploratory data on migrating and differentiation capacities of bone MSCs into hepatic progenitor cells. Curr Top Med Chem. (2021) 21:1251–67. doi: 10.2174/1568026621666210708092728
181. Yang G, Fan X, Mazhar M, Yang S, Xu H, Dechsupa N, et al. Mesenchymal stem cell application and its therapeutic mechanisms in intracerebral hemorrhage. Front Cell Neurosci. (2022) 16:898497. doi: 10.3389/fncel.2022.898497
182. Wang C, Fei Y, Xu C, Zhao Y, Pan Y. Bone marrow mesenchymal stem cells ameliorate neurological deficits and blood-brain barrier dysfunction after intracerebral hemorrhage in spontaneously hypertensive rats. Int J Clin Exp Pathol. (2015) 8:4715.
183. Bao X-J, Liu F-Y, Lu S, Han Q, Feng M, Wei J-J, et al. Transplantation of Flk-1+ human bone marrow-derived mesenchymal stem cells promotes behavioral recovery and anti-inflammatory and angiogenesis effects in an intracerebral hemorrhage rat model. Int J Mol Med. (2013) 31:1087–96. doi: 10.3892/ijmm.2013.1290
184. Azevedo RI, Minskaia E, Fernandes-Platzgummer A, Vieira AIS, da Silva CL, Cabral JMS, et al. Mesenchymal stromal cells induce regulatory T cells via epigenetic conversion of human conventional CD4 T cells in vitro. Stem Cells. (2020) 38:1007–19. doi: 10.1002/stem.3185
185. Luo J, Shi Y, Yao Y, Hao J. PD-L1 (programmed death ligand 1) protects against experimental intracerebral hemorrhage–induced brain injury. Stroke. (2017) 48:2255–62. doi: 10.1161/STROKEAHA.117.016705
186. Fujiwara H, Maeda Y, Kobayashi K, Nishimori H, Matsuoka K-I, Fujii N, et al. Programmed death-1 pathway in host tissues ameliorates Th17/Th1-mediated experimental chronic graft-versus-host disease. J Immunol. (2014) 193:2565–73. doi: 10.4049/jimmunol.1400954
187. Ding Y, Han R, Jiang W, Xiao J, Liu H, Chen X, et al. Programmed death ligand 1 plays a neuroprotective role in experimental autoimmune neuritis by controlling peripheral nervous system inflammation of rats. J Immunol. (2016) 197:3831–40. doi: 10.4049/jimmunol.1601083
188. Gong Y, Hao Sl, Wang B. Mesenchymal stem cells transplantation in intracerebral hemorrhage: application and challenges. Front Cell Neurosci. (2021) 15:653367. doi: 10.3389/fncel.2021.653367
189. Li J, Xiao L, He D, Luo Y, Sun H. Mechanism of white matter injury and promising therapeutic strategies of MSCs after intracerebral hemorrhage. Front Aging Neurosci. (2021) 13:632054. doi: 10.3389/fnagi.2021.632054
190. Kim J-M, Lee S-T, Chu K, Jung K-H, Song E-C, Kim S-J, et al. Systemic transplantation of human adipose stem cells attenuated cerebral inflammation and degeneration in a hemorrhagic stroke model. Brain Res. (2007) 1183:43–50. doi: 10.1016/j.brainres.2007.09.005
191. Kopen GC, Prockop DJ, Phinney DG. Marrow stromal cells migrate throughout forebrain and cerebellum, and they differentiate into astrocytes after injection into neonatal mouse brains. Proc Natl Acad Sci USA. (1999) 96:10711–6. doi: 10.1073/pnas.96.19.10711
192. Gao L, Xu W, Li T, Chen J, Shao A, Yan F, et al. Stem cell therapy: a promising therapeutic method for intracerebral hemorrhage. Cell Transplant. (2018) 27:1809–24. doi: 10.1177/0963689718773363
193. Wislet-Gendebien S, Hans G, Leprince P, Rigo J-M, Moonen G, Rogister B. Plasticity of cultured mesenchymal stem cells: switch from nestin-positive to excitable neuron-like phenotype. Stem Cells. (2005) 23:392–402. doi: 10.1634/stemcells.2004-0149
194. Nagai A, Kim WK, Lee HJ, Jeong HS, Kim KS, Hong SH, et al. Multilineage potential of stable human mesenchymal stem cell line derived from fetal marrow. PLoS ONE. (2007) 2:e1272. doi: 10.1371/journal.pone.0001272
195. Bae KS, Park JB, Kim HS, Kim DS, Park DJ, Kang SJ. Neuron-like differentiation of bone marrow-derived mesenchymal stem cells. Yonsei Med J. (2011) 52:401–12. doi: 10.3349/ymj.2011.52.3.401
196. Chen M, Li X, Zhang X, He X, Lai L, Liu Y, et al. The inhibitory effect of mesenchymal stem cell on blood–brain barrier disruption following intracerebral hemorrhage in rats: contribution of TSG-6. J Neuroinflammation. (2015) 12:1–14. doi: 10.1186/s12974-015-0284-x
197. Chen J, Tang Y-X, Liu Y-M, Chen J, Hu X-Q, Liu N, et al. Transplantation of adipose-derived stem cells is associated with neural differentiation and functional improvement in a rat model of intracerebral hemorrhage. CNS Neurosci Ther. (2012) 18:847–54. doi: 10.1111/j.1755-5949.2012.00382.x
198. Yang K-L, Lee J-T, Pang C-Y, Lee T-Y, Chen S-P, Liew H-K, et al. Human adipose-derived stem cells for the treatment of intracerebral hemorrhage in rats via femoral intravenous injection. Cell Mol Biol Lett. (2012) 17:376–92. doi: 10.2478/s11658-012-0016-5
199. Chen X, Xu C-X, Liang H, Xi Z, Pan J, Yang Y, et al. Bone marrow mesenchymal stem cells transplantation alleviates brain injury after intracerebral hemorrhage in mice through the Hippo signaling pathway. Aging (Albany NY). (2020) 12:6306. doi: 10.18632/aging.103025
200. Yang Y, Ren J, Sun Y, Xue Y, Zhang Z, Gong A, et al. A connexin43/YAP axis regulates astroglial-mesenchymal transition in hemoglobin induced astrocyte activation. Cell Death Different. (2018) 25:1870–84. doi: 10.1038/s41418-018-0137-0
201. Huang Z, Sun D, Hu J-X, Tang F-L, Lee D-H, Wang Y, et al. Neogenin promotes BMP2 activation of YAP and Smad1 and enhances astrocytic differentiation in developing mouse neocortex. J Neurosci. (2016) 36:5833–49. doi: 10.1523/JNEUROSCI.4487-15.2016
202. Wang Y. “The Hippo pathway in tissue homeostasis and regeneration”. Protein Cell. (2017) 8:349–59. doi: 10.1007/s13238-017-0371-0
203. Harmon KG, Clugston JR, Dec K, Hainline B, Herring SA, Kane S, et al. American Medical Society for Sports Medicine position statement: concussion in sport. Clin J Sport Med. (2013) 23:87–100. doi: 10.1097/JSM.0b013e31827f5f93
204. Harmon KG, Clugston JR, Dec K, Hainline B, Herring SA, Kane S, et al. American Medical Society for Sports Medicine position statement on concussion in sport. Br J Sports Med. (2019) 53:213–25. doi: 10.1136/bjsports-2018-100338
205. Barkhoudarian G, Hovda DA, Giza CC. The molecular pathophysiology of concussive brain injury–an update. Phys Med Rehabilit Clin. (2016) 27:373–93. doi: 10.1016/j.pmr.2016.01.003
206. McCrory P, Meeuwisse WH, Dvorák J, Echemendia RJ, Engebretsen L, Feddermann-Demont N, et al. 5th international conference on concussion in sport (Berlin). Br J Sports Med. (2017) 51:837. doi: 10.1136/bjsports-2017-097878
207. Hering C, Shetty AK. Extracellular vesicles derived from neural stem cells, astrocytes, and microglia as therapeutics for easing TBI-induced brain dysfunction. Stem Cells Transl Med. (2023) 12:140–53. doi: 10.1093/stcltm/szad004
208. Willing AE, Das M, Howell M, Mohapatra SS, Mohapatra S. Potential of mesenchymal stem cells alone, or in combination, to treat traumatic brain injury. CNS Neurosci Ther. (2020) 26:616–27. doi: 10.1111/cns.13300
209. Tian C, Wang X, Wang X, Wang L, Wang X, Wu S, et al. Autologous bone marrow mesenchymal stem cell therapy in the subacute stage of traumatic brain injury by lumbar puncture. Exp Clin Transplant. (2013) 11:176–81. doi: 10.6002/ect.2012.0053
210. Echemendia RJ, Gioia GA. Role of neuropsychologists in the evaluation and management of sport-related concussion: an inter-organization position statement. Arch Clin Neuropsychol. (2012) 27:119–22. doi: 10.1093/arclin/acr077
211. Ellis MJ, Leddy J, Willer B. Multi-disciplinary management of athletes with post-concussion syndrome: an evolving pathophysiological approach. Front Neurol. (2016) 7:136. doi: 10.3389/fneur.2016.00136
212. Mckee AC, Abdolmohammadi B, Stein TD. The neuropathology of chronic traumatic encephalopathy. Handb Clin Neurol. (2018) 158:297–307. doi: 10.1016/B978-0-444-63954-7.00028-8
213. Purcell LK, Davis GA, Gioia GA. What factors must be considered in ‘return to school'following concussion and what strategies or accommodations should be followed? A systematic review. Br J Sports Med. (2019) 53:250–250. doi: 10.1136/bjsports-2017-097853
214. Wilkins SA, Shannon CN, Brown ST, Vance EH, Ferguson D, Gran K, et al. Establishment of a multidisciplinary concussion program: impact of standardization on patient care and resource utilization. J Neurosurg. (2014) 13:82–9. doi: 10.3171/2013.10.PEDS13241
215. Mahmood A, Lu D, Chopp M. Intravenous administration of marrow stromal cells (MSCs) increases the expression of growth factors in rat brain after traumatic brain injury. J Neurotr. (2004) 21:33–9. doi: 10.1089/089771504772695922
216. Wang S, Kan Q, Sun Y, Han R, Zhang G, Peng T, et al. Caveolin-1 regulates neural differentiation of rat bone mesenchymal stem cells into neurons by modulating Notch signaling. Int J Dev Neurosci. (2013) 31:30–5. doi: 10.1016/j.ijdevneu.2012.09.004
217. Vlassov AV, Magdaleno S, Setterquist R, Conrad R. Exosomes: current knowledge of their composition, biological functions, and diagnostic and therapeutic potentials. Biochim Biophys Acta. (2012) 1820:940–8. doi: 10.1016/j.bbagen.2012.03.017
218. Mokarizadeh A, Delirezh N, Morshedi A, Mosayebi G, Farshid A-A, Mardani K. Microvesicles derived from mesenchymal stem cells: potent organelles for induction of tolerogenic signaling. Immunol Lett. (2012) 147:47–54. doi: 10.1016/j.imlet.2012.06.001
219. Yáñez-Mó M, Siljander PR, Andreu Z, Zavec AB, Borràs FE, Buzas EI, et al. Biological properties of extracellular vesicles and their physiological functions. J Extracell Vesicles. (2015) 4:27066. doi: 10.3402/jev.v4.27066
220. Gregorius J, Wang C, Stambouli O, Hussner T, Qi Y, Tertel T, et al. Small extracellular vesicles obtained from hypoxic mesenchymal stromal cells have unique characteristics that promote cerebral angiogenesis, brain remodeling and neurological recovery after focal cerebral ischemia in mice. Basic Res Cardiol. (2021) 116:1–19. doi: 10.1007/s00395-021-00881-9
221. Konari N, Nagaishi K, Kikuchi S, Fujimiya M. Mitochondria transfer from mesenchymal stem cells structurally and functionally repairs renal proximal tubular epithelial cells in diabetic nephropathy in vivo. Sci Rep. (2019) 9:1–14. doi: 10.1038/s41598-019-40163-y
222. Calió ML, Marinho DS, Ko GM, Ribeiro RR, Carbonel AF, Oyama LM, et al. Transplantation of bone marrow mesenchymal stem cells decreases oxidative stress, apoptosis, and hippocampal damage in brain of a spontaneous stroke model. Free Radical Biol Med. (2014) 70:141–54. doi: 10.1016/j.freeradbiomed.2014.01.024
223. Paliwal S, Chaudhuri R, Agrawal A, Mohanty S. Regenerative abilities of mesenchymal stem cells through mitochondrial transfer. J Biomed Sci. (2018) 25:1–12. doi: 10.1186/s12929-018-0429-1
224. Ratajczak J, Wysoczynski M, Hayek F, Janowska-Wieczorek A, Ratajczak MZ. Membrane-derived microvesicles: important and underappreciated mediators of cell-to-cell communication. Leukemia. (2006) 20:1487–95. doi: 10.1038/sj.leu.2404296
225. Rodriguez A-M, Nakhle J, Griessinger E, Vignais M-L. Intercellular mitochondria trafficking highlighting the dual role of mesenchymal stem cells as both sensors and rescuers of tissue injury. Cell Cycle. (2018) 17:712–21. doi: 10.1080/15384101.2018.1445906
226. Vignais M-L, Caicedo A, Brondello J-M, Jorgensen C. Cell connections by tunneling nanotubes: effects of mitochondrial trafficking on target cell metabolism, homeostasis, and response to therapy. Stem Cells Int. (2017) 2017:6917941. doi: 10.1155/2017/6917941
227. Shi X, Bai Y, Zhang G, Liu Y, Xiao H, Liu X, et al. Effects of over-expression of SOD2 in bone marrow-derived mesenchymal stem cells on traumatic brain injury. Cell Tissue Res. (2018) 372:67–75. doi: 10.1007/s00441-017-2716-7
228. Vargo M. Brain tumor rehabilitation. Am J Phys Med Rehabil. (2011) 90:S50–S62. doi: 10.1097/PHM.0b013e31820be31f
229. Young RM, Jamshidi A, Davis G, Sherman JH. Current trends in the surgical management and treatment of adult glioblastoma. Ann Transl Med. (2015) 3:121. doi: 10.3978/j.issn.2305-5839.2015.05.10
230. Yarahmadi A, Zare M, Aghayari M, Afkhami H, Jafari GA. Therapeutic bacteria and viruses to combat cancer: double-edged sword in cancer therapy: new insights for future. Cell Commun Signal. (2024) 22:239. doi: 10.1186/s12964-024-01622-w
231. Gallego O. Nonsurgical treatment of recurrent glioblastoma. Curr Oncol. (2015) 22:273–81. doi: 10.3747/co.22.2436
232. Javan MR, Khosrojerdi A, Moazzeni SM. New insights into implementation of mesenchymal stem cells in cancer therapy: prospects for anti-angiogenesis treatment. Front Oncol. (2019) 9:840. doi: 10.3389/fonc.2019.00840
233. Wei L, Li L, Liu L, Yu R, Li X, Luo Z. Knockdown of Annexin-A1 inhibits growth, migration and invasion of glioma cells by suppressing the PI3K/Akt signaling pathway. ASN Neuro. (2021) 13:17590914211001218. doi: 10.1177/17590914211001218
234. Eiro N, Fraile M, Fernández-Francos S, Sánchez R, Costa LA, Vizoso FJ. Importance of the origin of mesenchymal (stem) stromal cells in cancer biology: “alliance” or “war” in intercellular signals. Cell Biosci. (2021) 11:109. doi: 10.1186/s13578-021-00620-6
235. Shah K. Mesenchymal stem cells engineered for cancer therapy. Adv Drug Deliv Rev. (2012) 64:739–48. doi: 10.1016/j.addr.2011.06.010
236. Amsalem Z, Arif T, Shteinfer-Kuzmine A, Chalifa-Caspi V, Shoshan-Barmatz V. The mitochondrial protein VDAC1 at the crossroads of cancer cell metabolism: the epigenetic link. Cancers. (2020) 12:1031. doi: 10.3390/cancers12041031
237. Gilazieva Z, Ponomarev A, Rizvanov A, Solovyeva V. The dual role of mesenchymal stromal cells and their extracellular vesicles in carcinogenesis. Biology. (2022) 11:813. doi: 10.3390/biology11060813
238. Nowak B, Rogujski P, Janowski M, Lukomska B, Andrzejewska A. Mesenchymal stem cells in glioblastoma therapy and progression: how one cell does it all. Biochim Biophys Acta Rev Cancer. (2021) 1876:188582. doi: 10.1016/j.bbcan.2021.188582
239. Barin N, Balcioglu HE, de Heer I, de Wit M, Lamfers MLM, van Royen ME, et al. 3D-engineered scaffolds to study microtubes and localization of epidermal growth factor receptor in patient-derived glioma cells. Small. (2022) 2022:2204485. doi: 10.1002/smll.202204485
240. Calinescu A-A, Kauss MC, Sultan Z, Al-Holou WN, O'Shea SK. Stem cells for the treatment of glioblastoma: a 20-year perspective. CNS Oncol. (2021) 10:CNS73. doi: 10.2217/cns-2020-0026
241. Shimizu Y, Gumin J, Gao F, Hossain A, Shpall EJ, Kondo A, et al. Human bone marrow–derived mesenchymal stem cells for intravascular delivery of oncolytic adenovirus Δ24-RGD to human gliomas. Cancer Res. (2009) 69:8932–40. doi: 10.1158/0008-5472.CAN-08-3873
242. Yang K, Wu Z, Zhang H, Zhang N, Wu W, Wang Z, et al. Glioma targeted therapy: insight into future of molecular approaches. Mol Cancer. (2022) 21:39. doi: 10.1186/s12943-022-01513-z
243. Harrell CR, Volarevic A, Djonov VG, Jovicic N, Volarevic V. Mesenchymal stem cell: a friend or foe in anti-tumor immunity. Int J Mol Sci. (2021) 22:12429. doi: 10.3390/ijms222212429
244. Fisher DL, Defres S, Solomon T. Measles-induced encephalitis. QJM. (2014) 108:177–82. doi: 10.1093/qjmed/hcu113
245. Alam AM, Easton A, Nicholson TR, Irani SR, Davies NWS, Solomon T, et al. Encephalitis: diagnosis, management and recent advances in the field of encephalitides. Postgrad Med J. (2023) 99:815–25. doi: 10.1136/postgradmedj-2022-141812
246. Uy CE, Binks S, Irani SR. Autoimmune encephalitis: clinical spectrum and management. Pract Neurol. (2021) 21:412–23. doi: 10.1136/practneurol-2020-002567
247. Armangue T, Petit-Pedrol M, Dalmau J. Autoimmune encephalitis in children. J Child Neurol. (2012) 27:1460–9. doi: 10.1177/0883073812448838
248. Kennedy P. Viral encephalitis: causes, differential diagnosis, and management. J Neurol Neurosurg Psychiatry. (2004) 75:i10–5. doi: 10.1136/jnnp.2003.034280
249. Lima MN, Barbosa-Silva MC, Maron-Gutierrez TJ. New perspectives for mesenchymal stromal cells as an adjuvant therapy for infectious disease-associated encephalopathies. Neural Regen Res. (2022) 17:48–52. doi: 10.4103/1673-5374.314292
250. Fathollahi A, Hashemi SM, Hoseini MHM, Tavakoli S, Farahani E, Yeganeh F. Intranasal administration of small extracellular vesicles derived from mesenchymal stem cells ameliorated the experimental autoimmune encephalomyelitis. Int Immunopharmacol. (2021) 90:107207. doi: 10.1016/j.intimp.2020.107207
251. Trounson A, McDonald C. Stem cell therapies in clinical trials: progress and challenges. Cell Stem Cell. (2015) 17:11–22. doi: 10.1016/j.stem.2015.06.007
252. Chamberlain G, Fox J, Ashton B, Middleton J. Concise review: mesenchymal stem cells: their phenotype, differentiation capacity, immunological features, and potential for homing. Stem Cells. (2007) 25:2739–49. doi: 10.1634/stemcells.2007-0197
253. Donega V, Nijboer CH, Braccioli L, Slaper-Cortenbach I, Kavelaars A, van Bel F, et al. Intranasal administration of human MSC for ischemic brain injury in the mouse: in vitro and in vivo neuroregenerative functions. PLoS ONE. (2014) 9:e112339. doi: 10.1371/journal.pone.0112339
254. Yang Z, Cai X, Xu A, Xu F, Liang Q. Bone marrow stromal cell transplantation through tail vein injection promotes angiogenesis and vascular endothelial growth factor expression in cerebral infarct area in rats. Cytotherapy. (2015) 17:1200–12. doi: 10.1016/j.jcyt.2015.06.005
255. Martino G, Franklin RJ, Baron Van Evercooren A, Kerr DA. Stem cell transplantation in multiple sclerosis: current status and future prospects. Nat Rev Neurol. (2010) 6:247–55. doi: 10.1038/nrneurol.2010.35
256. Song M-S, Learman CR, Ahn K-C, Baker GB, Kippe J, Field EM, et al. In vitro validation of effects of BDNF-expressing mesenchymal stem cells on neurodegeneration in primary cultured neurons of APP/PS1 mice. Neuroscience. (2015) 307:37–50. doi: 10.1016/j.neuroscience.2015.08.011
257. Waterman RS, Tomchuck SL, Henkle SL, Betancourt AM. A new mesenchymal stem cell (MSC) paradigm: polarization into a pro-inflammatory MSC1 or an Immunosuppressive MSC2 phenotype. PLoS ONE. (2010) 5:e10088. doi: 10.1371/journal.pone.0010088
258. Bernardo ME, Fibbe WE. Mesenchymal stromal cells: sensors and switchers of inflammation. Cell Stem Cell. (2013) 13:392–402. doi: 10.1016/j.stem.2013.09.006
259. Yasamineh S, Kalajahi HG, Yasamineh P, Gholizadeh O, Youshanlouei HR, Matloub SK, et al. Spotlight on therapeutic efficiency of mesenchymal stem cells in viral infections with a focus on COVID-19. Stem Cell Res Ther. (2022) 13:257. doi: 10.1186/s13287-022-02944-7
260. Harrell CR, Jovicic BP, Djonov V, Volarevic V. Molecular mechanisms responsible for mesenchymal stem cell-based treatment of viral diseases. Pathogens. (2021) 10:409. doi: 10.3390/pathogens10040409
261. Bian P, Ye C, Zheng X, Yang J, Ye W, Wang Y, et al. Mesenchymal stem cells alleviate Japanese encephalitis virus-induced neuroinflammation and mortality. Stem Cell Res Ther. (2017) 8:1–13. doi: 10.1186/s13287-017-0486-5
262. Liu Y, Zhang R, Yan K, Chen F, Huang W, Lv B, et al. Mesenchymal stem cells inhibit lipopolysaccharide-induced inflammatory responses of BV2 microglial cells through TSG-6. J Neuroinflam. (2014) 11:1–12. doi: 10.1186/1742-2094-11-135
263. He Z, Hua J, Song Z. Concise review: mesenchymal stem cells ameliorate tissue injury via secretion of tumor necrosis factor-α stimulated protein/gene 6. Stem Cells Int. (2014) 2014:761091. doi: 10.1155/2014/761091
264. Chan MCW, Kuok DIT, Leung CYH, Hui KPY, Valkenburg SA, Lau EHY, et al. Human mesenchymal stromal cells reduce influenza A H5N1-associated acute lung injury in vitro and in vivo. Proc Natl Acad Sci USA. (2016) 113:3621–6. doi: 10.1073/pnas.1601911113
265. Sung DK, Chang YS, Sung SI, Yoo HS, Ahn SY, Park WS. Antibacterial effect of mesenchymal stem cells against Escherichia coli is mediated by secretion of beta-defensin-2 via toll-like receptor 4 signalling. Cell Microbiol. (2016) 18:424–36. doi: 10.1111/cmi.12522
266. Krasnodembskaya A, Song Y, Fang X, Gupta N, Serikov V, Lee J-W, et al. Antibacterial effect of human mesenchymal stem cells is mediated in part from secretion of the antimicrobial peptide LL-37. Stem Cells. (2010) 28:2229–38. doi: 10.1002/stem.544
267. Khaledi M, Sameni F, Yahyazade S, Radandish M, Owlia P, Bagheri N, et al. COVID-19 and the potential of Janus Family kinase (JAK) Pathway Inhibition: as a novel treatment strategy. Front Med. (2022) 9:961027. doi: 10.3389/fmed.2022.961027
268. Mei SH, Haitsma JJ, Dos Santos CC, Deng Y, Lai PF, Slutsky AS, et al. Mesenchymal stem cells reduce inflammation while enhancing bacterial clearance and improving survival in sepsis. Am J Respir Crit Care Med. (2010) 182:1047–57. doi: 10.1164/rccm.201001-0010OC
269. Aoki K, Shimada S, Simantini DS, Tun MM, Buerano CC, Morita K, et al. Type-I interferon response affects an inoculation dose-independent mortality in mice following Japanese encephalitis virus infection. Virol J. (2014) 11:1–7. doi: 10.1186/1743-422X-11-105
270. Thanunchai M, Hongeng S, Thitithanyanont A. Mesenchymal stromal cells and viral infection. Stem Cells Int. (2015) 2015:860950. doi: 10.1155/2015/860950
271. Yavagal DR, Lin B, Raval AP, Garza PS, Dong C, Zhao W, et al. Efficacy and dose-dependent safety of intra-arterial delivery of mesenchymal stem cells in a rodent stroke model. PLoS ONE. (2014) 9:e93735. doi: 10.1371/journal.pone.0093735
272. Sarmah D, Agrawal V, Rane P, Bhute S, Watanabe M, Kalia K, et al. Mesenchymal stem cell therapy in Ischemic stroke: a meta-analysis of preclinical studies. Clin Pharmacol Ther. (2018) 103:990–8. doi: 10.1002/cpt.927
273. Dharmasaroja P. Bone marrow-derived mesenchymal stem cells for the treatment of ischemic stroke. J Clin Neurosci. (2009) 16:12–20. doi: 10.1016/j.jocn.2008.05.006
274. Huang P, Freeman WD, Edenfield BH, Brott TG, Meschia JF, Zubair AC. Safety and efficacy of intraventricular delivery of bone marrow-derived mesenchymal stem cells in hemorrhagic stroke model. Sci Rep. (2019) 9:5674. doi: 10.1038/s41598-019-42182-1
275. Bedini G, Bersano A, Zanier ER, Pischiutta F, Parati EA. Mesenchymal stem cell therapy in intracerebral haemorrhagic stroke. Curr Med Chem. (2018) 25:2176–97. doi: 10.2174/0929867325666180111101410
276. Mello TG, Rosado-de-Castro PH, Campos RMP, Vasques JF, Rangel-Junior WS, de Mattos RSdR, et al. Intravenous human umbilical cord-derived mesenchymal stromal cell administration in models of moderate and severe intracerebral hemorrhage. Stem Cells Dev. (2020) 29:586–98. doi: 10.1089/scd.2019.0176
277. Schepici G, Silvestro S, Bramanti P, Mazzon E. Traumatic brain injury and stem cells: an overview of clinical trials, the current treatments and future therapeutic approaches. Medicina (Kaunas). (2020) 56:137. doi: 10.3390/medicina56030137
278. Bagno LL, Salerno AG, Balkan W, Hare JM. Mechanism of action of mesenchymal stem cells (MSCs): impact of delivery method. Expert Opin Biol Ther. (2022) 22:449–63. doi: 10.1080/14712598.2022.2016695
279. Yousefi F, Ebtekar M, Soleimani M, Soudi S, Hashemi SM. Comparison of in vivo immunomodulatory effects of intravenous and intraperitoneal administration of adipose-tissue mesenchymal stem cells in experimental autoimmune encephalomyelitis (EAE). Int Immunopharmacol. (2013) 17:608–16. doi: 10.1016/j.intimp.2013.07.016
280. Harting MT, Jimenez F, Xue H, Fischer UM, Baumgartner J, Dash PK, et al. Intravenous mesenchymal stem cell therapy for traumatic brain injury. J Neurosurg. (2009) 110:1189–97. doi: 10.3171/2008.9.JNS08158
281. Kumar Mishra S, Khushu S, Gangenahalli GJ. Neuroprotective response and efficacy of intravenous administration of mesenchymal stem cells in traumatic brain injury mice. Eur J Neurosci. (2021) 54:4392–407. doi: 10.1111/ejn.15261
282. Du S, Guan J, Mao G, Liu Y, Ma S, Bao X, et al. Intra-arterial delivery of human bone marrow mesenchymal stem cells is a safe and effective way to treat cerebral ischemia in rats. Cell Transplant. (2014) 23:73–82. doi: 10.3727/096368914X685023
283. Kim H, Na DL, Lee NK, Kim AR, Lee S, Jang H. Intrathecal injection in a rat model: a potential route to deliver human Wharton's jelly-derived mesenchymal stem cells into the brain. Int J Mol Sci. (2020) 21:1272. doi: 10.3390/ijms21041272
284. Gutiérrez-Fernández M, Rodríguez-Frutos B, Ramos-Cejudo J, Vallejo-Cremades MT, Fuentes B, Cerdán S, et al. Effects of intravenous administration of allogenic bone marrow-and adipose tissue-derived mesenchymal stem cells on functional recovery and brain repair markers in experimental ischemic stroke. Stem Cell Res Ther. (2013) 4:1–12. doi: 10.1186/scrt159
285. Sugiyama Y, Sato Y, Kitase Y, Suzuki T, Kondo T, Mikrogeorgiou A, et al. Intravenous administration of bone marrow-derived mesenchymal stem cell, but not adipose tissue-derived stem cell, ameliorated the neonatal hypoxic-ischemic brain injury by changing cerebral inflammatory state in rat. Front Neurol. (2018) 9:757. doi: 10.3389/fneur.2018.00757
286. Guzman R, Janowski M, Walczak P. Intra-arterial delivery of cell therapies for stroke. Stroke. (2018) 49:1075–82. doi: 10.1161/STROKEAHA.117.018288
287. Spiliopoulos S, Festas G, Reppas L, Brountzos E. Intra-arterial administration of cell-based biological agents for ischemic stroke therapy. Expert Opin Biol Ther. (2019) 19:249–59. doi: 10.1080/14712598.2019.1566454
288. Li L, Jiang Q, Ding G, Zhang L, Zhang ZG, Li Q, et al. Effects of administration route on migration and distribution of neural progenitor cells transplanted into rats with focal cerebral ischemia, an MRI study. J Cereb Blood Flow Metab. (2010) 30:653–62. doi: 10.1038/jcbfm.2009.238
289. Watanabe M, Yavagal DR. Intra-arterial delivery of mesenchymal stem cells. Brain Circ. (2016) 2:114–7. doi: 10.4103/2394-8108.192522
290. Zhang H-L, Xie X-F, Xiong Y-Q, Liu S-M, Hu G-Z, Cao W-F, et al. Comparisons of the therapeutic effects of three different routes of bone marrow mesenchymal stem cell transplantation in cerebral ischemic rats. Brain Res. (2018) 1680:143–54. doi: 10.1016/j.brainres.2017.12.017
291. Zhang Y, Zhang Y, Chopp M, Zhang ZG, Mahmood A, Xiong Y. Mesenchymal stem cell–derived exosomes improve functional recovery in rats after traumatic brain injury: a dose-response and therapeutic window study. Neurorehabil Neural Repair. (2020) 34:616–26. doi: 10.1177/1545968320926164
292. Moore TL, Bowley BGE, Pessina MA, Calderazzo SM, Medalla M, Go V, et al. Mesenchymal derived exosomes enhance recovery of motor function in a monkey model of cortical injury. Restor Neurol Neurosci. (2019) 37:347–62. doi: 10.3233/RNN-190910
293. Xiong Y, Mahmood A, Chopp M. Angiogenesis, neurogenesis and brain recovery of function following injury. Curr Opin Investig Drugs. (2010) 11:298.
294. Patel K, Sun D. Strategies targeting endogenous neurogenic cell response to improve recovery following traumatic brain injury. Brain Res. (2016) 1640:104–13. doi: 10.1016/j.brainres.2016.01.055
295. Fares J, Diab ZB, Nabha S, Fares Y. Neurogenesis in the adult hippocampus: history, regulation, and prospective roles. Int J Neurosci. (2019) 129:598–611. doi: 10.1080/00207454.2018.1545771
296. Zhang Y, Chopp M, Meng Y, Katakowski M, Xin H, Mahmood A, et al. Effect of exosomes derived from multipluripotent mesenchymal stromal cells on functional recovery and neurovascular plasticity in rats after traumatic brain injury. J Neurosurg. (2015) 122:856–67. doi: 10.3171/2014.11.JNS14770
297. Zhang L, Zhang ZG, Chopp M. The neurovascular unit and combination treatment strategies for stroke. Trends Pharmacol Sci. (2012) 33:415–22. doi: 10.1016/j.tips.2012.04.006
298. Yang Y, Ye Y, Su X, He J, Bai W, He X. MSCs-derived exosomes and neuroinflammation, neurogenesis and therapy of traumatic brain injury. Front Cell Neurosci. (2017) 11:55. doi: 10.3389/fncel.2017.00055
299. Liu Y-Y, Li Y, Wang L, Zhao Y, Yuan R, Yang M-M, et al. Mesenchymal stem cell-derived exosomes regulate microglia phenotypes: a promising treatment for acute central nervous system injury. Neural Regen Res. (2023) 18:1657–65. doi: 10.4103/1673-5374.363819
300. Dumbrava D-A, Surugiu R, Börger V, Ruscu M, Tertel T, Giebel B, et al. Mesenchymal stromal cell-derived small extracellular vesicles promote neurological recovery and brain remodeling after distal middle cerebral artery occlusion in aged rats. Geroscience. (2022) 44:293–310. doi: 10.1007/s11357-021-00483-2
301. Han Y, Seyfried D, Meng Y, Yang D, Schultz L, Chopp M, et al. Multipotent mesenchymal stromal cell–derived exosomes improve functional recovery after experimental intracerebral hemorrhage in the rat. J Neurosurg. (2018) 131:290–300. doi: 10.3171/2018.2.JNS171475
302. Xin H, Li Y, Cui Y, Yang JJ, Zhang ZG, Chopp M. Systemic administration of exosomes released from mesenchymal stromal cells promote functional recovery and neurovascular plasticity after stroke in rats. J Cereb Blood Flow Metab. (2013) 33:1711–5. doi: 10.1038/jcbfm.2013.152
303. Zhang ZG, Chopp M. Exosomes in stroke pathogenesis and therapy. J Clin Invest. (2016) 126:1190–7. doi: 10.1172/JCI81133
304. Venkat P, Chen J, Chopp M. Exosome-mediated amplification of endogenous brain repair mechanisms and brain and systemic organ interaction in modulating neurological outcome after stroke. J Cereb Blood Flow Metab. (2018) 38:2165–78. doi: 10.1177/0271678X18782789
305. Zhang ZG, Buller B, Chopp M. Exosomes—beyond stem cells for restorative therapy in stroke and neurological injury. Nat Rev Neurol. (2019) 15:193–203. doi: 10.1038/s41582-018-0126-4
306. Zhang L, Mao L, Wang H. The neuroprotection effects of exosome in central nervous system injuries: a new target for therapeutic intervention. Mol Neurobiol. (2022) 59:7152–69. doi: 10.1007/s12035-022-03028-6
307. Park SY, Kim DS, Kim HM, Lee JK, Hwang DY, Kim TH, et al. Human mesenchymal stem cell-derived extracellular vesicles promote neural differentiation of neural progenitor cells. Int J Mol Sci. (2022) 23:7047. doi: 10.3390/ijms23137047
308. Kuang Y, Zheng X, Zhang L, Ai X, Venkataramani V, Kilic E, et al. Adipose-derived mesenchymal stem cells reduce autophagy in stroke mice by extracellular vesicle transfer of miR-25. J Extracell Vesicles. (2020) 10:e12024. doi: 10.1002/jev2.12024
309. Doeppner TR, Herz J, Görgens A, Schlechter J, Ludwig A-K, Radtke S, et al. Extracellular vesicles improve post-stroke neuroregeneration and prevent postischemic immunosuppression. Stem Cells Transl Med. (2015) 4:1131–43. doi: 10.5966/sctm.2015-0078
310. Dabrowska S, Andrzejewska A, Strzemecki D, Muraca M, Janowski M, Lukomska B. Human bone marrow mesenchymal stem cell-derived extracellular vesicles attenuate neuroinflammation evoked by focal brain injury in rats. J Neuroinflammation. (2019) 16:216. doi: 10.1186/s12974-019-1602-5
311. Shi X, Zhong X, Deng L, Wu X, Zhang P, Zhang X, et al. Mesenchymal stem cell-derived extracellular vesicle-enclosed microRNA-93 prevents hypoxic-ischemic brain damage in rats. Neuroscience. (2022) 500:12–25. doi: 10.1016/j.neuroscience.2022.06.037
312. Thomi G, Joerger-Messerli M, Haesler V, Muri L, Surbek D, Schoeberlein A. Intranasally administered exosomes from umbilical cord stem cells have preventive neuroprotective effects and contribute to functional recovery after perinatal brain injury. Cells. (2019) 8:855. doi: 10.3390/cells8080855
313. Mot YY, Moses EJ, Mohd Yusoff N, Ling KH, Yong YK, Tan JJ. Mesenchymal stromal cells-derived exosome and the roles in the treatment of traumatic brain injury. Cell Mol Neurobiol. (2023) 43:469–89. doi: 10.1007/s10571-022-01201-y
314. Xiong Y, Mahmood A, Chopp M. Emerging potential of exosomes for treatment of traumatic brain injury. Neural Regen Res. (2017) 12:19–22. doi: 10.4103/1673-5374.198966
315. Shi M, Sheng L, Stewart T, Zabetian CP, Zhang J. New windows into the brain: central nervous system-derived extracellular vesicles in blood. Prog Neurobiol. (2019) 175:96–106. doi: 10.1016/j.pneurobio.2019.01.005
316. Budnik V, Ruiz-Cañada C, Wendler F. Extracellular vesicles round off communication in the nervous system. Nat Rev Neurosci. (2016) 17:160–72. doi: 10.1038/nrn.2015.29
317. Ni H, Yang S, Siaw-Debrah F, Hu J, Wu K, He Z, et al. Exosomes derived from bone mesenchymal stem cells ameliorate early inflammatory responses following traumatic brain injury. Front Neurosci. (2019) 13:14. doi: 10.3389/fnins.2019.00014
318. Williams AM, Bhatti UF, Brown JF, Biesterveld BE, Kathawate RG, Graham NJ, et al. Early single-dose treatment with exosomes provides neuroprotection and improves blood-brain barrier integrity in swine model of traumatic brain injury and hemorrhagic shock. J Trauma Acute Care Surg. (2020) 88:207–18. doi: 10.1097/TA.0000000000002563
319. Williams AM, Dennahy IS, Bhatti UF, Halaweish I, Xiong Y, Chang P, et al. Mesenchymal stem cell-derived exosomes provide neuroprotection and improve long-term neurologic outcomes in a swine model of traumatic brain injury and hemorrhagic shock. J Neurotrauma. (2019) 36:54–60. doi: 10.1089/neu.2018.5711
320. Medalla M, Chang W, Calderazzo SM, Go V, Tsolias A, Goodliffe JW, et al. Treatment with mesenchymal-derived extracellular vesicles reduces injury-related pathology in pyramidal neurons of monkey perilesional ventral premotor cortex. J Neurosci. (2020) 40:3385–407. doi: 10.1523/JNEUROSCI.2226-19.2020
321. Bunnell BA, Flaat M, Gagliardi C, Patel B, Ripoll C. Adipose-derived stem cells: isolation, expansion and differentiation. Methods. (2008) 45:115–20. doi: 10.1016/j.ymeth.2008.03.006
322. Strioga M, Viswanathan S, Darinskas A, Slaby O, Michalek J. Same or not the same? Comparison of adipose tissue-derived versus bone marrow-derived mesenchymal stem and stromal cells. Stem Cells Dev. (2012) 21:2724–52. doi: 10.1089/scd.2011.0722
323. Beard K, Meaney DF, Issadore D. Clinical applications of extracellular vesicles in the diagnosis and treatment of traumatic brain injury. J Neurotrauma. (2020) 37:2045–56. doi: 10.1089/neu.2020.6990
324. Mondello S, Thelin EP, Shaw G, Salzet M, Visalli C, Cizkova D, et al. Extracellular vesicles: pathogenetic, diagnostic and therapeutic value in traumatic brain injury. Expert Rev Proteomics. (2018) 15:451–61. doi: 10.1080/14789450.2018.1464914
325. Kodali M, Madhu LN, Reger RL, Milutinovic B, Upadhya R, Gonzalez JJ, et al. Intranasally administered human MSC-derived extracellular vesicles inhibit NLRP3-p38/MAPK signaling after TBI and prevent chronic brain dysfunction. Brain Behav Immun. (2023) 108:118–34. doi: 10.1016/j.bbi.2022.11.014
326. Kim D-k, Nishida H, An SY, Shetty AK, Bartosh TJ, Prockop DJ. Chromatographically isolated CD63+ CD81+ extracellular vesicles from mesenchymal stromal cells rescue cognitive impairments after TBI. Proc Natl Acad Sci USA. (2016) 113:170–5. doi: 10.1073/pnas.1522297113
327. Zhang Y, Chopp M, Zhang ZG, Katakowski M, Xin H, Qu C, et al. Systemic administration of cell-free exosomes generated by human bone marrow derived mesenchymal stem cells cultured under 2D and 3D conditions improves functional recovery in rats after traumatic brain injury. Neurochem Int. (2017) 111:69–81. doi: 10.1016/j.neuint.2016.08.003
328. Zhang Y, Zhang Y, Chopp M, Pang H, Zhang ZG, Mahmood A, et al. MiR-17–92 cluster-enriched exosomes derived from human bone marrow mesenchymal stromal cells improve tissue and functional recovery in rats after traumatic brain injury. J Neurotrauma. (2021) 38:1535–50. doi: 10.1089/neu.2020.7575
329. Zhang Y, Zhang Y, Chopp M, Pang H, Chen L, Zhang ZG, et al. Therapeutic role of microRNAs of small extracellular vesicles from human mesenchymal stromal/stem cells in treatment of experimental traumatic brain injury. J Neurotrauma. (2023) 40:758–71. doi: 10.1089/neu.2022.0296
330. Zhuang Z, Liu M, Luo J, Zhang X, Dai Z, Zhang B, et al. Exosomes derived from bone marrow mesenchymal stem cells attenuate neurological damage in traumatic brain injury by alleviating glutamate-mediated excitotoxicity. Exp Neurol. (2022) 357:114182. doi: 10.1016/j.expneurol.2022.114182
331. Chen Y, Li J, Ma B, Li N, Wang S, Sun Z, et al. MSC-derived exosomes promote recovery from traumatic brain injury via microglia/macrophages in rat. Aging (Albany NY). (2020) 12:18274. doi: 10.18632/aging.103692
332. Bambakidis T, Dekker SE, Williams AM, Biesterveld BE, Bhatti UF, Liu B, et al. Early treatment with a single dose of mesenchymal stem cell derived extracellular vesicles modulates the brain transcriptome to create neuroprotective changes in a porcine model of traumatic brain injury and hemorrhagic shock. Shock. (2022) 57:281–90. doi: 10.1097/SHK.0000000000001889
333. De Miguel MP, Fuentes-Julián S, Blázquez-Martínez A, Pascual CY, Aller MA, Arias J, et al. Immunosuppressive properties of mesenchymal stem cells: advances and applications. Curr Mol Med. (2012) 12:574–91. doi: 10.2174/156652412800619950
334. Ren G, Zhang L, Zhao X, Xu G, Zhang Y, Roberts AI, et al. Mesenchymal stem cell-mediated immunosuppression occurs via concerted action of chemokines and nitric oxide. Cell Stem Cell. (2008) 2:141–50. doi: 10.1016/j.stem.2007.11.014
335. Han Z, Jing Y, Zhang S, Liu Y, Shi Y, Wei L. The role of immunosuppression of mesenchymal stem cells in tissue repair and tumor growth. Cell Biosci. (2012) 2:8. doi: 10.1186/2045-3701-2-8
336. Qin A, Chen S, Wang P, Huang X, Zhang Y, Liang L, et al. Knockout of NOS2 promotes adipogenic differentiation of rat MSCs by enhancing activation of JAK/STAT3 signaling. Front Cell Dev Biol. (2021) 9:638518. doi: 10.3389/fcell.2021.638518
337. Plumas J, Chaperot L, Richard MJ, Molens JP, Bensa JC, Favrot MC. Mesenchymal stem cells induce apoptosis of activated T cells. Leukemia. (2005) 19:1597–604. doi: 10.1038/sj.leu.2403871
338. Krampera M, Cosmi L, Angeli R, Pasini A, Liotta F, Andreini A, et al. Role for interferon-γ in the immunomodulatory activity of human bone marrow mesenchymal stem cells. Stem Cells. (2006) 24:386–98. doi: 10.1634/stemcells.2005-0008
339. Li W, Ren G, Huang Y, Su J, Han Y, Li J, et al. Mesenchymal stem cells: a double-edged sword in regulating immune responses. Cell Death Differ. (2012) 19:1505–13. doi: 10.1038/cdd.2012.26
340. Lu L, Chen G, Yang J, Ma Z, Yang Y, Hu Y, et al. Bone marrow mesenchymal stem cells suppress growth and promote the apoptosis of glioma U251 cells through downregulation of the PI3K/AKT signaling pathway. Biomed Pharmacother. (2019) 112:108625. doi: 10.1016/j.biopha.2019.108625
341. Jotzu C, Alt E, Welte G, Li J, Hennessy BT, Devarajan E, et al. Adipose tissue derived stem cells differentiate into carcinoma-associated fibroblast-like cells under the influence of tumor derived factors. Cell Oncol. (2011) 34:55–67. doi: 10.1007/s13402-011-0012-1
342. Barcellos-de-Souza P, Comito G, Pons-Segura C, Taddei ML, Gori V, Becherucci V, et al. Mesenchymal stem cells are recruited and activated into carcinoma-associated fibroblasts by prostate cancer microenvironment-derived TGF-β1. Stem Cells. (2016) 34:2536–47. doi: 10.1002/stem.2412
343. Aoto K, Ito K, Aoki S. Complex formation between platelet-derived growth factor receptor β and transforming growth factor β receptor regulates the differentiation of mesenchymal stem cells into cancer-associated fibroblasts. Oncotarget. (2018) 9:34090. doi: 10.18632/oncotarget.26124
344. Mastrolia I, Foppiani EM, Murgia A, Candini O, Samarelli AV, Grisendi G, et al. Challenges in clinical development of mesenchymal stromal/stem cells: concise review. Stem Cells Transl Med. (2019) 8:1135–48. doi: 10.1002/sctm.19-0044
345. d'Aquino R, Graziano A, Sampaolesi M, Laino G, Pirozzi G, De Rosa A, et al. Human postnatal dental pulp cells co-differentiate into osteoblasts and endotheliocytes: a pivotal synergy leading to adult bone tissue formation. Cell Death Differ. (2007) 14:1162–71. doi: 10.1038/sj.cdd.4402121
346. Mead B, Logan A, Berry M, Leadbeater W, Scheven BA. Paracrine-mediated neuroprotection and neuritogenesis of axotomised retinal ganglion cells by human dental pulp stem cells: comparison with human bone marrow and adipose-derived mesenchymal stem cells. PLoS ONE. (2014) 9:e109305. doi: 10.1371/journal.pone.0109305
347. Yu S, Zhao Y, Ma Y, Ge L. Profiling the secretome of human stem cells from dental apical papilla. Stem Cells Dev. (2016) 25:499–508. doi: 10.1089/scd.2015.0298
348. Sammour I, Somashekar S, Huang J, Batlahally S, Breton M, Valasaki K, et al. The effect of gender on mesenchymal stem cell (MSC) efficacy in neonatal hyperoxia-induced lung injury. PLoS ONE. (2016) 11:e0164269. doi: 10.1371/journal.pone.0164269
349. Zhu M, Kohan E, Bradley J, Hedrick M, Benhaim P, Zuk P. The effect of age on osteogenic, adipogenic and proliferative potential of female adipose-derived stem cells. J Tissue Eng Regen Med. (2009) 3:290–301. doi: 10.1002/term.165
350. Ogawa R, Mizuno H, Hyakusoku H, Watanabe A, Migita M, Shimada T. Chondrogenic and osteogenic differentiation of adipose-derived stem cells isolated from GFP transgenic mice. J Nippon Med Sch. (2004) 71:240–1. doi: 10.1272/jnms.71.240
351. Alonso-Goulart V, Westendorf JJ, Yaszemski MJ, Khosla S. Mesenchymal stem cells from human adipose tissue and bone repair: a literature review. Mayo Clin Proc. (2018) 2:74–80. doi: 10.1016/j.biori.2017.10.005
352. Mahmood R, Shaukat M, Choudhery M. Biological properties of mesenchymal stem cells derived from adipose tissue, umbilical cord tissue and bone marrow. AIMS Cell Tissue Eng. (2018) 2:78–90. doi: 10.3934/celltissue.2018.2.78
353. Stolzing A, Jones E, McGonagle D, Scutt A. Age-related changes in human bone marrow-derived mesenchymal stem cells: consequences for cell therapies. Mech Ageing Dev. (2008) 129:163–73. doi: 10.1016/j.mad.2007.12.002
354. Rezabakhsh A, Cheraghi O, Nourazarian A, Hassanpour M, Kazemi M, Ghaderi S, et al. Type 2 diabetes inhibited human mesenchymal stem cells angiogenic response by over-activity of the autophagic pathway. J Cell Biochem. (2017) 118:1518–30. doi: 10.1002/jcb.25814
355. Ferland-McCollough D, Maselli D, Spinetti G, Sambataro M, Sullivan N, Blom A, et al. MCP-1 feedback loop between adipocytes and mesenchymal stromal cells causes fat accumulation and contributes to hematopoietic stem cell rarefaction in the bone marrow of patients with diabetes. Diabetes. (2018) 67:1380–94. doi: 10.2337/db18-0044
356. Geissler PJ, Davis K, Roostaeian J, Unger J, Huang J, Rohrich RJ. Improving fat transfer viability: the role of aging, body mass index, and harvest site. Plast Reconstr Surg. (2014) 134:227–32. doi: 10.1097/PRS.0000000000000398
357. Ulum B, Teker HT, Sarikaya A, Balta G, Kuskonmaz B, Uckan-Cetinkaya D, et al. Bone marrow mesenchymal stem cell donors with a high body mass index display elevated endoplasmic reticulum stress and are functionally impaired. J Cell Physiol. (2018) 233:8429–36. doi: 10.1002/jcp.26804
358. Mitterberger MC, Mattesich M, Zwerschke W. Bariatric surgery and diet-induced long-term caloric restriction protect subcutaneous adipose-derived stromal/progenitor cells and prolong their life span in formerly obese humans. Exp Gerontol. (2014) 56:106–13. doi: 10.1016/j.exger.2014.03.030
359. Tsuji W, Schnider JT, McLaughlin MM, Schweizer R, Zhang W, Solari MG, et al. Effects of immunosuppressive drugs on viability and susceptibility of adipose-and bone marrow-derived mesenchymal stem cells. Front Immunol. (2015) 6:135496. doi: 10.3389/fimmu.2015.00131
360. Pike S, Zhang P, Wei Z, Wu N, Klinger A, Chang S, et al. In vitro effects of tamoxifen on adipose-derived stem cells. Wound Repair Regen. (2015) 23:728–36. doi: 10.1111/wrr.12322
361. Poglio S, Galvani S, Bour S, André M, Prunet-Marcassus B, Pénicaud L, et al. Adipose tissue sensitivity to radiation exposure. Am J Pathol. (2009) 174:44–53. doi: 10.2353/ajpath.2009.080505
362. Holan V, Cechova K, Zajicova A, Kossl J, Hermankova B, Bohacova P, et al. The impact of morphine on the characteristics and function properties of human mesenchymal stem cells. Stem Cell Rev Rep. (2018) 14:801–11. doi: 10.1007/s12015-018-9843-8
363. Andrzejewska A, Lukomska B, Janowski M. Concise review: mesenchymal stem cells: from roots to boost. Stem Cells. (2019) 37:855–64. doi: 10.1002/stem.3016
364. Hass R, Kasper C, Böhm S, Jacobs R. Different populations and sources of human mesenchymal stem cells (MSC): a comparison of adult and neonatal tissue-derived MSC. Cell Commun Signal. (2011) 9:12. doi: 10.1186/1478-811X-9-12
365. Kern S, Eichler H, Stoeve J, Klüter H, Bieback K. Comparative analysis of mesenchymal stem cells from bone marrow, umbilical cord blood, or adipose tissue. Stem Cells. (2006) 24:1294–301. doi: 10.1634/stemcells.2005-0342
366. Heo JS, Choi Y, Kim H-S, Kim HO. Comparison of molecular profiles of human mesenchymal stem cells derived from bone marrow, umbilical cord blood, placenta and adipose tissue. Int J Mol Med. (2016) 37:115–25. doi: 10.3892/ijmm.2015.2413
367. Phinney DG, Kopen G, Righter W, Webster S, Tremain N, Prockop DJ. Donor variation in the growth properties and osteogenic potential of human marrow stromal cells. J Cell Biochem. (1999) 75:424–36. doi: 10.1002/(SICI)1097-4644(19991201)75:3<424::AID-JCB8>3.3.CO;2-#
368. Zhou S, Greenberger JS, Epperly MW, Goff JP, Adler C, Leboff MS, Glowacki J. Age-related intrinsic changes in human bone-marrow-derived mesenchymal stem cells and their differentiation to osteoblasts. Aging Cell. (2008) 7:335–43. doi: 10.1111/j.1474-9726.2008.00377.x
369. Colter DC, Class R, DiGirolamo CM, Prockop DJ. Rapid expansion of recycling stem cells in cultures of plastic-adherent cells from human bone marrow. Proc Natl Acad Sci USA. (2000) 97:3213–8. doi: 10.1073/pnas.97.7.3213
370. Walter SG, Randau TM, Hilgers C, Haddouti E-M, Masson W, Gravius S, et al. Molecular and functional phenotypes of human bone marrow-derived mesenchymal stromal cells depend on harvesting techniques. Int J Mol Sci. (2020) 21:4382. doi: 10.3390/ijms21124382
371. Karnoub AE, Dash AB, Vo AP, Sullivan A, Brooks MW, Bell GW, et al. Mesenchymal stem cells within tumour stroma promote breast cancer metastasis. Nature. (2007) 449:557–63. doi: 10.1038/nature06188
372. König A, Menzel T, Lynen S, Wrazel L, Rosén A, Al-Katib A, et al. Basic fibroblast growth factor (bFGF) upregulates the expression of bcl-2 in B cell chronic lymphocytic leukemia cell lines resulting in delaying apoptosis. Leukemia. (1997) 11:258–65. doi: 10.1038/sj.leu.2400556
373. Dias S, Shmelkov SV, Lam G, Rafii S. VEGF165 promotes survival of leukemic cells by Hsp90-mediated induction of Bcl-2 expression and apoptosis inhibition. Blood. (2002) 99:2532–40. doi: 10.1182/blood.V99.7.2532
374. Zhu Z, Gan X, Yu H. NF-κB-miR15a-bFGF/VEGFA axis contributes to the impaired angiogenic capacity of BM-MSCs in high fat diet-fed mice. Mol Med Rep. (2017) 16:7609–16. doi: 10.3892/mmr.2017.7498
375. Rivera-Cruz CM, Shearer JJ, Neto MF, Figueiredo ML. The immunomodulatory effects of mesenchymal stem cell polarization within the tumor microenvironment niche. Stem Cells Int. (2017) 2017:4015039. doi: 10.1155/2017/4015039
376. Deschepper M, Oudina K, David B, Myrtil V, Collet C, Bensidhoum M, et al. Survival and function of mesenchymal stem cells (MSCs) depend on glucose to overcome exposure to long-term, severe and continuous hypoxia. J Cell Mol Med. (2011) 15:1505–14. doi: 10.1111/j.1582-4934.2010.01138.x
377. Musiał-Wysocka A, Kot M, Majka M. The pros and cons of mesenchymal stem cell-based therapies. Cell Transplant. (2019) 28:801–12. doi: 10.1177/0963689719837897
378. Galipeau J, Sensébé L. Mesenchymal stromal cells: clinical challenges and therapeutic opportunities. Cell Stem Cell. (2018) 22:824–33. doi: 10.1016/j.stem.2018.05.004
379. Caplan H, Olson SD, Kumar A, George M, Prabhakara KS, Wenzel P, et al. Mesenchymal stromal cell therapeutic delivery: translational challenges to clinical application. Front Immunol. (2019) 10:1645. doi: 10.3389/fimmu.2019.01645
380. Jiang XX, Zhang Y, Liu B, Zhang SX, Wu Y, Yu XD, et al. Human mesenchymal stem cells inhibit differentiation and function of monocyte-derived dendritic cells. Blood. (2005) 105:4120–6. doi: 10.1182/blood-2004-02-0586
381. Sato K, Ozaki K, Oh I, Meguro A, Hatanaka K, Nagai T, et al. Nitric oxide plays a critical role in suppression of T-cell proliferation by mesenchymal stem cells. Blood. (2007) 109:228–34. doi: 10.1182/blood-2006-02-002246
382. Volarevic V, Al-Qahtani A, Arsenijevic N, Pajovic S, Lukic ML. Interleukin-1 receptor antagonist (IL-1Ra) and IL-1Ra producing mesenchymal stem cells as modulators of diabetogenesis. Autoimmunity. (2010) 43:255–63. doi: 10.3109/08916930903305641
383. Lee HJ, Ko JH, Jeong HJ, Ko AY, Kim MK, Wee WR, et al. Mesenchymal stem/stromal cells protect against autoimmunity via CCL2-dependent recruitment of myeloid-derived suppressor cells. J Immunol. (2015) 194:3634–45. doi: 10.4049/jimmunol.1402139
384. Birnbaum T, Roider J, Schankin CJ, Padovan CS, Schichor C, Goldbrunner R, et al. Malignant gliomas actively recruit bone marrow stromal cells by secreting angiogenic cytokines. J Neurooncol. (2007) 83:241–7. doi: 10.1007/s11060-007-9332-4
385. Zacharek A, Chen J, Cui X, Li A, Li Y, Roberts C, et al. Angiopoietin1/Tie2 and VEGF/Flk1 induced by MSC treatment amplifies angiogenesis and vascular stabilization after stroke. J Cereb Blood Flow Metab. (2007) 27:1684–91. doi: 10.1038/sj.jcbfm.9600475
386. Huang W, Chang MC, Tsai KS, Hung MC, Chen HL, Hung SC. Mesenchymal stem cells promote growth and angiogenesis of tumors in mice. Oncogene. (2013) 32:4343–54. doi: 10.1038/onc.2012.458
387. O'Malley G, Heijltjes M, Houston AM, Rani S, Ritter T, Egan LJ, et al. Mesenchymal stromal cells (MSCs) and colorectal cancer: a troublesome twosome for the anti-tumour immune response? Oncotarget. (2016) 7:60752. doi: 10.18632/oncotarget.11354
388. Spaeth EL, Dembinski JL, Sasser AK, Watson K, Klopp A, Hall B, et al. Mesenchymal stem cell transition to tumor-associated fibroblasts contributes to fibrovascular network expansion and tumor progression. PLoS ONE. (2009) 4:e4992. doi: 10.1371/journal.pone.0004992
389. Ishihara S, Inman DR, Li WJ, Ponik SM, Keely PJ. Mechano-signal transduction in mesenchymal stem cells induces prosaposin secretion to drive the proliferation of breast cancer cells. Cancer Res. (2017) 77:6179–89. doi: 10.1158/0008-5472.CAN-17-0569
390. Xu WT, Bian ZY, Fan QM, Li G, Tang TT. Human mesenchymal stem cells (hMSCs) target osteosarcoma and promote its growth and pulmonary metastasis. Cancer Lett. (2009) 281:32–41. doi: 10.1016/j.canlet.2009.02.022
391. Yan C, Chang J, Song X, Qi Y, Ji Z, Liu T, et al. Lung cancer-associated mesenchymal stem cells promote tumor metastasis and tumorigenesis by induction of epithelial–mesenchymal transition and stem-like reprogram. Aging (Albany NY). (2021) 13:9780. doi: 10.18632/aging.202732
392. Yin S, Zhou S, Ren D, Zhang J, Xin H, He X, et al. Mesenchymal stem cell-derived exosomes attenuate epithelial–mesenchymal transition of HK-2 cells. Tissue Eng Part A. (2022) 28:651–9. doi: 10.1089/ten.tea.2021.0190
393. Shi H, Sun Y, Ruan H, Ji C, Zhang J, Wu P, et al. 3,3′-diindolylmethane promotes gastric cancer progression via β-TrCP-mediated NF-κB activation in gastric cancer-derived MSCs. Front Oncol. (2021) 11:603533. doi: 10.3389/fonc.2021.603533
394. Liang W, Chen X, Zhang S, Fang J, Chen M, Xu Y, et al. Mesenchymal stem cells as a double-edged sword in tumor growth: focusing on MSC-derived cytokines. Cell Mol Biol Lett. (2021) 26:1–25. doi: 10.1186/s11658-020-00246-5
395. Kalluri R. The biology and function of fibroblasts in cancer. Nat Rev Cancer. (2016) 16:582–98. doi: 10.1038/nrc.2016.73
396. Loo SJQ, Wong NK. Advantages and challenges of stem cell therapy for osteoarthritis. Biomed Rep. (2021) 15:67. doi: 10.3892/br.2021.1443
397. Winickoff D. A bold experiment: Iceland's genomic venture, in Ethics, law and governance of biobanking: national. Eur Int Approach. (2015) 2015:187–209. doi: 10.1007/978-94-017-9573-9_13
398. Prictor M, Teare HJ, Kaye JJ. Equitable participation in biobanks: the risks and benefits of a “dynamic consent” approach. Front Public Health. (2018) 6:410631. doi: 10.3389/fpubh.2018.00253
399. Zhang J, Huang Z, Ong B, Sahu C, Zeng H, Ruan HB. Adipose-derived stromal cells in regulation of hematopoiesis. Cell Mol Biol Lett. (2020) 25:1–11. doi: 10.1186/s11658-020-00209-w
400. Wei X, Yang X, Han ZP, Qu FF, Shao L, Shi YF. Mesenchymal stem cells: a new trend for cell therapy. Acta Pharmacol Sinica. (2013) 34:747–54. doi: 10.1038/aps.2013.50
401. Salem HK, Thiemermann C. Mesenchymal stromal cells: current understanding and clinical status. Stem Cells. (2010) 28:585–96. doi: 10.1002/stem.269
402. Klopp AH, Gupta A, Spaeth E, Andreeff M, Marini F. Concise review: dissecting a discrepancy in the literature: do mesenchymal stem cells support or suppress tumor growth? Stem Cells. (2011) 29:11–9. doi: 10.1002/stem.559
403. Zhang Y, Daquinag A, Traktuev DO, Amaya-Manzanares F, Simmons PJ, March KL, et al. White adipose tissue cells are recruited by experimental tumors and promote cancer progression in mouse models. Cancer Res. (2009) 69:5259–66. doi: 10.1158/0008-5472.CAN-08-3444
404. Pinilla S, Alt E, Khalek FJA, Jotzu C, Muehlberg F, Beckmann C, et al. Tissue resident stem cells produce CCL5 under the influence of cancer cells and thereby promote breast cancer cell invasion. Cancer Lett. (2009) 284:80–5. doi: 10.1016/j.canlet.2009.04.013
405. Krohn A, Song Y-H, Muehlberg F, Droll L, Beckmann C, Alt E. CXCR4 receptor positive spheroid forming cells are responsible for tumor invasion in vitro. Cancer Lett. (2009) 280:65–71. doi: 10.1016/j.canlet.2009.02.005
406. Lamagna C, Aurrand-Lions M, Imhof BA. Dual role of macrophages in tumor growth and angiogenesis. J Leukocyte Biol. (2006) 80:705–13. doi: 10.1189/jlb.1105656
407. Li HJ, Reinhardt F, Herschman HR, Weinberg RA. Cancer-stimulated mesenchymal stem cells create a carcinoma stem cell niche via prostaglandin E2 signaling. Cancer Discov. (2012) 2:840–55. doi: 10.1158/2159-8290.CD-12-0101
408. Torisu H, Ono M, Kiryu H, Furue M, Ohmoto Y, Nakayama J, et al. Macrophage infiltration correlates with tumor stage and angiogenesis in human malignant melanoma: possible involvement of TNFα and IL-1α. Int J Cancer. (2000) 85:182–8. doi: 10.1002/(SICI)1097-0215(20000115)85:2<182::AID-IJC6>3.0.CO;2-M
Keywords: brain, traumatic brain injury (TBI), mesenchymal stem cells (MSCs), cell therapy, extracellular vesicles (EVs)
Citation: Yarahmadi A, Dorri Giv M, Hosseininejad R, Rezaie A, Mohammadi N, Afkhami H and Farokhi A (2025) Mesenchymal stem cells and their extracellular vesicle therapy for neurological disorders: traumatic brain injury and beyond. Front. Neurol. 16:1472679. doi: 10.3389/fneur.2025.1472679
Received: 29 July 2024; Accepted: 08 January 2025;
Published: 05 February 2025.
Edited by:
Daniel Daneshvar, Harvard Medical School, United StatesReviewed by:
Bharathi Hattiangady, Texas A&M University College of Medicine, United StatesCopyright © 2025 Yarahmadi, Dorri Giv, Hosseininejad, Rezaie, Mohammadi, Afkhami and Farokhi. This is an open-access article distributed under the terms of the Creative Commons Attribution License (CC BY). The use, distribution or reproduction in other forums is permitted, provided the original author(s) and the copyright owner(s) are credited and that the original publication in this journal is cited, in accordance with accepted academic practice. No use, distribution or reproduction is permitted which does not comply with these terms.
*Correspondence: Hamed Afkhami, aGFtZWRhZmtoYW1pNzBAZ21haWwuY29t; Arastoo Farokhi, YXJhc190dUB5YWhvby5jb20=
†These authors share first authorship
Disclaimer: All claims expressed in this article are solely those of the authors and do not necessarily represent those of their affiliated organizations, or those of the publisher, the editors and the reviewers. Any product that may be evaluated in this article or claim that may be made by its manufacturer is not guaranteed or endorsed by the publisher.
Research integrity at Frontiers
Learn more about the work of our research integrity team to safeguard the quality of each article we publish.