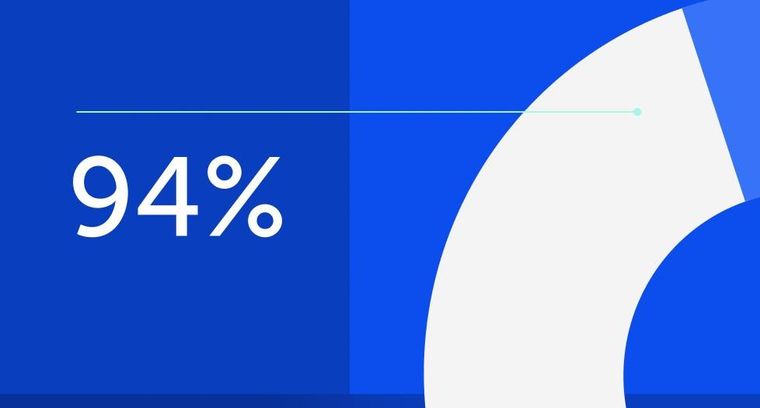
94% of researchers rate our articles as excellent or good
Learn more about the work of our research integrity team to safeguard the quality of each article we publish.
Find out more
REVIEW article
Front. Neurol., 29 July 2024
Sec. Stroke
Volume 15 - 2024 | https://doi.org/10.3389/fneur.2024.1410525
Recently, the role of high-concentration oxygen therapy in cerebral hemorrhage has been extensively discussed. This review describes the research progress in high-concentration oxygen therapy after cerebral hemorrhage. High-concentration oxygen therapy can be classified into two treatment methods: hyperbaric and normobaric high-concentration oxygen therapy. Several studies have reported that high-concentration oxygen therapy uses the pathological mechanisms of secondary ischemia and hypoxia after cerebral hemorrhage as an entry point to improve cerebral oxygenation, metabolic rate, cerebral edema, intracranial pressure, and oxidative stress. We also elucidate the mechanisms by which molecules such as Hypoxia-inducible factor 1-alpha (HIF-1α), vascular endothelial growth factor, and erythropoietin (EPO) may play a role in oxygen therapy. Although people are concerned about the toxicity of hyperoxia, combined with relevant literature, the evidence discussed in this article suggests that as long as the duration, concentration, pressure, and treatment interval of patients with cerebral hemorrhage are properly understood and oxygen is administered within the treatment window, it can be effective to avoid hyperoxic oxygen toxicity. Combined with the latest research, we believe that high-concentration oxygen therapy plays an important positive role in injuries and outcomes after cerebral hemorrhage, and we recommend expanding the use of normal-pressure high-concentration oxygen therapy for cerebral hemorrhage.
Spontaneous intracerebral hemorrhage (ICH) refers to the hemorrhage of brain parenchyma caused by vascular rupture caused by non-traumatic causes. With an increase in population age and widespread use of antithrombotic drugs, risk factors such as hypertension, diabetes, obesity, and alcohol abuse have increased, and the incidence of ICH is also increasing (1). Its high mortality and disability rates are closely related to neuronal damage caused by pathological reactions such as perifocal hypoxia after ICH. Surviving patients often experience permanent sequelae (2).
Brain injury after ICH can be classified into primary and secondary injuries. The primary injury is mechanical compression and expansion of the hematoma, which are key factors in determining the progression and outcome of ICH. They are generally caused by continued bleeding from ruptured blood vessels (3), usually occurring within 6 h after ICH, which can induce a space-occupying effect, compress blood vessels, reduce the volume of the vascular bed, increase intracranial pressure (ICP), decrease local perfusion, inhibit membrane ion pump activity (4), increase intracellular sodium ions, decrease intracellular crystal osmotic pressure, and form cytotoxic edema (5). The decrease of calcium ions in serum impairs the thrombin cascade reaction, and coagulation dysfunction (3) forms thrombosis, resulting in cerebral microcirculation obstruction and decreased oxygenation.
Hematoma components can induce secondary ICH. Thrombin is one of the components of hematoma. A high concentration of thrombin activated by the thrombin cascade reaction after ICH is closely associated with secondary ICH injury (4, 6). Thrombin can induce the production of inflammatory mediators, cause nerve cell damage (7), and influence neurological outcomes after ICH. Thrombin can also cleave the protease-activated receptors (PARs) receptor in microglia, which in turn phosphorylates the Src family kinase, thereby aggravating brain edema and the destruction of the blood–brain barrier. Stimulated microglia can also destroy tight junction proteins by up-regulating the expression of tumor necrosis factor (TNF) (6), increasing blood–brain barrier permeability. The complement cascade activated by thrombin may promote inflammation and edema after ICH through anaphylactoid toxins, or it may lyse red blood cells through membrane complexes to produce hemoglobin, iron, and oxygen free radicals, promote edema and oxidative stress and accelerate apoptosis after ICH, death, and blood–brain barrier disruption (4, 6). Red blood cells are also one of the components of hematoma. After intracerebral hemorrhage, the blood overflows from the ruptured blood vessels, and some red blood cells that are not completely phagocytized by microglia and infiltrating macrophages are directly released into the central system. Substances such as hemoglobin, iron, and carbonic anhydrase-1 are neurotoxic and are closely related to secondary brain injury after ICH (8). In addition, the automatic adjustment function of cerebral blood flow is based on a formula for cerebral blood flow, cerebral perfusion pressure (CPP), and cerebral vascular resistance (CVR). CVR can be adjusted to ensure the supply of cerebral blood flow (CBF) when ICP increases and CPP decreases. Expanded hematoma and high intracranial pressure after ICH cause decompensation of this regulation, further reducing CPP and aggravating cerebral ischemia (9). It is important to note that because hypertension is the most common cause of ICH (10), antihypertensive measures are often taken to alleviate the expansion of ICH hematoma; however, this is undoubtedly a challenge to the automatic regulation of cerebral blood flow. Therefore, attention should be paid to the tolerance of patients with hypertensive ICH to rapid blood pressure reduction; otherwise, insufficient cerebral perfusion will occur (11). Adnan et al. recommended that for intensive blood pressure reduction in ICH, systolic blood pressure should be controlled at 130–150 mmHg (12). Notably, cerebral venous outflow disorder also has a significant impact on the pathological mechanisms of ICH. Some researchers have found that cerebral venous outflow disorders are closely related to cerebrospinal fluid dynamics (13), especially in the internal jugular vein (IJV), which is the main route of brain drainage (14). Feng et al. (14) found that among patients with ICH, those with positive internal jugular venous reflux had larger perihematomal edema (PHE) volume than those with negative internal jugular venous reflux and demonstrated a positive correlation between jugular venous reflux (JVR) and PHE volume. When JVR worsens, it can further increase ICP, reduce CBF and CPP, aggravate vasogenic edema, and reduce cerebral oxygenation (14, 15). In general, secondary injuries can include vasogenic edema, neuroinflammatory reactions, blood–brain barrier damage, decompensation of cerebral blood flow autoregulation, excessive lowering of blood pressure, and cerebral venous return disorder, which are related. The pathological mechanisms are different, but to a certain extent, they can synergistically aggravate pathological reactions such as brain edema, neuroinflammatory reactions, high ICP, low CPP, oxidative stress, cell apoptosis, and destruction of the blood–brain barrier after ICH. From a comprehensive literature review, we believe that ischemia and hypoxia after ICH intersect and interact with these pathological reactions (16, 17). (The details are shown in Figure 1.)
A green stroke channel has been established clinically, and the slogan “time is brain” has been put forward because ICH is dangerous and progresses rapidly. Although there is currently no specific clinical treatment for ICH, the pathological basis of ICH can be explored to improve patient prognosis. Recently, high-concentration oxygen therapy has become increasingly active in the public eye. This emerging and effective intervention can alleviate ischemic hypoxic conditions after ICH, reduce intracranial pressure and cerebral edema, and improve neuroinflammatory reactions and other adverse effects. This study aimed to explore the progress in the efficacy of high-concentration oxygen therapy for ICH. Clinically, common high-concentration oxygen therapies used after ICH can be categorized into hyperbaric oxygen (HBO) and normobaric oxygen (NBO).
HBO is a treatment method in which a patient intermittently inhales 100% pure oxygen in a high-pressure chamber at a pressure greater than sea level [>1 Atmospheres Absolute (ATA)] (9). Henry’s law provides an understanding of the basis of HBO treatment; that is, the proportion of dissolved oxygen is positively related to the partial pressure of oxygen (18). HBO can increase the oxygen partial pressure in the blood and damaged tissues by increasing the dissolved oxygen content, improving cerebral oxygenation, and increasing the oxygen metabolic rate (19, 20) (Table 1).
Table 1. The possible mechanism and effect of high-concentration oxygen therapy after ICH and the potential therapeutic effect of high-concentration oxygen therapy.
Noemí et al. (31) conducted a longitudinal [18F]-fluorimidazole ([18F]-FMISO) PET/MRI study. They found that when the intracerebral hematoma volume peaked after ICH, the HIF-1α content in the damaged tissue was equal to that of [18F]-FMISO. The increased absorption of FMISO indicates a hypoperfusion state around the hematoma after ICH. Studies have found that HBO can supply oxygen to damaged areas of brain tissue, improve brain microcirculation, and promote angiogenesis in damaged brain tissue by promoting the regeneration of vascular endothelial cells (23). In an experiment to explore the effect of HBO on intracerebral blood vessels in rats after ICH, Zheng et al. found that on days 14 and 21, respectively, compared with the sham operation group and the ICH group, the HBO group had significantly increased HIF-1a expression and vascular endothelial growth factor (VEGF) positive microvessels. On days 14–28, the number of new blood vessels in the HBO group increased significantly. Combined with the rapid decline in behavioral scores in the HBO group over time, they believe HBO enables hematoma absorption by supplying oxygen and promoting the expression of HIF-1a and VEGF. Vascular endothelial cells proliferate, blood vessels regenerate and sprout, and cerebral blood flow significantly increases. Thus, oxygen and blood fully moisturize damaged brain tissue and neurons, ultimately improving brain microcirculation and nervous system damage after ICH. Some scholars have also discovered through ([18F]-FMISO) PET that early HBO can increase the glucose brain metabolism rate in ischemic tissue to a certain extent and effectively improve cerebral ischemia and hypoxia (26).
The severity of cerebral edema after ICH is closely related to the degree of brain damage. Therefore, reducing cerebral edema is an entry point for ICH treatment, and HBO has been shown to reduce cerebral edema in previous studies (32). In an experimental study (24), Zhou et al. found that after ICH induction, the aggravation of vasogenic edema accompanied an increase in barrier permeability, and the levels of pro-matrix metalloproteinase-9 (proMMP-9), matrix metalloproteinase-9 (MMP-9), and occludin in the bleeding hemisphere were significantly increased. Early initiation of HBO could reduce the levels of proMMP-9 expression, thereby avoiding the activation of MMP-9 and the destruction of occludin. Simultaneously, edema and destruction of the blood–brain barrier were significantly alleviated. These results demonstrate that HBO reduces brain edema and blood–brain barrier permeability after ICH by inhibiting the activation of MMP-9. MMP-9 belongs to the matrix metalloproteinase family. Its activation can degrade blood–brain barrier tight junction proteins, increase blood–brain barrier permeability, and promote brain edema. Tight junction proteins, including occludin, claudins, and zonula occludins (ZO-1, ZO-2, ZO-3), are essential for maintaining the stability of endothelial cells in the blood–brain barrier (33).
In a study by Yamamoto et al. (21), it was found that when exposed to HBO for a short period, endothelial cells produced the vasodilators nitric oxide (NO) and superoxide (O2-), and their combined product, peroxynitrite (ONOO-) can cause vasoconstriction and decrease blood flow, which appears to contradict the treatment needs of ICH. Interestingly, over a longer period, HBO can fully compensate for this reduction in CBF, resulting in an overall improvement in microinflammation, circulation, blood flow, and oxygen tension. From another perspective, HBO-induced vasoconstriction can reduce the extravasation of tissue fluid and cerebral blood volume, thereby reducing intracranial pressure. A reduction in intracranial pressure is also beneficial for alleviating brain swelling, ischemia, and oxygen deficiency (19).
After ICH, a large amount of heme is released from damaged red blood cells and infiltrates the surrounding hematoma of the injured brain tissue. After metabolism, the heme releases ferric iron. Iron overload induces excessive reactive oxygen species (ROS) production and oxidative stress. These effects may be one of the causes of increased lipid peroxidation observed after ICH (34). The same finding was reported in a previous clinical study. Compared to healthy subjects, the expression of low-density lipoprotein in the plasma of patients with acute stroke was significantly increased, indicating that the lipid peroxidation level of the latter was significantly higher than that of the former (35). It is clinically believed that the time of HBO treatment is controlled within 1 h and the pressure is controlled between 2-3ATA, which can reduce the probability of hyperoxia damage to the body (36). Oxidative stress refers to the body’s excessive production of oxygen free radicals under exogenous stimulation, leading to an imbalance in the body’s oxidation-antioxidation system. It can destroy normal cells, lipids, and proteins and damage the central nervous system (37). Therefore, active measures should be taken to fight against it. Breitenbach et al. believe that HBO can maintain the body’s oxidative and antioxidant balance as long as it is within the therapeutic and approved range. Within this range, the body’s spontaneous anti-oxidant mechanism is sufficient to combat the oxidative stress damage caused by HBO. HBO can also evoke antioxidant enzyme activity through the nuclear factor 2 (Nrf2) pathway, effectively reducing ROS levels. Furthermore, long-term HBO treatment can reduce mitophagy-induced apoptosis by enhancing mitochondrial activity (36). In other diseases, such as fibromyalgia, HBO has been shown to reduce lipid peroxidation levels and alleviate oxidative stress (38).
Clinical studies show (22) that HBO has a long-term neurological improvement effect in patients with diabetes and hemorrhagic stroke, Qian Xu et al. randomly divided 79 eligible patients into two groups, 31 patients in the HBO group received 2.5ATA for 60 min, and 48 patients in the NBO group received 1,5ATA for 60 min. There was no significant difference in the outcomes between the two groups 1 month after the onset of the disease, but 6 months after the onset of the disease, compared with the NBO group, the efficacy of the HBO group was significantly improved, especially in the two scales of Modified Rankin score and National Institute of Health Stroke Scale (NIHSS), they believed HBO may have functions such as increasing oxygenation, reducing cerebral edema, and regulating glial cell metabolism. Microglia are the guards of the brain. They are the first to activate the immune defense line after ICH and play an important role in clearing hematomas, engulfing damaged cells, and reducing inflammation (39). Lim-ing Yang et al. found through Iba1-DAPI double staining that compared with the ICH group, the microglia around the hematoma in the hyperbaric oxygen preconditioning (HBOP) group showed a significant downward trend at 12, 24, and 72 h. Fluoro-Jade C detection revealed that the hematoma and peripheral neuron degeneration in the HBOP group were also significantly reduced compared to that in the ICH group. Based on the neurobehavioral scores of the three groups of mice, they believed that HBOP reduced neuroinflammation by regulating the expression of microglia after ICH (25). When a patient undergoes HBOP, the partial pressure of oxygen in the blood remains high for some time. Therefore, clinical research has been conducted on the effects of administering HBO before ICH. This treatment method, called hyperbaric oxygen preconditioning (HBOP), also protects fragile nerves in ICH and has achieved clinical success (40). Some studies have also found that HBO has significantly more vascular endothelial cells and microvascular structures on 14d ~ 28d than the ICH group on 14d ~ 28d, and the behavioral scores of mice in the HBO group decreased significantly over time. Therefore, they believed that HBO could upregulate the expression levels of HIF-1α, VEGF, and other factors can promote vascular endothelial cell proliferation, angiogenesis, and hematoma absorption, alleviating damaged brain tissue’s ischemic and hypoxic state and improving nerve cell function and behavioral defects (23).
There are differing opinions on the optimal pressure and exposure time for HBO in experimental studies. These differences may be because of the different selection of experimental subjects and methods used to induce ICH models. Based on the treatment methods described in the literature, we believe that the two most commonly used animal models of ICH are injection of collagenase and infusion of autologous blood. Although both are not identical to the pathophysiology of clinical ICH, they provide a molecular basis. The former may be due to the fact that collagenase can degrade the basal interstitium, basement membrane, and blood leakage of blood vessels, leading to inflammation, edema, and destruction of the blood–brain barrier, which could be more dangerous than the clinical manifestations (6, 41, 42).
Meanwhile, the latter better imitates the blood mass effect, allowing for better efficacy in experimental studies of HBO treatment of ICH. In animal experiments, we also found that the therapeutic effect of rabbits may be better than that of mice (6, 41, 42). However, the results of animal experiments cannot be completely copied into clinical practice (1). Additional clinical studies are required to support this argument.
Based on current experimental research, long-term exposure to HBO with inappropriate pressure (pressure between 4 and 5 ATA, lasting more than 2 h) can easily induce central nervous system poisonings such as epilepsy, retinal damage, and pulmonary toxicity. This effect may be because the body produces excessive oxygen-free radicals, which leads to the exhaustion of antioxidants, causing oxidative stress damage, cell necrosis, and apoptosis (43–45). However, these side effects can be avoided by choosing an appropriate pressure and exposure time (44). In one study, it was considered safe to start the air brake every 20 to 30 min after exposure to 2.4 ATA for 60 to 90 min (46). After analyzing 22 patients with acute stroke who received HBO, McCormick et al. (47) believed that the most effective time window for HBO is within 3 h after ICH, and the earlier oxygen is administered, the better the effect. Their findings indicate that there is a time window for hyperbaric oxygen therapy. Further research is required to explore and standardize the safety of HBO. In addition, given the tightness and narrowness of the HBO treatment chamber, ICH patients need to be treated in the chamber alone for up to 2 h, which is very difficult for some patients with low compliance, especially those with severe conditions and high-risk factors for epilepsy. If there is a sudden attack during the treatment, the hyperbaric oxygen chamber cannot be abruptly stopped like MRI, because it involves the process of decompression, which is one of the limitations of HBO treatment (48). In addition, the paralysis rate of ICH is high, and most patients still have sequelae within a short period, requiring medical staff and family members to push them to the HBO treatment cabin, which reflects the inconvenience of HBO.
NBO is a treatment method that provides high-concentration oxygen through a mask or nasal cannula at sea level (1ATA) to increase the oxygen partial pressure. The oxygen concentration fraction was greater than 30% (10). NBO has low cost, high portability and safety, and no restrictions on its place of use. It is suitable for the use of ambulances when transporting patients with sudden ICH. Zhou et al. (24) found no difference in efficacy between HBO and NBO within 30 min of ICH, illustrating the effectiveness of the early adoption of NBO after ICH. NBO is also suitable for bedridden patients with limited mobility or home-based oxygen therapy after discharge. Its treatment mechanism is similar to that of HBO and is clinically effective in improving the damage and prognosis of ICH. Therefore, we recommend expanding the therapeutic applications of NBO after ICH.
NBO can relieve ischemia and hypoxia following ICH by improving oxygenation and reducing cerebral edema damage (49). You et al. (50) found through animal experiments that compared with the control group, the NBO group could significantly reduce the water content of damaged brain tissue, downregulate the initial expression of HIF-1α and VEGF, reduce cell apoptosis, and improve damaged nerve function, especially oxygen. The NBO group with a concentration of 90% showed the best efficacy. As mentioned earlier, HIF-1α and VEGF also benefit angiogenesis during brief hypoxia, thereby increasing cerebral blood flow and improving cerebral oxygenation and microcirculation (23).
In addition to improving the water content of tissue around hematoma by regulating the expression of HIF-1α and VEGF, NBO can also reduce brain edema by down-regulating the expression of aquaporin4 (AQP4). An animal experiment found that the AQP4 content of mice in the NBO group treated with 60 and 100% oxygen was significantly lower than that in the sham surgery group (29). AQP4 is a water-permeable channel protein, and its knockout can greatly reduce brain edema (51). Low AQP4 expression can indirectly alleviate secondary vasogenic edema by reducing initial cytotoxic edema after ICH, thereby improving blood–brain barrier permeability (52). NBO can also protect the blood–brain barrier by inhibiting the activity of MMP-9 to alleviate the degradation of occludin (53). This protective effect was also demonstrated in other studies (54).
Clinically, intermittent NBO is administered to patients with ICH based on the paradox of normal-pressure oxygen, similar to HBO. The normobaric oxygen paradox refers to the relative hypoxic response of the body when hyperoxia fades and returns to normal oxygen levels. Hypoxia can trigger cellular cascade reactions and stimulate tissue regeneration without adverse effects (46). After exposure to 30 and 100% NBO for 60 min, the former showed hypoxic stress, whereas the latter showed oxidative stress (55). Under 30% NBO, HIF-1α can be activated by hypoxia stress; however, at 100% NBO, the protective effect of atmospheric oxygen paradox decreased. The activation of Nrf2 and NF-κB and the production of glutathione (GSH) reflected the enhancement of oxidative stress (30). We can use the normobaric oxygen paradox to promote the efficacy of NBO after ICH, but we should also pay attention to avoiding the side effects caused by excessive oxygen concentration.
A clinical study showed that NBO protects neuronal function and improves oxygenation by reducing lactic acid (Lac) and N-acetyl-aspartate (NAA) (56). Henninger et al. (57) found that NBO could reduce cell apoptosis around the ischemic penumbra, provide lasting nerve protection, and prolong the reperfusion time window. NBO has been proven to have excellent efficacy in early stroke. However, some studies have found that NBO combined with n-acetylcysteine (NAC) can exert a more powerful neuroprotective effect, which is similar to HIF-1α, VEGF, and poly ADP-ribose polymerase (PAPR-1), the degradation of tight junction proteins is closely related (58). In addition, NBO can enhance the neuroprotective effects of melatonin (59), minocycline (60), ethanol (61), and other drugs.
In addition to the influence of the animal model induction method mentioned above, NBO treatment should also focus on exposure time and concentration. Zhou et al. (24) found that oxygen therapy was effective in treating ICH only if administered within 30 min of ICH. However, this experiment has some limitations. Different oxygen concentrations and pressures produced different effects. No hierarchical surveys were conducted in this experiment. Long-term exposure to inappropriate concentrations of NBO produces excessive oxygen free radicals, increases lipid peroxidation levels, and damages mitochondrial function. Oxygen toxicity can ultimately lead to adverse reactions such as hyperoxic acute lung injury (62). Therefore, NBO with an oxygen concentration of 41%–90% provides intermittent treatment to avoid adverse reactions and regularly check blood pondus hydrogenii (PH) (63). In addition to vitamins C and E, which can reduce oxidative damage (63), curcumin (64), its analogs (65), and resveratrol (66) can also reduce lung damage, which can be considered if necessary (67). In addition, it should be noted that hyperoxia therapy is not recommended for severely ill patients (67). It should also be noted that NBO is mainly administered through tracheal intubation, and long-term tracheal intubation will inevitably cause damage to the patient’s throat (68) and even bring risks to patients with severe laryngeal edema (69). This suggests that we should develop NBO treatment time according to the patient’s condition, or alternate NBO and HBO treatment as a new direction for future exploration.
Under normal physiological conditions, HIF-1α is almost not expressed. However, when the tissue is hypoxic, the E3 ubiquitin ligase is recruited by the tumor suppressor protein von hippel–lindau (VHL), ubiquitination of this pathway is inhibited (70), and asparagine hydroxylase factor (FIH) binds to the hypoxia response element (HRES) in the nucleus and increases HIF transcriptional activity (71). The body is in a state of hypoxia after ICH, so HIF-1α shows a high expression level (72); at this time, HIF-1α can up-regulate the levels of pro-apoptotic gene BNIP3 and Beclin1 through the HIF-1α/BNIP3/Beclin1 pathway and promote mitophagy (73). Ostrowski et al. (74) exposed mice 1 h after subarachnoid hemorrhage (SAH) to 2.8 ATA HBO for 2 h. After 24 h, HIF-1α was significantly increased in the untreated SAH group and significantly increased in the HBO-treated group; at the same time, the BNIP3 level in the HBO group decreased to the level of the sham operation group. Although the brain needs BNIP3 to remove necrotic cells and promote apoptosis after ICH, mitophagy is a double-edged sword beneficial for clearing excess ROS, damaging mitochondria, and protecting neurological function after ICH (75, 76). However, excessive autophagy activates NADPH oxidase, producing excessive ROS, engulfing normal cells, and aggravating damage (77). Therefore, after ICH, timely administration of medical oxygen is essential, and monitoring the pressure, concentration, and exposure time to medical oxygen is crucial to avoid adverse injuries.
In brain tissue with focal ischemia, HIF transcriptional activity is enhanced after HBO pretreatment, the expression of its downstream target gene EPO is increased, and the two are regulated positively (28). In addition, the neuroprotective functions of EPO have been widely reported in both clinical and experimental studies. Fernando et al. found that when rats with middle cerebral artery occlusion (MCAO) received exogenous EPO, the apoptosis marker anti-cleaved caspase-3 (CC3) decreased significantly, and the cell proliferation marker Ki67 increased more than in the control group. In clinical trials on neonatal stroke, EPO was found to mobilize the proliferative activity of neurons and oligodendrocytes in the early stages of stroke, inhibit astrocyte generation, and play a neuro-protective role (78). Xiong et al. (79) also found that early EPO treatment after traumatic brain injury (TBI) can significantly restore sensorimotor and spatial learning functions. EPO activates a series of pathways by binding to the homodimer EPOR, leading to autophosphorylation of Janus kinase 2 (JAK2), including signal transducer and activator of transcription 5 (STAT-5), phosphatidylinositol 3-kinase (PI3K)/AKT and SHC (SH2 adapter protein C)/mitogen-activated protein kinase (MAPK) (80), thereby playing an anti-apoptotic and neuroprotective role (78, 81). Among them, the JAK/STAT pathway is the most commonly used pathway to provide EPO-induced neuroprotective effects. Phosphorylated JAK2 is involved in the phosphorylation of tyrosine residues in EPOR cytoplasm; these residues can bind to the SH2 domain of STAT5; when brain trauma occurs, STAT5 activity can be inhibited by negative feedback factors such as SOCS-3 activated by proinflammatory cytokines, thereby preventing EPO’s intracellular signal transduction and weakening EPO’s neuroprotective effect (82); this pathway can be blocked by JAK2 inhibitors (83). Zhang Feng et al. (83) found that the phosphorylation of STAT5 was enhanced under the induction of EPO, and the expression of its downstream target genes B-cell lymphoma-extra-large (BCL-xL) and X-linked inhibitor of apoptosis (XIAP) also increased, these two target genes are one of the genes that inhibit the mitochondrial apoptosis pathway.
HIF regulates multiple target genes, including vascular endothelial growth factor (VEGF). During hypoxia, HIF transcriptional activity increases, and VEGF expression is upregulated. VEGF plays a major role in early embryonic development and angiogenesis (84). It can promote angiogenesis and endothelial cell migration and proliferation (85). Under hypoxia, the transcriptional activity of HIF increased and the expression of VEGF was up-regulated. Repeated intermittent hyperoxia treatment after ICH can increase the expression of HIF, VEGF, and endothelial progenitor cells (EPC), increase the number of new blood vessels and cerebral blood flow in the damaged brain tissue, and improve the neuroinflammatory response and damaged cells due to rich blood supply and oxygen supply (23, 27). Among them, VEGF can promote the extracellular signal-regulated kinase (ERK) and PI3k/AKT pathways by activating vascular endothelial growth factor receptor 2 (VEGF-R2) (86). Jing et al. found that when the injured blood vessels were exposed to 30% NBO, the levels of ERK and protein kinase B (AKT) were significantly lower than those in the control group receiving 21% oxygen, indicating that hyperoxia inhibited the phosphorylation of ERK and PI3K; at the same time, the proliferation of smooth muscle cells (SMC) in injured vascular smooth muscle cells in the 30% NBO group was significantly inhibited, which was similar to the changes of ERK and AKT levels in the same group (87). SMC is the main cell type in vascular walls, being an important cell type involved in vascular remodeling and blood distribution control (88), which has positive significance for the prognosis of ICH. Notably, EPO can up-regulate the production of VEGF through the PI3K/AKT and ERK1/2 pathways and promote the secretion of VEGF-R2 by neural progenitor cells, which can synergistically promote neurovascular remodeling and neurobehavioral improvement (89, 90).
HIF-1α can improve the oxygen metabolism and mitochondrial function of hypoxic cells after ICH and promote tissue to overcome pathological hypoxic injury by regulating oxygenation (71, 91). When the tissue is anoxic, the mitochondrial respiratory function is impaired, resulting in the aggregation of the reducing equivalent at cytochrome c oxidase (COX) rather than oxygen, so the electrons prematurely react with the molecular oxygen in complexes 1 and 3 to generate superoxide (O2-) (92). O2- is a kind of ROS that is closely related to inflammatory reactions and is not conducive to the prognosis of ICH (93). However, HIF-1α expression increases under hypoxia, and COX4-1 in normoxia is degraded by mitochondrial protease LON, which activates COX4-2 production. The latter can increase the levels of H2O2 and ATP and improve tissue oxygenation and electron respiratory chain activity (94). In addition, HIF-1α can enhance glycolysis and reduce mitochondrial oxygen consumption to adapt to a hypoxic environment and avoid excessive ROS production (95). EPO regulates the production of erythroferrone (ERFE) by erythroblasts, inhibits hepcidin activity, enhances iron absorption and storage, promotes hematopoietic function (96), and relieves iron overload (96). Thus, the oxidative stress caused by iron overload can be improved (34). (The details are shown in Figure 2.)
In order to avoid oxygen toxicity to the greatest extent and improve efficacy, HBO exposure for 1 h under 2-3ATA is usually used clinically (36). Some scholars also believe that the use of 2.4 ATA HBO treatment for 60–90 min and activation of the air brake program every 20–30 min can bring positive results (45). Lan et al. (39) suggested that a single exposure to 2.5 ATA HBO for 1 h, once a day for 30 days after ICH can significantly improve NIHSS. Zhou et al. (24) found that giving 3ATA HBO 1 h at the very early stage (within 30 min) after ICH could also improve cerebral microcirculation and reduce cerebral edema. However, Qin et al. (97) found that under the same 3 ATA HBO, a single exposure of 1 h can significantly reduce the volume of PHE at 24 h, while the treatment of 3 times within 24 and 1 h each time cannot reduce the volume of PHE at 72 h, and even increase the toxicity of iron. This suggests that we should not only pay attention to the pressure range and interval time of HBO, but also the number of treatments.
For the safe concentration of NBO, the clinical recommendation is 41%–90% (63). Fratantonio et al. (30) though 100% of NBO can also promote the increase of HIF-1α, the excessive concentration will promote the oxidative stress response, which will make the body spend more time to restore balance. Zhou et al. (24) found that there was no significant difference between the effect of NBO treatment and HBO treatment in the very early stage (within 30 min) after ICH.
This article elaborates on the hypoxic pathological process of ICH and the molecular mechanisms by which HBO and NBO may be effective against ICH. Combined with the latest research, we draw the following conclusions. (i) High-concentration oxygen therapy can damage and protect the brain after ICH and plays an important and positive role in the outcome; (ii) Understanding the duration, concentration, pressure, and treatment interval of patients with ICH and exposure to hyperoxia can effectively avoid hyperoxia toxicity. (iii) There may be a specific time window for hyperoxia therapy following ICH. Hyperoxic therapy after ICH can reduce cerebral edema and oxidative stress, reduce ICP, increase CBF and cerebral oxygenation, protect neurological function, and improve the prognosis of patients with ICH. We recommend intermittent administration of high-concentration oxygen therapy to avoid oxygen toxicity and improve efficacy. We believe that the air brake is activated every 20–30 min within 60–90 min of HBO at 2.4 ATA (46) or NBO with an oxygen concentration of 41%–90% under normal pressure (63). There was no significant difference in the efficacy of NBO and HBO in the very early period after ICH, and the earlier oxygen was administered, the better the efficacy. When giving patients high-concentration oxygen therapy for the first time after the onset of the disease, NBO is the preferred ICH treatment. This article summarizes three directions that need to be studied in the future: (i) Defining the interval duration, concentration, and pressure of hyperoxic therapy after ICH to maximize efficacy. (ii) Is there a more precise time window for hyperoxia treatment after ICH onset? and (iii) Is there an outbreak of oxygen toxicity during long-term treatment?
Regarding long-term effects, HBO appears more effective than NBO regarding brain metabolism (98). Perhaps combined therapy can be used to maximize the advantages of hyperoxia therapy. Studies have found that, in TBI, the efficacy of HBO + NBO as a single treatment is more significant than that of the two treatments alone. Oxygen toxicity markers were significantly reduced, cerebral microcirculation and mitochondrial function were improved, ICP and mortality were reduced, and prognosis was better (99). These findings serve as a significant reference point for the treatment of ICH. In the future, we will explore the mechanism of combined HBO + NBO therapy in ICH and how to select a treatment plan to achieve the best effect of synergistic therapy.
HZ: Conceptualization, Data curation, Formal analysis, Writing – original draft. DZ: Conceptualization, Data curation, Formal analysis, Writing – review & editing. XY: Conceptualization, Data curation, Formal analysis, Writing – review & editing. WZ: Conceptualization, Data curation, Formal analysis, Writing – review & editing. MW: Conceptualization, Data curation, Formal analysis, Writing – review & editing. ZC: Conceptualization, Data curation, Formal analysis, Writing – review & editing.
The author(s) declare that financial support was received for the research, authorship, and/or publication of this article. This study was supported partially by the National Natural Science Foundation of China (81960221 and 82260249 to XY), the National Science and Technology Fundamental Resource Investigation Program of China (2018FY100903 to XY), Jiangxi Provincial Health Commission Science and Technology Plan project (202311506 to ZC), Jiangxi Provincial Administration of Traditional Chinese Medicine science and technology plan project (2022A322 to ZC), Key Projects of Jiangxi Provincial Department of Education (GJJ2201902 to ZC), and Youth Foundation of Natural Science Foundation of Jiangxi Province (20224BAB216045 to ZC).
The authors thank all our colleagues who participated in this study for their cooperation. We sincerely thank the teachers and students from the Jiujiang Precision Medicine Research Center—ZC, Weixin Zhou, MW, WZ, and Qinghua Huang, and teacher DZ from the Third Affiliated Hospital of Wenzhou Medical University—for their guidance and help.
The authors declare that the research was conducted in the absence of any commercial or financial relationships that could be construed as a potential conflict of interest.
All claims expressed in this article are solely those of the authors and do not necessarily represent those of their affiliated organizations, or those of the publisher, the editors and the reviewers. Any product that may be evaluated in this article, or claim that may be made by its manufacturer, is not guaranteed or endorsed by the publisher.
1. Ren, H, Han, R, Chen, X, Liu, X, Wan, J, Wang, L, et al. Potential therapeutic targets for intracerebral hemorrhage-associated inflammation: an update. J Cereb Blood Flow Metab. (2020) 40:1752–68. doi: 10.1177/0271678X20923551
2. Keep, RF, Hua, Y, and Xi, G. Intracerebral haemorrhage: mechanisms of injury and therapeutic targets. Lancet Neurol. (2012) 11:720–31. doi: 10.1016/S1474-4422(12)70104-7
3. Jafari, M, Di Napoli, M, Datta, YH, Bershad, EM, and Divani, AA. The role of serum calcium level in intracerebral hemorrhage hematoma expansion: is there any? Neurocrit Care. (2019) 31:188–95. doi: 10.1007/s12028-018-0564-2
4. Belur, PK, Chang, JJ, He, S, Emanuel, BA, and Mack, WJ. Emerging experimental therapies for intracerebral hemorrhage: targeting mechanisms of secondary brain injury. Neurosurg Focus. (2013) 34:E9. doi: 10.3171/2013.2.FOCUS1317
5. Urday, S, Kimberly, WT, Beslow, LA, Vortmeyer, AO, Selim, MH, Rosand, J, et al. Targeting secondary injury in intracerebral haemorrhage—perihaematomal oedema. Nat Rev Neurol. (2015) 11:111–22. doi: 10.1038/nrneurol.2014.264
6. Bodmer, D, Vaughan, KA, Zacharia, BE, Hickman, ZL, and Connolly, ES. The molecular mechanisms that promote edema after intracerebral hemorrhage. Transl Stroke Res. (2012) 3:52–61. doi: 10.1007/s12975-012-0162-0
7. Xiao, M, Xiao, ZJ, Yang, B, Lan, Z, and Fang, F. Blood-brain barrier: more contributor to disruption of central nervous system homeostasis than victim in neurological disorders. Front Neurosci. (2020) 14:764. doi: 10.3389/fnins.2020.00764
8. Xia, F, Keep, RF, Ye, F, Holste, KG, Wan, S, Xi, G, et al. The fate of erythrocytes after cerebral hemorrhage. Transl Stroke Res. (2022) 13:655–64. doi: 10.1007/s12975-021-00980-8
9. Castro, P, Azevedo, E, and Sorond, F. Cerebral autoregulation in stroke. Curr Atheroscler Rep. (2018) 20:37. doi: 10.1007/s11883-018-0739-5
10. Chazalviel, L, David, HN, Haelewyn, B, Blatteau, JE, Vallée, N, Risso, JJ, et al. The underestimated effect of normobaric hyperoxia on cerebral blood flow and its relationship to neuroprotection. Brain. (2016) 139:e62. doi: 10.1093/brain/aww178
11. Buletko, AB, Thacker, T, Cho, SM, Mathew, J, Thompson, NR, Organek, N, et al. Cerebral ischemia and deterioration with lower blood pressure target in intracerebral hemorrhage. Neurology. (2018) 91:e1058–66. doi: 10.1212/WNL.0000000000006156
12. Qureshi, AI, Palesch, YY, and Suarez, JI. Intensive blood-pressure lowering in cerebral hemorrhage. N Engl J Med. (2016) 375:e48. doi: 10.1056/NEJMc1613117
13. Zhou, D, Ding, J, Asmaro, K, Pan, L, Ya, J, Yang, Q, et al. Clinical characteristics and neuroimaging findings in internal jugular venous outflow disturbance. Thromb Haemost. (2019) 119:308–18. doi: 10.1055/s-0038-1676815
14. Feng, H, Jin, Z, He, W, and Zhao, X. Cerebral venous outflow participates in perihematomal edema after spontaneous intracerebral hemorrhage: a cross-sectional study. Medicine. (2018) 97:e12034. doi: 10.1097/MD.0000000000012034
15. Feng, H, Zhang, H, He, W, Zhou, J, and Zhao, X. Jugular venous reflux is associated with perihematomal edema after intracerebral hemorrhage. Biomed Res Int. (2017) 2017:7514639. doi: 10.1155/2017/7514639
16. Murthy, SB, Cho, SM, Gupta, A, Shoamanesh, A, Navi, BB, Avadhani, R, et al. A pooled analysis of diffusion-weighted imaging lesions in patients with acute intracerebral hemorrhage. JAMA Neurol. (2020) 77:1390–7. doi: 10.1001/jamaneurol.2020.2349
17. Zhou, J, Zhang, H, Gao, P, Lin, Y, and Li, X. Assessment of perihematomal hypoperfusion injury in subacute and chronic intracerebral hemorrhage by CT perfusion imaging. Neurol Res. (2010) 32:642–9. doi: 10.1179/016164109X12445616596328
18. Jones, MW, Brett, K, Han, N, Cooper, JS, and Wyatt, HA. Hyperbaric physics. Treasure Island, (FL): StatPearls Publishing (2024).
19. Calvert, JW, Cahill, J, and Zhang, JH. Hyperbaric oxygen and cerebral physiology. Neurol Res. (2007) 29:132–41. doi: 10.1179/016164107X174156
20. Zhai, WW, Sun, L, Yu, ZQ, and Chen, G. Hyperbaric oxygen therapy in experimental and clinical stroke. Med Gas Res. (2016) 6:111–8. doi: 10.4103/2045-9912.184721
21. Yamamoto, N, Takada, R, Maeda, T, Yoshii, T, Okawa, A, and Yagishita, K. Microcirculation and tissue oxygenation in the head and limbs during hyperbaric oxygen treatment. Diving Hyperb Med. (2021) 51:338–44. doi: 10.28920/dhm51.4.338-344
22. Xu, Q, Fan, SB, Wan, YL, Liu, XL, and Wang, L. The potential long-term neurological improvement of early hyperbaric oxygen therapy on hemorrhagic stroke in the diabetics. Diabetes Res Clin Pract. (2018) 138:75–80. doi: 10.1016/j.diabres.2018.01.017
23. Peng, ZR, Yang, AL, and Yang, QD. The effect of hyperbaric oxygen on intracephalic angiogenesis in rats with intracerebral hemorrhage. J Neurol Sci. (2014) 342:114–23. doi: 10.1016/j.jns.2014.04.037
24. Zhou, W, Marinescu, M, and Veltkamp, R. Only very early oxygen therapy attenuates posthemorrhagic edema formation and blood-brain barrier disruption in murine intracerebral hemorrhage. Neurocrit Care. (2015) 22:121–32. doi: 10.1007/s12028-014-0013-9
25. Yang, L, Tang, J, Chen, Q, Jiang, B, Zhang, B, Tao, Y, et al. Hyperbaric oxygen preconditioning attenuates neuroinflammation after intracerebral hemorrhage in rats by regulating microglia characteristics. Brain Res. (2015) 1627:21–30. doi: 10.1016/j.brainres.2015.08.011
26. Lou, M, Zhang, H, Wang, J, Wen, SQ, Tang, ZQ, Chen, YZ, et al. Hyperbaric oxygen treatment attenuated the decrease in regional glucose metabolism of rats subjected to focal cerebral ischemia: a high resolution positron emission tomography study. Neuroscience. (2007) 146:555–61. doi: 10.1016/j.neuroscience.2007.01.046
27. Hsu, SL, Yin, TC, Shao, PL, Chen, KH, Wu, RW, Chen, CC, et al. Hyperbaric oxygen facilitates the effect of endothelial progenitor cell therapy on improving outcome of rat critical limb ischemia. Am J Transl Res. (2019) 11:1948–64.
28. Gu, GJ, Li, YP, Peng, ZY, Xu, JJ, Kang, ZM, Xu, WG, et al. Mechanism of ischemic tolerance induced by hyperbaric oxygen preconditioning involves upregulation of hypoxia-inducible factor-1alpha and erythropoietin in rats. J Appl Physiol. (2008) 104:1185–91. doi: 10.1152/japplphysiol.00323.2007
29. Yang, D, Ma, L, Wang, P, Yang, D, Zhang, Y, Zhao, X, et al. Normobaric oxygen inhibits AQP4 and NHE1 expression in experimental focal ischemic stroke. Int J Mol Med. (2019) 43:1193–202. doi: 10.3892/ijmm.2018.4037
30. Fratantonio, D, Virgili, F, Zucchi, A, Lambrechts, K, Latronico, T, Lafère, P, et al. Increasing oxygen partial pressures induce a distinct transcriptional response in human PBMC: a pilot study on the “normobaric oxygen paradox”. Int J Mol Sci. (2021) 22:458. doi: 10.3390/ijms22010458
31. Gómez-Lado, N, López-Arias, E, Iglesias-Rey, R, Díaz-Platas, L, Medín-Aguerre, S, Fernández-Ferreiro, A, et al. [18F]-FMISO PET/MRI imaging shows ischemic tissue around hematoma in intracerebral hemorrhage. Mol Pharm. (2020) 17:4667–75. doi: 10.1021/acs.molpharmaceut.0c00932
32. Ostrowski, RP, Stępień, K, Pucko, E, and Matyja, E. The efficacy of hyperbaric oxygen in hemorrhagic stroke: experimental and clinical implications. Arch Med Sci. (2017) 5:1217–23. doi: 10.5114/aoms.2017.65081
33. Zhang, S, An, Q, Wang, T, Gao, S, and Zhou, G. Autophagy- and MMP-2/9-mediated reduction and redistribution of ZO-1 contribute to hyperglycemia-increased blood-brain barrier permeability during early reperfusion in stroke. Neuroscience. (2018) 377:126–37. doi: 10.1016/j.neuroscience.2018.02.035
34. Wan, J, Ren, H, and Wang, J. Iron toxicity, lipid peroxidation and ferroptosis after intracerebral haemorrhage. Stroke Vasc Neurol. (2019) 4:93–5. doi: 10.1136/svn-2018-000205
35. Ferretti, G, Bacchetti, T, Masciangelo, S, Nanetti, L, Mazzanti, L, Silvestrini, M, et al. Lipid peroxidation in stroke patients. Clin Chem Lab Med. (2008) 46:113–7. doi: 10.1515/CCLM.2008.011
36. Schottlender, N, Gottfried, I, and Ashery, U. Hyperbaric oxygen treatment: effects on mitochondrial function and oxidative stress. Biomol Ther. (2021) 11:1827. doi: 10.3390/biom11121827
37. Huang, M, Wu, Q, and Jiang, ZH. Epigenetic alterations under oxidative stress in stem cells. Oxidative Med Cell Longev. (2022) 2022:6439097. doi: 10.1155/2022/6439097
38. Assavarittirong, C, Samborski, W, and Grygiel-Górniak, B. Oxidative stress in fibromyalgia: from pathology to treatment. Oxidative Med Cell Longev. (2022) 2022:1–11. doi: 10.1155/2022/1582432
39. Lan, X, Han, X, Li, Q, Yang, QW, and Wang, J. Modulators of microglial activation and polarization after intracerebral haemorrhage. Nat Rev Neurol. (2017) 13:420–33. doi: 10.1038/nrneurol.2017.69
40. Cozene, B, Sadanandan, N, Gonzales-Portillo, B, Saft, M, Cho, J, Park, YJ, et al. An extra breath of fresh air: hyperbaric oxygenation as a stroke therapeutic. Biomol Ther. (2020) 10:1279. doi: 10.3390/biom10091279
41. Cui, HJ, He, HY, Yang, AL, Zhou, HJ, Tang, T, and Luo, JK. Hyperbaric oxygen for experimental intracerebral haemorrhage: systematic review and stratified meta-analysis. Brain Inj. (2017) 31:456–65. doi: 10.1080/02699052.2017.1279752
42. Thiex, R, and Tsirka, SE. Brain edema after intracerebral hemorrhage: mechanisms, treatment options, management strategies, and operative indications. Neurosurg Focus. (2007) 22:E6:1–7. doi: 10.3171/foc.2007.22.5.7
43. Simsek, K, Ozler, M, Yildirim, AO, Sadir, S, Demirbas, S, Oztosun, M, et al. Evaluation of the oxidative effect of long-term repetitive hyperbaric oxygen exposures on different brain regions of rats. ScientificWorldJournal. (2012) 2012:849183. doi: 10.1100/2012/849183
44. Thomson, L, and Paton, J. Oxygen toxicity. Paediatr Respir Rev. (2014) 15:120–3. doi: 10.1016/j.prrv.2014.03.003
45. Oter, S, Korkmaz, A, Topal, T, Ozcan, O, Sadir, S, Ozler, M, et al. Correlation between hyperbaric oxygen exposure pressures and oxidative parameters in rat lung, brain, and erythrocytes. Clin Biochem. (2005) 38:706–11. doi: 10.1016/j.clinbiochem.2005.04.005
46. Hadanny, A, and Efrati, S. The hyperoxic-hypoxic paradox. Biomol Ther. (2020) 10:958. doi: 10.3390/biom10060958
47. McCormick, JG, Houle, TT, Saltzman, HA, Whaley, RC, and Roy, RC. Treatment of acute stroke with hyperbaric oxygen: time window for efficacy. Undersea Hyperb Med. (2011) 38:321–34.
48. Mijajlovic, MD, Aleksic, V, Milosevic, N, and Bornstein, NM. Hyperbaric oxygen therapy in acute stroke: is it time for Justitia to open her eyes? Neurol Sci. (2020) 41:1381–90. doi: 10.1007/s10072-020-04241-8
49. Chen, Z, Ding, J, Wu, X, Bao, B, Cao, X, Wu, X, et al. Safety and efficacy of normobaric oxygenation on rescuing acute intracerebral hemorrhage-mediated brain damage-a protocol of randomized controlled trial. Trials. (2021) 22:93. doi: 10.1186/s13063-021-05048-4
50. You, P, Lin, M, Li, K, Ye, X, and Zheng, J. Normobaric oxygen therapy inhibits HIF-1α and VEGF expression in perihematoma and reduces neurological function defects. Neuroreport. (2016) 27:329–36. doi: 10.1097/WNR.0000000000000542
51. Nagelhus, EA, and Ottersen, OP. Physiological roles of aquaporin-4 in brain. Physiol Rev. (2013) 93:1543–62. doi: 10.1152/physrev.00011.2013
52. Sadana, P, Coughlin, L, Burke, J, Woods, R, and Mdzinarishvili, A. Anti-edema action of thyroid hormone in MCAO model of ischemic brain stroke: possible association with AQP4 modulation. J Neurol Sci. (2015) 354:37–45. doi: 10.1016/j.jns.2015.04.042
53. Shi, S, Qi, Z, Ma, Q, Pan, R, Timmins, GS, Zhao, Y, et al. Normobaric hyperoxia reduces blood occludin fragments in rats and patients with acute ischemic stroke. Stroke. (2017) 48:2848–54. doi: 10.1161/STROKEAHA.117.017713
54. Michalski, D, Hobohm, C, Weise, C, Pelz, J, Heindl, M, Kamprad, M, et al. Interrelations between blood-brain barrier permeability and matrix metalloproteinases are differently affected by tissue plasminogen activator and hyperoxia in a rat model of embolic stroke. Med Gas Res. (2012) 2:2. doi: 10.1186/2045-9912-2-2
55. Leveque, C, Mrakic-Sposta, S, Lafère, P, Vezzoli, A, Germonpré, P, Beer, A, et al. Oxidative stress response’s kinetics after 60 minutes at different (30% or 100%) normobaric hyperoxia exposures. Int J Mol Sci. (2022) 24:664. doi: 10.3390/ijms24010664
56. Singhal, AB, Ratai, E, Benner, T, Vangel, M, Lee, V, Koroshetz, WJ, et al. Magnetic resonance spectroscopy study of oxygen therapy in ischemic stroke. Stroke. (2007) 38:2851–4. doi: 10.1161/STROKEAHA.107.487280
57. Henninger, N, Bouley, J, Nelligan, JM, Sicard, KM, and Fisher, M. Normobaric hyperoxia delays perfusion/diffusion mismatch evolution, reduces infarct volume, and differentially affects neuronal cell death pathways after suture middle cerebral artery occlusion in rats. J Cereb Blood Flow Metab. (2007) 27:1632–42. doi: 10.1038/sj.jcbfm.9600463
58. Liu, Y, Liu, WC, Sun, Y, Shen, X, Wang, X, Shu, H, et al. Normobaric hyperoxia extends neuro- and vaso-protection of N-acetylcysteine in transient focal ischemia. Mol Neurobiol. (2017) 54:3418–27. doi: 10.1007/s12035-016-9932-0
59. Beker, MC, Caglayan, AB, Kelestemur, T, Caglayan, B, Yalcin, E, Yulug, B, et al. Effects of normobaric oxygen and melatonin on reperfusion injury: role of cerebral microcirculation. Oncotarget. (2015) 6:30604–14. doi: 10.18632/oncotarget.5773
60. Jin, X, Liu, J, Liu, KJ, Rosenberg, GA, Yang, Y, and Liu, W. Normobaric hyperoxia combined with minocycline provides greater neuroprotection than either alone in transient focal cerebral ischemia. Exp Neurol. (2013) 240:9–16. doi: 10.1016/j.expneurol.2012.11.018
61. Geng, X, Fu, P, Ji, X, Peng, C, Fredrickson, V, Sy, C, et al. Synergetic neuroprotection of normobaric oxygenation and ethanol in ischemic stroke through improved oxidative mechanism. Stroke. (2013) 44:1418–25. doi: 10.1161/STROKEAHA.111.000315
62. Alva, R, Mirza, M, Baiton, A, Lazuran, L, Samokysh, L, Bobinski, A, et al. Oxygen toxicity: cellular mechanisms in normobaric hyperoxia. Cell Biol Toxicol. (2023) 39:111–43. doi: 10.1007/s10565-022-09773-7
63. Chen, Z, Ding, Y, Ji, X, and Meng, R. Advances in normobaric hyperoxia brain protection in experimental stroke. Front Neurol. (2020) 11:50. doi: 10.3389/fneur.2020.00050
64. Sakurai, R, Villarreal, P, Husain, S, Liu, J, Sakurai, T, Tou, E, et al. Curcumin protects the developing lung against long-term hyperoxic injury. Am J Physiol Lung Cell Mol Physiol. (2013) 305:L301–11. doi: 10.1152/ajplung.00082.2013
65. Stamenkovska, M, Thaçi, Q, Hadzi-Petrushev, N, Angelovski, M, Bogdanov, J, Reçica, S, et al. Curcumin analogs (B2BrBC and C66) supplementation attenuates airway hyperreactivity and promote airway relaxation in neonatal rats exposed to hyperoxia. Physiol Rep. (2020) 8:e14555. doi: 10.14814/phy2.14555
66. Yang, K, Yang, M, Shen, Y, Kang, L, Zhu, X, Dong, W, et al. Resveratrol attenuates hyperoxia lung injury in neonatal rats by activating SIRT1/PGC-1α signaling pathway. Am J Perinatol. (2024) 41:1039–49. doi: 10.1055/a-1787-3396
67. O’Driscoll, BR, Howard, LS, Earis, J, and Mak, V. British Thoracic Society emergency oxygen guideline group, BTS emergency oxygen guideline development group. BTS guideline for oxygen use in adults in healthcare and emergency settings. Thorax. (2017) 72:ii1–ii90. doi: 10.1136/thoraxjnl-2016-209729
68. Mehel, DM, Özdemir, D, Çelebi, M, Aydemir, S, Akgül, G, and Özgür, A. Classification of laryngeal injury in patients with prolonged intubation and to determine the factors that cause the injury. Am J Otolaryngol. (2020) 41:102432. doi: 10.1016/j.amjoto.2020.102432
69. Wittekamp, BH, van Mook, WN, Tjan, DH, Zwaveling, JH, and Bergmans, DC. Clinical review: post-extubation laryngeal edema and extubation failure in critically ill adult patients. Crit Care. (2009) 13:233. doi: 10.1186/cc8142
70. Pugh, CW, and Ratcliffe, PJ. New horizons in hypoxia signaling pathways. Exp Cell Res. (2017) 356:116–21. doi: 10.1016/j.yexcr.2017.03.008
71. Taylor, CT, and Scholz, CC. The effect of HIF on metabolism and immunity. Nat Rev Nephrol. (2022) 18:573–87. doi: 10.1038/s41581-022-00587-8
72. Durocher, M, Knepp, B, Yee, A, Jickling, G, Rodriguez, F, Ng, K, et al. Molecular correlates of hemorrhage and edema volumes following human intracerebral hemorrhage implicate inflammation, autophagy, mRNA splicing, and T cell receptor signaling. Transl Stroke Res. (2021) 12:754–77. doi: 10.1007/s12975-020-00869-y
73. Zhang, Y, Liu, D, Hu, H, Zhang, P, Xie, R, and Cui, W. HIF-1α/BNIP3 signaling pathway-induced-autophagy plays protective role during myocardial ischemia-reperfusion injury. Biomed Pharmacother. (2019) 120:109464. doi: 10.1016/j.biopha.2019.109464
74. Ostrowski, RP, Colohan, AR, and Zhang, JH. Mechanisms of hyperbaric oxygen-induced neuroprotection in a rat model of subarachnoid hemorrhage. J Cereb Blood Flow Metab. (2005) 25:554–71. doi: 10.1038/sj.jcbfm.9600048
75. Diao, RY, and Gustafsson, ÅB. Mitochondrial quality surveillance: mitophagy in cardiovascular health and disease. Am J Physiol Cell Physiol. (2022) 322:C218–30. doi: 10.1152/ajpcell.00360.2021
76. Li, A, Gao, M, Liu, B, Qin, Y, Chen, L, Liu, H, et al. Mitochondrial autophagy: molecular mechanisms and implications for cardiovascular disease. Cell Death Dis. (2022) 13:444. doi: 10.1038/s41419-022-04906-6
77. Cadenas, S . ROS and redox signaling in myocardial ischemia-reperfusion injury and cardioprotection. Free Radic Biol Med. (2018) 117:76–89. doi: 10.1016/j.freeradbiomed.2018.01.024
78. Gonzalez, FF, Larpthaveesarp, A, McQuillen, P, Derugin, N, Wendland, M, Spadafora, R, et al. Erythropoietin increases neurogenesis and oligodendrogliosis of subventricular zone precursor cells after neonatal stroke. Stroke. (2013) 44:753–8. doi: 10.1161/STROKEAHA.111.000104
79. Xiong, Y, Mahmood, A, Lu, D, Qu, C, Goussev, A, Schallert, T, et al. Role of gender in outcome after traumatic brain injury and therapeutic effect of erythropoietin in mice. Brain Res. (2007) 1185:301–12. doi: 10.1016/j.brainres.2007.09.052
81. Choi, JI, Choi, JW, Shim, KH, Choung, JS, Kim, HJ, Sim, HR, et al. Synergistic effect in neurological recovery via anti-apoptotic Akt signaling in umbilical cord blood and erythropoietin combination therapy for neonatal hypoxic-ischemic brain injury. Int J Mol Sci. (2021) 22:11995. doi: 10.3390/ijms222111995
82. Tao, W, Wen, F, Zhang, H, and Liu, G. The signal transduction mediated by erythropoietin and proinflammatory cytokines in the JAK/STAT pathway in the children with cerebral palsy. Brain and Development. (2009) 31:200–7. doi: 10.1016/j.braindev.2008.06.011
83. Zhang, F, Wang, S, Cao, G, Gao, Y, and Chen, J. Signal transducers and activators of transcription 5 contributes to erythropoietin-mediated neuroprotection against hippocampal neuronal death after transient global cerebral ischemia. Neurobiol Dis. (2007) 25:45–53. doi: 10.1016/j.nbd.2006.08.007
84. Carmeliet, P, Ferreira, V, Breier, G, Pollefeyt, S, Kieckens, L, Gertsenstein, M, et al. Abnormal blood vessel development and lethality in embryos lacking a single VEGF allele. Nature. (1996) 380:435–9. doi: 10.1038/380435a0
85. Melincovici, CS, Boşca, AB, Şuşman, S, Mărginean, M, Mihu, C, Istrate, M, et al. Vascular endothelial growth factor (VEGF) – key factor in normal and pathological angiogenesis. Romanian J Morphol Embryol. (2018) 59:455–67.
86. Adya, R, Tan, BK, Punn, A, Chen, J, and Randeva, HS. Visfatin induces human endothelial VEGF and MMP-2/9 production via MAPK and PI3K/Akt signalling pathways: novel insights into visfatin-induced angiogenesis. Cardiovasc Res. (2008) 78:356–65. doi: 10.1093/cvr/cvm111
87. Wan, J, Lata, C, Santilli, A, Green, D, Roy, S, and Santilli, S. Supplemental oxygen reverses hypoxia-induced smooth muscle cell proliferation by modulating HIF-alpha and VEGF levels in a rabbit arteriovenous fistula model. Ann Vasc Surg. (2014) 28:725–36. doi: 10.1016/j.avsg.2013.10.007
88. Liu, M, and Gomez, D. Smooth muscle cell phenotypic diversity. Arterioscler Thromb Vasc Biol. (2019) 39:1715–23. doi: 10.1161/ATVBAHA.119.312131
89. Xiong, Y, Zhang, Y, Mahmood, A, Meng, Y, Qu, C, and Chopp, M. Erythropoietin mediates neurobehavioral recovery and neurovascular remodeling following traumatic brain injury in rats by increasing expression of vascular endothelial growth factor. Transl Stroke Res. (2011) 2:619–32. doi: 10.1007/s12975-011-0120-2
90. Wang, L, Chopp, M, Gregg, SR, Zhang, RL, Teng, H, Jiang, A, et al. Neural progenitor cells treated with EPO induce angiogenesis through the production of VEGF. J Cereb Blood Flow Metab. (2008) 28:1361–8. doi: 10.1038/jcbfm.2008.32
91. Semenza, GL . Hypoxia-inducible factors in physiology and medicine. Cell. (2012) 148:399–408. doi: 10.1016/j.cell.2012.01.021
92. Cerretelli, P, and Gelfi, C. Energy metabolism in hypoxia: reinterpreting some features of muscle physiology on molecular grounds. Eur J Appl Physiol. (2011) 111:421–32. doi: 10.1007/s00421-010-1399-5
93. Jie, Z, Liu, J, Shu, M, Ying, Y, and Yang, H. Detection strategies for superoxide anion: a review. Talanta. (2022) 236:122892. doi: 10.1016/j.talanta.2021.122892
94. Fukuda, R, Zhang, H, Kim, JW, Shimoda, L, Dang, CV, and Semenza, GL. HIF-1 regulates cytochrome oxidase subunits to optimize efficiency of respiration in hypoxic cells. Cell. (2007) 129:111–22. doi: 10.1016/j.cell.2007.01.047
95. Samanta, D, and Semenza, GL. Metabolic adaptation of cancer and immune cells mediated by hypoxia-inducible factors. Biochim Biophys Acta Rev Cancer. (2018) 1870:15–22. doi: 10.1016/j.bbcan.2018.07.002
96. Kautz, L, Jung, G, Valore, EV, Rivella, S, Nemeth, E, and Ganz, T. Identification of erythroferrone as an erythroid regulator of iron metabolism. Nat Genet. (2014) 46:678–84. doi: 10.1038/ng.2996
97. Qin, Z, Xi, G, Keep, RF, Silbergleit, R, He, Y, and Hua, Y. Hyperbaric oxygen for experimental intracerebral hemorrhage. Acta Neurochir Suppl. (2008) 105:113–7. doi: 10.1007/978-3-211-09469-3_23
98. Rockswold, SB, Rockswold, GL, Zaun, DA, Zhang, X, Cerra, CE, Bergman, TA, et al. A prospective, randomized clinical trial to compare the effect of hyperbaric to normobaric hyperoxia on cerebral metabolism, intracranial pressure, and oxygen toxicity in severe traumatic brain injury. J Neurosurg. (2010) 112:1080–94. doi: 10.3171/2009.7.JNS09363
99. Rockswold, SB, Rockswold, GL, Zaun, DA, and Liu, J. A prospective, randomized phase II clinical trial to evaluate the effect of combined hyperbaric and normobaric hyperoxia on cerebral metabolism, intracranial pressure, oxygen toxicity, and clinical outcome in severe traumatic brain injury. J Neurosurg. (2013) 118:1317–28. doi: 10.3171/2013.2.JNS121468
Keywords: cerebral hemorrhage, secondary ischemia, oxygen metabolic rate, hyperbaric oxygen, normobaric high-concentration oxygen
Citation: Zeng H, Zeng D, Yin X, Zhang W, Wu M and Chen Z (2024) Research progress on high-concentration oxygen therapy after cerebral hemorrhage. Front. Neurol. 15:1410525. doi: 10.3389/fneur.2024.1410525
Received: 01 April 2024; Accepted: 18 July 2024;
Published: 29 July 2024.
Edited by:
Costantino Balestra, Haute École Bruxelles-Brabant (HE2B), BelgiumReviewed by:
Michele Salvagno, Université libre de Bruxelles, BelgiumCopyright © 2024 Zeng, Zeng, Yin, Zhang, Wu and Chen. This is an open-access article distributed under the terms of the Creative Commons Attribution License (CC BY). The use, distribution or reproduction in other forums is permitted, provided the original author(s) and the copyright owner(s) are credited and that the original publication in this journal is cited, in accordance with accepted academic practice. No use, distribution or reproduction is permitted which does not comply with these terms.
*Correspondence: Moxin Wu, bXh3dTE5ODZAamp1LmVkdS5jbg==; Zhiying Chen, enljaGVuampAamp1LmVkdS5jbg==
Disclaimer: All claims expressed in this article are solely those of the authors and do not necessarily represent those of their affiliated organizations, or those of the publisher, the editors and the reviewers. Any product that may be evaluated in this article or claim that may be made by its manufacturer is not guaranteed or endorsed by the publisher.
Research integrity at Frontiers
Learn more about the work of our research integrity team to safeguard the quality of each article we publish.