- 1Department of Neurosurgery, University of Virginia, Charlottesville, VA, United States
- 2Department of Neurological Surgery, Cleveland Clinic Lerner College of Medicine of Case Western Reserve University, Cleveland, OH, United States
- 3Department of Neurosurgery, Rockefeller Neuroscience Institute, West Virginia University, Morgantown, WV, United States
Ultrasound waves were initially used as a diagnostic tool that provided critical insights into several pathological conditions (e.g., gallstones, ascites, pneumothorax, etc.) at the bedside. Over the past decade, advancements in technology have led to the use of ultrasound waves in treating many neurological conditions, such as essential tremor and Parkinson’s disease, with high specificity. The convergence of ultrasound waves at a specific region of interest/target while avoiding surrounding tissue has led to the coined term “focused ultrasound (FUS).” In tumor research, ultrasound technology was initially used as an intraoperative guidance tool for tumor resection. However, in recent years, there has been growing interest in utilizing FUS as a therapeutic tool in the management of brain tumors such as gliomas. This mini-review highlights the current knowledge surrounding using FUS as a treatment modality for gliomas. Furthermore, we discuss the utility of FUS in enhanced drug delivery to the central nervous system (CNS) and highlight promising clinical trials that utilize FUS as a treatment modality for gliomas.
1 Introduction
Central nervous system (CNS) tumors are diverse tumors with distinct and variable intrinsic characteristics. Of this broad category, roughly 28.8% are comprised of tumors with neuroepithelial origin, with an incidence rate of 5.56 per 100,000 persons. Glioblastoma, the most common and one of the most aggressive primary glial tumors has an incidence rate of 2.52 per 100,000 persons (1). In other words, there are over 10,000 new glioblastoma diagnoses in the United States annually. Notably, these tumors are not homogenously distributed among the population; they have higher incidences in specific ethnicity subgroups (2).
Over the past decades, much research has been conducted on the epidemiology of primary CNS tumors, including glial tumors; however, advancement has yet to be made in novel treatment modalities that significantly extend the duration and quality of life in patients suffering from these tumors. Epidemiology and early detection strategies are essential to studying any malignant process; however, due to the aggressive nature of certain subsets of these tumors, including glioblastoma, their clinical impact is limited (3). The current mainstay treatment of glioblastoma includes resection, radiation therapy, and chemotherapy (4, 5). Even with gross total resection and maximal radiation therapy and chemotherapy, survival rates are less than 2 years for most patients. New surgical tools, such as 5-aminolevulinic acid (5-ALA) and volumetric MRI evaluation, can help maximize the extent of surgical resection; however, they still offer relatively low survival benefits (6).
Ultrasound technology has been studied for its ablative effects on the brain since the 1950s. Still, significant limitations, such as the need for a craniotomy window and difficulty with targeting precision, prevented it from gaining mainstream attention until decades later. Elias et al. conducted the first pilot study of focused ultrasound thalamotomy for essential tremors, showing that this was a safe and effective treatment (7). Thereafter, the indications for FUS have continued to grow, including treating Parkinsonian tremors/rigidity or managing many neuropsychiatric conditions (8). New indications for FUS beyond functional neurodegenerative disease conditions remain under study; however, data from several neurosurgical laboratories have begun to provide new evidence that supports the use of FUS in treating CNS glioma.
Additionally, the effect of novel drugs, such as immune checkpoint inhibitors, is significantly dampened secondary to the difficulty of such inhibitors permeating the intact blood–brain barrier. To address this difficulty, blood–brain barrier opening utilizing FUS provides a novel solution that allows novel chemo/immunotherapy to reach their desired targets and more effectively treat CNS tumors. This mini-review article discusses focused ultrasound and its novel applications in treating gliomas. Finally, we highlight promising clinical trials utilizing FUS as a glioma treatment modality.
2 Types and mechanisms of FUS
“Ultrasound” refers to a wave possessing a frequency beyond human hearing, typically f > 20 kHz (9). Ultrasound imaging has been employed for decades to visualize anatomic structures in the medical field—for example, prenatal or transthoracic echocardiogram. However, recent research focuses on the applications of ultrasound for therapeutic purposes. Ultrasound imaging converts electrical energy into mechanical energy by transmitting acoustic waves through a transducer (10). These waves penetrate tissues –such as skin and muscle–to reach molecules within deeper structures. Thereafter, the waves can either be absorbed, scattered, or reflected. When a molecule with a suitable frequency encounters the ultrasound waveform, energy transfer occurs; this concept is known as resonance (11).
Interventional ultrasound for ablative and non-ablative purposes utilizes the same principles governing ultrasound imaging. The waves produced can interfere constructively or destructively. These unique properties underlie the use of ultrasound waves as a diagnostic and therapeutic tool by controlling the interference of ultrasound waves (12). Constructive interference occurs when two or more waves meet, and their peaks or troughs overlap. The resultant wave in constructive interference results in a final wave with a greater amplitude than each individual wave. Conversely, destructive interference occurs when the consequent wave from two or more waves results in an overall final waveform with a smaller amplitude due to waves canceling out (13). In general, FUS aims to allow in-phase waves to converge at the therapeutic target location (12, 14).
FUS is an ultrasound modality that utilizes a concave transducer to converge ultrasound waves into a focused beam. FUS can be categorized as higher-frequency (HIFUS) and low-frequency (LIFUS). HIFUS is often used to ablate specific targets, whereas LIFUS is often used to improve drug delivery to specific targets (14). HIFUS beam intensities are typically between 100–10,000 with the objective of the thermal ablation of tissue, while LIFUS ranges from 0.125–3 W/cm2 (14). The applications of HIFUS for functional neurosurgery have been vast, ranging from ablating the globus pallidus internus in Parkinson’s disease to ablating an epileptic hippocampal focus (15, 16). Delivery of FUS waves is affected by skull fat and bone. FUS wave delivery can be enhanced by applying gasless water between the ultrasound transducer and the scalp. Concurrently, this also minimizes thermal damage. Magnetic resonance-guided imaging (MRgFUS) is critical in identifying/planning the target area and monitoring ablation size. If the target area is heated beyond 56°C for a few seconds, thermo-ablation occurs via protein denaturation and coagulative necrosis. MRgFUS allows monitored and controlled real-time thermometry, which enables immediate evaluation of treatment response (17).
Wave energy is created by passing an electrical current through a transducer to achieve thermoablation with HIFUS. Continuous high-pressure waves are then directed at a small target point, resulting in tissue destruction via a thermal effect. LIFUS ultrasound utilizes injected exogenous microbubbles to open the blood–brain barrier (BBB) by applying ultrasound non-thermal waves that promote microbubble size change/expansion – this is referred to as “stable cavitation.” The perturbations in the size of the microbubbles promote BBB opening (18). Recent studies suggest BBB closure occurs within 48 h after LIFUS without causing injury or harm to the patient (19). Figure 1 demonstrates the proposed mechanisms and application of HIFUS and LIFUS in treating CNS tumors.
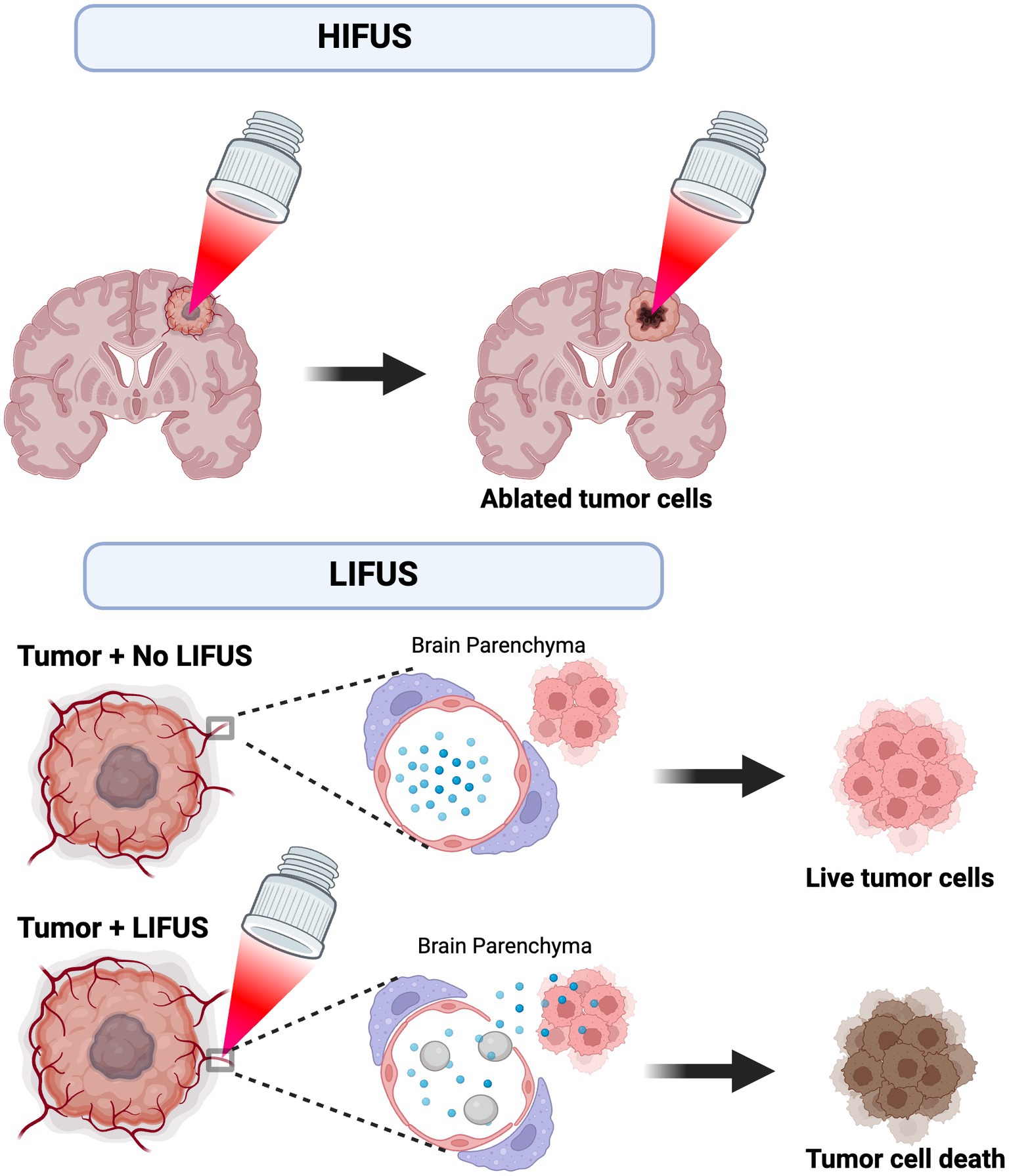
Figure 1. Proposed mechanism and application of HIFUS and LIFUS. HIFUS utilizes non-invasive, high-energy focused ultrasound waves to thermally ablate tumors. Alternatively, LIFUS utilizes lower ultrasound wave energy and microbubbles (grey) to disrupt the blood–brain barrier (BBB) and transiently allow chemotherapy/immunotherapy (blue) to permeate into the brain parenchyma. Ultimately, these treatments reach the tumor cell targets and promote cell death.
3 FUS in the management of glioma
The BBB is a selective dynamic barrier composed of endothelial cells with tight junction proteins, astrocytic end-feet processes, surrounding pericytes, and basal lamina that plays a critical role as a semi-permeable interface between the systemic circulation and brain parenchyma. In health, the BBB is important as it protects the brain from harmful toxins/molecules and inflammatory immune cells - and ultimately maintains cerebral homeostasis by regulating nutrients via modulation of proteins and enzymes found in several cell types of the BBB (20). However, in many disease states, such as CNS tumors, the selectivity of the BBB presents a challenge for therapeutic drugs to reach desired targets. Traditionally, neurosurgeons have mitigated this problem by increasing the dose of the drug – which worsens the adverse effect. Another option is intraventricular or intrathecal drug delivery. Though the effects of these two drug delivery methods strategies are pronounced compared to intra-arterial drug delivery, they do not effectively address the lesion of interest. Furthermore, specificity is significantly reduced (21).
In the early stages of glioma growth, tumor cells initially resemble the BBB, and as they continue to proliferate and progress, they form a new barrier known as the blood–brain tumor barrier (BBTB). The BBTB is distinct from the BBB in that it is characterized by an aberrant distribution of healthy BBB cell types (e.g., pericytes), astrocytic end-feet loss, neuronal dysfunction, increased expression of proteins that encourage drug transport efflux, and a heterogenous permeability between the tumor core and periphery (22–24). The BBB and BBTB limit the entry of chemotherapies and immunotherapies to reach CNS tumor targets (25). Traditionally, hyperosmotic agents such as mannitol have increased BBTB permeability to therapeutic agents when managing glioma and other CNS tumors (26, 27). Though mannitol has provided some success, it has many drawbacks that include limited BBTB opening (15 min), non-selective BBTB opening that can also affect healthy BBB, limited permeability to larger molecules, and systemic effects such as electrolyte abnormalities, injury to kidneys, and worsening of heart failure (28). Numerous strategies, including the use of prodrug formulations, chemical barrier disruption, intraarterial injection, surgical circumvention, thermotherapy, etc., have all been investigated as ways to circumvent the BBB and BBTB but have had limited success (25).
FUS is a safe thermotherapy modality shown to ablate CNS tumors directly or enhance drug delivery across BBB/BBTB for tumor treatment. Other forms of thermotherapies include radiofrequency microwaves, laser-interstitial thermotherapy (LITT), and magnetic disruption (25, 29). These modalities work via induction of intracranial hyperthermia, which causes potentiation of radiotherapy and chemotherapy. Additionally, the resulting hyperthermia demonstrates preferential glioma cell cytotoxicity and increases BBB permeability and tumor cell death through heat-shock protein-mediated cytotoxicity (25, 29, 30). FUS holds the most promise of the various thermotherapy modalities due to its noninvasive nature, efficacy, and ease of performing. Experiments in rat models have demonstrated a higher (38.6%) CSF-to-plasma ratio for temozolomide transferred with focused ultrasound relative to 22% observed in control modalities (25, 31). Like other modalities in this treatment class, FUS thermos-mechanically disrupts the BBB; however, a combination of LIFUS with intravenous injection of albumin-coated octafluropropane microbubbles has been shown to produce only a transient opening of the BBB, thereby significantly decreasing permanent tissue damage (25, 32, 33).
FUS–mediated BBB disruption has been used successfully to deliver numerous agents in animal models including doxorubicin (34, 35), trastuzumab (36), temozolomide (31), interleukin-12 (37), anti-programmed cell death-ligand-1 antibody (38), poly (ethylene glycol) - poly (lactic acid) nanoparticles (39), adeno-associated virus (40), and AP-1 lipoplatin (41). Recent studies have quantified 2000kD as the upper particle size limit for successful FUS-mediated BBB transfer – a size limit encompassing numerous therapeutic agents (25, 42, 43). Beyond the mechanical opening of the BBB/BBTB to allow for enhanced drug delivery, FUS has also been shown to decrease the expression of efflux transporters, reduce junctional proteins, and modify the dispersion of nanoparticles in the extracellular space (44–47). There is growing translational research evidence for successful BBB disruption using FUS, as discussed above, which has led to numerous clinical trials further to assess the role of FUS in glioma treatment, as detailed in Table 1.
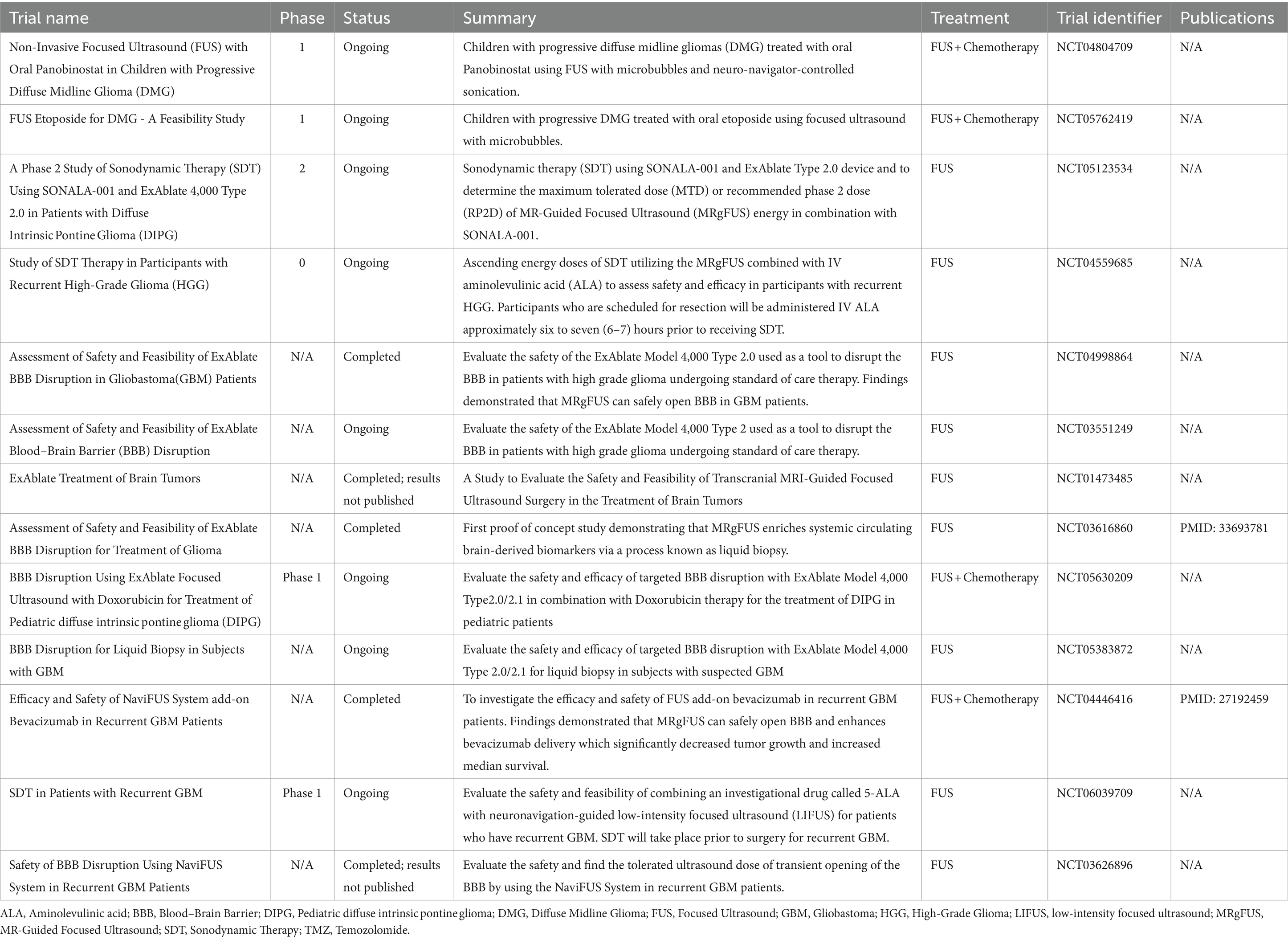
Table 1. Summary of findings for completed and ongoing clinical trials utilizing fus to treat glioma/glioblastoma.
In conjunction with BBB/BBTB opening, FUS use in managing CNS tumors has allowed for better sampling of tumor-specific biomarkers secreted into systemic circulation during FUS-associated BBB/BBTB opening. This process is referred to as liquid biopsy (48). Analyses of these biomarkers may enable early detection, predict recurrence, and assess treatment response. Alternatively, FUS can be used to achieve CNS tumor ablation via hyperthermia. McDannold et al. and Coluccia et al. and others have demonstrated the successful utility of HIFUS ablation in managing CNS tumors (49, 50). Yet, the definitive role of HIFUS ablation in the management of glioma remains to be clinically validated, though preclinical studies have demonstrated success in glioma treatment (14). Interestingly, preclinical data also suggest that the hyperthermia from HIFUS ablation may sensitize glioma cells to radiation therapy (51).
4 Adjuncts to FUS in the treatment of glioma
4.1 Sonodynamic therapy (SDT)
Sonodynamic therapy (SDT), a treatment modality similar in mechanism to photodynamic therapy (PDT), is a promising alternative treatment being investigated for glioma treatment. In PDT, a light-activated photosensitizer generates reactive oxygen species (ROS), facilitating cytotoxic effects on neoplastic cells. While effective, PDT is limited to superficial lesions because of the limited penetration of laser light into brain tissue (52, 53). This challenge is overcome in SDT, which employs a low-intensity ultrasound, offering superior tissue penetrance (54). SDT combines focused ultrasound with sonosensitizers, which sensitize cells to sound-induced destruction, minimizing adverse events and maximizing target responses (48, 55). Examples of sonosensitizers include 5-ALA, ATX-70, and Hypocrellin (56–58). The efficacy of SDT has been shown in studies by Sheehan et al., which demonstrated SDT’s efficacy in rat C6 and human U87 glioma cells by showing FUS and 5-ALA-induced cell death through ROS generation (59). These findings were further validated in other experiments demonstrating that focused ultrasound combined with systemic 5-ALA effectively treated gliomas in rodent models (60–63).
Overcoming the blood–brain barrier (BBB) remains a critical challenge in SDT, as most sonosensitizers cannot cross it. As a result, other studies have investigated the possibility of combining LIFUS with BBB modifiers, such as microbubbles, to increase the permeability of the BBB and improve SDT efficacy (64–66). Sonosensitizers used in Sonodynamic Therapy (SDT) comprise benign molecules that induce cytotoxic effects under an acoustic field (56). Several of these molecules are similar to those used in photodynamic therapy and are usually porphyrin-based or related compounds such as protoporphyrin IX and hematoporphyrin, among others. Emerging evidence suggests these molecules generate ROS upon exposure to ultrasound waves (67). In vitro investigations by Shen et al. demonstrated the efficacy of sinoporphyrin sodium, derived from photofrin II, as a sonosensitizer, showcasing significant antitumor effects on human glioblastoma cell lines (67) Particularly noteworthy was this sonosensitizer’s ability to infiltrate cancer cells and accumulate within mitochondria, thus instigating cytotoxicity via ROS production (67).
It is crucial to highlight that despite their preferential uptake by tumors, these agents exhibit considerable hydrophobicity, which results in ubiquitous distribution (68). However, as postulated by Raspagliesi et al., for cytotoxic effects to manifest in any tissue, three concurrent events must occur: (1) ultrasound administration, (2) sonosensitizer administration, and (3) the presence of a lesion where the latter attains significant concentration. Consequently, our current acceptance of SDT’s non-invasiveness towards normal brain tissue resulted from this concept, which suggests that the accumulation of sonosensitizer in healthy tissue without the other two concurrent events will render it inconsequential in the healthy tissue (69). Thus, the ideal sonosensitizer selection is crucial in SDT and should demonstrate high tumor cell affinity, prolonged neoplasm retention, and minimal impact on healthy brain parenchyma (70–72).
4.2 Histotripsy
Histotripsy is a non-thermal HIFUS technique that presents a promising avenue for mechanical ablation of brain tissue and tumors with precise localization, devoid of thermal effects (73). This technique employs short-duration, high-amplitude ultrasound pulses to induce acoustic cavitation within tissues, which results in inward erosion at tissue-liquid interfaces and liquefaction in dense tissue (74–76). The liquefaction process forms acellular debris, which is then gradually resorbed by the body over several months (77). Histotripsy differs from earlier thermal techniques like shockwave therapy and HIFUS because it produces more precise ablations with well-defined margins, minimizing damage to surrounding healthy tissue (14, 78).
The short duration of histotripsy ultrasound pulses restricts cavitation to the focal zone of interest, preventing extraneous tissue damage and allowing for precisely targeted ablations (79–81). This is achieved by forming dense cavitation “bubble clouds” at the focal point, which generates mechanical shearing forces and stress in the target tissue, causing cell disintegration and extracellular matrix fragmentation within those target tissues (74, 82). Cavitation migration is notably hindered outside the focal region due to insufficient amplitude to sustain dense bubble cloud formation in the off-target sites (83). The ability of histotripsy to produce clear margin lesions with minimal complications in cortical tissue has been demonstrated in detail in porcine models, suggesting its potential in brain tumor treatment (73).
Recent studies have highlighted that the acellular debris resulting from histotripsy-induced liquefaction contains tumor antigens, damage-associated molecular patterns, and heat shock proteins, potentially stimulating a tumor-specific cytotoxic T-cell response (84). Additionally, histotripsy may elicit inflammatory responses involving macrophages and B-cell lymphocytes, as evidenced in melanoma and hepatocellular carcinoma preclinical studies (74). Qu et al. showed that histotripsy in mice with melanoma or hepatocellular carcinoma not only stimulated local tumor infiltration by immune cells but also stimulated inflammation at other tumor sites not targeted by histotripsy (14, 85). While these results are promising, it is important to highlight that this study was not conducted in gliomas. Thus, whether a similar inflammatory response will be replicated in glioma remains to be determined. Further work is needed to delineate the role of histotripsy in glioma treatment.
5 Challenges associated with FUS
The evolving landscape of FUS holds promise for improving therapeutic outcomes through thermal ablation and novel treatment modalities, including focal BBB disruption for drug delivery enhancement. Yet, substantial challenges persist in achieving consistent and clinically meaningful outcomes. Historically, inadequate visual monitoring, thermometric control, and precise focal point determination were predominant challenges faced with FUS utility in managing CNS conditions. Advances in the utility of stereotactic skull frames, MRI-guided imaging, and thermometric monitoring have helped address these challenges (49). Though MRI-guided FUS is advantageous over Ultrasound-guided FUS because it provides a superior resolution, it should be noted that thermal ablation may interfere with MRI resolution. MRI-based acoustic radiation is a novel tool that may be useful in limiting the effects of thermal ablation (86, 87).
Further technical difficulties have been reported with FUS use, such as skull and scalp heterogeneities. These may attenuate US propagation to the target location, impeding the desired temperature for an ablative effect. Utilization of lower frequencies aids in addressing this issue; however, lowering the frequency may also induce tissue damage by cavitation. Furthermore, the translation of findings from animal studies to human clinical trials must be carefully analyzed, mainly since the human skull is thicker and harder than that of rodents. Hence, US wave attenuation is expected to be more significant in humans (87).
While FUS is a promising tool, a recent study investigating the risk of bias in animal studies and non-randomized clinical brain tumor trials showed a high risk of bias, methodological inconsistencies, and significant ethical limitations in animal and human brain tumor studies (88). Given the increasing popularity of FUS use in treating several CNS clinical conditions, it is paramount that a global initiative is established to standardize research methodologies and uphold stringent ethical norms.
6 Conclusion
Glioma/glioblastoma is a life-altering diagnosis for the patient. Significant advancements in developing new therapeutics to treat glioma and glioblastoma have flourished. However, these advancements have been halted by the inability of a vast number of these therapies to cross the BBB/BBTB. FUS serves as an emerging non-invasive treatment modality that could address enhanced drug delivery across BBTB and/or be used in conjunction with radiosurgery or surgical resection to improve outcomes in patients diagnosed with glioma/glioblastoma. While several ongoing clinical trials are exploring the role of FUS in brain tumors (i.e., enhanced drug delivery and tumor ablation), data on FUS use in treating spinal cord tumors is lacking. Further investigation is required to address microbubbles’ type and administration route and the FUS’s short- and long-term impact on the host immune response profile.
Author contributions
DN: Writing – original draft, Writing – review & editing. DO-Y: Writing – original draft, Writing – review & editing. FF: Writing – original draft, Writing – review & editing. WB: Writing – original draft, Writing – review & editing. MM: Writing – original draft, Writing – review & editing.
Funding
The author(s) declare that no financial support was received for the research, authorship, and/or publication of this article.
Conflict of interest
The authors declare that the research was conducted in the absence of any commercial or financial relationships that could be construed as a potential conflict of interest.
Publisher's note
All claims expressed in this article are solely those of the authors and do not necessarily represent those of their affiliated organizations, or those of the publisher, the editors and the reviewers. Any product that may be evaluated in this article, or claim that may be made by its manufacturer, is not guaranteed or endorsed by the publisher.
References
1. Ostrom, QT, Gittleman, H, Liao, P, Vecchione-Koval, T, Wolinsky, Y, Kruchko, C, et al. CBTRUS statistical report: primary brain and other central nervous system tumors diagnosed in the United States in 2010-2014. Neuro-Oncology. (2017) 19:v1–v88. doi: 10.1093/neuonc/nox158
2. Dubrow, R, and Darefsky, AS. Demographic variation in incidence of adult glioma by subtype, United States, 1992-2007. BMC Cancer. (2011) 11:325. doi: 10.1186/1471-2407-11-325
3. Omuro, A, and DeAngelis, LM. Glioblastoma and other malignant gliomas: a clinical review. JAMA. (2013) 310:1842–50. doi: 10.1001/jama.2013.280319
4. Kanderi, T, and Gupta, V. Glioblastoma Multiforme In StatPearls. Stefaan De Smedt editor. (Netherlands: StatPearls Publishing) (2024)
5. Stummer, W, Reulen, HJ, Meinel, T, Pichlmeier, U, Schumacher, W, Tonn, JC, et al. Extent of resection and survival in glioblastoma multiforme: identification of and adjustment for bias. Neurosurgery. (2008) 62:564–76. doi: 10.1227/01.neu.0000317304.31579.17
6. Aiudi, D, Iacoangeli, A, Dobran, M, Polonara, G, Chiapponi, M, Mattioli, A, et al. The prognostic role of volumetric MRI evaluation in the surgical treatment of glioblastoma. J Clin Med. (2024) 13:849. doi: 10.3390/jcm13030849
7. Elias, WJ, Huss, D, Voss, T, Loomba, J, Khaled, M, Zadicario, E, et al. A pilot study of focused ultrasound thalamotomy for essential tremor. N Engl J Med. (2013) 369:640–8. doi: 10.1056/NEJMoa1300962
8. Baek, H, Lockwood, D, Mason, EJ, Obusez, E, Poturalski, M, Rammo, R, et al. Clinical intervention using focused ultrasound (FUS) stimulation of the brain in diverse neurological disorders. Front Neurol. (2022) 13:880814. doi: 10.3389/fneur.2022.880814
9. Darmani, G, Bergmann, TO, Butts Pauly, K, Caskey, CF, de Lecea, L, Fomenko, A, et al. Non-invasive transcranial ultrasound stimulation for neuromodulation. Clin Neurophysiol. (2022) 135:51–73. doi: 10.1016/j.clinph.2021.12.010
10. Siedek, F, Yeo, SY, Heijman, E, Grinstein, O, Bratke, G, Heneweer, C, et al. Magnetic resonance-guided high-intensity focused ultrasound (MR-HIFU): technical background and overview of current clinical applications (part 1). Rofo. (2019) 191:522–30. doi: 10.1055/a-0817-5645
11. Johns, LD . Nonthermal effects of therapeutic ultrasound: the frequency resonance hypothesis. J Athl Train. (2002) 37:293–9.
12. Darrow, DP . Focused ultrasound for neuromodulation. Neurotherapeutics. (2019) 16:88–99. doi: 10.1007/s13311-018-00691-3
13. Canney, MS, Bailey, MR, Crum, LA, Khokhlova, VA, and Sapozhnikov, OA. Acoustic characterization of high intensity focused ultrasound fields: a combined measurement and modeling approach. J Acoust Soc Am. (2008) 124:2406–20. doi: 10.1121/1.2967836
14. Hersh, AM, Bhimreddy, M, Weber-Levine, C, Jiang, K, Alomari, S, Theodore, N, et al. Applications of focused ultrasound for the treatment of glioblastoma: a new frontier. Cancers (Basel). (2022) 14:4920. doi: 10.3390/cancers14194920
15. Lescrauwaet, E, Vonck, K, Sprengers, M, Raedt, R, Klooster, D, Carrette, E, et al. Recent advances in the use of focused ultrasound as a treatment for epilepsy. Front Neurosci. (2022) 16:886584. doi: 10.3389/fnins.2022.886584
16. Moosa, S, Martínez-Fernández, R, Elias, WJ, Del Alamo, M, Eisenberg, HM, and Fishman, PS. The role of high-intensity focused ultrasound as a symptomatic treatment for Parkinson's disease. Mov Disord. (2019) 34:1243–51. doi: 10.1002/mds.27779
17. Jolesz, FA . MRI-guided focused ultrasound surgery. Annu Rev Med. (2009) 60:417–30. doi: 10.1146/annurev.med.60.041707.170303
18. Zhong, YX, Liao, JC, Liu, X, Tian, H, Deng, LR, and Long, L. Low intensity focused ultrasound: a new prospect for the treatment of Parkinson's disease. Ann Med. (2023) 55:2251145. doi: 10.1080/07853890.2023.2251145
19. Rezai, AR, Ranjan, M, Haut, MW, Carpenter, J, D'Haese, PF, Mehta, RI, et al. Focused ultrasound-mediated blood-brain barrier opening in Alzheimer's disease: long-term safety, imaging, and cognitive outcomes. J Neurosurg. (2023) 139:275–83. doi: 10.3171/2022.9.Jns221565
20. Nwafor, DC, Brichacek, AL, Mohammad, AS, Griffith, J, Lucke-Wold, BP, Benkovic, SA, et al. Targeting the blood-brain barrier to prevent Sepsis-associated cognitive impairment. J Cent Nerv Syst Dis. (2019) 11:1179573519840652. doi: 10.1177/1179573519840652
21. Wu, D, Chen, Q, Chen, X, Han, F, Chen, Z, and Wang, Y. The blood-brain barrier: structure, regulation, and drug delivery. Signal Transduct Target Ther. (2023) 8:217. doi: 10.1038/s41392-023-01481-w
22. Abbott, NJ, Patabendige, AA, Dolman, DE, Yusof, SR, and Begley, DJ. Structure and function of the blood-brain barrier. Neurobiol Dis. (2010) 37:13–25. doi: 10.1016/j.nbd.2009.07.030
23. van Tellingen, O, Yetkin-Arik, B, de Gooijer, MC, Wesseling, P, Wurdinger, T, and de Vries, HE. Overcoming the blood-brain tumor barrier for effective glioblastoma treatment. Drug Resist Updat. (2015) 19:1–12. doi: 10.1016/j.drup.2015.02.002
24. Wu, T, and Dai, Y. Tumor microenvironment and therapeutic response. Cancer Lett. (2017) 387:61–8. doi: 10.1016/j.canlet.2016.01.043
25. Hendricks, BK, Cohen-Gadol, AA, and Miller, JC. Novel delivery methods bypassing the blood-brain and blood-tumor barriers. Neurosurg Focus. (2015) 38:E10. doi: 10.3171/2015.1.Focus14767
26. Iwadate, Y, Namba, H, Saegusa, T, and Sueyoshi, K. Intra-arterial mannitol infusion in the chemotherapy for malignant brain tumors. J Neuro-Oncol. (1993) 15:185–93. doi: 10.1007/bf01053940
27. Siegal, T, Rubinstein, R, Bokstein, F, Schwartz, A, Lossos, A, Shalom, E, et al. In vivo assessment of the window of barrier opening after osmotic blood-brain barrier disruption in humans. J Neurosurg. (2000) 92:599–605. doi: 10.3171/jns.2000.92.4.0599
28. Doolittle, ND, Muldoon, LL, Culp, AY, and Neuwelt, EA. Delivery of chemotherapeutics across the blood-brain barrier: challenges and advances. Adv Pharmacol. (2014) 71:203–43. doi: 10.1016/bs.apha.2014.06.002
29. Lee Titsworth, W, Murad, GJ, Hoh, BL, and Rahman, M. Fighting fire with fire: the revival of thermotherapy for gliomas. Anticancer Res. (2014) 34:565–74.
30. Watanabe, M, Tanaka, R, Hondo, H, and Kuroki, M. Effects of antineoplastic agents and hyperthermia on cytotoxicity toward chronically hypoxic glioma cells. Int J Hyperth. (1992) 8:131–8. doi: 10.3109/02656739209052885
31. Wei, KC, Chu, PC, Wang, HY, Huang, CY, Chen, PY, Tsai, HC, et al. Focused ultrasound-induced blood-brain barrier opening to enhance temozolomide delivery for glioblastoma treatment: a preclinical study. PLoS One. (2013) 8:e58995. doi: 10.1371/journal.pone.0058995
32. Hynynen, K, McDannold, N, Vykhodtseva, N, and Jolesz, FA. Noninvasive MR imaging-guided focal opening of the blood-brain barrier in rabbits. Radiology. (2001) 220:640–6. doi: 10.1148/radiol.2202001804
33. Konofagou, EE . Optimization of the ultrasound-induced blood-brain barrier opening. Theranostics. (2012) 2:1223–37. doi: 10.7150/thno.5576
34. Kovacs, Z, Werner, B, Rassi, A, Sass, JO, Martin-Fiori, E, and Bernasconi, M. Prolonged survival upon ultrasound-enhanced doxorubicin delivery in two syngenic glioblastoma mouse models. J Control Release. (2014) 187:74–82. doi: 10.1016/j.jconrel.2014.05.033
35. Treat, LH, McDannold, N, Vykhodtseva, N, Zhang, Y, Tam, K, and Hynynen, K. Targeted delivery of doxorubicin to the rat brain at therapeutic levels using MRI-guided focused ultrasound. Int J Cancer. (2007) 121:901–7. doi: 10.1002/ijc.22732
36. Kinoshita, M, McDannold, N, Jolesz, FA, and Hynynen, K. Noninvasive localized delivery of Herceptin to the mouse brain by MRI-guided focused ultrasound-induced blood-brain barrier disruption. Proc Natl Acad Sci USA. (2006) 103:11719–23. doi: 10.1073/pnas.0604318103
37. Chen, PY, Hsieh, HY, Huang, CY, Lin, CY, Wei, KC, and Liu, HL. Focused ultrasound-induced blood-brain barrier opening to enhance interleukin-12 delivery for brain tumor immunotherapy: a preclinical feasibility study. J Transl Med. (2015) 13:93. doi: 10.1186/s12967-015-0451-y
38. Ye, D, Yuan, J, Yue, Y, Rubin, JB, and Chen, H. Focused ultrasound-enhanced delivery of Intranasally administered anti-programmed cell death-ligand 1 antibody to an intracranial murine glioma model. Pharmaceutics. (2021) 13:190. doi: 10.3390/pharmaceutics13020190
39. Yao, L, Song, Q, Bai, W, Zhang, J, Miao, D, Jiang, M, et al. Facilitated brain delivery of poly (ethylene glycol)-poly (lactic acid) nanoparticles by microbubble-enhanced unfocused ultrasound. Biomaterials. (2014) 35:3384–95. doi: 10.1016/j.biomaterials.2013.12.043
40. Thévenot, E, Jordão, JF, O'Reilly, MA, Markham, K, Weng, YQ, Foust, KD, et al. Targeted delivery of self-complementary adeno-associated virus serotype 9 to the brain, using magnetic resonance imaging-guided focused ultrasound. Hum Gene Ther. (2012) 23:1144–55. doi: 10.1089/hum.2012.013
41. Yang, FY, and Horng, SC. Chemotherapy of glioblastoma by targeted liposomal platinum compounds with focused ultrasound. Annu Int Conf IEEE Eng Med Biol Soc. (2013) 2013:6289–92. doi: 10.1109/embc.2013.6610991
42. Chen, H, and Konofagou, EE. The size of blood-brain barrier opening induced by focused ultrasound is dictated by the acoustic pressure. J Cereb Blood Flow Metab. (2014) 34:1197–204. doi: 10.1038/jcbfm.2014.71
43. Choi, JJ, Wang, S, Tung, YS, Morrison, B 3rd, and Konofagou, EE. Molecules of various pharmacologically-relevant sizes can cross the ultrasound-induced blood-brain barrier opening in vivo. Ultrasound Med Biol. (2010) 36:58–67. doi: 10.1016/j.ultrasmedbio.2009.08.006
44. Bunevicius, A, McDannold, NJ, and Golby, AJ. Focused ultrasound strategies for brain tumor therapy. Oper Neurosurg (Hagerstown). (2020) 19:9–18. doi: 10.1093/ons/opz374
45. Hersh, DS, Anastasiadis, P, Mohammadabadi, A, Nguyen, BA, Guo, S, Winkles, JA, et al. MR-guided transcranial focused ultrasound safely enhances interstitial dispersion of large polymeric nanoparticles in the living brain. PLoS One. (2018) 13:e0192240. doi: 10.1371/journal.pone.0192240
46. Hersh, DS, Nguyen, BA, Dancy, JG, Adapa, AR, Winkles, JA, Woodworth, GF, et al. Pulsed ultrasound expands the extracellular and perivascular spaces of the brain. Brain Res. (2016) 1646:543–50. doi: 10.1016/j.brainres.2016.06.040
47. Jalali, S, Huang, Y, Dumont, DJ, and Hynynen, K. Focused ultrasound-mediated bbb disruption is associated with an increase in activation of AKT: experimental study in rats. BMC Neurol. (2010) 10:114. doi: 10.1186/1471-2377-10-114
48. Roberts, JW, Powlovich, L, Sheybani, N, and LeBlang, S. Focused ultrasound for the treatment of glioblastoma. J Neuro-Oncol. (2022) 157:237–47. doi: 10.1007/s11060-022-03974-0
49. Coluccia, D, Fandino, J, Schwyzer, L, O'Gorman, R, Remonda, L, Anon, J, et al. First noninvasive thermal ablation of a brain tumor with MR-guided focused ultrasound. J Ther Ultrasound. (2014) 2:17. doi: 10.1186/2050-5736-2-17
50. MacDonell, J, Patel, N, Rubino, S, Ghoshal, G, Fischer, G, Burdette, EC, et al. Magnetic resonance-guided interstitial high-intensity focused ultrasound for brain tumor ablation. Neurosurg Focus. (2018) 44:E11. doi: 10.3171/2017.11.Focus17613
51. Man, J, Shoemake, JD, Ma, T, Rizzo, AE, Godley, AR, Wu, Q, et al. Hyperthermia sensitizes glioma stem-like cells to radiation by inhibiting AKT signaling. Cancer Res. (2015) 75:1760–9. doi: 10.1158/0008-5472.Can-14-3621
52. Gong, Z, and Dai, Z. Design and challenges of Sonodynamic therapy system for Cancer Theranostics: from equipment to sensitizers. Adv Sci (Weinh). (2021) 8:2002178. doi: 10.1002/advs.202002178
53. Yoshida, M, Kobayashi, H, Terasaka, S, Endo, S, Yamaguchi, S, Motegi, H, et al. Sonodynamic therapy for malignant glioma using 220-kHz transcranial magnetic resonance imaging-guided focused ultrasound and 5-Aminolevulinic acid. Ultrasound Med Biol. (2019) 45:526–38. doi: 10.1016/j.ultrasmedbio.2018.10.016
54. McHale, AP, Callan, JF, Nomikou, N, Fowley, C, and Callan, B. Sonodynamic therapy: concept, mechanism and application to Cancer treatment. Adv Exp Med Biol. (2016) 880:429–50. doi: 10.1007/978-3-319-22536-4_22
55. Pan, X, Wang, H, Wang, S, Sun, X, Wang, L, Wang, W, et al. Sonodynamic therapy (SDT): a novel strategy for cancer nanotheranostics. Sci China Life Sci. (2018) 61:415–26. doi: 10.1007/s11427-017-9262-x
56. Bonosi, L, Marino, S, Benigno, UE, Musso, S, Buscemi, F, Giardina, K, et al. Sonodynamic therapy and magnetic resonance-guided focused ultrasound: new therapeutic strategy in glioblastoma. J Neuro-Oncol. (2023) 163:219–38. doi: 10.1007/s11060-023-04333-3
57. Wu, SK, Santos, MA, Marcus, SL, and Hynynen, K. MR-guided focused ultrasound facilitates Sonodynamic therapy with 5-Aminolevulinic acid in a rat glioma model. Sci Rep. (2019) 9:10465. doi: 10.1038/s41598-019-46832-2
58. Zhang, C, Wu, J, Liu, W, Zheng, X, Zhang, W, Lee, CS, et al. A novel hypocrellin-based assembly for sonodynamic therapy against glioblastoma. J Mater Chem B. (2021) 10:57–63. doi: 10.1039/d1tb01886h
59. Sheehan, K, Sheehan, D, Sulaiman, M, Padilla, F, Moore, D, Sheehan, J, et al. Investigation of the tumoricidal effects of sonodynamic therapy in malignant glioblastoma brain tumors. J Neuro-Oncol. (2020) 148:9–16. doi: 10.1007/s11060-020-03504-w
60. Anttinen, H, Terho, EO, Myllyla, R, and Savolainen, ER. Two serum markers of collagen biosynthesis as possible indicators of irreversible pulmonary impairment in farmer's lung. Am Rev Respir Dis. (1986) 133:88–93. doi: 10.1164/arrd.1986.133.1.88
61. Jeong, EJ, Seo, SJ, Ahn, YJ, Choi, KH, Kim, KH, and Kim, JK. Sonodynamically induced antitumor effects of 5-aminolevulinic acid and fractionated ultrasound irradiation in an orthotopic rat glioma model. Ultrasound Med Biol. (2012) 38:2143–50. doi: 10.1016/j.ultrasmedbio.2012.07.026
62. Ohmura, T, Fukushima, T, Shibaguchi, H, Yoshizawa, S, Inoue, T, Kuroki, M, et al. Sonodynamic therapy with 5-aminolevulinic acid and focused ultrasound for deep-seated intracranial glioma in rat. Anticancer Res. (2011) 31:2527–33.
63. Song, D, Yue, W, Li, Z, Li, J, Zhao, J, and Zhang, N. Study of the mechanism of sonodynamic therapy in a rat glioma model. Onco Targets Ther. (2014) 7:1801–10. doi: 10.2147/ott.S52426
64. Arvanitis, CD, Vykhodtseva, N, Jolesz, F, Livingstone, M, and McDannold, N. Cavitation-enhanced nonthermal ablation in deep brain targets: feasibility in a large animal model. J Neurosurg. (2016) 124:1450–9. doi: 10.3171/2015.4.Jns142862
65. Jones, RM, McMahon, D, and Hynynen, K. Ultrafast three-dimensional microbubble imaging in vivo predicts tissue damage volume distributions during nonthermal brain ablation. Theranostics. (2020) 10:7211–30. doi: 10.7150/thno.47281
66. McDannold, N, Clement, GT, Black, P, Jolesz, F, and Hynynen, K. Transcranial magnetic resonance imaging- guided focused ultrasound surgery of brain tumors: initial findings in 3 patients. Neurosurgery. (2010) 66:323–32; discussion 332. doi: 10.1227/01.Neu.0000360379.95800.2f
67. Shen, Y, Chen, Y, Huang, Y, Zeng, X, Huang, L, Diao, X, et al. An in vitro study on the antitumor effect of sonodynamic therapy using sinoporphyrin sodium on human glioblastoma cells. Ultrasonics. (2021) 110:106272. doi: 10.1016/j.ultras.2020.106272
68. Liu, Q, Wang, X, Wang, P, Xiao, L, and Hao, Q. Comparison between sonodynamic effect with protoporphyrin IX and hematoporphyrin on sarcoma 180. Cancer Chemother Pharmacol. (2007) 60:671–80. doi: 10.1007/s00280-006-0413-4
69. Raspagliesi, L, D'Ammando, A, Gionso, M, Sheybani, ND, Lopes, MB, Moore, D, et al. Intracranial Sonodynamic therapy with 5-Aminolevulinic acid and sodium fluorescein: safety study in a porcine model. Front Oncol. (2021) 11:679989. doi: 10.3389/fonc.2021.679989
70. Nomikou, N, Li, YS, and McHale, AP. Ultrasound-enhanced drug dispersion through solid tumours and its possible role in aiding ultrasound-targeted cancer chemotherapy. Cancer Lett. (2010) 288:94–8. doi: 10.1016/j.canlet.2009.06.028
71. Son, S, Kim, JH, Wang, X, Zhang, C, Yoon, SA, Shin, J, et al. Multifunctional sonosensitizers in sonodynamic cancer therapy. Chem Soc Rev. (2020) 49:3244–61. doi: 10.1039/c9cs00648f
72. Wang, C, Tian, Y, Wu, B, and Cheng, W. Recent Progress toward imaging application of multifunction Sonosensitizers in Sonodynamic therapy. Int J Nanomedicine. (2022) 17:3511–29. doi: 10.2147/ijn.S370767
73. Sukovich, JR, Cain, CA, Pandey, AS, Chaudhary, N, Camelo-Piragua, S, Allen, SP, et al. In vivo histotripsy brain treatment. J Neurosurg. (2018) 131:1331–8. doi: 10.3171/2018.4.Jns172652
74. Khokhlova, VA, Fowlkes, JB, Roberts, WW, Schade, GR, Xu, Z, Khokhlova, TD, et al. Histotripsy methods in mechanical disintegration of tissue: towards clinical applications. Int J Hyperth. (2015) 31:145–62. doi: 10.3109/02656736.2015.1007538
75. Xu, Z, Ludomirsky, A, Eun, LY, Hall, TL, Tran, BC, Fowlkes, JB, et al. Controlled ultrasound tissue erosion. IEEE Trans Ultrason Ferroelectr Freq Control. (2004) 51:726–36. doi: 10.1109/tuffc.2004.1308731
76. Xu, Z, Owens, G, Gordon, D, Cain, C, and Ludomirsky, A. Noninvasive creation of an atrial septal defect by histotripsy in a canine model. Circulation. (2010) 121:742–9. doi: 10.1161/circulationaha.109.889071
77. Xu, Z, Hall, TL, Vlaisavljevich, E, and Lee, FT Jr. Histotripsy: the first noninvasive, non-ionizing, non-thermal ablation technique based on ultrasound. Int J Hyperth. (2021) 38:561–75. doi: 10.1080/02656736.2021.1905189
78. Gambihler, S, Delius, M, and Brendel, W. Biological effects of shock waves: cell disruption, viability, and proliferation of L1210 cells exposed to shock waves in vitro. Ultrasound Med Biol. (1990) 16:587–94. doi: 10.1016/0301-5629(90)90024-7
79. Giorgio, A, Tarantino, L, de Stefano, G, Coppola, C, and Ferraioli, G. Complications after percutaneous saline-enhanced radiofrequency ablation of liver tumors: 3-year experience with 336 patients at a single center. AJR Am J Roentgenol. (2005) 184:207–11. doi: 10.2214/ajr.184.1.01840207
80. Vlaisavljevich, E, Kim, Y, Owens, G, Roberts, W, Cain, C, and Xu, Z. Effects of tissue mechanical properties on susceptibility to histotripsy-induced tissue damage. Phys Med Biol. (2014) 59:253–70. doi: 10.1088/0031-9155/59/2/253
81. Vlaisavljevich, E, Maxwell, A, Warnez, M, Johnsen, E, Cain, CA, and Xu, Z. Histotripsy-induced cavitation cloud initiation thresholds in tissues of different mechanical properties. IEEE Trans Ultrason Ferroelectr Freq Control. (2014) 61:341–52. doi: 10.1109/TUFFC.2014.6722618
82. Edsall, C, Khan, ZM, Mancia, L, Hall, S, Mustafa, W, Johnsen, E, et al. Bubble cloud behavior and ablation capacity for histotripsy generated from intrinsic or artificial cavitation nuclei. Ultrasound Med Biol. (2021) 47:620–39. doi: 10.1016/j.ultrasmedbio.2020.10.020
83. Parsons, JE, Cain, CA, Abrams, GD, and Fowlkes, JB. Pulsed cavitational ultrasound therapy for controlled tissue homogenization. Ultrasound Med Biol. (2006) 32:115–29. doi: 10.1016/j.ultrasmedbio.2005.09.005
84. Roberts, WW . Development and translation of histotripsy: current status and future directions. Curr Opin Urol. (2014) 24:104–10. doi: 10.1097/mou.0000000000000001
85. Qu, S, Worlikar, T, Felsted, AE, Ganguly, A, Beems, MV, Hubbard, R, et al. Non-thermal histotripsy tumor ablation promotes abscopal immune responses that enhance cancer immunotherapy. J Immunother Cancer. (2020) 8:e000200. doi: 10.1136/jitc-2019-000200
86. Ghanouni, P, Pauly, KB, Elias, WJ, Henderson, J, Sheehan, J, Monteith, S, et al. Transcranial MRI-guided focused ultrasound: a review of the technologic and neurologic applications. AJR Am J Roentgenol. (2015) 205:150–9. doi: 10.2214/AJR.14.13632
87. Perez-Neri, I, Gonzalez-Aguilar, A, Sandoval, H, Pineda, C, and Rios, C. Potential goals, challenges, and safety of focused ultrasound application for central nervous system disorders. Curr Neuropharmacol. (2022) 20:1807–10. doi: 10.2174/1570159X20666220201092908
Keywords: focused ultrasound, glioma, glioblastoma, clinical trials, HIFUS, LIFUS, blood–brain barrier
Citation: Nwafor DC, Obiri-Yeboah D, Fazad F, Blanks W and Mut M (2024) Focused ultrasound as a treatment modality for gliomas. Front. Neurol. 15:1387986. doi: 10.3389/fneur.2024.1387986
Edited by:
Cesare Zoia, San Matteo Hospital Foundation (IRCCS), ItalyReviewed by:
Felix Stengel, Cantonal Hospital St.Gallen, SwitzerlandYan Zou, Henan University, China
Copyright © 2024 Nwafor, Obiri-Yeboah, Fazad, Blanks and Mut. This is an open-access article distributed under the terms of the Creative Commons Attribution License (CC BY). The use, distribution or reproduction in other forums is permitted, provided the original author(s) and the copyright owner(s) are credited and that the original publication in this journal is cited, in accordance with accepted academic practice. No use, distribution or reproduction is permitted which does not comply with these terms.
*Correspondence: Melike Mut, bW0yZWVAdXZhaGVhbHRoLm9yZw==