- 1Vision Science Group (Orthoptics), Faculty of Health, UTS, Sydney, NSW, Australia
- 2Ophthalmology Department, Prince of Wales Hospital, UNSW, Darlington, NSW, Australia
- 3Save Sight Institute, University of Sydney, Sydney, NSW, Australia
Detecting and monitoring elevated intracranial pressure (ICP) is crucial in managing various neurologic and neuro-ophthalmic conditions, where early detection is essential to prevent complications such as seizures and stroke. Although traditional methods such as lumbar puncture, intraparenchymal and intraventricular cannulation, and external ventricular drainage are effective, they are invasive and carry risks of infection and brain hemorrhage. This has prompted the development of non-invasive techniques. Given that direct, non-invasive access to the brain is limited, a significant portion of research has focused on utilizing the eyes, which uniquely provide direct access to their internal structure and offer a cost-effective tool for non-invasive ICP assessment. This review explores the existing non-invasive ocular techniques for assessing chronically elevated ICP. Additionally, to provide a comprehensive perspective on the current landscape, invasive techniques are also examined. The discussion extends to the limitations inherent to each technique and the prospective pathways for future advancements in the field.
1 Introduction
A range of neurological and neuro-ophthalmic conditions, including hydrocephalus, space-occupying lesions, and idiopathic intracranial hypertension (IIH), can lead to a chronic rise in the cerebrospinal fluid pressure (CSFp). IIH is characterized by the presence of pathological signs of chronic raised ICP in the absence of any identified dilated ventricles, cranial tumors, or lesions evident on a brain scan with a normal ICP composition (1–4). We use the term CSFp interchangeably with intracranial pressure (ICP) where the latter is in the context of neurological or neuro-ophthalmic disease. Elevated ICP is a critical concern in these conditions, as it can lead to significant morbidity and mortality (5, 6). Rapid detection and management of raised ICP are crucial in preventing further damage to the central nervous system and reducing adverse outcomes. The conventional methods for measuring ICP, such as lumbar puncture (LP) and external ventricular drainage (EVD), are invasive and require trained clinicians to perform (5). These procedures, while effective, are associated with potential complications such as infection, hemorrhage and equipment failure (5, 6).
Headache, nausea, pulsatile tinnitus, and transient vision obscuration have been reported by patients who have elevated ICP (7–9). However, a correlation between the severity of symptoms and the level of elevated ICP has not been found (7, 10). More than 90% of patients complain of throbbing or bursting headaches that can be worsened by sneezing and coughing (2, 7, 11, 12). Loss of consciousness can also occur, which can be due to the displacement of the midbrain and diencephalon (e.g., because of head trauma) or reduction in cerebral perfusion pressure (CPP), leading to decreased cerebral blood flow (13, 14). Other possible clinical signs reported include pupillary dilation, impaired upgaze, and bilateral droopy eyelid (7). Blood pressure and respiratory changes are also often noticed in the late stages of raised ICP, often caused by brain stem distortion and ischemia (7).
In response to the clinical need for safer and more accessible monitoring techniques, non-invasive methods such as transcranial Doppler (TCD), Computed tomography (CT) and Magnetic Resonance imaging (MRI), and non-invasive ophthalmic methods such as the ultrasound assessment of optic nerve sheath diameter (ONSD) have been explored. The TCD methods assesses cerebral blood flow dynamics, which can be altered in the context of raised ICP, while ONSD method is based on the premise that the optic nerve sheath expands in response to elevated ICP. However, studies investigating these non-invasive methods have reported varying degrees of accuracy, suggesting that they may not yet be suitable to replace conventional invasive techniques entirely. Instead, they are often used as complementary tools or to assist in deciding whether to proceed with invasive monitoring (5, 6, 15–18).
2 Invasive techniques
Clinical guidelines recommend immediate ICP measurement in patients suspected of elevated ICP (19). However, there is debate concerning the type of pressure being monitored and the optimal location for measuring CSF pressure (20). Currently, raised ICP can be detected invasively at various anatomical locations, including intraventricular, intraparenchymal, epidural, subdural, and subarachnoid sites. LPs are the most commonly used invasive technique to measure and detect raised ICP in acute and chronic conditions (21, 22).
2.1 Lumbar puncture
LP is a widely used invasive method for measuring and monitoring chronic and acute raised ICP, such as hydrocephalus and IIH (23). It provides opening and closing CSFp values. Despite its broad use, LP can be a painful procedure and carries risks of infection and brain herniation, however the risks can be reduced by improving skills and techniques (24, 25). Headache is the most common complication reported post lumbar puncture, with up to 32% of the patients reporting some degree of headache (26). Despite the risks associated, LP remains the gold standard technique in measuring and detecting elevated CSFp in acute and chronic raised ICP (27, 28).
2.2 Transducer implantation
Various invasive transducer devices have been developed to detect and monitor acute and chronic elevated ICP. These devices involve implanting a catheter connected to a transducer into the brain cavity, with the procedure named after the anatomical location of the insertion site (29). While epidural, subdural, intraparenchymal, and intraventricular methods were previously used, currently only intraventricular, and parenchymal sites are commonly performed (30). The intraparenchymal method measures brain tissue pressure as the catheter is inserted directly into the brain tissue (31, 32). The intraventricular technique was first performed in 1866 by Hollingsworth et al. (30). A catheter is inserted into the ventricles, close to the Foramen of Monroe, through a hole that is drilled into the skull, and connected to an external transducer (20, 29, 30). An External Ventricular Drain (EVD) originating from the intraventricular method is considered the gold standard for measuring global ICP (20, 23, 29, 33–35). An EVD also facilitates CSF drainage to maintain normal ICP levels and allows for the administration of drugs, such as antibiotics (20, 29, 33). The surgical procedure to place an EVD is considered minor and low risk (29). EVD placement can be challenging in younger patients due to their smaller ventricular system, while in older adults, the procedure is relatively straightforward due to age-related ventricular widening (29). A review and meta-analysis reported a 5.7% post-operative risk of hemorrhage in 1790 EVD procedures (36). Infection and colonization of bacteria in the catheter are other complications, potentially leading to skin infection, ventriculitis, meningitis, and septicemia (37–39). Nosocomial infections are the most common complication of EVD, with rates ranging from 5% to over 20% (37). Factors such as catheter misplacement, defective catheters, or skin infection at the insertion site can increase the risk of EVD-related infection (29). A study of 138 patients undergoing EVD found that 12.3% of the catheters were misplaced, necessitating secondary operations (40). EVD is mainly used in acutely raised ICP, such as traumatic brain injury and neurologic intensive care (41, 42).
3 Non-invasive assessment of ICP
Due to the complications associated with invasive techniques for measuring ICP, various non-invasive methods have been developed and trialed; however, none have made it into mainstream clinical practice. Techniques such as Computed Tomography (CT), Magnetic Resonance Imaging (MRI), Transcranial Doppler Ultrasonography (TCD), and Tympanic Membrane Displacement (TMD) have been used to screen for signs of elevated ICP and assess the need for invasive monitoring in both chronic and acute raised ICP.
3.1 Neuro-imaging
3.1.1 Computed tomography
CT is a non-invasive modality that can be used to detect elevated ICP by evaluating the integrity of intracranial structures. It provides insights into the morphology of midline shifts, basal cisterns, and ventricles. CT scans are often used in combination with other clinical symptoms to evaluate elevated ICP (43, 44). In a study involving 218 patients with severe head injuries, 74% of patients without effacement of basal cisterns had an ICP of more than 30 mmHg (44). Midline shift and compression or destruction of mesencephalic cisterns as observed on CT scans are the most accurate signs of raised ICP (43, 44). In a review of non-invasive methods of measuring ICP, it was concluded that abnormal cisterns are associated with a 3-fold increased risk of elevated ICP (45). CT scans are valuable non-invasive tools used in chronic and acute raised ICP, but a normal CT scan does not necessarily indicate normal ICP. The major reason for performing a CT scan of the brain in IIH patients is to exclude any other underlying cause leading to raised ICP (46, 47).
3.1.2 Magnetic resonance imaging
MRI scans are used to characterize cerebral vascular circulation for non-invasive ICP assessments (48). The exponential relationship between volume and pressure was introduced by Marmarou et al. (49), demonstrating that compliance and resistance to fluid absorption are key in determining ICP levels. Intracranial elastance, which has a linear relationship with ICP, can be measured using MRI (50). Elastance is defined as the change in volume over the change in pressure (Elastance = Δν/∆p). This volume change is obtained using phase-contrast MRI measuring intracranial vein, arterial, and CSF flows during each cardiac cycle, while the pressure change is derived from CSF velocity extracted from velocity-encoded MRI images (43, 48). Studies have examined MRI’s reliability and accuracy in measuring elevated ICP, with findings ranging from modest to poor repeatability to significant correlations with invasive ICP measurements (48, 51) (Table 1).
3.2 Transcranial Doppler ultrasonography
TCD utilizes the interaction between CSF and cerebral blood flow to assess ICP non-invasively. It assesses the blood flow velocity of cerebral arteries and applies mathematical models for ICP estimation, which includes the TCD-derived pulsatility index reflecting downstream vessel resistance, calculation of cerebral perfusion pressure (CPP), and other mathematical estimations. However, the accuracy of these TCD-based methods varies significantly, as noted in a systematic review (15). TCD provides critical information on the direction and velocity of blood flow within intracranial vessels, which can change due to external pressures like ICP or internal pressures such as arterial blood pressure (15).
Despite its advantages of being cost-effective, portable, and risk-free with high temporal resolution, making it ideal for emergency departments and bedside use, TCD has limitations. It cannot detect abnormal ICP if the cause is an obstruction in CSF circulation rather than a dysfunction in the cerebral arterial system (15). Furthermore, its utility in monitoring raised ICP in patients with idiopathic intracranial hypertension (IIH) has shown conflicted results. Some studies suggest that while TCD, particularly the pulsatility index, correlates with raised ICP, it cannot replace invasive methods and is more suitable for monitoring chronically raised ICP during follow-up sessions (52–54). These complexities highlight the need for cautious application and further research into TCD’s effectiveness in various clinical scenarios.
3.3 Tympanic membrane displacement
TMD, developed in 1981, is an audiological measurement device for detecting raised ICP. It measures the movement of the tympanic membrane in response to the acoustic muscle reflex (55). The stapedius muscle contracts in response to sound stimulation, leading to the movement of the stapes. The cochlear fluid pressure level determines the resting position and direction of stapes movement due to its anatomical position on the oval window. High cochlear fluid pressure results in the inward displacement of the tympanic membrane, while low pressure leads to outward movement. The CSF pressure is transmitted to cochlear fluid pressure through the cochlear aqueduct (Figure 1). Therefore, monitoring the direction of tympanic membrane displacement can indicate normal or raised ICP (39, 56) and it can be an alternative non-invasive method of monitoring raised ICP in Hydrocephalus and neurological conditions (57, 58). To our knowledge, no study in the literature has investigated this method to detect raised ICP in IIH patients.
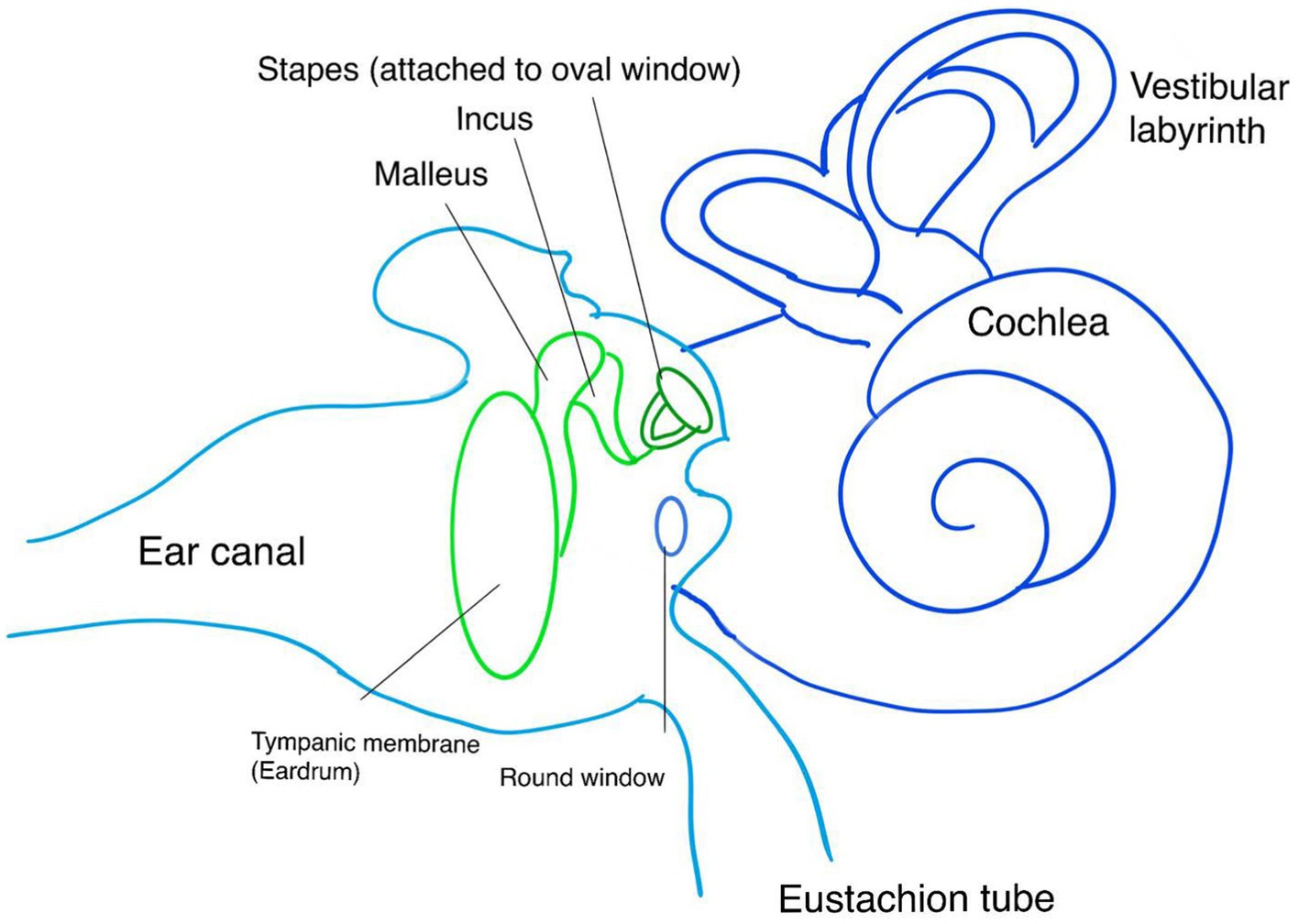
Figure 1. Anatomy of the ear and tympanic membrane involved in the measurement of TMD. CA, Cochlear Aqueduct; OW, Oval Window; RW, Round Window; T, Tympanic membrane; S, Sensor.
3.4 Otoacoustic emissions
Another novel non-invasive method of detecting elevated ICP is otoacoustic emissions, which is another auditory measurement of acutely elevated ICP. Otoacoustic emissions are produced by cochlea as a response to elevated ICP (59). Distortion product otoacoustic emissions (DPOAE) are subtypes of otoacoustic emissions that are produced in response to two specific frequencies in the ear canal (59). This technique uses microphones placed in the ear canal. Previous studies have shown that as the ICP changes, the magnitude and angles of otoacoustic emissions change; however, the shortcoming of this technique is that small changes in ICP do not produce any systematic changes in DPOAE (59, 60).
3.5 Visual evoked potential
Visual stimuli cause the production of electrical waves in the occipital lobes, which measure the conduction time from the retina to the visual cortex clinically used to examine the integrity of the visual pathway (61). VEP waveforms consist of positive (P) and negative (N) waves, the shape of these waves, the time taken to respond to these stimuli, or the latency (62). In a clinical setting, VEP is obtained by placing electrodes on the patient’s head to measure the optic pathway activity from the retinal pigment epithelium to the occipital lobe (61, 62). This method uses flashes of lights or pattern stimuli; however, flashing VEP (F-VEP) has shown more variability (61). F-VEP lasts for 5 milliseconds, covering a visual field of at least 20 degrees with a recommended flash rate of 1.0 Hz ±0.1 (1 flash per second), and pattern stimuli is a checkboard pattern that reverses every half second (Figure 2A) (63). Previous studies have demonstrated a positive correlation between F-VEP and elevated ICP, mainly causing prolonged latency of N2 waves in patients with traumatic brain injuries, hydrocephalus indicating shunt dysfunction and drug-related cerebral oedema (61, 64–69). Although limited studies are available on the utility of this method in detecting raised ICP in IIH patients, available studies have presented conflicting results depending on the technique used and test time (70). During the early stages of IIH, VEP has been reported to be normal (70–73). However, other studies have shown significant delayed response to stimuli in VEP (74, 75). In conclusion, VEP is useful in monitoring the severity or the regression of the visual function of patients with raised intracranial pressure (76).
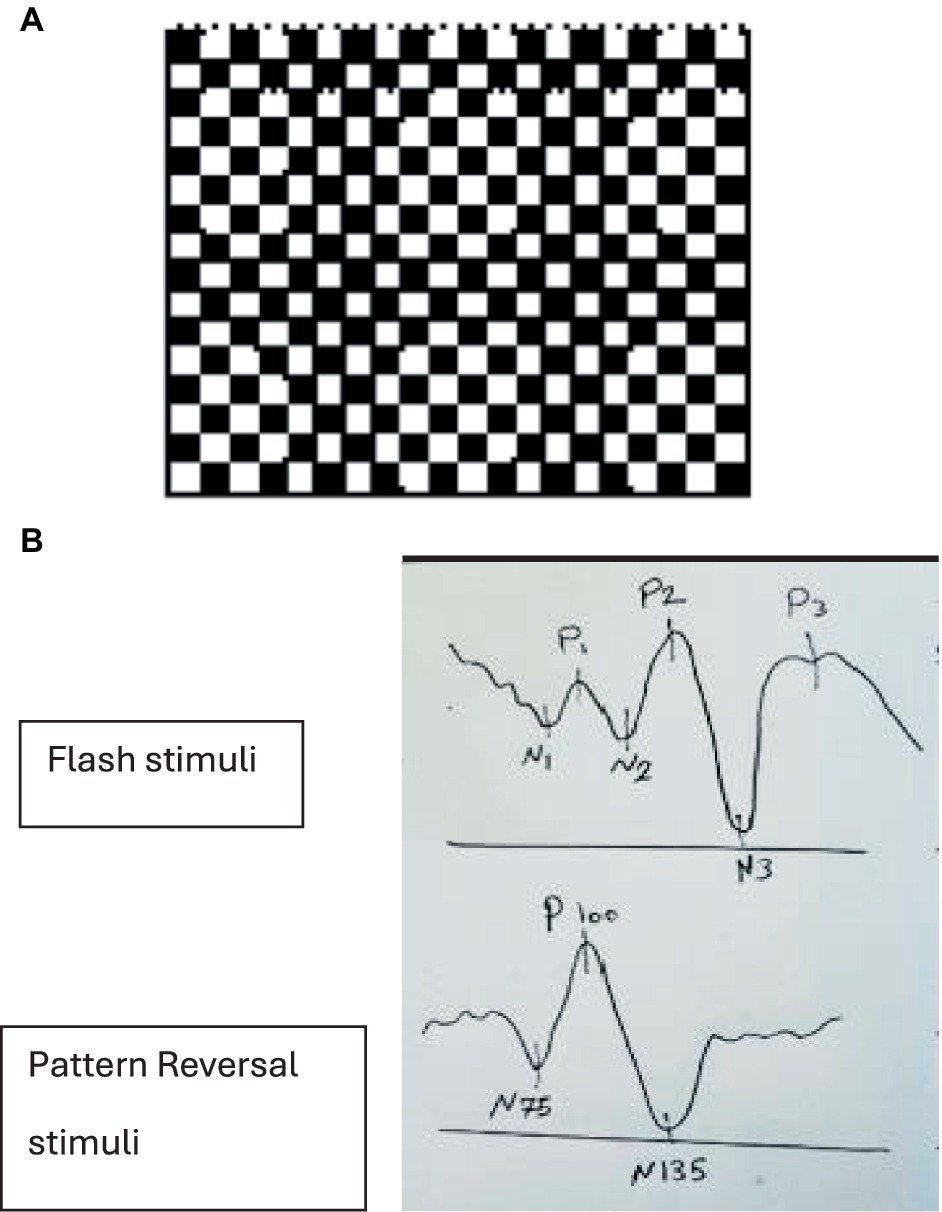
Figure 2. (A) Checkboard pattern with a red point fixation target in the middle. (B) Positive (P) and negative (N) responses to flash vs. pattern reversal stimuli.
Positive (P) and negative (N) responses with different stimuli. In flash stimuli, the second negative peak (N2), with a normal latency of approximately 70–90 ms and the second positive peak (P2), with a normal latency of approximately 100–120 ms, are the most prominent waves. Pattern-reversal VEPs normally comprised of three main features: an initial negativity with a latency of approximately 70 to 80 milliseconds (N75), a larger positive part with a latency of about 90–110 ms (P100), and a large negative part with a latency of around 130–140 ms (N135) (63, 65, 77) (Figure 2B).
4 Non-invasive ophthalmic-based assessment
Given the limitations and challenges in directly accessing the brain’s structure, as demonstrated above, alternative ophthalmic-based techniques have been explored due to the anatomical link between the eye and the brain’s structure, vasculature, and CSF drainage pathway. These non-invasive ocular assessments aim to eliminate the need for invasive approaches as the first line of assessment in chronic raised ICP such as IIH. The most commonly studied methods are measuring the optic nerve sheath diameter (ONSD), investigating structural changes at the optic nerve head (papilledema), and examining static and dynamic retinal vessel changes.
4.1 Optic nerve sheath diameter
The optic nerve, the second cranial nerve, is responsible for transmitting visual information. It exits the globe at the optic disk, where the lamina cribrosa resides. The optic nerve is surrounded by the optic nerve sheath (ONS), an extension of the brain’s meninges, with CSF running between the optic nerve and the ONS. Early studies have reported that a rise in ICP levels leads to an increase in ONSD, swelling of the optic nerve head, and deformation of the retinal pigment epithelium and Bruch’s membrane in papilledema (78–81). Chronic elevated ICP leads to increased ONSD and increased retinal vein diameter (82).
Various studies have investigated the correlation between raised ICP and increased ONSD (83–90). Ultrasound is the most investigated non-invasive technique for detecting raised ICP by measuring ONSD, depicted in Figure 1 (91). A study measuring ONSD by ultrasound in 50 participants concluded that an increased diameter of greater than 5.5 mm has 100% specificity and sensitivity in detecting raised ICP of more than 20 cmH2O (86, 91). Previous studies have demonstrated high sensitivity of ONSD in detecting abnormal ICP (83, 85, 87–95). However, there are inconsistent reports in the literature concerning the ONSD cut-off value and the efficacy of tools used to measure ONSD distension in elevated ICP. Cut-off values ranging from 5.3 mm to 5.8 mm have been reported as indicators of raised ICP. Notably, there is considerable inter-individual variation in ONSD measurements. Studies among the Chinese population reported an ONSD threshold of 4.1 mm as a marker of elevated ICP (90), while studies in Western populations reported cut-off values over 5 mm (88–90). In Bangladeshi patients aged over 16, the upper limit of ONSD in healthy individuals is 4.75 mm (96). These variations in cut-off values across different populations lead to increased sensitivity and decreased specificity in estimating raised ICP. Some studies have reported differing findings (85, 90, 95, 96). For example, a study of 51 patients undergoing lumbar puncture showed that ONSD obtained by ultrasound is not an appropriate marker for measuring raised ICP in non-traumatic intracranial hypertension due to its low specificity and sensitivity of 44 and 75%, respectively (85). Conversely, a study examining ICP in patients with severe traumatic brain injury and measuring their ONSD with a CT scan simultaneously reported that assessing ONSD with a portable CT is a reliable method, with a specificity of 42% and sensitivity of 97% (95). Table 2 summarizes the differences in specificity, sensitivity, and cut-off values reported in meta-analyses conducted over the last 10 years (92, 97–103). The variation in findings could be attributed to differences in population ethnicity, sample size, and the modalities used to measure ONSD. Ultrasound of ONSD is highly user-dependent, and measurements can vary significantly depending on the examiner’s skill (Figure 3).
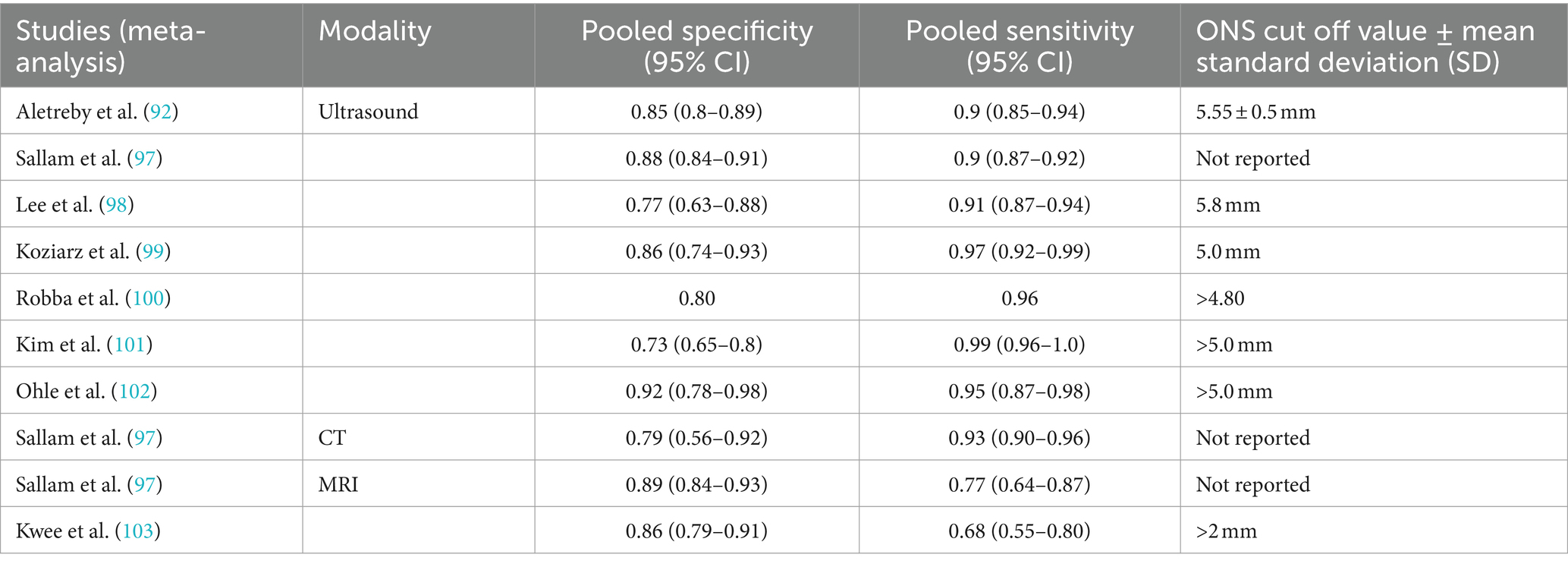
Table 2. Specificity, sensitivity, and cut-off value of ONSD in raised ICP, measured non-invasively.
4.2 OCT of the optic nerve head
Optical coherence tomography (OCT) is a widely used non-invasive technique for diagnosing papilledema, mainly in chronically raised ICP such as IIH. OCT provides cross-sectional images and quantifies retinal nerve fiber thickness (RNFL) and macular ganglion cell layer (GCL), which are key indicators for monitoring papilledema (104). There is thickening of the RNFL in papilledema. The macular GCL thickness is typically normal in patients with preserved visual function. However, in chronic, severe or untreated papilledema, irreversible damage to the retinal ganglion cells is seen as thinning of the macular GCL (105, 106). OCT of optic nerve head (Figure 4) has been instrumental in assessing peripapillary, choroidal, and retinal folds in IIH patients with papilledema, a phenomenon suggested to result from shear stress on the optic nerve head due to raised ICP (107). However, papilledema may not be an optimal marker for diagnosing and monitoring abnormal ICP, as its development and resolution can be delayed, depending on changes in ICP and axoplasmic disturbance. Additionally, optic atrophy may prevent nerve swelling in cases of raised ICP (108). Monitoring the severity of papilledema is suitable for monitoring raised ICP in chronic conditions that may guide treatment (84, 109). Previous studies denoted in Table 3 show the various findings of OCT of the optic nerve head in IIH patients with papilledema (104, 110–113). The increase in RNFL thickness and the decrease in macular GCL are correlated with the severity of papilledema and used to diagnose and monitor the progression and regression of raised ICP, mainly in chronic raised ICP (104, 105, 110, 114). Thinning of macular GCL results in visual dysfunction from neural loss.
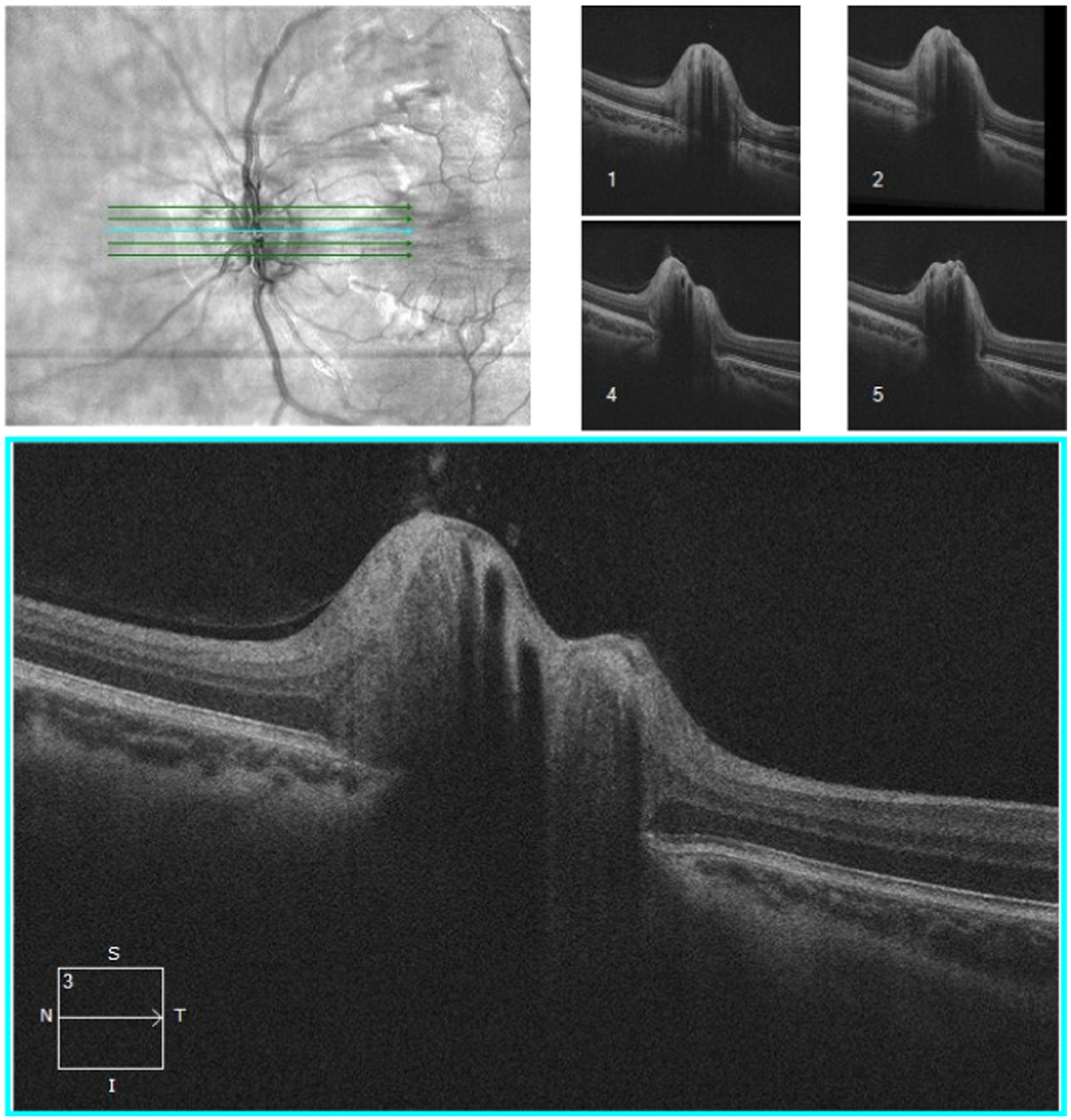
Figure 4. OCT of optic nerve head of an IIH patient conducted by CIRRUS SW Ver: 11.5.2.54532 Copyright 2020 Carl Zeiss Meditec.
4.3 Retinal vessel analysis
4.3.1 Static vessel analysis
Static changes in the retinal vasculature include tortuosity, arteriovenous nicking, and variations in vessel caliber. As ICP exceeds 20 cmH2O, retinal vein diameter increases, resulting in a decreased arteriole-to-venule diameter ratio (115). A study of 20 adults with papilledema observed a reduction in retinal vein and arterial diameter one-hour post-ICP lowering (82). Retinal vein diameter has been proposed as a biomarker for detecting increased retinal vein pressure due to raised ICP (116). In a randomized controlled trial involving 115 individuals with IIH, optic nerve swelling was correlated with increased retinal vein diameter, which decreased 6 months post-treatment for raised ICP (117). Retinal vessel tortuosity, associated with chronic raised ICP, is believed to result from increased vessel diameter and subsequent tortuosity (117, 118) however, no study has systematically investigated the improvement of vessel tortuosity due to the resolution of papilledema. Investigating static vessel changes is mainly used as additional
Markers for diagnosis and monitoring chronic raised ICP.
4.3.2 Dynamic vessel analysis
Dynamic retinal vascular changes are characterized by spatial–temporal features extracted from retinal videos, including pulse wave velocity, arterial pulsatility index, and spontaneous venous pulsations (SVPs) (119–123). SVPs, observed as slight variations in retinal vein diameter at the optic nerve head, occur as a result of intra- and extra-ocular pressure compartments intersecting at the lamina cribrosa, affecting the pressure gradient (120, 122). Elevated ICP leads to increased intracranial pulse pressure and an imbalanced pressure gradient between IOP and ICP, resulting in the cessation of SVPs (122, 124). SVPs have been postulated as markers of raised ICP due to their physiological relationship with ICP and IOP (118–124). A study of 338 measurements from 9 patients showed that ICP pulse pressure controls the timing of venous pulsation (121). While earlier studies reported increased pulsation of the central retinal vein due to increased IOP (125), recent modeling studies have indicated a close relationship between SVP, IOP, and ICP, with any imbalance disrupting SVP oscillation (118, 120, 121).
4.3.2.1 Assessment of SVP
The validity and usefulness of SVPs as a predictive marker of raised ICP has been debated (122–124, 126). The presence of SVPs indicates normal ICP, but their absence does not necessarily signify raised ICP, as they can be absent in 10-20% of the population (122). A prospective study evaluating SVP and ICP in 106 patients undergoing LP concluded that SVP was not a reliable predictor of ICP, with a sensitivity of 94% to exclude raised ICP (126). However, this study assessed ICP and SVP in different positions, which could affect the results. Another study investigated the validity of smartphone-based video ophthalmoscopy for detecting SVP in 233 patients, finding a sensitivity of 82.77 and 76.82% for two observers, respectively (127) demonstrated in Table 4. However, this study faced challenges like poor video quality and camera shake. Subjective measurement of SVPs can be one of the limitations presented in previous studies however objective measurements have shown 100% detection in healthy individuals (128).

Table 4. Responses given by two blind observers were asked to identify SVP on Videos, compared with gold standard identification using a slit lamp (127).
A recent study using motion-stabilized infrared videography found a significant association between SVP and ICP in 101 patients with intracranial hypertension (124). This study measured ICP and SVP in a seated position to account for postural variations in ICP (126). While further investigation is needed, there is potential for using SVP to non-invasively and reliably assess ICP (126) (Figure 5).
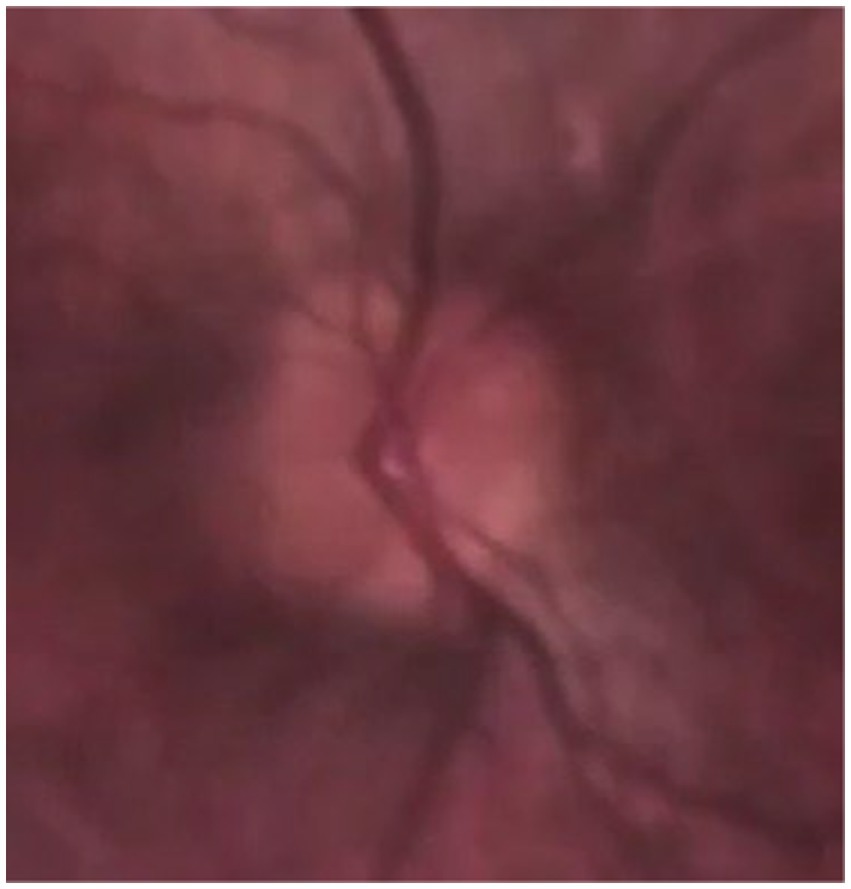
Figure 5. A fundus photo of retinal blood vessels as they exit the globe where SVP is detected with a smartphone-based handheld ophthalmoscope.
5 Limitations of non-invasive methods
Despite technological advances and the development of various non-invasive techniques, none have yet replaced invasive methods. This is due to limitations such as lack of portability, practicality, and availability in all clinical settings. Additionally, non-invasive methods often cannot monitor ICP continuously or measure it quantitatively, and they tend to be operator-dependent. Currently, non-invasive methods are primarily used as complementary tools in detecting raised ICP.
5.1 Neuro-imaging
MRI and CT scans provide qualitative information about structural brain changes, such as midline shift, lateral ventricular compression, and dilation of the ventricular system. These neuro-imaging methods are essential for differentiating underlying conditions from secondary causes of raised ICP. However, they are not universally available. MRI and CT scans cannot measure ICP quantitatively and are not reliable for continuous ICP monitoring. MRI is time-consuming, limiting its use in emergency settings where rapid detection of raised ICP is crucial. CT scans, which use ionizing radiation, are particularly concerning in pediatric patients. Collectively, neuro-imaging techniques are primarily used as screening tools where available.
5.2 Tympanic membrane displacement and otoacoustic emissions
The TMD technique requires a normal middle ear, an intact acoustic stapedial reflex, and a patent cochlear aqueduct. It is less optimal in elderly patients, as cochlear aqueduct patency often decreases with age. TMD cannot measure absolute ICP but can provide mean ICP values; however, it is not suitable for continuous ICP monitoring. Despite these limitations, TMD has proven reliable for screening patients suspected of having raised ICP. Otoacoustic emissions do not show any changes if the elevation of ICP is minor, which makes this method unreliable in acute smaller changes of ICP.
5.3 VEP
This non-invasive method is useful in detecting abnormalities in visual pathway dysfunction based on raised ICP. VEP cannot measure absolute ICP as it only detects damage to the optic nerve. If the patient is at an early stage of the disease and optic nerve damage has not happened yet, then the diagnosis can be missed or remain undetected. Another point to consider is that VEP is affected by anesthesia and cannulation and cannot be used in patients who are under anesthesia. However, it can be used as a non-invasive way of monitoring the progression of raised ICP in chronic intracranial hypertension patients.
5.4 Ophthalmic-based assessment
Ocular-based technologies and methods for non-invasive assessment of ICP have made significant advancements but have not yet achieved the clinical reliability needed to replace invasive methods. Limitations include structural variations in the eye, such as ONSD, across different ethnicities. Additionally, ocular changes may not be clinically sensitive to detect subtle, temporary ICP changes. An increase in ICP must be sustained and significant to affect retinal structures, including papilledema and blood vessel changes like the cessation of SVPs. Furthermore, none of the non-invasive ophthalmic methods developed so far can measure mean ICP; they assess abnormalities in retinal and optic nerve head structure and vasculature as surrogate biomarkers. Quantitative measurement of retinal and cranial pulse pressure also remains challenging.
6 Future directions
Developing an accurate, non-invasive method for monitoring elevated ICP could significantly impact patient quality of life. Traditional invasive methods, such as lumbar puncture, external ventricular drainage, and intraparenchymal probes, carry risks of complications like infection and brain herniation. The non-invasive techniques developed to date have their shortcomings and do not provide precise measurements of raised ICP. A holistic understanding of the link between ICP and structural and vascular changes is necessary to better comprehend the pathophysiology of raised ICP and its effects on retinal structures and blood vessels. We propose that elevated ICP could be monitored using a combination of non-invasive parameters, such as SVP, OCT of RNFL, and fundus photos, to establish a panel of biomarkers. In taking such approach a versatile panel of biomarkers can be tuned for detecting both acute and chronic elevations in ICP. For chronic conditions, more structural changes are expected, making markers such as RNFL assessments or static vascular features particularly relevant. In contrast, acute conditions, due to their dynamic nature, may show more prominent changes in dynamic vascular features (e.g., SVPs), which could more accurately reflect acute elevations in ICP. This does not imply that certain manifestations are exclusive to one condition over the other but suggests that the likelihood of observing a change may be more pronounced in one type of feature compared to another. Collectively, this approach could enhance the specificity and sensitivity of using ophthalmic-based markers to assess raised ICP non-invasively. While this research might lead to superior non-invasive ICP assessment methods through ocular examination, it is unlikely to replace gold-standard invasive techniques but rather act as a complementary approach where access to invasive techniques is limited or not warranted. It could also be valuable for early screening and triaging, thereby improving clinical management.
Author contributions
SB: Conceptualization, Data curation, Formal analysis, Funding acquisition, Investigation, Methodology, Project administration, Resources, Software, Supervision, Validation, Visualization, Writing – original draft, Writing – review & editing. AA: Supervision, Validation, Visualization, Writing – review & editing, Conceptualization, Funding acquisition, Methodology, Project administration, Resources. ML: Supervision, Validation, Visualization, Writing – review & editing. CF: Supervision, Validation, Visualization, Writing – review & editing, Conceptualization, Data curation, Funding acquisition, Investigation, Methodology, Resources, Writing – original draft. MG: Conceptualization, Data curation, Formal analysis, Funding acquisition, Investigation, Methodology, Project administration, Resources, Software, Supervision, Validation, Visualization, Writing – original draft, Writing – review & editing.
Funding
The author(s) declare that financial support was received for the research, authorship, and/or publication of this article. This work was supported by UTS HDR Scholarship.
Conflict of interest
The authors declare that the research was conducted in the absence of any commercial or financial relationships that could be construed as a potential conflict of interest.
Publisher’s note
All claims expressed in this article are solely those of the authors and do not necessarily represent those of their affiliated organizations, or those of the publisher, the editors and the reviewers. Any product that may be evaluated in this article, or claim that may be made by its manufacturer, is not guaranteed or endorsed by the publisher.
Abbreviations
ICP, Intracranial pressure; IIH, Idiopathic intracranial hypertension; ONSD, Optic nerve sheath diameter; SVP, Spontaneous venous pulsation; CSF, Cerebrospinal fluid; LP, Lumbar puncture; ONS, Optic nerve sheath.
References
1. Raichle, ME, Grubb, RL, Phelps, ME, Gado, MH, and Caronna, JJ. Cerebral hemodynamics and metabolism in Pseudotumor cerebri. Ann Neurol. (1978) 4:104–11. doi: 10.1002/ana.410040203
2. Lueck, CJ, and McIlwaine, GG. Interventions for idiopathic intracranial hypertension In: CJ Lueck, editor. Cochrane database of systematic reviews. Chichester: John Wiley & Sons, Ltd (2005)
3. Kesler, A, and Gadoth, N. Epidemiology of idiopathic Intracranial hypertension in Israel. J Neuroophthalmol. (2001) 21:12–4. doi: 10.1097/00041327-200103000-00003
4. Mollan, SP, Aguiar, M, Evison, F, Frew, E, and Sinclair, AJ. The expanding burden of idiopathic intracranial hypertension. Eye. (2019) 33:478–85. doi: 10.1038/s41433-018-0238-5
5. Changa, AR, Czeisler, BM, and Lord, AS. Management of elevated intracranial pressure: a review. Curr Neurol Neurosci Rep. (2019) 19:99. doi: 10.1007/s11910-019-1010-3
6. Schizodimos, T, Soulountsi, V, Iasonidou, C, and Kapravelos, N. An overview of management of intracranial hypertension in the intensive care unit. J Anesth. (2020) 34:741–57. doi: 10.1007/s00540-020-02795-7
7. Dunn, LT. Raised intracranial pressure. J Neurol Neurosurg Psychiatry. (2002) 73:i23–7. doi: 10.1136/jnnp.73.suppl_1.i23
8. Qvarlander, S, Sundström, N, Malm, J, and Eklund, A. Postural effects on intracranial pressure: modeling and clinical evaluation. J Appl Physiol. (2013) 115:1474–80. doi: 10.1152/japplphysiol.00711.2013
9. Marmarou, A, Shulman, K, and Rosende, RM. A nonlinear analysis of the cerebrospinal fluid system and intracranial pressure dynamics. J Neurosurg. (1978) 48:332–44. doi: 10.3171/jns.1978.48.3.0332
10. Ko, MW, Chang, SC, Ridha, MA, Ney, JJ, Ali, TF, Friedman, DI, et al. Weight gain and recurrence in idiopathic intracranial hypertension: a case-control study. Neurology. (2011) 76:1564–7. doi: 10.1212/WNL.0b013e3182190f51
11. Radojicic, A, Vukovic-Cvetkovic, V, Pekmezovic, T, Trajkovic, G, Zidverc-Trajkovic, J, and Jensen, RH. Predictive role of presenting symptoms and clinical findings in idiopathic intracranial hypertension. J Neurol Sci. (2019) 399:89–93. doi: 10.1016/j.jns.2019.02.006
12. Pickard, JD, and Czosnyka, M. Management of raised intracranial pressure. J Neurol Neurosurg Psychiatry. (1993) 56:845–58. doi: 10.1136/jnnp.56.8.845
13. Balestreri, M, Czosnyka, M, Hutchinson, P, Steiner, LA, Hiler, M, Smielewski, P, et al. Impact of Intracranial pressure and cerebral perfusion pressure on severe disability and mortality after head injury. Neurocrit Care. (2006) 4:008–13. doi: 10.1385/NCC:4:1:008
14. Chen, H, Wang, J, Mao, S, Dong, W, and Yang, H. A new method of Intracranial pressure Monitoring by EEG power Spectrum analysis. Can J Neurol Sci. (2012) 39:483–7. doi: 10.1017/S0317167100013998
15. Cardim, D, Robba, C, Bohdanowicz, M, Donnelly, J, Cabella, B, Liu, X, et al. Non-invasive monitoring of intracranial pressure using transcranial Doppler ultrasonography: is it possible? Neurocrit Care. (2016) 25:473–91. doi: 10.1007/s12028-016-0258-6
16. Aaslid, R, Markwalder, TM, and Nornes, H. Noninvasive transcranial Doppler ultrasound recording of flow velocity in basal cerebral arteries. J Neurosurg. (1982) 57:769–74. doi: 10.3171/jns.1982.57.6.0769
17. Robba, C, Cardim, D, Sekhon, M, Budohoski, K, and Czosnyka, M. Transcranial Doppler: a stethoscope for the brain-neurocritical care use. J Neurosci Res. (2018) 96:720–30. doi: 10.1002/jnr.24148
18. Fernando, SM, Tran, A, Cheng, W, Rochwerg, B, Taljaard, M, Kyeremanteng, K, et al. Diagnosis of elevated intracranial pressure in critically ill adults: systematic review and meta-analysis. BMJ. (2019) 366:l4225. doi: 10.1136/bmj.l4225
19. Cook, AM, Morgan Jones, G, Hawryluk, GWJ, Mailloux, P, McLaughlin, D, Papangelou, A, et al. Guidelines for the acute treatment of cerebral edema in Neurocritical care patients. Neurocrit Care. (2020) 32:647–66. doi: 10.1007/s12028-020-00959-7
20. Smith, M. Monitoring Intracranial pressure in traumatic brain injury. Anesth Analg. (2008) 106:240–8. doi: 10.1213/01.ane.0000297296.52006.8e
21. Bothwell, SW, Janigro, D, and Patabendige, A. Cerebrospinal fluid dynamics and intracranial pressure elevation in neurological diseases. Fluids Barriers CNS. 16:9. doi: 10.1186/s12987-019-0129-6
22. Sakka, L, Coll, G, and Chazal, J. Anatomy and physiology of cerebrospinal fluid. Eur Ann Otorhinolaryngol Head Neck Dis. (2011) 128:309–16. doi: 10.1016/j.anorl.2011.03.002
23. Czosnyka, M. Monitoring and interpretation of intracranial pressure. J Neurol Neurosurg Psychiatry. (2004) 75:813–21. doi: 10.1136/jnnp.2003.033126
24. Van Crevel, H, Hijdra, A, and De Gans, J. Lumbar puncture and the risk of herniation: when should we first perform CT? J Neurol. (2002) 249:129–37. doi: 10.1007/PL00007855
26. Armon, C, and Evans, RW. Addendum to assessment: prevention of post–lumbar puncture headaches [RETIRED]. Neurology. (2005) 65:510–2. doi: 10.1212/01.wnl.0000173034.96211.1b
27. Wright, BLC, Lai, JTF, and Sinclair, AJ. Cerebrospinal fluid and lumbar puncture: a practical review. J Neurol. (2012) 259:1530–45. doi: 10.1007/s00415-012-6413-x
29. Raboel, PH, Bartek, J, Andresen, M, Bellander, BM, and Romner, B. Intracranial pressure Monitoring: invasive versus non-invasive methods—a review. Crit Care Res Pract. (2012) 2012:1–14. doi: 10.1155/2012/950393
30. Olson, DM, Ortega Peréz, S, Ramsay, J, Venkatasubba Rao, CP, Suarez, JI, McNett, M, et al. Differentiate the source and site of Intracranial pressure measurements using more precise nomenclature. Neurocrit Care. (2019) 30:239–43. doi: 10.1007/s12028-018-0613-x
31. Ostrup, RC, Luerssen, TG, Marshall, LF, and Zornow, MH. Continuous monitoring of intracranial pressure with a miniaturized fiberoptic device. J Neurosurg. (1987) 67:206–9. doi: 10.3171/jns.1987.67.2.0206
32. Reulen, HJ, and Kreysch, HG. Measurement of brain tissue pressure in cold induced cerebral oedema. Acta Neurochir. (1973) 29:29–40.
33. Steiner, LA, and Andrews, PJD. Monitoring the injured brain: ICP and CBF. Br J Anaesth. (2006) 97:26–38. doi: 10.1093/bja/ael110
34. Zhong, J, Dujovny, M, Park, HK, Perez, E, Perlin, AR, and Diaz, FG. Advances in ICP monitoring techniques. Neurol Res. (2003) 25:339–50. doi: 10.1179/016164103101201661
35. March, K. Intracranial pressure monitoring. AACN Clin Issues. (2005) 16:456–75. doi: 10.1097/00044067-200510000-00004
36. Binz, DD, Toussaint, LG, and Friedman, JA. Hemorrhagic complications of Ventriculostomy placement: a Meta-analysis. Neurocrit Care. (2009) 10:253. doi: 10.1007/s12028-009-9193-0
37. Beer, R, Lackner, P, Pfausler, B, and Schmutzhard, E. Nosocomial ventriculitis and meningitis in neurocritical care patients. J Neurol. (2008) 255:1617–24. doi: 10.1007/s00415-008-0059-8
38. Lozier, AP, Sciacca, RR, Romagnoli, MF, and Connolly, ES. Ventriculostomy-related infections: a critical review of the literature. Neurosurgery. (2002) 51:170–82. doi: 10.1097/00006123-200207000-00024
39. Reid, A, Marchbanks, RJ, Bateman, DE, Martin, AM, Brightwell, AP, and Pickard, JD. Mean intracranial pressure monitoring by a non-invasive audiological technique: a pilot study. J Neurol Neurosurg Psychiatry. (1989) 52:610–2. doi: 10.1136/jnnp.52.5.610
40. Saladino, A, White, JB, Wijdicks, EFM, and Lanzino, G. Malplacement of ventricular catheters by neurosurgeons: a single institution experience. Neurocrit Care. (2009) 10:248–52. doi: 10.1007/s12028-008-9154-z
41. Muralidharan, R. External ventricular drains: management and complications. Surg Neurol Int. (2015) 6:271. doi: 10.4103/2152-7806.157620
42. Chau, CYC, Craven, CL, Rubiano, AM, Adams, H, Tülü, S, Czosnyka, M, et al. The evolution of the role of external ventricular drainage in traumatic brain injury. J Clin Med. (2019) 8:1422. doi: 10.3390/jcm8091422
43. Kristiansson, H, Nissborg, E, Bartek, J, Andresen, M, Reinstrup, P, and Romner, B. Measuring elevated Intracranial pressure through noninvasive methods. J Neurosurg Anesthesiol. (2013) 25:372–85. doi: 10.1097/ANA.0b013e31829795ce
44. Toutant, SM, Klauber, MR, Marshall, LF, Toole, BM, Bowers, SA, Seelig, JM, et al. Absent or compressed basal cisterns on first CT scan: ominous predictors of outcome in severe head injury. J Neurosurg. (1984) 61:691–4. doi: 10.3171/jns.1984.61.4.0691
45. Rosenberg, JB, Shiloh, AL, Savel, RH, and Eisen, LA. Non-invasive methods of estimating intracranial pressure. Neurocrit Care. (2011) 15:599–608. doi: 10.1007/s12028-011-9545-4
46. Bidot, S, Saindane, AM, Peragallo, JH, Bruce, BB, Newman, NJ, and Biousse, V. Brain imaging in idiopathic Intracranial hypertension. J Neuroophthalmol. (2015) 35:400–11. doi: 10.1097/WNO.0000000000000303
47. Maralani, PJ, Hassanlou, M, Torres, C, Chakraborty, S, Kingstone, M, Patel, V, et al. Accuracy of brain imaging in the diagnosis of idiopathic intracranial hypertension. Clin Radiol. (2012) 67:656–63. doi: 10.1016/j.crad.2011.12.002
48. Alperin, NJ, Lee, SH, Loth, F, Raksin, PB, and Lichtor, T. MR-Intracranial pressure (ICP): a method to measure Intracranial Elastance and pressure noninvasively by means of MR imaging: baboon and human study. Radiology. (2000) 217:877–85. doi: 10.1148/radiology.217.3.r00dc42877
49. Marmarou, A, Shulman, K, and LaMorgese, J. Compartmental analysis of compliance and outflow resistance of the cerebrospinal fluid system. J Neurosurg. (1975) 43:523–34.
50. Ridha, MA, Saindane, AM, Bruce, BB, Riggeal, BD, Kelly, LP, Newman, NJ, et al. Magnetic resonance imaging findings of elevated Intracranial pressure in cerebral venous thrombosis versus idiopathic Intracranial hypertension with transverse sinus stenosis. Neuro-Ophthalmology. (2013) 37:1–6. doi: 10.3109/01658107.2012.738759
51. Marshall, I, Maccormick, I, Sellar, R, and Whittle, I. Assessment of factors affecting MRI measurement of intracranial volume changes and elastance index. Br J Neurosurg. (2008) 22:389–97. doi: 10.1080/02688690801911598
52. Hunter, G, Voll, C, and Rajput, M. Utility of transcranial Doppler in idiopathic Intracranial hypertension. Can J Neurol Sci. (2010) 37:235–9. doi: 10.1017/S0317167100009987
53. Gur, AY, Kesler, A, Shopin, L, and Bornstein, NM. Transcranial Doppler for evaluation of idiopathic intracranial hypertension. Acta Neurol Scand. (2007) 116:239–42. doi: 10.1111/j.1600-0404.2007.00861.x
54. Pradeep, R, Gupta, D, Shetty, N, Bhushan, AK, Haskar, K, Gogineni, S, et al. Transcranial Doppler for Monitoring and evaluation of idiopathic Intracranial hypertension. J Neurosci Rural Pract. (2020) 11:309–14. doi: 10.1055/s-0040-1710086
55. Marchbanks, RJ. Measurement of tympanic membrane displacement arising from aural cardiovascular activity, swallowing, and intra-aural muscle reflex. Acta Otolaryngol. (1984) 98:119–29.
56. Samuel, M, Burge, DM, and Marchbanks, RJ. Tympanic membrane displacement testing in regular assessment of intracranial pressure in eight children with shunted hydrocephalus. J Neurosurg. (1998) 88:983–95. doi: 10.3171/jns.1998.88.6.0983
57. Reid, A, Marchbanks, RJ, Burge, DM, Martin, AM, Bateman, DE, Pickard, JD, et al. The relationship between intracranial pressure and tympanic membrane displacement. Br J Audiol. (1990) 24:123–9.
58. Campbell-Bell, CM, Birch, AA, Vignali, D, Bulters, D, and Marchbanks, RJ. Reference intervals for the evoked tympanic membrane displacement measurement: a non-invasive measure of intracranial pressure. Physiol Meas. (2018) 39:015008. doi: 10.1088/1361-6579/aaa1d3
59. Bershad, EM, Urfy, MZ, Pechacek, A, McGrath, M, Calvillo, E, Horton, NJ, et al. Intracranial pressure modulates distortion product Otoacoustic emissions. Neurosurgery. (2014) 75:445–55. doi: 10.1227/NEU.0000000000000449
60. Voss, SE, Adegoke, MF, Horton, NJ, Sheth, KN, Rosand, J, and Shera, CA. Posture systematically alters ear-canal reflectance and DPOAE properties. Hear Res. (2010) 263:43–51. doi: 10.1016/j.heares.2010.03.003
61. MACS, V, MAS, C, Costa, DL, Eulálio, KD, Vale, OC, CPB, V, et al. Visual evoked potentials show strong positive association with intracranial pressure in patients with cryptococcal meningitis. Arq Neuropsiquiatr. (2015) 73:309–13. doi: 10.1590/0004-282X20150002
62. Xu, W, Gerety, P, Aleman, T, Swanson, J, and Taylor, J. Noninvasive methods of detecting increased intracranial pressure. Childs Nerv Syst. (2016) 32:1371–86. doi: 10.1007/s00381-016-3143-x
63. Drislane, FW. Visual evoked potentials In: The clinical neurophysiology primer. Totowa, NJ: Humana Press. 461–73.
64. York, D, Legan, M, Benner, S, and Watts, C. Further studies with a noninvasive method of Intracranial pressure estimation. Neurosurgery. (1984) 14:456–61. doi: 10.1227/00006123-198404000-00011
65. York, DH, Pulliam, MW, Rosenfeld, JG, and Watts, C. Relationship between visual evoked potentials and intracranial pressure. J Neurosurg. (1981) 55:909–16. doi: 10.3171/jns.1981.55.6.0909
66. Stone, JL, Ghaly, RF, and Hughes, JR. Evoked potentials in head injury and states of increased Intracranial pressure. J Clin Neurophysiol. (1988) 5:135–60. doi: 10.1097/00004691-198804000-00002
67. Sjöström, A, Uvebrant, P, and Roos, A. The light-flash-evoked response as a possible indicator of increased intracranial pressure in hydrocephalus. Childs Nerv Syst. (1995) 11:381–7. doi: 10.1007/BF00717400
68. Desch, LW. Longitudinal stability of visual evoked potentials in children and adolescents with hydrocephalus. Dev Med Child Neurol. (2001) 43:113. doi: 10.1017/s0012162201000196
69. StuartG, C, and Cochrane, DD. Visual evoked potentials, intracranial pressure and ventricular size in hydrocephalus. Doc Ophthalmol. (1987) 66:321–9. doi: 10.1007/BF00213660
70. Kesler, A, Vakhapova, V, Korczyn, AD, and Drory, VE. Visual evoked potentials in idiopathic intracranial hypertension. Clin Neurol Neurosurg. (2009) 111:433–6. doi: 10.1016/j.clineuro.2008.12.008
71. Bobak, P. Visual evoked potentials to multiple temporal frequencies. Arch Ophthalmol. (1988) 106:936. doi: 10.1001/archopht.1988.01060140082029
72. Falsini, B, Tamburrelli, C, Porciatti, V, Anile, C, Porrello, G, and Mangiola, N. Pattern Electroretinograms and visual evoked potentials in idiopathic Intracranial hypertension. Ophthalmologica. (1992) 205:194–203. doi: 10.1159/000310341
73. Verplanck, M, Kaufman, DI, Parsons, T, Yedavally, S, and Kokinakis, D. Electrophysiology versus psychophysics in the detection of visual loss in pseudotumor cerebri. Neurology. (1988) 38:1789–9. doi: 10.1212/WNL.38.11.1789
74. Onofrj, M, Bodis-Wollner, I, and Mylin, L. Visual evoked potential latencies in papilledema and hydrocephalus. Neuro-Ophthalmology. (1981) 2:85–92.
75. Sorensen, PS, Trojaborg, W, Gjerris, F, and Krogsaa, B. Visual evoked potentials in Pseudotumor Cerebri. Arch Neurol. (1985) 42:150–3. doi: 10.1001/archneur.1985.04060020064017
76. Hamamci, M, and Tombul, T. Visual evoked potentials follow-up in idiopathic intracranial hypertension. Neurosciences. (2019) 24:185–91. doi: 10.17712/nsj.2019.3.20190004
77. Donnell, CP. Visually Evoked Potentials [Internet]. Salt Lake City, UT: University of Utah Health Sciences Center (1995) Webvision: The Organization of the Retina and Visual System; 2012 [cited 2024 Aug 31]. 1–30. Available at: https://www.ncbi.nlm.nih.gov/books/NBK107218/.
78. Canac, N, Jalaleddini, K, Thorpe, SG, Thibeault, CM, and Hamilton, RB. Review: pathophysiology of intracranial hypertension and noninvasive intracranial pressure monitoring. Fluids Barr CNS. (2020) 17:40. doi: 10.1186/s12987-020-00201-8
79. Kupersmith, MJ, Sibony, P, Mandel, G, Durbin, M, and Kardon, RH. Optical coherence tomography of the swollen optic nerve head: deformation of the Peripapillary retinal pigment epithelium layer in papilledema. Investigat Opthalmol Vis Sci. (2011) 52:6558. doi: 10.1167/iovs.10-6782
80. Hayreh, SS. PATHOGENESIS OF OEDEMA OF THE OPTIC DISC (PAPILLOEDEMA). A PRELIMINARY REPORT. Brit J Ophthal [Internet]. (1964) 48:522–43. doi: 10.1136/bjo.48.10.522
81. Karam, EZ. Optical coherence tomography of the retinal nerve fibre layer in mild papilloedema and pseudopapilloedema. Br J Ophthalmol. (2005) 89:294–8. doi: 10.1136/bjo.2004.049486
82. Moss, HE, Vangipuram, G, Shirazi, Z, and Shahidi, M. Retinal vessel diameters change within 1 hour of Intracranial pressure lowering. Transl Vis Sci Technol [Internet]. (2018) 7:6. doi: 10.1167/tvst.7.2.6
83. Onder, H, Goksungur, G, Eliacik, S, Kasim Ulusoy, E, and Arslan, G. Neurological research the significance of ONSD, ONSD/ETD ratio, and other neuroimaging parameters in idiopathic intracranial hypertension the significance of ONSD, ONSD/ETD ratio, and other neuroimaging parameters in idiopathic intracranial hypertension. (2021). Available at: https://www.tandfonline.com/action/journalInformation?journalCode=yner20.
84. Huang-Link, YM, Al-Hawasi, A, Oberwahrenbrock, T, and Jin, YP. OCT measurements of optic nerve head changes in idiopathic intracranial hypertension. Clin Neurol Neurosurg. (2015) 130:122–7. doi: 10.1016/j.clineuro.2014.12.021
85. Caffery, TS, Perret, JN, Musso, MW, and Jones, GN. Optic nerve sheath diameter and lumbar puncture opening pressure in nontrauma patients suspected of elevated intracranial pressure. Am J Emerg Med. (2014) 32:1513–5. doi: 10.1016/j.ajem.2014.09.014
86. Amini, A, Kariman, H, Arhami Dolatabadi, A, Hatamabadi, HR, Derakhshanfar, H, Mansouri, B, et al. Use of the sonographic diameter of optic nerve sheath to estimate intracranial pressure. Am J Emerg Med. (2013) 31:236–9. doi: 10.1016/j.ajem.2012.06.025
87. Abdelrahman, AS, and Barakat, MMK. MRI measurement of optic nerve sheath diameter using 3D driven equilibrium sequence as a non-invasive tool for the diagnosis of idiopathic intracranial hypertension. Egypt J Radiol Nucl Med. (2020) 51:24. doi: 10.1186/s43055-020-0149-x
88. Kimberly, HH, Shah, S, Marill, K, and Noble, V. Correlation of optic nerve sheath diameter with direct measurement of Intracranial pressure. Acad Emerg Med. (2008) 15:201–4. doi: 10.1111/j.1553-2712.2007.00031.x
89. Blaivas, M, Theodoro, D, and Sierzenski, PR. BRIEF REPORTS elevated Intracranial pressure detected by bedside emergency ultrasonography of the optic nerve sheath [internet]. Acad Emerg Med. (2003) 10:376–81. doi: 10.1111/j.1553-2712.2003.tb01352.x
90. Wang, L, Feng, L, Yao, Y, Wang, Y, Chen, Y, Feng, J, et al. Optimal optic nerve sheath diameter threshold for the identification of elevated opening pressure on lumbar puncture in a Chinese population. PLoS One. (2015) 10:117939. doi: 10.1371/journal.pone.0119723
91. Price, DA, Grzybowski, A, Eikenberry, J, Januleviciene, I, Verticchio Vercellin, AC, Mathew, S, et al. Review of non-invasive intracranial pressure measurement techniques for ophthalmology applications. Br J Ophthalmol. (2020) 104:887–92. doi: 10.1136/bjophthalmol-2019-314704
92. Aletreby, W, Alharthy, A, Brindley, PG, Kutsogiannis, DJ, Faqihi, F, Alzayer, W, et al. Optic nerve sheath diameter ultrasound for raised intracranial pressure a literature review and meta-analysis of its diagnostic accuracy. J Ultrasound Med. (2022) 41:585–95. doi: 10.1002/jum.15732
93. Geeraerts, T, Newcombe, VF, Coles, JP, Abate, M, Perkes, IE, Hutchinson, PJ, et al. Use of T2-weighted magnetic resonance imaging of the optic nerve sheath to detect raised intracranial pressure. Crit Care. (2008) 12:R114. doi: 10.1186/cc7006
94. Watanabe, A, Kinouchi, H, Horikoshi, T, Uchida, M, and Ishigame, K. Effect of intracranial pressure on the diameter of the optic nerve sheath. J Neurosurg. (2008) 109:255–8. doi: 10.3171/JNS/2008/109/8/0255
95. Sekhon, MS, Griesdale, DE, Robba, C, McGlashan, N, Needham, E, Walland, K, et al. Optic nerve sheath diameter on computed tomography is correlated with simultaneously measured intracranial pressure in patients with severe traumatic brain injury. Intensive Care Med. (2014) 40:1267–74. doi: 10.1007/s00134-014-3392-7
96. Maude, RR, Hossain, A, Hassan, MU, Osbourne, S, Langan, K, Sayeed, A, et al. Transorbital sonographic evaluation of normal optic nerve sheath diameter in healthy volunteers in Bangladesh. PLoS One. (2013) 8:e81013. doi: 10.1371/journal.pone.0081013
97. Sallam, A, Alkhatip, AAAMM, Kamel, MG, Hamza, MK, Yassin, HM, Hosny, H, et al. The diagnostic accuracy of noninvasive methods to measure the Intracranial pressure: a systematic review and meta-analysis. Anesth Analg. (2021) 132:686–95. doi: 10.1213/ANE.0000000000005189
98. Lee, SH, Kim, HS, and Yun, SJ. Optic nerve sheath diameter measurement for predicting raised intracranial pressure in adult patients with severe traumatic brain injury: a meta-analysis. J Crit Care. (2020) 56:182–7. doi: 10.1016/j.jcrc.2020.01.006
99. Koziarz, A, Sne, N, Kegel, F, Nath, S, Badhiwala, JH, Nassiri, F, et al. Bedside optic nerve ultrasonography for diagnosing increased Intracranial pressure. Ann Intern Med. (2019) 171:896. doi: 10.7326/M19-0812
100. Robba, C, Santori, G, Czosnyka, M, Corradi, F, Bragazzi, N, Padayachy, L, et al. Optic nerve sheath diameter measured sonographically as non-invasive estimator of intracranial pressure: a systematic review and meta-analysis. Intensive Care Med. (2018) 44:1284–94. doi: 10.1007/s00134-018-5305-7
101. Kim, SE, Hong, EP, Kim, HC, Lee, SU, and Jeon, JP. Ultrasonographic optic nerve sheath diameter to detect increased intracranial pressure in adults: a meta-analysis. Acta Radiol. (2019) 60:221–9. doi: 10.1177/0284185118776501
102. Ohle, R, McIsaac, SM, Woo, MY, and Perry, JJ. Sonography of the optic nerve sheath diameter for detection of raised Intracranial pressure compared to computed tomography. J Ultrasound Med. (2015) 34:1285–94. doi: 10.7863/ultra.34.7.1285
103. Kwee, RM, and Kwee, TC. Systematic review and meta-analysis of MRI signs for diagnosis of idiopathic intracranial hypertension. Eur J Radiol. (2019) 116:106–15. doi: 10.1016/j.ejrad.2019.04.023
104. Rebolleda, G, and Muñoz-Negrete, FJ. Follow-up of mild papilledema in idiopathic Intracranial hypertension with optical coherence tomography. Investigat Opthalmol Vis Sci. (2009) 50:5197. doi: 10.1167/iovs.08-2528
105. Scott, CJ. Diagnosis and grading of papilledema in patients with raised Intracranial pressure using optical coherence tomography vs clinical expert assessment using a clinical staging scale. Arch Ophthalmol. (2010) 128:705. doi: 10.1001/archophthalmol.2010.94
106. Malhotra, K, Padungkiatsagul, T, and Moss, HE. Optical coherence tomography use in idiopathic intracranial hypertension. Ann Eye Sci. (2020) 5:7. doi: 10.21037/aes.2019.12.06
107. Sibony, PA, Kupersmith, MJ, Feldon, SE, Wang, JK, Garvin, M, Auinger, P, et al. Retinal and choroidal folds in papilledema. Invest Ophthalmol Vis Sci. (2015) 56:5670–80. doi: 10.1167/iovs.15-17459
108. Laemmer, R, Heckmann, JG, Mardin, CY, Schwab, S, and Laemmer, AB. Detection of nerve fiber atrophy in apparently effectively treated papilledema in idiopathic intracranial hypertension. Graefes Arch Clin Exp Ophthalmol. (2010) 248:1787–93. doi: 10.1007/s00417-010-1465-z
109. Lee, A, Rigi, M, Almarzouqi, S, and Morgan, M. Papilledema: epidemiology, etiology, and clinical management. Eye Brain. (2015) 7:47. doi: 10.2147/EB.S69174
110. Eren, Y, Kabatas, N, Guven, H, Comoglu, S, and Gurdal, C. Evaluation of optic nerve head changes with optic coherence tomography in patients with idiopathic intracranial hypertension. Acta Neurol Belg. (2019) 119:351–7. doi: 10.1007/s13760-018-1000-2
111. Waisbourd, M, Leibovitch, I, Goldenberg, D, and Kesler, A. OCT assessment of morphological changes of the optic nerve head and macula in idiopathic intracranial hypertension. Clin Neurol Neurosurg. (2011) 113:839–43. doi: 10.1016/j.clineuro.2011.05.015
112. Jacobsen, HH, Jørstad, ØK, Moe, MC, Petrovski, G, Pripp, AH, Sandell, T, et al. Noninvasive estimation of pulsatile and static Intracranial pressure by optical coherence tomography. Transl Vis Sci Technol. (2022) 11:31. doi: 10.1167/tvst.11.1.31
113. Kaufhold, F, Kadas, EM, Schmidt, C, Kunte, H, Hoffmann, J, Zimmermann, H, et al. Optic nerve head quantification in idiopathic Intracranial hypertension by spectral domain OCT. PLoS One. (2012) 7:e36965. doi: 10.1371/journal.pone.0036965
114. Skau, M, Yri, H, Sander, B, Gerds, TA, Milea, D, and Jensen, R. Diagnostic value of optical coherence tomography for intracranial pressure in idiopathic intracranial hypertension. Graefes Arch Clin Exp Ophthalmol. (2013) 251:567–74. doi: 10.1007/s00417-012-2039-z
115. Moss, HE, Treadwell, G, Wanek, J, DeLeon, S, and Shahidi, M. Retinal vessel diameter assessment in papilledema by semi-automated analysis of SLO images: feasibility and reliability. Investigat Opthalmol Vis Sci. (2014) 55:2049. doi: 10.1167/iovs.13-13621
116. Andersen, MS, Pedersen, CB, and Poulsen, FR. A new novel method for assessing intracranial pressure using non-invasive fundus images: a pilot study. Sci Rep. (2020) 10:13062. doi: 10.1038/s41598-020-70084-0
117. Wall, M, McDermott, MP, Kieburtz, KD, Corbett, JJ, Feldon, SE, Friedman, DI, et al. Effect of acetazolamide on visual function in patients with idiopathic Intracranial hypertension and mild visual loss. JAMA. (2014) 311:1641. doi: 10.1001/jama.2014.3312
118. Moss, HE. Retinal vein changes as a biomarker to guide diagnosis and Management of Elevated Intracranial Pressure. Front Neurol. (2021) 12:751370. doi: 10.3389/fneur.2021.751370
119. Morgan, WH, Hazelton, ML, Azar, SL, House, PH, Yu, DY, Cringle, SJ, et al. Retinal venous pulsation in glaucoma and glaucoma suspects. Ophthalmology. (2004) 111:1489–94. doi: 10.1016/j.ophtha.2003.12.053
120. Morgan, WH, Hazelton, ML, and Yu, DY. Retinal venous pulsation: expanding our understanding and use of this enigmatic phenomenon. Prog Retin Eye Res. (2016) 55:82–107. doi: 10.1016/j.preteyeres.2016.06.003
121. Morgan, WH, Lind, CRP, Kain, S, Fatehee, N, Bala, A, and Yu, DY. Retinal vein pulsation is in phase with intracranial pressure and not intraocular pressure. Invest Ophthalmol Vis Sci. (2012) 53:4676–81. doi: 10.1167/iovs.12-9837
122. Jacks, AS, and Miller, NR. Spontaneous retinal venous pulsation: Aetiology and significance. J Neurol Neurosurg Psychiatry. (2003) 74:7–9. doi: 10.1136/jnnp.74.1.7
123. Levin, BE. The clinical significance of spontaneous pulsations of the retinal vein. Arch Neurol. (1978) 35:37–40. doi: 10.1001/archneur.1978.00500250041009
124. D’Antona, L, McHugh, JA, Ricciardi, F, Thorne, LW, Matharu, MS, Watkins, LD, et al. Association of intracranial pressure and spontaneous retinal venous pulsation. JAMA Neurol. (2019) 76:1502. doi: 10.1001/jamaneurol.2019.2935
125. Engel, S. Venous pulsation as a symptom of early Glaucoma*. Am J Ophthalmol. (1946) 29:1446–8. doi: 10.1016/0002-9394(46)92041-7
126. Wong, SH, and White, RP. The clinical validity of the spontaneous retinal venous pulsation. J Neuroophthalmol. (2013) 33:17–20. doi: 10.1097/WNO.0b013e3182622207
127. Laurent, C, Hong, SC, Cheyne, KR, and Ogbuehi, KC. The detection of spontaneous venous pulsation with smartphone video ophthalmoscopy. Clin Ophthalmol. (2020) 14:331–7. doi: 10.2147/OPTH.S238897
128. Shariflou, S, Agar, A, Rose, K, Bowd, C, and Golzan, SM. Objective quantification of spontaneous retinal venous pulsations using a novel tablet-based ophthalmoscope. Transl Vis Sci Technol. (2020) 9:19. doi: 10.1167/tvst.9.4.19
129. Yuh, WTC, Zhu, M, Taoka, T, Quets, JP, Maley, JE, Muhonen, MG, et al. MR imaging of pituitary morphology in idiopathic intracranial hypertension. J Magn Reson Imaging. (2000) 12:808–13. doi: 10.1002/1522-2586(200012)12:6<808::AID-JMRI3>3.0.CO;2-N
130. Brodsky, M. Magnetic resonance imaging in pseudotumor cerebri. Ophthalmology. (1998) 105:1686–93. doi: 10.1016/S0161-6420(98)99039-X
131. Agid, R, Farb, RI, Willinsky, RA, Mikulis, DJ, and Tomlinson, G. Idiopathic intracranial hypertension: the validity of cross-sectional neuroimaging signs. Neuroradiology. (2006) 48:521–7. doi: 10.1007/s00234-006-0095-y
132. Saindane, AM, Bruce, BB, Riggeal, BD, Newman, NJ, and Biousse, V. Association of MRI findings and visual outcome in idiopathic Intracranial hypertension. Am J Roentgenol. (2013) 201:412–8. doi: 10.2214/AJR.12.9638
133. da Silveira Carvalho, G, de Andrade Matas, SL, Idagawa, MH, Tibana, LAT, de Carvalho, RS, Silva, MLS, et al. A new index for the assessment of transverse sinus stenosis for diagnosing idiopathic intracranial hypertension. J Neurointerv Surg. (2017) 9:173–7. doi: 10.1136/neurintsurg-2016-012605
Keywords: optic nerve sheath diameter, idiopathic intracranial hypertension, spontaneous venous pulsation, vessel analysis, non-invasive and invasive, intracranial pressure, cerebrospinal fluid
Citation: Bastani Viarsagh S, Agar A, Lawlor M, Fraser C and Golzan M (2024) Non-invasive assessment of intracranial pressure through the eyes: current developments, limitations, and future directions. Front. Neurol. 15:1442821. doi: 10.3389/fneur.2024.1442821
Edited by:
Sachin Kedar, Emory University, United StatesReviewed by:
Rebecca Wicklein, Technical University of Munich, GermanyLucia Ambrosio, University of Naples Federico II, Italy
Michael Dattilo, Emory University, United States
Copyright © 2024 Bastani Viarsagh, Agar, Lawlor, Fraser and Golzan. This is an open-access article distributed under the terms of the Creative Commons Attribution License (CC BY). The use, distribution or reproduction in other forums is permitted, provided the original author(s) and the copyright owner(s) are credited and that the original publication in this journal is cited, in accordance with accepted academic practice. No use, distribution or reproduction is permitted which does not comply with these terms.
*Correspondence: Solmaz Bastani Viarsagh, c29sbWF6LmJhc3Rhbml2aWFyc2FnaEBzdHVkZW50LnV0cy5lZHUuYXU=