- Department of Neurology, University Hospital Frankfurt, Frankfurt, Germany
Background: Neuroplasticity as a mechanism to overcome central nervous system injury resulting from different neurological diseases has gained increasing attention in recent years. However, deficiency of these repair mechanisms leads to the accumulation of neuronal damage and therefore long-term disability. To date, the mechanisms by which remyelination occurs and why the extent of remyelination differs interindividually between multiple sclerosis patients regardless of the disease course are unclear. A member of the neurotrophins family, the brain-derived neurotrophic factor (BDNF) has received particular attention in this context as it is thought to play a central role in remyelination and thus neuroplasticity, neuroprotection, and memory.
Objective: To analyse the current literature regarding BDNF in different areas of multiple sclerosis and to provide an overview of the current state of knowledge in this field.
Conclusion: To date, studies assessing the role of BDNF in patients with multiple sclerosis remain inconclusive. However, there is emerging evidence for a beneficial effect of BDNF in multiple sclerosis, as studies reporting positive effects on clinical as well as MRI characteristics outweighed studies assuming detrimental effects of BDNF. Furthermore, studies regarding the Val66Met polymorphism have not conclusively determined whether this is a protective or harmful factor in multiple sclerosis, but again most studies hypothesized a protective effect through modulation of BDNF secretion and anti-inflammatory effects with different effects in healthy controls and patients with multiple sclerosis, possibly due to the pro-inflammatory milieu in patients with multiple sclerosis. Further studies with larger cohorts and longitudinal follow-ups are needed to improve our understanding of the effects of BDNF in the central nervous system, especially in the context of multiple sclerosis.
1 Introduction
Neuroplasticity as a mechanism to overcome central nervous system (CNS) injury has gained increasing attention in the context of different neurological diseases during the last years. In the field of multiple sclerosis (MS), histopathological studies have shown that remyelination can be found in a subset of patients with inflammatory processes as well as marked neurodegeneration (1). However, deficiency of these repair mechanisms leads to accumulation of neuronal damage and therefore long-term disability (2).
To date, the mechanisms by which remyelination occurs and why the extent of remyelination differs interindividually between MS patients regardless of the disease course are unclear. However, as increasingly effective therapeutic options designed to halt inflammation and slow neurodegeneration are available, approaches that might reverse preexisting, already accumulated damage are becoming increasingly important.
1.1 General information on BDNF and its cellular effects
A member of the neurotrophins family, the brain-derived neurotrophic factor (BDNF) has received particular attention in this context. As the most abundant growth factor in the brain, BDNF is thought to be of uttermost importance for the regulation of neuronal survival and death, neuronal proliferation as well as differentiation, synaptic plasticity, and repair processes, thus playing a key role in neuroprotection and neuroplasticity (3–6).
In the CNS, BDNF is synthesized and secreted by neurons as well as activated astrocytes and microglial cells. Astrocytes are the first cells to react to inflammatory CNS damage by expressing various surface markers, neurotrophic factors, and cytokines (7), thereby attracting microglia and macrophages to the site of damage. In turn, their activation leads to further release of cytokines as well as neurotrophic factors and phagocytosis of damaged cells (8). Microglia and macrophages exist in three different phenotypes. M0 is the resting state of microglial cells, while M1 and M2 are active forms, with M1 exerting inflammatory and M2 anti-inflammatory effects (9, 10). The M2-polarization can be induced by BDNF via activation of the tropomyosin-related kinase B (TrkB) receptor, which in turn induces the synthesis of anti-inflammatory cytokines and neurotrophic factors such as BDNF (10–12).
BDNF is synthesized and released in an activity-dependent manner (8) and through the expression of TrkB receptors, macrophages and microglia are able to react to BDNF, thus BDNF release occurs in an autocrine manner (8). Therefore, damage of astrocytes exerts neuroprotective effects through recruitment of macrophages and microglia by inducing their BDNF synthesis. This is further supported, as lesions with ongoing inflammation show higher concentrations of BDNF with upregulation of TrkB receptors on damaged neurons. Therefore, signaling of infiltrating microglia and macrophages through BDNF to damaged neurons supports the resolution of inflammation, neuronal survival and regeneration (13).
In the periphery, BDNF is also synthesized and released by platelets, monocytes and macrophages as well as activated T- and B-cells (14–16). Furthermore, endothelial cells in the periphery and in the brain are also capable of secreting BDNF (17–19), which can be triggered by various stimuli such as TNF-α, a proinflammatory cytokine (20) thought to play a pivotal role in MS pathophysiology (21). As endothelial cells release BDNF and also express TrkB receptors, an autocrine effect is hypothesized. However, TNF-α can not only induce the release of BDNF from endothelial cells, but also activates microglia, thus TNF-α can induce further cytokine release, thus amplifying the neuroinflammatory process (22). Yet, besides these deleterious effects, TNF-α also exhibits neuroprotective effects, as it is capable of inducing BDNF synthesis and release of astrocytes through MEK/ERK pathway (23, 24). Furthermore, as a negative feedback mechanism, BDNF was found to be capable of reducing TNF-a levels (24). On astrocytes, two different TNF-α receptors (TNFR) activating MAP kinase as well as NF-ĸB pathways were found (23): TNFR1 mainly exerts inflammatory and pro-apoptotic processes, while TNFR2 exerts anti-inflammatory effects, proliferation of oligodendrocyte progenitor cells (OPC) and thus remyelination and neuronal survival. TNFR2 was also found to be capable of enhancing BDNF translation through activation of NF-ĸB and cyclic adenosine monophosphate (AMP)-response element binding protein (CREB) (23–25). Still, these mechanisms seem to be time dependent. Rowhani-Rad and colleagues found the early inflammatory processes in experimental autoimmune encephalomyelitis (EAE)-lesions to reduce BDNF through activation of TNFR1, with increasing TNF-a levels. Within 2 weeks after induction of the lesions, BDNF levels increased while TNF-a levels decreased through TFNR-2 activation (24).
The release of BDNF depends on the neuronal activity, though different neuropeptides and hormones can also influence the secretion. If glutamate is released from excitatory synapses, depolarization is caused by an influx of Ca2+ and Na+ via α-amino-3-hydroxy-5-methyl-4-isoxazolepropionic acid (AMPA), N-methyl-D-aspartate (NMDA) and voltage-dependent Ca2+-channels. This causes calcium-dependent activation of Ca2+-calmodulin-dependent protein kinases (CaMK), protein kinase C (PKC), and mitogen-activated protein kinases (MAPKs), which in turn leads to transcription of the BDNF gene by CREB and nuclear factor ĸB (NF-ĸB). After transcription, BDNF is packaged in vesicles and transported axonally to the dendrites and presynaptic endings, where it can be released through the activation of glutamate receptors (26). A detailed description of the synthesis and release of BDNF was reviewed by Kowiański et al. (27) as well as Marosi and Mattson (26).
1.2 BDNF signaling pathways
After release, BDNF binds to its two receptors: TrkB receptor and neurotrophin receptor p75 (nt-p75). The nt-p75 receptor, as a member of the tumor necrosis factor (TNF) family, provides pro-apoptotic functions. However, because of its low affinity to BDNF, expression of ceramides, NF-ĸB, and jun-kinases leading to apoptosis only will be activated at higher concentrations (28). It is hypothesized that because of increasing BDNF secretion in inflammatory lesions with a high density of these receptors at the edge of inflammatory lesions, cell death will be initiated in case of severe neuronal damage.
Contrarily, due to its high affinity for BDNF, TrkB is activated even at low concentrations of BDNF, thereby activating phospholipase C gamma (PLC-γ), phosphatidylinositol-3 kinase (PI3-K) and MAP kinase pathway, illustrated in Figure 1. For remyelination, the proliferation, migration, and maturation of OPC to mature oligodendrocytes forming new myelin sheaths is crucial. Among others, intrinsic signaling cascades involving mTOR activation were shown to be relevant for this process (29). mTOR – a serine/threonine kinase - binds different proteins to form two protein complexes referred to as mTORC1 and mTORC2 (30). For synaptic plasticity, particularly mTORC1, consisting of mTOR as well as two proteins called raptor and GbL, is relevant (31, 32). By activation of the ribosomal protein S6 kinase (p70S6K), mTORC1 induces translation of proteins related to synaptic formation as well as excitatory postsynaptic currents (31), outgrowth of cytoskeletal filaments, axons as well as dendrites and dendritic spines (31, 33). Furthermore, the PI3K signaling pathway controls suppression of pro-apoptotic factors as Bad, Forkhead and thus reducing Fas ligand (33). Synergistically, activation of the MEK/ERK signaling pathway induces translation of the anti-apoptotic Bcl-2 (33). mTORC1 also inhibits autophagy through inhibition of ULK1, thus exerting further neuroprotective effects (31). However, as BDNF can activate these pathways through TrkB-receptors, BDNF is hypothesized to be a key factor promoting maturation of OPCs. Wong et al. could show, that in TrkB knock-out mice myelin protein expression, myelination as well as proliferative potential of OPCs was significantly decreased, thus proving that TrkB receptors are expressed on OPCs (34).
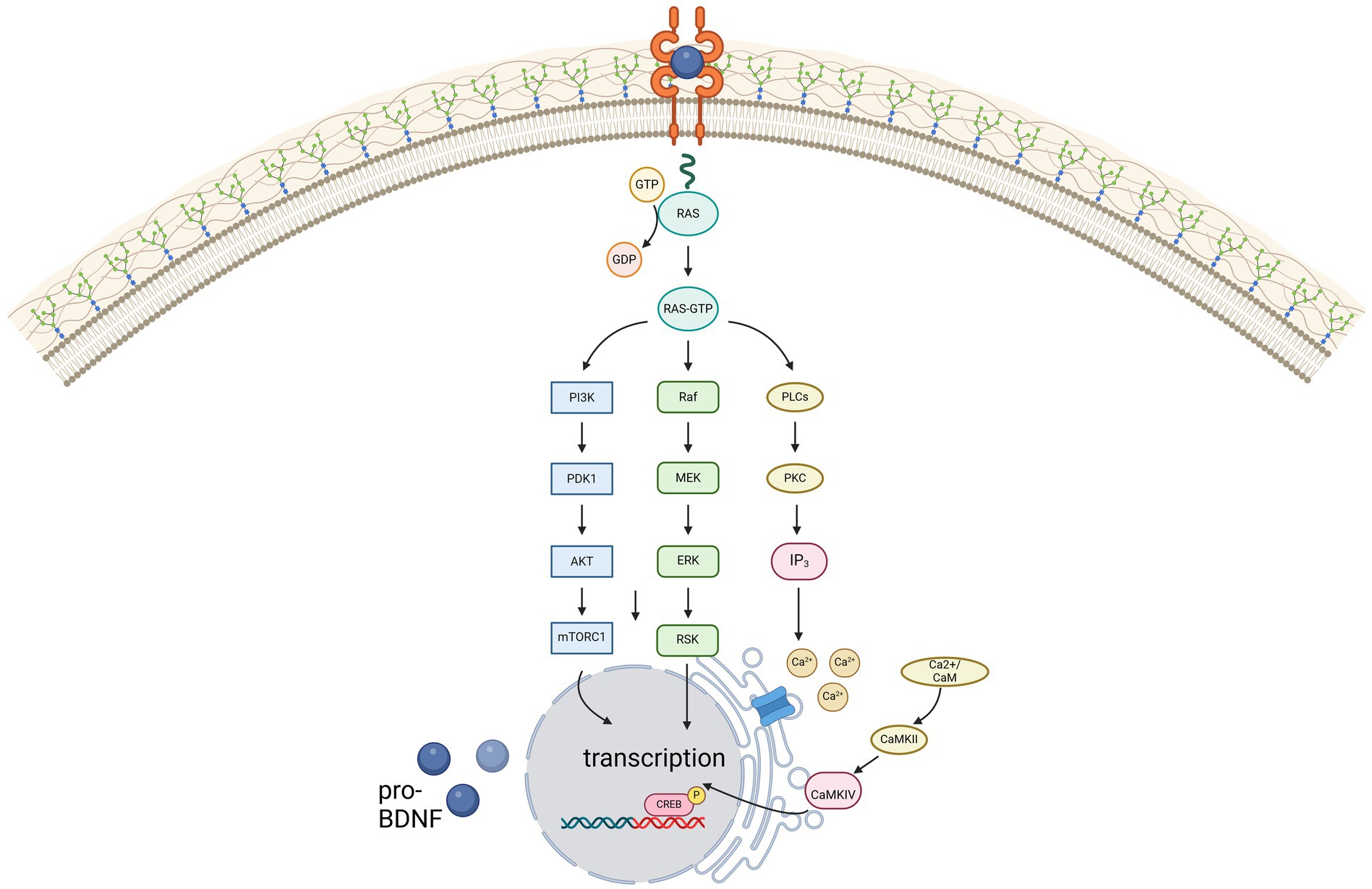
Figure 1. Illustration of the three main signaling pathways of BDNF, mediated via the high-affinity receptor TrkB. Created in BioRender.com.
However, the increased expression of antioxidant enzymes and improved DNA repair mechanisms also contribute to the survival of neurons. But not only the survival of neurons is supported by BDNF: by activating p21-ras, BDNF can stimulate neurite outgrowth and synaptogenesis, increase cytoskeletal dynamics, and modulate cell adhesion (23). Furthermore, the NMDA-receptor subunits NR1 and early growth response factor-3 mediated mechanisms can be increased by BDNF via CREB, being hypothesized to improve cognition by increasing Ca2+ influx in synapses involved in learning and memory (23). Therefore, BDNF plays a pivotal role in long-term potentiation. Additionally, as BDNF can increase the synthesis of the peroxisome proliferator-activated receptor gamma coactivator 1-alpha (PGC-1α) as well as the transcriptional factor FOXO3a, with both playing a key role in the cellular energy homeostasis, maintenance, and formation of synapses is enhanced (23, 35).
1.3 BDNF in context of MS
Because of these effects, BDNF has gained increasing attention in the context of MS, especially regarding possible effects on clinical improvement by its remyelinating mechanisms (36, 37). Interestingly, decreased (38–42) as well as increased (39, 43–46) or normal (37, 47, 48) BDNF levels were found in patients with MS (pwMS) compared to healthy controls (49). Still, most studies reported an increase of BDNF concentrations in presence of disease activity as clinical relapse (37, 39, 40, 42, 46, 50). Decreasing BDNF concentrations with disease progression may be linked to decreased BDNF secretion of lymphocytes, as a change of lymphocyte phenotype was found with disease progression (14). Still, some studies found no association of low BDNF levels with disease progression (51, 52).
To date, knowledge of the role of BDNF in the field of MS is scarce as studies have been inconclusive so far. Accordingly, this review aimed to analyse the current literature regarding BDNF in different areas of multiple sclerosis and to provide an overview of the current state of knowledge in this field.
2 BDNF-polymorphism
BDNF is encoded on chromosome 11 band p13-14 (53). Only a few genetic variants were discovered in the coding intron regions, with the Val66Met polymorphism being the best-studied variant to date. In this single nucleotide polymorphism (SNP), the amino acid valine is exchanged for methionine in codon 66 (54). Despite this polymorphism, a functional protein with an altered pro-domain is synthesized, which, however, leads to changes in protein folding and thus reduced binding to TrĸB (55), but also to changes in the interaction with various other proteins (54). It has been shown that the binding behavior of translin, a DNA-binding protein, to BDNF messenger ribonucleic acid (mRNA) is disrupted, resulting in impaired trafficking of BDNF transcripts within dendrites (56). Additionally, it is hypothesized that the SNP results in altered intracellular packing and transport (1, 55, 57). Both in vitro studies and studies in healthy volunteers have shown that the altered intracellular transport leads to an abnormal secretion, thereby reducing the activity-dependent BDNF concentration by 18-30% (1, 55, 57). Some studies could not find a reduction of peripheral BDNF levels, which is why a local influence of activity-dependent secretion by the polymorphism has been postulated (45, 58). Altered secretion of a pro-apoptotically active precursor of BDNF due to altered intracellular trafficking (55, 57, 59) and alteration of the rate at which pro-BDNF is cleaved extracellularly by enzymes into mature BDNF have also been shown to be associated with the SNP (60). However, the reduced activity-dependent secretion has also been confirmed in Val66Met knock-in mice (61).
Nonetheless, studies remain inconclusive with some showing higher (62, 63) and some showing lower (57, 64) or equal (65, 66) BDNF-serum concentrations in healthy Val66Met carriers, whereas RRMS patients with Met alleles had higher BDNF concentrations than healthy carriers (45). However, some studies did not find differences in the BDNF serum concentration between healthy controls and RRMS patients with Val66Met polymorphism (45, 52, 67, 68). Studies also showed different results about peripheral mRNA levels, on the one hand with no detectable difference between Val homozygotes and Met carriers in either healthy subjects or RRMS patients (52, 67, 68), but on the other hand higher mRNA levels in RRMS patients compared to healthy Met carriers (45, 54).
3 BDNF and cognition
BDNF is thought to play a central role in synaptic plasticity (36, 69). It is hypothesized to play an important role in the long-term potentiation and neuroplasticity of the hippocampus (70), which is essential for learning and memory (71, 72). Several pathways how BDNF could affect episodic memory are discussed. However, increased activation of NMDA and AMPA-receptors and therefore increased intracellular calcium as well as sodium and potassium concentrations due to enhancement of protein translation via TrkB is hypothesized to play a key role (72, 73).
However, studies assessing the relationship between serum BDNF concentrations and cognitive performance have been inconclusive so far.
In animal models, low BDNF concentrations resulted in cognitive deficits due to disturbed neurotransmission and neuroplasticity (5, 74–76). In pwMS, a relationship between BDNF concentrations and cognitive performance was assumed (77). It was considered that the pathological changes leading to cognitive impairment were compensated through hippocampal hyperactivation to preserve episodic memory as seen in functional MRI (78, 79), which could be driven by BDNF (55, 80–83). Some studies reported a correlation of high BDNF levels with improvement of memory (84–86) and correlation of decreased BDNF concentrations with mild cognitive impairment even in newly diagnosed pwMS with no or mild disability (36). A study analysing changes in BDNF concentration after physical activity for 1 year found increasing BDNF levels with improvement of cognitive functions (87, 88). In reverse, a lack of BDNF or a disruption of the BDNF-TrkB pathway led to loss of synapses and decreasing cognitive functions (69, 89).
Regarding the effects of the Val66Met SNP on cognition, it is hypothesized, that the SNP prevents the development of maladaptive cortical plasticity in pwMS, resulting in improved recovery after relapses compared to Val homozygotes (90). A deleterious effect of the polymorphism on the parieto-prefrontal network and hippocampal activity, however, has been demonstrated in healthy subjects, whereas the opposite was found in RRMS patients (91). Val homozygote RRMS patients showed higher activity in the episodic memory network, maybe as form of compensatory mechanisms (92). Furthermore, pwMS with Val66Met polymorphism showing altered hippocampal activity while performing an episodic memory task (81, 82) had poorer episodic memory and executive functions (49, 81, 93). Still, some studies did not find any association of the Val66Met polymorphism with cognitive impairment in pwMS (45, 60, 94).
To summarize, some studies concluded that BDNF influences cognition, rather on a global level than influencing specific cognitive domains (86, 95), while other studies did not find an association between BDNF levels and cognition. Furthermore, most studies reported no detrimental effect of the Val66Met polymorphism on cognition. Table 1 provides an overview of the above-mentioned studies investigating the relationship between BDNF and cognition.
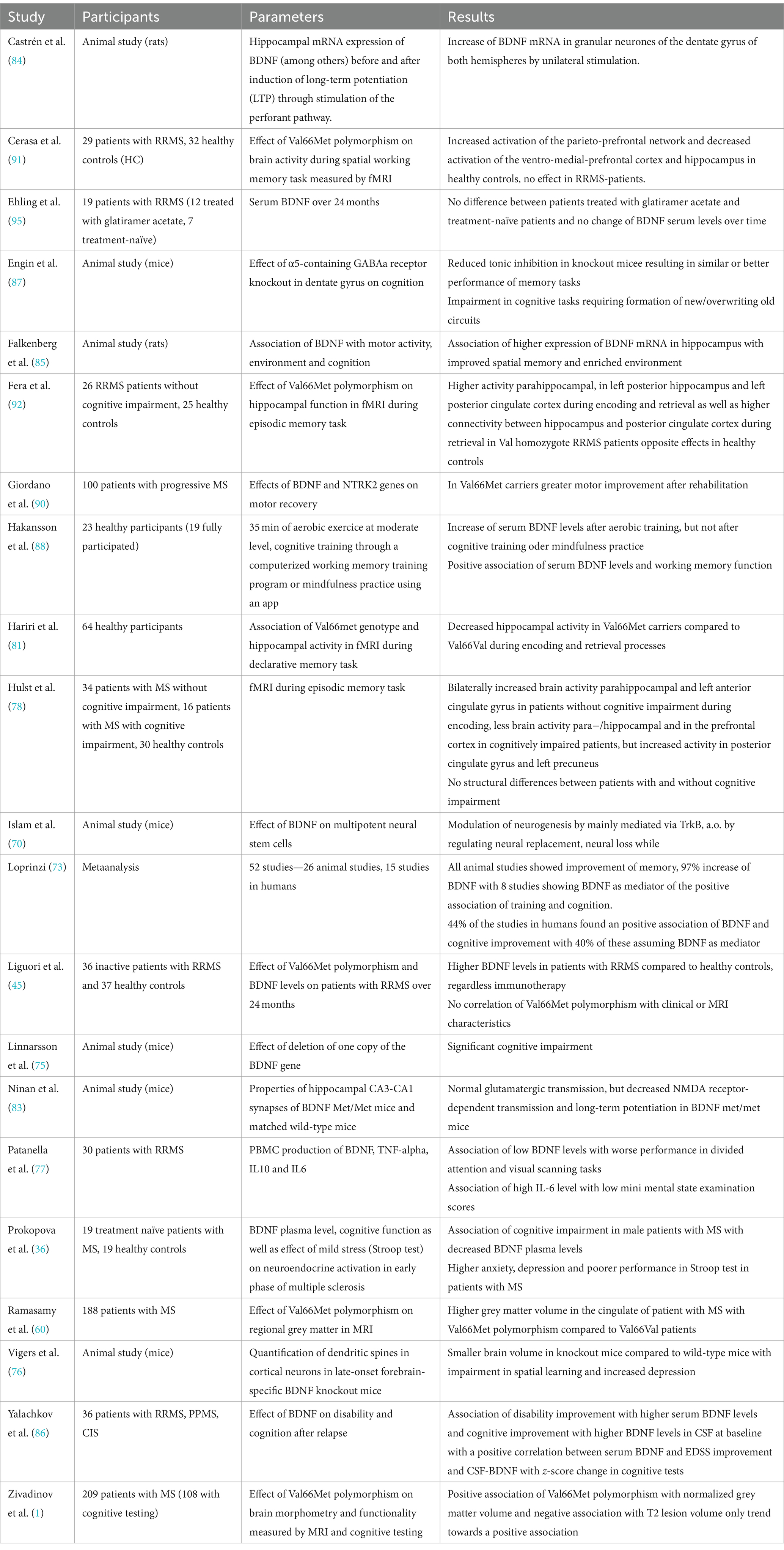
Table 1. Overview of the above-mentioned studies investigating the relationship between BDNF and cognition.
4 BDNF and disability
Studies assessing the effect of BDNF on disease severity, disability improvement, or worsening are scarce.
Some studies found no relationship between the BDNF concentration and the disease severity assessed by multiple sclerosis Severity Score (96). Contrarily, in a pilot study, Yalachkov and colleagues found that higher serum BDNF concentrations at baseline were associated with a disability improvement measured as an EDSS decrease of ≥0,5 12 months after relapse (86).
However, most studies focused on the relationship between the disease severity and the BDNF Val66Met polymorphism. No differences between Val66Met carriers and Val homozygotes were found regarding disease susceptibility (58, 94, 97), age at onset or disease severity (1, 49, 97, 98) assessed by EDSS (45, 94, 97, 98). Still, Val66Met carriers showed greater motor recovery after rehabilitation assessed by 6 min walk test (6-MWT) (90). Lower methylation of the BDNF gene was associated with higher gene expression and a higher risk of achieving an EDSS of 6,0 (99, 100). Only one study found susceptibility and disease outcome to be influenced by the polymorphism, especially in females (101). Furthermore, no association of the Val66Met polymorphism was found for disease duration, relapse rate, time between relapses, or time to reach EDSS milestones like 4 or 6 or secondary progression. To this end, no detrimental effect of the SNP was found in pwMS (98, 99).
However, further studies with larger sample sizes and long-term follow-up are needed to assess the effect of BDNF on disease activity and severity, as studies are scarce and results inconclusive so far. A summary of the studies assessing the relationship between BDNF and disability can be found in Table 2.
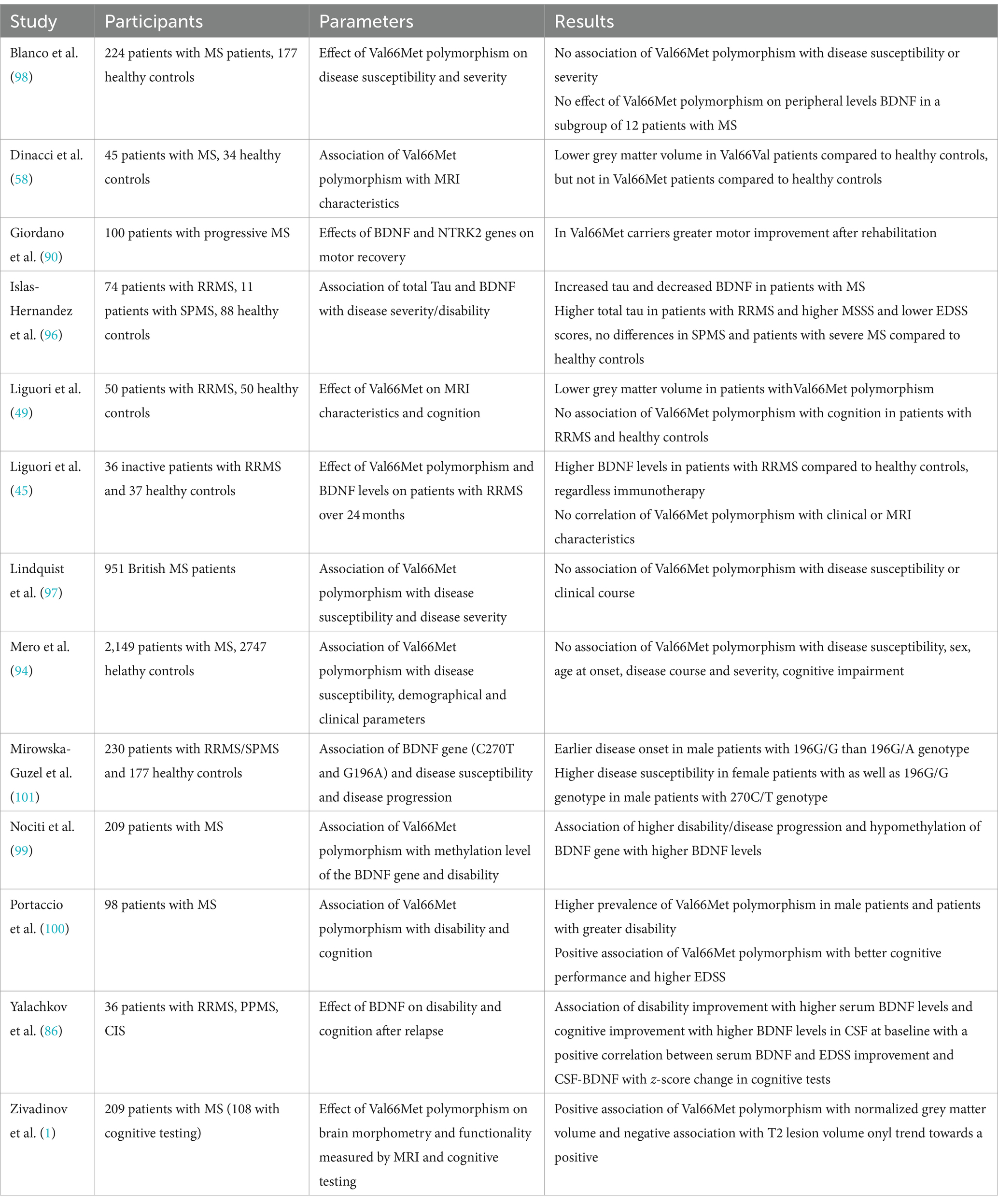
Table 2. Overview of the above-mentioned studies investigating the relationship between BDNF and disability.
5 Effect of BDNF on MRI parameters
Again, more studies analysed the association of the Val66Met polymorphism to MRI characteristics in pwMS, compared to only few studies assessing the association of these to BDNF levels.
Comini-Frota and colleagues examined the correlation between BDNF and T2-lesion number in 28 patients with RRMS compared to 28 healthy controls. While they found BDNF levels correlating negatively with the number of lesions, BDNF levels did not correlate with T1 hypointense lesions or the presence of gadolinium-enhancing lesions. The authors concluded that as BDNF controls remyelinating processes, no correlation with T1 lesions as chronic lesions was found (102). However, this argumentation does not fully explain the missing correlation between BDNF and gadolinium-enhancing lesions as they display acute inflammatory lesions (102). Furthermore, another study found a negative correlation of serum BDNF levels with T1 lesion volume as well as quotient of T1 lesion volume to total lesion volume, displaying the relative contribution of T1 lesion volume to the total lesion volume, while no correlations were found for CSF BDNF level (103). In contrast, Weinstock-Guttman and colleagues found an association between higher BDNF concentrations and higher inflammation in white matter (WM), correlating with a higher WM volume. Moreover, they found that higher BDNF is associated with microscopic damage in the normal-appearing WM (NAWM) and concluded that BDNF may play a role in the early formation of inflammatory lesions or that diffuse inflammatory infiltrates without correlate on MRI are the pathological explanation for this (52). Regarding magnetization transfer ratio (MTR), the study found a negative correlation with increased BDNF, while no correlation between grey matter (GM) volume and normal appearing GM (NAGM) MTR was found with BDNF-levels (52), which might indicate that BDNF secretion only increases in inflammatory tissue (37).
However, more studies investigated the relationship between Val66Met polymorphism with MRI changes.
Though some studies reported a higher risk of GM atrophy in pwMS with Val66Met polymorphism (49, 91), most studies reported a protective effect of the SNP on brain atrophy (52, 58, 90) as well as GM atrophy (1, 52, 58, 60). Though higher GM volume was found throughout the brain in pwMS with Val66Met polymorphism, higher volume was especially found in the cingulate (1, 60), inferior frontal gyrus, parahippocampal gyrus, middle temporal gyrus, precentral gyrus, and inferior parietal lobule of the left cerebral hemisphere, in the right cerebral hemisphere, in the precuneus and middle frontal gyrus (1), consistent with regions of pronounced expression of BDNF (104, 105). As the highest atrophy related to the lesion volume in pwMS was found in the anterior cingulate (106) especially on the left hemisphere (107), a local influence of the polymorphism on BDNF secretion and thus on local inflammation has also been discussed to have a protective effect on regional brain volume in pwMS (58). Decreased cortical thickness was found in one study (108), thus a detrimental effect of the SNP was hypothesized. In another study, higher hippocampal volumes were found in pwMS with Val66Met polymorphism, compared to Val homozygotes (109). Therefore, protection of hippocampal tissue was assumed (109). Moreover, an association of the Val66Met polymorphism was found with lower T2-lesion volume (1) in comparison with Val66Val patients, though other studies did not find differences between groups in T2 or T1 lesion volume (45, 52, 108). Matching these structural imaging findings, increased functional connectivity between the hippocampus and posterior cingulate cortex was found in pwMS with Val66Met polymorphism during an episodic memory task (92). Still, two other studies reported a negative impact of the SNP polymorphism on functional activity of the brain. While Egan and colleagues showed an altered hippocampal activation (55), another study showed altered functional connectivity extending the hippocampal system with decreased functional connectivity in the ventromedial PFC and ACC-networks (91).
Overall, the study results indicate lower rates of brain atrophy and less pronounced signs of damage associated with BDNF. Regarding the Val66Met SNP, most studies brought evidence that the SNP has different effects on MRI measures in healthy controls compared to pwMS with the majority of studies assuming a protective effect of Val66Met polymorphism in pwMS. An overview of the above-mentioned studies can be found in Table 3.
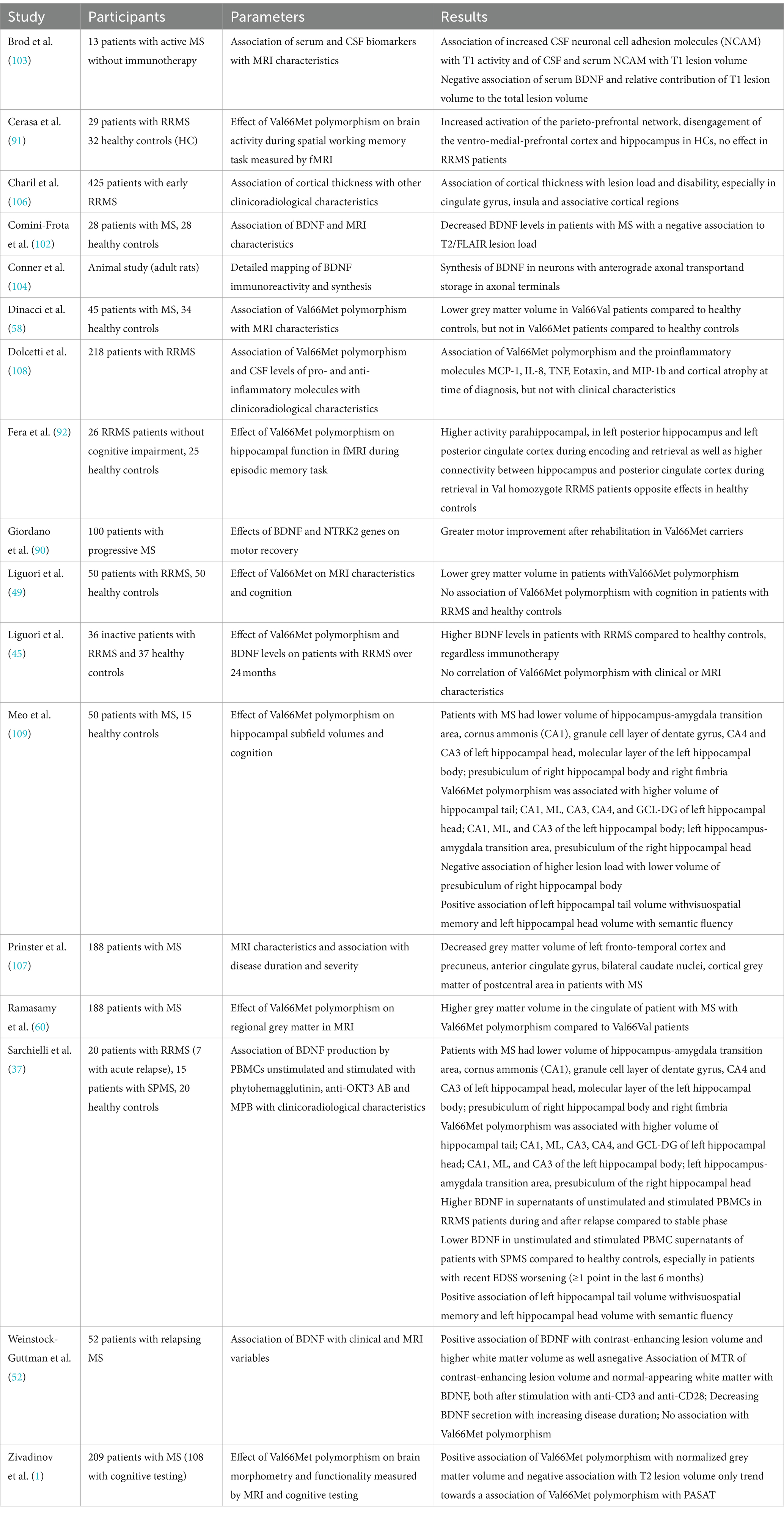
Table 3. Overview of the above-mentioned studies investigating the relationship between BDNF and MRI characteristics.
6 Effect of immunotherapies on BDNF
Immunotherapies have been hypothesized to modulate the disease course not only by exerting anti-inflammatory effects but also neuroprotective and neuroregenerative mechanisms. Therefore, the correlation of immunotherapies with BDNF was investigated in several studies. So far, especially interferon-beta (IFN- β) and glatiramer acetate have been analysed.
For glatiramer acetate, higher BDNF levels were found in pwMS (39, 45, 46, 110–112). In an ex-vivo study, a significant increase of BDNF levels in supernatants of unstimulated peripheral blood mononuclear cells (PBMC) was found 6 months after initiation of glatiramer acetate treatment, supported by studies analysing glatiramer acetate specific T-cell lines (110). As a possible mechanism, a shift of pro-inflammatory T helper (TH) 1 cells to anti-inflammatory TH2 cells, which is the main therapeutic action of glatiramer acetate, is thought to promote neuroregenerative mechanisms due to BDNF induction and release (110, 113). Furthermore, besides anti-inflammatory TH2-cytokines, glatiramer acetate-specific cells were thought to express BDNF themselves (95, 114, 115). This is further supported by animal studies also providing evidence that glatiramer acetate exhibits neuroprotective effects through BDNF. In EAE, increased BDNF levels were found after treatment initiation (39, 111, 112, 116–118) as well as an augmentation of BDNF expression in histological glatiramer acetate treated EAE (116) with reduced axonal damage and decreased neurodegeneration (119). Moreover, in EAE the clinical efficacy of glatiramer acetate was limited after BDNF deletion (120). However, other studies showed no increase of BDNF levels in pwMS treated with glatiramer acetate (50, 95, 121).
In clinically stable patients with INF-β treatment, significantly increased BDNF levels were found (38, 46) compared to treatment naïve patients, sustained after 6 months as well as a trend to increased level after 1 year in RRMS, but not in SPMS-patients (43). Though patients treated with INF-ß showed higher BDNF levels in relapse-free intervals compared to healthy controls, no additional increase of BDNF was found in relapses (42). Moreover, patients treated with IFN-β not only had higher BDNF levels compared to treatment naïve patients but also in comparison to patients treated with mitoxantrone with an inverse correlation between BDNF levels and degree of disability (51). Contrary to this, some studies reported no effect of IFN-β on BDNF levels (15, 43, 45, 122). In a mixed cohort with 38 subjects treated with IFN-β and 12 with glatiramer acetate, no significant increase of BDNF levels was found after 1 year, with no differences between treatment groups (123).
Studies investigating the effect of other immunotherapies than INF-ß and glatiramer acetate are rare. However, Golan and colleagues showed a significant increase of BDNF in patients treated with fingolimod after 6 and 12 months (121), which is in line with a previous animal study, showing that the neuroprotective effect of Fingolimod is associated with the induction of the BDNF production (124). One study also could show increased BDNF levels in RRMS-patients treated with natalizumab (125). Moreover, significantly lower BDNF concentrations in patients treated with high-dose immunoglobulins were found, possibly due to interference of immunoglobulins with neuroprotective mechanisms (122). Regarding the effects of therapy of acute relapses with glucocorticoids, increased BDNF levels could be shown in EAE (95), but could not be confirmed in humans (37, 42).
To summarize, most studies hypothesized increased BDNF concentrations in patients treated with glatiramer acetate and IFN-β, while the number of studies in patients with other immunotherapies was too small to draw a conclusion. For details of the respective studies, see Table 4.
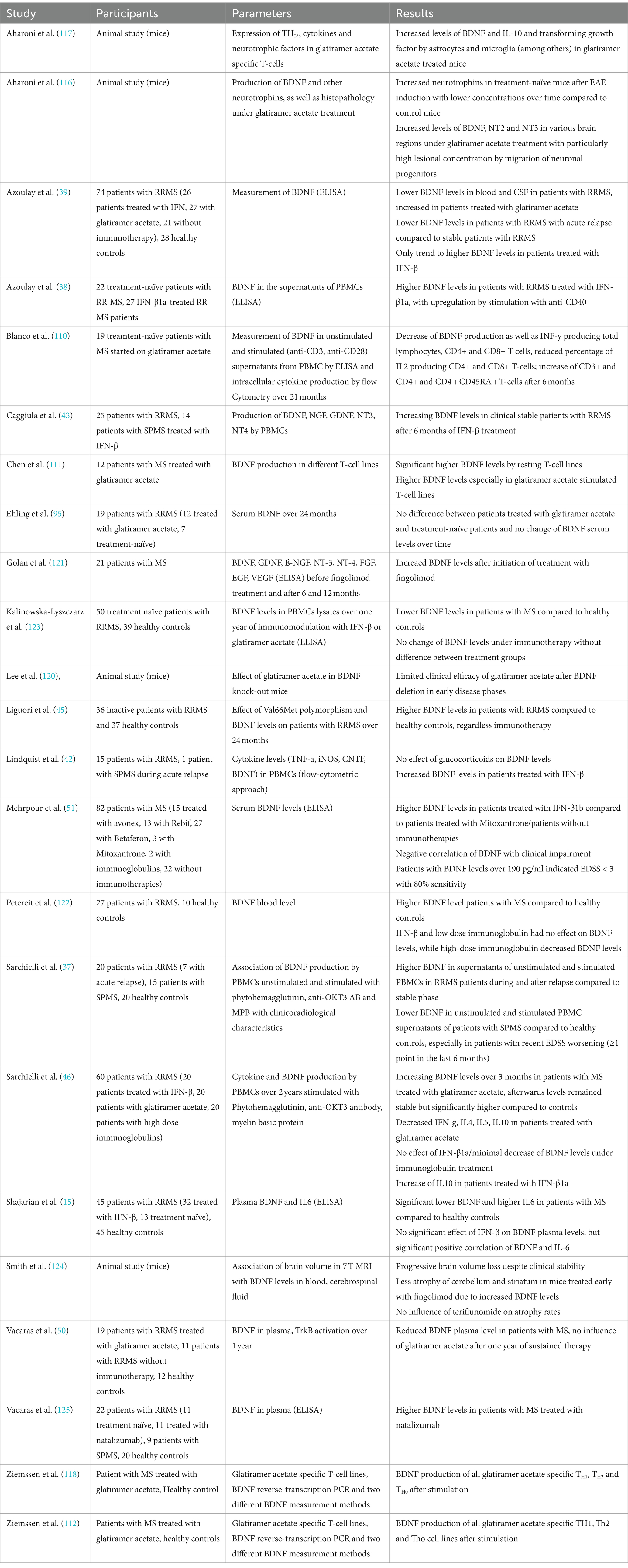
Table 4. Overview of the above-mentioned studies investigating the relationship between BDNF and immunotherapies.
7 Effect of autologous hematopoietic stem cell transplantation on BDNF
Autologous hematopoietic stem cell transplantation (HSCT) has received increasing attention in the field of MS therapy in the past few years. Although studies could show a sustained suppression of inflammation and therefore disease activity, the exact mechanisms of action remain unclear (126). Muraro and colleagues showed a significant increase of naïve CD4-positive T-cells two years after HSCT, suggesting the suppression of inflammatory activity in pwMS after HSCT does not depend on persisting suppression of lymphocytes, but qualitative immunological changes (126). Consequently, an increasing number of studies with follow-up data from large registries have shown positive effects on disease activity with stabilization (127), disease severity as measured by EDSS either with stabilization or even improvement (127), fatigue and quality of life (128). Positive effects of HSCT have also been demonstrated on paraclinical markers of disease activity such as new or enlarging T2 lesions or contrast-enhancing lesions (127). However, mostly patients with active RRMS or SPMS benefit from HSCT (129). In a study comparing immunotherapies and HSCT in 110 patients with RRMS, non-myeloablative HSCT resulted in prolonged time to disease progression (130).
Our literature research revealed only one study examining the effect of HSCT on BDNF levels in serum and CSF (127). In 14 pwMS, serum BDNF levels at baseline measurement were significantly higher compared to controls with a significant decrease over 12 months, almost at the level of healthy controls. Furthermore, a significant decrease of BDNF levels was also found in CSF, though CSF BDNF levels were only accessible in 9 patients. Possibly, the decreasing BDNF levels were due to the sustained suppression of CD4+ T-cells. However, only a positive correlation was found between CSF BDNF level and the T2 lesion load at baseline and at 12 month follow-up. Though one could assume a possible detrimental effect of decreased BDNF, no neurological deterioration nor correlations between BDNF and atrophy measurement were found. Blanco and colleagues provided evidence that BDNF levels decreased due to HSCT, possibly due to a shift of pro-inflammatory TH1 to anti-inflammatory TH2-cells, supporting the hypothesis that HSCT does not only has immunosuppressive but also immunomodulatory effects (127).
As HSCT becomes more and more relevant in the treatment of MS, it is likely that the number of studies analysing the immunomodulatory effects and its relationship with BDNF will increase over the next few years. Table 5 summarises the studies mentioned above.
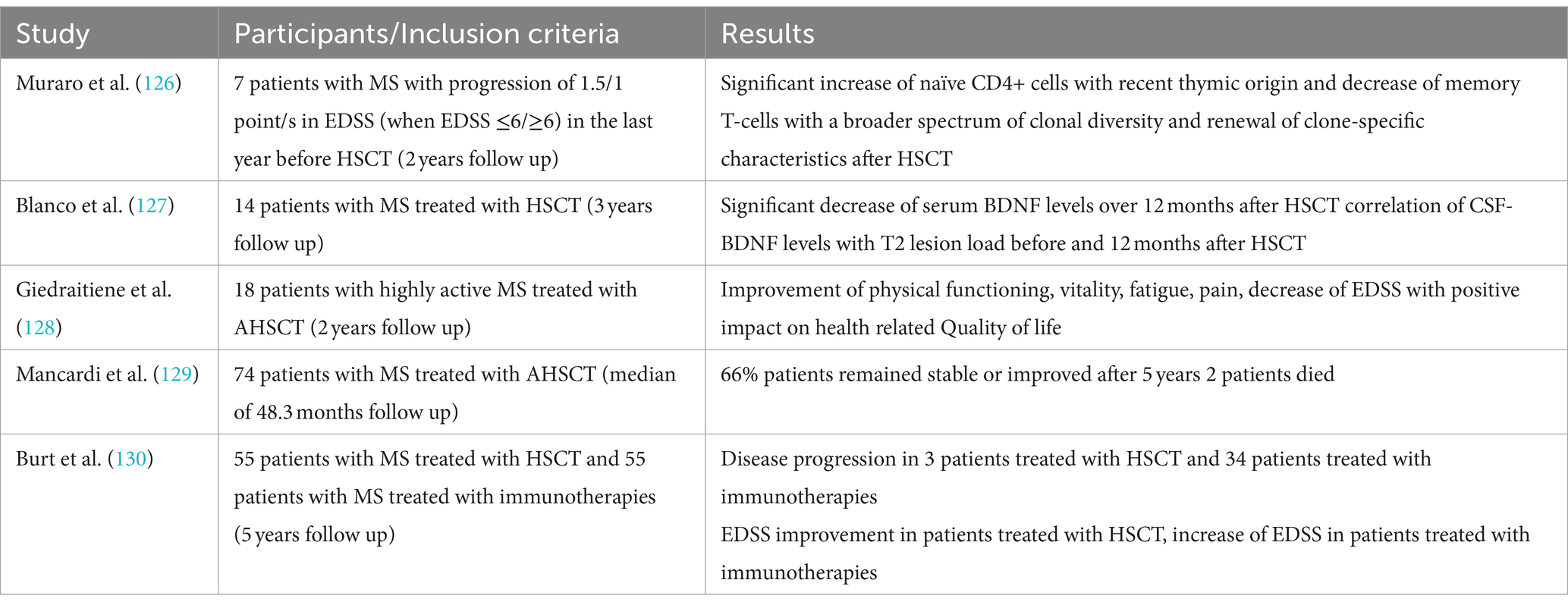
Table 5. Overview of the above-mentioned studies investigating the relationship between BDNF and HSCT.
8 Influence of physical activity and exercise training on BDNF
In recent years, there has been increasing evidence that physical activity as well as exercise has positive effects on pwMS, exerting neuroprotective effects and thus slowing neurodegeneration and disease progression (131). It is hypothesized that BDNF – as well as other neurotrophins – are secreted in dependency of neuronal activity (132–134) and due to certain factors transmitted in the bloodstream from peripheral organs as skeletal muscles (135–137). Hence, muscle activity leads to increasing neurotrophin levels in the blood, ultimately migrating in the central and peripheral nervous system (135, 137). As a result, there have been numerous studies linking neuroprotective effects of exercise to neurotrophic factors such as BDNF (138, 139). In case of running, increasing intracellular Ca2+ concentrations and induction of CaMK via CREB were found to induce BDNF synthesis (23, 140). Still, it is controversial whether peripheral BDNF can pass the blood–brain barrier to induce effects in the CNS. Hakansson and colleagues found an immediate increase of serum BDNF levels after 35 min of physical activity, while participants doing cognitive training or mindfulness practice for 35 min showed no increases (88). A study assessing the effect of resistance training on BDNF within two hours after the training found no within and between-group differences before and no within-group differences after the 24 weeks long training intervention (141). Moreover, four other studies failed to assess within or between-group differences in BDNF serum level (142–145), while two studies only assessed within group differences with an increase of BDNF level during the exercise intervention (146, 147). However, the majority of studies assessed between-group differences favouring the exercise intervention (148–153). In a meta-analysis assessing the effects of exercise training on neurotrophic factors, the results for different neurotrophins (NGF, CNTF, IGF-1, NT3-5, GDNF, PDGF as well as VEGF) remained inconclusive, but for BDNF a significant increase after training intervention was found (154). The main problem making the interpretation of these results difficult is the different handling of the samples and the usage of different analysing kits with different sensitivity and detection ranges as well as the different training interventions, the frequency of the training as well as the duration of the intervention. Though studies in healthy controls indicated that the modality of the training as well as the intensity and duration are crucial for the positive effects of training on increasing BDNF levels (155–162), a study in pwMS found no difference of the immediate BDNF increases after continuous moderate or high-intensity interval aerobic training (163). However, aerobic exercise seems to be one of the most potent training forms regarding the effect on neurotrophic factors (164), which was confirmed for BDNF by a recent meta-analysis (154). While studies evaluating the effect of exercise on the expression of neurotrophic factors and the link to neuroprotection in humans have not been conclusive so far (154), animal models already brought strong evidence for exercise-induced increase of BDNF (138, 139, 165–168). Moreover, effects of physical training on cognitive functions mediated by BDNF were analysed in several studies. Hakansson and colleagues found a positive effect on working memory function and peripheral BDNF levels after physical activity (88). Loprinzi and colleagues furthermore reviewed studies focusing on exercise effects on memory and the role of BDNF (73). Most studies found increasing serum BDNF levels after exercise, with 8 studies also blocking BDNF pharmacologically to evaluate exercise effects on memory. When BDNF was blocked, no effects of exercise on memory could be found. Without blocking BDNF, almost half of the studies found a positive effect of exercise on memory (73).
Interestingly, only a few studies assessed the association of training with other characteristics of disease activity as MRI parameters. Savšek and colleagues found protective effects of 12 week aerobic training on atrophy of some substructures as well as a decreasing active lesion volume and count, but without effect on total brain volume, grey matter atrophy, and T2 lesion volume and count as well as cortical lesion count, accompanied with increases of BDNF serum levels in the exercise group (152).
All in all, there is emerging evidence that physical activity increases serum BDNF levels with positive effects on cognition and MRI characteristics. However, the endurance of these increases as well as the effects on physical disability remains subject of further studies. Table 6 provides an overview of the studies assessing the relationship between BDNF and training.
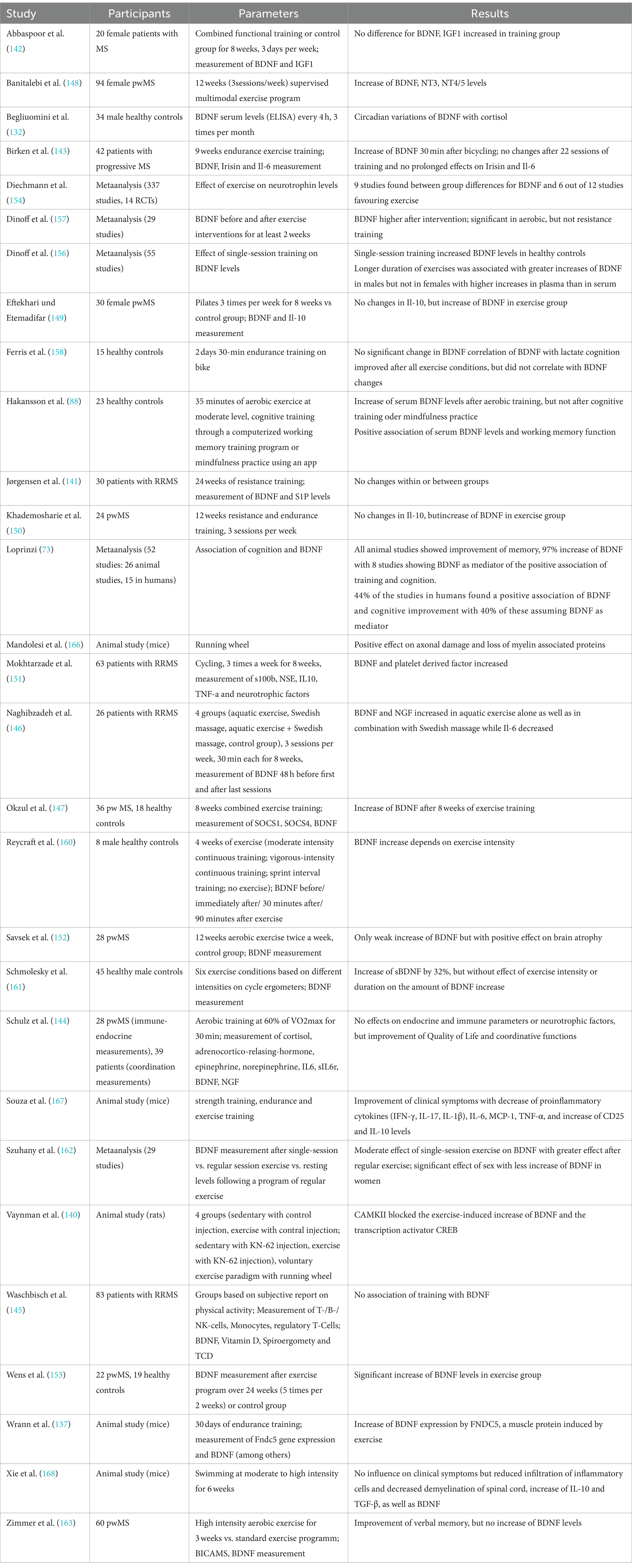
Table 6. Overview of the above-mentioned studies investigating the relationship between BDNF and training.
9 Discussion and conclusion
With increasingly effective therapeutic options to halt inflammation, mechanisms to reverse preexisting, already accumulated damage are becoming increasingly important in context of neuroinflammatory diseases as MS. We reviewed the current literature regarding BDNF, which is thought to play a central role in remyelination and thus neuroplasticity, neuroprotection, and memory, in different areas of MS.
In terms of cognition, studies assuming a positive impact of BDNF on cognitive functions outweigh studies assuming a detrimental effect, although it remains unclear whether the effect is limited on different cognitive domains or whether BDNF improves cognitive functions on a more global level. In this context, especially longitudinal, prospective studies are needed, which also include other so-called “hidden symptoms” of MS such as depression and fatigue.
With regard to the effect of BDNF on disability, especially the improvement of neurological deficits, there is even less evidence, although a positive influence of BDNF on the deficits is also to be favoured here.
However, most of the studies analysed the effect of BDNF on different clinicoradiological characteristics in patients with relapsing disease course or in mixed cohorts mainly consisting of patients with relapsing disease course, therefore, drawing conclusions on the effects of BDNF in patients with progressive disease is difficult. Still, as it is hypothesized that in patients with progressive disease neuroregenerative mechanisms are failing, one could assume that BDNF mainly plays a role in relapsing disease. To date, it is unclear why these mechanisms fail in patients with progressive disease. An exhaustion of BDNF because of compensatory increased synthesis and release in the early years of the disease could be an explanation but needs to be evaluated in further studies with a larger proportion of patients with progressive disease course compared to patients with relapsing disease course.
Furthermore, studies regarding the Val66Met polymorphism have not conclusively determined whether the SNP is a protective or harmful factor in pwMS, but the majority of studies hypothesize a protective effect through modulation of BDNF secretion and anti-inflammatory effects (60). Interestingly, the polymorphism, detectable in up to 72% of the population and thus in a large proportion of healthy subjects (54), seems to have different effects in healthy subjects and pwMS (1, 100). In conclusion, the pro-inflammatory milieu in which BDNF acts in pwMS seems to be essential for these different effects (1, 92, 100). Increased de-methylation of BDNF in an inflammatory milieu could lead to increased secretion and thus neuroprotection. However, Noiciti and colleagues assumed a detrimental effect of increased BDNF secretion as it may result in depletion of the functional reserves and thus faster accumulation of long-term disability (99).
In the author’s opinion, one of the main problems here is the lack of a standardized method for measurement of BDNF. BDNF can be measured in serum as well as normal and platelet-poor plasma. However, as no correlations between serum and plasma BDNF concentrations could be found (169), it has to be assumed that the material used has a relevant influence on the study results. In plasma, BDNF is mainly stored in platelets, therefore, only low concentrations of free BDNF can be assessed. In serum, BDNF is released from platelets due to coagulation. However, in most studies serum was used for measurement of peripheral BDNF concentrations (170). But besides the different material that can be used for measurement of BDNF levels, different methods have been described (170). Mainly, different techniques using immunoassays were used and validated (171), with most studies using enzyme linked immunosorbent assay (ELISA), and to a lesser extent western blot techniques (172). However, since 2013 a single molecule array (SIMOA) technology being able to detect fluid biomarkers on a single-molecule level (171) has been introduced and is increasingly used in studies.
However, despite the inconclusive results and technical difficulties, the author thinks that there is emerging evidence for a neuroprotective and neuroregenerative effect of BDNF in MS, as studies reporting a protective effect outweighed studies assuming detrimental effects of BDNF. BDNF could therefore be a promising biomarker in the field of MS. Still, there is an urgent need for longitudinal prospective studies to improve our understanding of the effects of BDNF in the CNS, especially in context of MS.
Most studies have already shown that BDNF concentrations increase during relapse, although the extent of the increase and thus presumably the neuroregenerative capacity and corresponding recovery from relapses appears to vary between individuals. In consequence, patients with insufficient neuroregenerative capacity could be identified at an early stage and primarily be put on high efficacy therapies. However, understanding which pwMS are at risk of incomplete recovery from relapses by measuring BDNF levels and analysing the association with different clinicoradiological characteristics would significantly improve the clinical management of MS patients.
It has already been shown for glatiramer acetate or IFN-β that BDNF concentrations increase after initiation of these immunotherapies, for glatiramer acetate possibly due to a switch from pro-inflammatory TH1 to anti-inflammatory TH2 cells. Therefore, further studies comparing the effect of baseline therapies vs. high efficacy therapies on BDNF concentrations are needed. The role of BDNF as a potential therapeutic agent to improve pre-existing neurological deficits should also be analysed in further studies. Nagahara and Tuszynski already reviewed the use of BDNF in different neurological diseases as Alzheimer’s and Parkinson disease as well as spinal cord injuries (173). However, to our best knowledge, similar studies in MS are lacking. Still, as positive results in studies evaluating the effect of BDNF therapy on spinal cord injuries promoting axonal regrowth were found, BDNF could also be a promising approach in multiple sclerosis reversing the demyelination in inflammatory CNS lesions.
Author contributions
MM: Writing – original draft, Writing – review & editing.
Funding
The author(s) declare that no financial support was received for the research, authorship, and/or publication of this article.
Conflict of interest
The author declares that the research was conducted in the absence of any commercial or financial relationships that could be construed as a potential conflict of interest.
Publisher’s note
All claims expressed in this article are solely those of the authors and do not necessarily represent those of their affiliated organizations, or those of the publisher, the editors and the reviewers. Any product that may be evaluated in this article, or claim that may be made by its manufacturer, is not guaranteed or endorsed by the publisher.
References
1. Zivadinov, R, Weinstock-Guttman, B, Benedict, R, Tamaño-Blanco, M, Hussein, S, Abdelrahman, N, et al. Preservation of gray matter volume in multiple sclerosis patients with the met allele of the rs6265 (Val66Met) SNP of brain-derived neurotrophic factor. Hum Mol Genet. (2007) 16:2659–68. doi: 10.1093/hmg/ddm189
2. KhorshidAhmad, T, Acosta, C, Cortes, C, Lakowski, TM, Gangadaran, S, and Namaka, M. Transcriptional regulation of brain-derived neurotrophic factor (BDNF) by methyl CpG binding protein 2 (MeCP2): a novel mechanism for re-myelination and/or myelin repair involved in the treatment of multiple sclerosis (MS). Mol Neurobiol. (2016) 53:1092–107. doi: 10.1007/s12035-014-9074-1
3. Chao, MV . Neurotrophins and their receptors: a convergence point for many signalling pathways. Nat Rev Neurosci. (2003) 4:299–309. doi: 10.1038/nrn1078
4. Chao, MV, Rajagopal, R, and Lee, FS. Neurotrophin signalling in health and disease. Clin Sci (Lond). (2006) 110:167–73. doi: 10.1042/CS20050163
5. Lu, B, Nagappan, G, and Lu, Y. BDNF and synaptic plasticity, cognitive function, and dysfunction. Handb Exp Pharmacol. (2014) 220:223–50. doi: 10.1007/978-3-642-45106-5_9
6. Xu, B, Gottschalk, W, Chow, A, Wilson, RI, Schnell, E, Zang, K, et al. The role of brain-derived neurotrophic factor receptors in the mature hippocampus: modulation of long-term potentiation through a presynaptic mechanism involving TrkB. J Neurosci. (2000) 20:6888–97. doi: 10.1523/JNEUROSCI.20-18-06888.2000
7. Yang, H, Feng, G-D, Liang, Z, Vitale, A, Jiao, X-Y, Ju, G, et al. In vitro beneficial activation of microglial cells by mechanically-injured astrocytes enhances the synthesis and secretion of BDNF through p38MAPK. Neurochem Int. (2012) 61:175–86. doi: 10.1016/j.neuint.2012.04.020
8. Asami, T, Ito, T, Fukumitsu, H, Nomoto, H, Furukawa, Y, and Furukawa, S. Autocrine activation of cultured macrophages by brain-derived neurotrophic factor. Biochem Biophys Res Commun. (2006) 344:941–7. doi: 10.1016/j.bbrc.2006.03.228
9. Charlton, T, Prowse, N, McFee, A, Heiratifar, N, Fortin, T, Paquette, C, et al. Brain-derived neurotrophic factor (BDNF) has direct anti-inflammatory effects on microglia. Front Cell Neurosci. (2023) 17:1188672. doi: 10.3389/fncel.2023.1188672
10. Miao, H, Li, R, Han, C, Lu, X, and Zhang, H. Minocycline promotes posthemorrhagic neurogenesis via M2 microglia polarization via upregulation of the TrkB/BDNF pathway in rats. J Neurophysiol. (2018) 120:1307–17. doi: 10.1152/jn.00234.2018
11. Miron, VE, Boyd, A, Zhao, J-W, Yuen, TJ, Ruckh, JM, Shadrach, JL, et al. M2 microglia and macrophages drive oligodendrocyte differentiation during CNS remyelination. Nat Neurosci. (2013) 16:1211–8. doi: 10.1038/nn.3469
12. Yu, H-C, Huang, H-B, Tseng, H, Hsien-Yu, A, and Lu, M-C. Brain-derived neurotrophic factor suppressed proinflammatory cytokines secretion and enhanced MicroRNA(miR)-3168 expression in macrophages. Int J Mol Sci. (2022) 23:570. doi: 10.3390/ijms23010570
13. Stadelmann, C, Kerschensteiner, M, Misgeld, T, Brück, W, Hohlfeld, R, and Lassmann, H. BDNF and gp145trkB in multiple sclerosis brain lesions: neuroprotective interactions between immune and neuronal cells? Brain. (2002) 125:75–85. doi: 10.1093/brain/awf015
14. Ksiazek-Winiarek, DJ, Szpakowski, P, and Glabinski, A. Neural plasticity in multiple sclerosis: the functional and molecular background. Neural Plast. (2015) 2015:307175. doi: 10.1155/2015/307175
15. Shajarian, M, Alsahebfosoul, F, and Etemadifar, M. The effect of IFN-β treatment on plasma levels of BDNF and IL-6 in relapsing-remitting multiple sclerosis patients. Neuroimmunomodulation. (2021) 28:150–7. doi: 10.1159/000515595
16. Yamamoto, H, and Gurney, ME. Human platelets contain brain-derived neurotrophic factor. J Neurosci. (1990) 10:3469–78. doi: 10.1523/JNEUROSCI.10-11-03469.1990
17. Cefis, M, Chaney, R, Quirié, A, Santini, C, Marie, C, Garnier, P, et al. Endothelial cells are an important source of BDNF in rat skeletal muscle. Sci Rep. (2022) 12:311. doi: 10.1038/s41598-021-03740-8
18. Guo, S, Kim, W, Lok, J, Lee, S-R, Besancon, E, Luo, B-H, et al. Neuroprotection via matrix-trophic coupling between cerebral endothelial cells and neurons. Proc Natl Acad Sci USA. (2008) 105:7582–7. doi: 10.1073/pnas.0801105105
19. Leventhal, C, Rafii, S, Rafii, D, Shahar, A, and Goldman, SA. Endothelial trophic support of neuronal production and recruitment from the adult mammalian subependyma. Mol Cell Neurosci. (1999) 13:450–64. doi: 10.1006/mcne.1999.0762
20. Bayas, A, Hummel, V, Kallmann, BA, Karch, C, Toyka, KV, and Rieckmann, P. Human cerebral endothelial cells are a potential source for bioactive BDNF. Cytokine. (2002) 19:55–8. doi: 10.1006/cyto.2002.0892
21. Fresegna, D, Bullitta, S, Musella, A, Rizzo, F, de Vito, F, Guadalupi, L, et al. Re-examining the role of TNF in MS pathogenesis and therapy. Cells. (2020) 9:e2290. doi: 10.3390/cells9102290
22. Brum, C, Stertz, L, Borba, E, Rumi, D, Kapczinski, F, and Camozzato, A. Association of serum brain-derived neurotrophic factor (BDNF) and tumor necrosis factor-alpha (TNF-α) with diagnosis of delirium in oncology inpatients. Revista brasileira de psiquiatria. (2015) 37:197–202. doi: 10.1590/1516-4446-2014-1450
23. Saha, RN, Liu, X, and Pahan, K. Up-regulation of BDNF in astrocytes by TNF-alpha: a case for the neuroprotective role of cytokine. J. Neuroimmune Pharmacol. (2006) 1:212–22. doi: 10.1007/s11481-006-9020-8
24. Rowhani-Rad, Soodeh, and Taherianfard, M. (2019): Crosstalk between BDNF and TNFα in brain versus serum of the cuprizone-induced multiple sclerosis in C57BL/6 mice. Physiology and Pharmacology. Online verfügbar unter, Available at: https://www.semanticscholar.org/paper/Crosstalk-between-BDNF-and-TNF%CE%B1-in-brain-versus-of-Rowhani-Rad-Taherianfard/31d392be49773c998d1d1d2bdf1ede062c982c20
25. Bałkowiec-Iskra, E, Vermehren-Schmaedick, A, and Balkowiec, A. Tumor necrosis factor-α increases brain-derived neurotrophic factor expression in trigeminal ganglion neurons in an activity-dependent manner. Neuroscience. (2011) 180:322–33. doi: 10.1016/j.neuroscience.2011.02.028
26. Marosi, K, and Mattson, MP. BDNF mediates adaptive brain and body responses to energetic challenges. Trends Endocrinol Metab. (2014) 25:89–98. doi: 10.1016/j.tem.2013.10.006
27. Kowiański, P, Lietzau, G, Czuba, E, Waśkow, M, Steliga, A, and Moryś, J. BDNF: a key factor with multipotent impact on brain Signaling and synaptic plasticity. Cell Mol Neurobiol. (2018) 38:579–93. doi: 10.1007/s10571-017-0510-4
28. Casaccia-Bonnefil, P, Gu, C, Khursigara, G, and Chao, MV. p75 neurotrophin receptor as a modulator of survival and death decisions. Microsc Res Tech. (1999) 45:217–24. doi: 10.1002/(SICI)1097-0029(19990515/01)45:4/5<217::AID-JEMT5>3.0.CO;2-5
29. Ma, D, Zhang, H, Yin, L, Xu, H, Wu, L, Shaji, R, et al. Human iPSC-derived endothelial cells promote CNS remyelination via BDNF and mTORC1 pathway. Glia. (2024) 72:133–55. doi: 10.1002/glia.24466
30. Chen, A, Xiong, L-J, Tong, Y, and Mao, M. Neuroprotective effect of brain-derived neurotrophic factor mediated by autophagy through the PI3K/Akt/mTOR pathway. Mol Med Rep. (2013) 8:1011–6. doi: 10.3892/mmr.2013.1628
31. An, X, Yao, X, Li, B, Yang, W, Cui, R, Zhao, G, et al. Role of BDNF-mTORC1 Signaling pathway in female depression. Neural Plast. (2021) 2021:6619515. doi: 10.1155/2021/6619515
32. Zhao, T, Qi, Y, Li, Y, and Xu, K. PI3 kinase regulation of neural regeneration and muscle hypertrophy after spinal cord injury. Mol Biol Rep. (2012) 39:3541–7. doi: 10.1007/s11033-011-1127-1
33. Akram, R, Anwar, H, Javed, M, Rasul, A, Imran, A, Malik, SA, et al. Axonal regeneration: underlying molecular mechanisms and potential therapeutic targets. Biomedicines. (2022) 10:123186. doi: 10.3390/biomedicines10123186
34. Wong, AW, Xiao, J, Kemper, D, Kilpatrick, TJ, and Murray, SS. Oligodendroglial expression of TrkB independently regulates myelination and progenitor cell proliferation. J Neurosci. (2013) 33:4947–57. doi: 10.1523/JNEUROSCI.3990-12.2013
35. Cheng, A, Wan, R, Yang, J-L, Kamimura, N, Son, T, Ouyang, X, et al. Involvement of PGC-1α in the formation and maintenance of neuronal dendritic spines. Nat Commun. (2012) 3:1250. doi: 10.1038/ncomms2238
36. Prokopova, B, Hlavacova, N, Vlcek, M, Penesova, A, Grunnerova, L, Garafova, A, et al. Early cognitive impairment along with decreased stress-induced BDNF in male and female patients with newly diagnosed multiple sclerosis. J Neuroimmunol. (2017) 302:34–40. doi: 10.1016/j.jneuroim.2016.11.007
37. Sarchielli, P, Greco, L, Stipa, A, Floridi, A, and Gallai, V. Brain-derived neurotrophic factor in patients with multiple sclerosis. J Neuroimmunol. (2002) 132:180–8. doi: 10.1016/s0165-5728(02)00319-3
38. Azoulay, D, Mausner-Fainberg, K, Urshansky, N, Fahoum, F, and Karni, A. Interferon-beta therapy up-regulates BDNF secretion from PBMCs of MS patients through a CD40-dependent mechanism. J Neuroimmunol. (2009) 211:114–9. doi: 10.1016/j.jneuroim.2009.04.004
39. Azoulay, D, Vachapova, V, Shihman, B, Miler, A, and Karni, A. Lower brain-derived neurotrophic factor in serum of relapsing remitting MS: reversal by glatiramer acetate. J Neuroimmunol. (2005) 167:215–8. doi: 10.1016/j.jneuroim.2005.07.001
40. Frota, ER, Rodrigues, DH, Donadi, EA, Brum, DG, Maciel, DRK, and Teixeira, AL. Increased plasma levels of brain derived neurotrophic factor (BDNF) after multiple sclerosis relapse. Neurosci Lett. (2009) 460:130–2. doi: 10.1016/j.neulet.2009.05.057
41. Lalive, PH, Kantengwa, S, Benkhoucha, M, Juillard, C, and Chofflon, M. Interferon-beta induces brain-derived neurotrophic factor in peripheral blood mononuclear cells of multiple sclerosis patients. J Neuroimmunol. (2008) 197:147–51. doi: 10.1016/j.jneuroim.2008.04.033
42. Lindquist, S, Hassinger, S, Lindquist, JA, and Sailer, M. The balance of pro-inflammatory and trophic factors in multiple sclerosis patients: effects of acute relapse and immunomodulatory treatment. Multiple Sclerosis. (2011) 17:851–66. doi: 10.1177/1352458511399797
43. Caggiula, M, Batocchi, A, Frisullo, G, Angelucci, F, Patanella, AK, Sancricca, C, et al. Neurotrophic factors in relapsing remitting and secondary progressive multiple sclerosis patients during interferon beta therapy. Clin. Immunol. (2006) 118:77–82. doi: 10.1016/j.clim.2005.09.005
44. Gielen, A, Khademi, M, Muhallab, S, Olsson, T, and Piehl, F. Increased brain-derived neurotrophic factor expression in white blood cells of relapsing-remitting multiple sclerosis patients. Scand J Immunol. (2003) 57:493–7. doi: 10.1046/j.1365-3083.2003.01260.x
45. Liguori, M, Fera, F, Patitucci, A, Manna, I, Condino, F, Valentino, P, et al. A longitudinal observation of brain-derived neurotrophic factor mRNA levels in patients with relapsing-remitting multiple sclerosis. Brain Res. (2009) 1256:123–8. doi: 10.1016/j.brainres.2008.11.047
46. Sarchielli, P, Zaffaroni, M, Floridi, A, Greco, L, Candeliere, A, Mattioni, A, et al. Production of brain-derived neurotrophic factor by mononuclear cells of patients with multiple sclerosis treated with glatiramer acetate, interferon-beta 1a, and high doses of immunoglobulins. Multiple Sclerosis. (2007) 13:313–31. doi: 10.1177/1352458506070146
47. Damasceno, A, Damasceno, B, Cendes, F, Damasceno, A, Moraes, AS, Farias, A, et al. Serum BDNF levels are not reliable correlates of neurodegeneration in MS patients. Mult Scler Relat Disord. (2015) 4:65–6. doi: 10.1016/j.msard.2014.11.003
48. Naegelin, Y, Saeuberli, K, Schaedelin, S, Dingsdale, H, Magon, S, Baranzini, S, et al. Levels of brain-derived neurotrophic factor in patients with multiple sclerosis. Ann. Clin. Trans. Neurol. (2020) 7:2251–61. doi: 10.1002/acn3.51215
49. Liguori, M, Fera, F, Gioia, MC, Valentino, P, Manna, I, Condino, F, et al. Investigating the role of brain-derived neurotrophic factor in relapsing-remitting multiple sclerosis. Genes Brain Behav. (2007) 6:177–83. doi: 10.1111/j.1601-183X.2006.00245.x
50. Vacaras, V, Major, ZZ, Muresanu, DF, Krausz, TL, Marginean, I, and Buzoianu, DA. Effect of glatiramer acetate on peripheral blood brain-derived neurotrophic factor and phosphorylated TrkB levels in relapsing-remitting multiple sclerosis. CNS Neurol Disord Drug Targets. (2014) 13:647–51. doi: 10.2174/1871527313666140618110049
51. Mehrpour, M, Akhoundi, FH, Delgosha, M, Keyvani, H, Motamed, MR, Sheibani, B, et al. Increased serum brain-derived neurotrophic factor in multiple sclerosis patients on interferon-β and its impact on functional abilities. Neurologist. (2015) 20:57–60. doi: 10.1097/NRL.0000000000000053
52. Weinstock-Guttman, B, Zivadinov, R, Tamaño-Blanco, M, Abdelrahman, N, Badgett, D, Durfee, J, et al. Immune cell BDNF secretion is associated with white matter volume in multiple sclerosis. J Neuroimmunol. (2007) 188:167–74. doi: 10.1016/j.jneuroim.2007.06.003
53. Maisonpierre, PC, le Beau, MM, Espinosa, R, Ip, NY, Belluscio, L, de la Monte, SM, et al. Human and rat brain-derived neurotrophic factor and neurotrophin-3: gene structures, distributions, and chromosomal localizations. Genomics. (1991) 10:558–68. doi: 10.1016/0888-7543(91)90436-i
54. Shen, T, You, Y, Joseph, C, Mirzaei, M, Klistorner, A, Graham, SL, et al. BDNF polymorphism: a review of its diagnostic and clinical relevance in neurodegenerative disorders. Aging Dis. (2018) 9:523–36. doi: 10.14336/AD.2017.0717
55. Egan, MF, Kojima, M, Callicott, JH, Goldberg, TE, Kolachana, BS, Bertolino, A, et al. The BDNF val66met polymorphism affects activity-dependent secretion of BDNF and human memory and hippocampal function. Cell. (2003) 112:257–69. doi: 10.1016/s0092-8674(03)00035-7
56. Chiaruttini, C, Vicario, A, Li, Z, Baj, G, Braiuca, P, Wu, Y, et al. Dendritic trafficking of BDNF mRNA is mediated by translin and blocked by the G196A (Val66Met) mutation. Proc Natl Acad Sci USA. (2009) 106:16481–6. doi: 10.1073/pnas.0902833106
57. Chen, Z-Y, Patel, PD, Sant, G, Meng, C-X, Teng, KK, Hempstead, BL, et al. Variant brain-derived neurotrophic factor (BDNF) (Met66) alters the intracellular trafficking and activity-dependent secretion of wild-type BDNF in neurosecretory cells and cortical neurons. J Neurosci. (2004) 24:4401–11. doi: 10.1523/JNEUROSCI.0348-04.2004
58. Dinacci, D, Tessitore, A, Russo, A, de Bonis, ML, Lavorgna, L, Picconi, O, et al. BDNF Val66Met polymorphism and brain volumes in multiple sclerosis. Neurol. Sci. (2011) 32:117–23. doi: 10.1007/s10072-010-0433-z
59. Hamamcioglu, K, and Reder, AT. Interferon-beta regulates cytokines and BDNF: greater effect in relapsing than in progressive multiple sclerosis. Multiple sclerosis (Houndmills, Basingstoke, England). (2007) 13:459–70. doi: 10.1177/1352458506069672
60. Ramasamy, DP, Ramanathan, M, Cox, JL, Antulov, R, Weinstock-Guttman, B, Bergsland, N, et al. Effect of Met66 allele of the BDNF rs6265 SNP on regional gray matter volumes in patients with multiple sclerosis: a voxel-based morphometry study. Pathophysiology. (2011) 18:53–60. doi: 10.1016/j.pathophys.2010.04.006
61. Chen, Z-Y, Jing, D, Bath, KG, Ieraci, A, Khan, T, Siao, C-J, et al. Genetic variant BDNF (Val66Met) polymorphism alters anxiety-related behavior. Science. (2006) 314:140–3. doi: 10.1126/science.1129663
62. Lang, UE, Hellweg, R, Sander, T, and Gallinat, J. The met allele of the BDNF Val66Met polymorphism is associated with increased BDNF serum concentrations. Mol Psychiatry. (2009) 14:120–2. doi: 10.1038/mp.2008.80
63. Minelli, A, Zanardini, R, Bonvicini, C, Sartori, R, Pedrini, L, Gennarelli, M, et al. BDNF serum levels, but not BDNF Val66Met genotype, are correlated with personality traits in healthy subjects. Eur Arch Psychiatry Clin Neurosci. (2011) 261:323–9. doi: 10.1007/s00406-011-0189-3
64. Ozan, E, Okur, H, Eker, C, Eker, OD, Gönül, A, and Akarsu, N. The effect of depression, BDNF gene val66met polymorphism and gender on serum BDNF levels. Brain Res Bull. (2010) 81:61–5. doi: 10.1016/j.brainresbull.2009.06.022
65. Yoshimura, R, Kishi, T, Suzuki, A, Umene-Nakano, W, Ikenouchi-Sugita, A, Hori, H, et al. The brain-derived neurotrophic factor (BDNF) polymorphism Val66Met is associated with neither serum BDNF level nor response to selective serotonin reuptake inhibitors in depressed Japanese patients. Prog Neuro-Psychopharmacol Biol Psychiatry. (2011) 35:1022–5. doi: 10.1016/j.pnpbp.2011.02.009
66. Zhou, Z, Lu, T, Xu, G, Yue, X, Zhu, W, Ma, M, et al. Decreased serum brain-derived neurotrophic factor (BDNF) is associated with post-stroke depression but not with BDNF gene Val66Met polymorphism. Clin Chem Lab Med. (2011) 49:185–9. doi: 10.1515/CCLM.2011.039
67. Hashimoto, T, and Lewis, DA. BDNF Val66Met polymorphism and GAD67 mRNA expression in the prefrontal cortex of subjects with schizophrenia. Am J Psychiatry. (2006) 163:534–7. doi: 10.1176/appi.ajp.163.3.534
68. Tramontina, J, Frey, BN, Andreazza, AC, Zandona, M, Santin, A, and Kapczinski, F. Val66met polymorphism and serum brain-derived neurotrophic factor levels in bipolar disorder. Mol Psychiatry. (2007) 12:230–1. doi: 10.1038/sj.mp.4001941
69. Amidfar, M, de Oliveira, J, Kucharska, E, Budni, J, and Kim, Y-K. The role of CREB and BDNF in neurobiology and treatment of Alzheimer’s disease. Life Sci. (2020) 257:118020. doi: 10.1016/j.lfs.2020.118020
70. Islam, O, Loo, TX, and Heese, K. Brain-derived neurotrophic factor (BDNF) has proliferative effects on neural stem cells through the truncated TRK-B receptor, MAP kinase, AKT, and STAT-3 signaling pathways. Curr Neurovasc Res. (2009) 6:42–53. doi: 10.2174/156720209787466028
71. Leal, G., Bramham, C. R., and Duarte, C. B. (2017): Chapter 8—BDNF and hippocampal synaptic plasticity. Gerald Litwack (Ed.), Vitamins and hormones: Neurotrophins, Academic Press, 153–195
72. Seidler, K, and Barrow, M. Intermittent fasting and cognitive performance - targeting BDNF as potential strategy to optimise brain health. Front Neuroendocrinol. (2022) 65:100971. doi: 10.1016/j.yfrne.2021.100971
73. Loprinzi, PD . Does brain-derived neurotrophic factor mediate the effects of exercise on memory? Phys Sportsmed. (2019) 47:395–405. doi: 10.1080/00913847.2019.1610255
74. Dincheva, I, Lynch, NB, and Lee, FS. The role of BDNF in the development of fear learning. Depress Anxiety. (2016) 33:907–16. doi: 10.1002/da.22497
75. Linnarsson, S, Björklund, A, and Ernfors, P. Learning deficit in BDNF mutant mice. Eur J Neurosci. (1997) 9:2581–7. doi: 10.1111/j.1460-9568.1997.tb01687.x
76. Vigers, AJ, Amin, DS, Talley-Farnham, T, Gorski, JA, Xu, B, and Jones, KR. Sustained expression of brain-derived neurotrophic factor is required for maintenance of dendritic spines and normal behavior. Neuroscience. (2012) 212:1–18. doi: 10.1016/j.neuroscience.2012.03.031
77. Patanella, AK, Zinno, M, Quaranta, D, Nociti, V, Frisullo, G, Gainotti, G, et al. Correlations between peripheral blood mononuclear cell production of BDNF, TNF-alpha, IL-6, IL-10 and cognitive performances in multiple sclerosis patients. J Neurosci Res. (2010) 88:1106–12. doi: 10.1002/jnr.22276
78. Hulst, HE, Schoonheim, MM, Roosendaal, SD, Popescu, V, Schweren, LJS, van der Werf, YD, et al. Functional adaptive changes within the hippocampal memory system of patients with multiple sclerosis. Hum Brain Mapp. (2012) 33:2268–80. doi: 10.1002/hbm.21359
79. Kern, KC, Ekstrom, AD, Suthana, NA, Giesser, BS, Montag, M, Arshanapalli, A, et al. Fornix damage limits verbal memory functional compensation in multiple sclerosis. NeuroImage. (2012) 59:2932–40. doi: 10.1016/j.neuroimage.2011.09.071
80. Greenberg, ME, Xu, B, Lu, B, and Hempstead, BL. New insights in the biology of BDNF synthesis and release: implications in CNS function. J Neurosci. (2009) 29:12764–7. doi: 10.1523/JNEUROSCI.3566-09.2009
81. Hariri, AR, Goldberg, TE, Mattay, VS, Kolachana, BS, Callicott, JH, Egan, MF, et al. Brain-derived neurotrophic factor val66met polymorphism affects human memory-related hippocampal activity and predicts memory performance. J Neurosci. (2003) 23:6690–4. doi: 10.1523/JNEUROSCI.23-17-06690.2003
82. Lu, B . BDNF and activity-dependent synaptic modulation. Learn Memory. (2003) 10:86–98. doi: 10.1101/lm.54603
83. Ninan, I, Bath, KG, Dagar, K, Perez-Castro, R, Plummer, MR, et al. The BDNF Val66Met polymorphism impairs NMDA receptor-dependent synaptic plasticity in the hippocampus. J Neurosci. (2010) 30:8866–70. doi: 10.1523/JNEUROSCI.1405-10.2010
84. Castrén, E, Pitkänen, M, Sirviö, J, Parsadanian, A, Lindholm, D, Thoenen, H, et al. The induction of LTP increases BDNF and NGF mRNA but decreases NT-3 mRNA in the dentate gyrus. Neuroreport. (1993) 4:895–8. doi: 10.1097/00001756-199307000-00014
85. Falkenberg, T, Mohammed, AK, Henriksson, B, Persson, H, Winblad, B, and Lindefors, N. Increased expression of brain-derived neurotrophic factor mRNA in rat hippocampus is associated with improved spatial memory and enriched environment. Neurosci Lett. (1992) 138:153–6. doi: 10.1016/0304-3940(92)90494-r
86. Yalachkov, Y, Anschütz, V, Maiworm, M, Jakob, J, Schaller-Paule, MA, Schäfer, JH, et al. Serum and cerebrospinal fluid BDNF concentrations are associated with neurological and cognitive improvement in multiple sclerosis: a pilot study. Mult Scler Relat Disord. (2023) 71:104567. doi: 10.1016/j.msard.2023.104567
87. Engin, E., Żarnowska, E., Sigal, M., Keist, R., Zeller, A., Pearce, R., et al., (2013): Alpha5-containing GABAA receptors in dentate gyrus enable cognitive flexibility. FASEB J. Online verfügbar unter, Available at: https://www.semanticscholar.org/paper/Alpha5%E2%80%90containing-GABAA-receptors-in-dentate-gyrus-Engin-%C5%BBarnowska/2ee0614f381d68b821e698e92403e16659a7ead6
88. Håkansson, K, Ledreux, A, Daffner, K, Terjestam, Y, Bergman, P, Carlsson, R, et al. BDNF responses in healthy older persons to 35 minutes of physical exercise, cognitive training, and mindfulness: associations with working memory function. J Alzheimer’s Disease. (2017) 55:645–57. doi: 10.3233/JAD-160593
89. Song, J-H, Yu, J-T, and Tan, L. Brain-derived neurotrophic factor in Alzheimer’s disease: risk, mechanisms, and therapy. Mol Neurobiol. (2015) 52:1477–93. doi: 10.1007/s12035-014-8958-4
90. Giordano, A, Clarelli, F, Cannizzaro, M, Mascia, E, Santoro, S, Sorosina, M, et al. BDNF Val66Met polymorphism is associated with motor recovery after rehabilitation in progressive multiple sclerosis patients. Front Neurol. (2022) 13:790360. doi: 10.3389/fneur.2022.790360
91. Cerasa, A, Tongiorgi, E, Fera, F, Gioia, MC, Valentino, P, Liguori, M, et al. The effects of BDNF Val66Met polymorphism on brain function in controls and patients with multiple sclerosis: an imaging genetic study. Behav Brain Res. (2010) 207:377–86. doi: 10.1016/j.bbr.2009.10.022
92. Fera, F, Passamonti, L, Cerasa, A, Gioia, M, Liguori, M, Manna, I, et al. The BDNF Val66Met polymorphism has opposite effects on memory circuits of multiple sclerosis patients and controls. PLoS One. (2013) 8:e61063. doi: 10.1371/journal.pone.0061063
93. Kambeitz, JP, Bhattacharyya, S, Kambeitz-Ilankovic, LM, Valli, I, Collier, DA, and McGuire, P. Effect of BDNF val(66)met polymorphism on declarative memory and its neural substrate: a meta-analysis. Neurosci Biobehav Rev. (2012) 36:2165–77. doi: 10.1016/j.neubiorev.2012.07.002
94. Mero, I-L, Smestad, C, Lie, BA, Lorentzen, ÅR, Sandvik, L, Landrø, NI, et al. Polymorphisms of the BDNF gene show neither association with multiple sclerosis susceptibility nor clinical course. J Neuroimmunol. (2012) 244:107–10. doi: 10.1016/j.jneuroim.2012.01.011
95. Ehling, R, di Pauli, F, Lackner, P, Rainer, C, Kraus, V, Hegen, H, et al. Impact of glatiramer acetate on paraclinical markers of neuroprotection in multiple sclerosis: a prospective observational clinical trial. J Neuroimmunol. (2015) 287:98–105. doi: 10.1016/j.jneuroim.2015.08.004
96. Islas-Hernandez, A, Aguilar-Talamantes, H, Bertado-Cortes, B, Mejia-delCastillo, G d J, Carrera-Pineda, R, Cuevas-Garcia, CF, et al. BDNF and tau as biomarkers of severity in multiple sclerosis. Biomark Med. (2018) 12:717–26. doi: 10.2217/bmm-2017-0374
97. Lindquist, S, Schott, BH, Ban, M, Compston, DAS, Sawcer, S, and Sailer, M. The BDNF-Val66Met polymorphism: implications for susceptibility to multiple sclerosis and severity of disease. J Neuroimmunol. (2005) 167:183–5. doi: 10.1016/j.jneuroim.2005.06.008
98. Blanco, Y, Gómez-Choco, M, Arostegui, JL, Casanova, B, Martínez-Rodríguez, JE, Boscá, I, et al. No association of the Val66Met polymorphism of brain-derived neurotrophic factor (BDNF) to multiple sclerosis. Neurosci Lett. (2006a) 396:217–9. doi: 10.1016/j.neulet.2005.11.032
99. Nociti, V, Santoro, M, Quaranta, D, Losavio, F, de Fino, C, Giordano, R, et al. BDNF rs6265 polymorphism methylation in multiple sclerosis: a possible marker of disease progression. PLoS One. (2018) 13:e0206140. doi: 10.1371/journal.pone.0206140
100. Portaccio, E, Bellinvia, A, Prestipino, E, Nacmias, B, Bagnoli, S, Razzolini, L, et al. The brain-derived neurotrophic factor Val66Met polymorphism can protect against cognitive impairment in multiple sclerosis. Front Neurol. (2021) 12:645220. doi: 10.3389/fneur.2021.645220
101. Mirowska-Guzel, D, Mach, A, Gromadzka, G, Czlonkowski, A, and Czlonkowska, A. BDNF A196G and C270T gene polymorphisms and susceptibility to multiple sclerosis in the polish population. Gender differences. J Neuroimmunol. (2008) 193:170. doi: 10.1016/j.jneuroim.2007.10.013
102. Comini-Frota, ER, Rodrigues, DH, Miranda, EC, Brum, DG, Kaimen-Maciel, DR, Donadi, EA, et al. Serum levels of brain-derived neurotrophic factor correlate with the number of T2 MRI lesions in multiple sclerosis. Braz. J. Med. Biol. Res. (2012) 45:68–71. doi: 10.1590/s0100-879x2011007500165
103. Brod, SA, Lincoln, JA, and Nelson, F. Myelinating proteins in MS are linked to volumetric brain MRI changes. J. Neuroimaging. (2019) 29:400–5. doi: 10.1111/jon.12605
104. Conner, JM, Lauterborn, JC, Yan, Q, Gall, CM, and Varon, S. Distribution of brain-derived neurotrophic factor (BDNF) protein and mRNA in the normal adult rat CNS: evidence for anterograde axonal transport. J Neurosci. (1997) 17:e2295. doi: 10.1523/JNEUROSCI.17-07-02295.1997
105. Zachary, C, Gorski, JA, and Jones, KR. Early striatal dendrite deficits followed by neuron loss with advanced age in the absence of anterograde cortical brain-derived neurotrophic factor. J Neurosci. (2004) 24:4250–8. doi: 10.1523/JNEUROSCI.3920-03.2004
106. Charil, A, Dagher, A, Lerch, JP, Zijdenbos, AP, Worsley, KJ, and Evans, AC. Focal cortical atrophy in multiple sclerosis: relation to lesion load and disability. NeuroImage. (2007) 34:e6. doi: 10.1016/j.neuroimage.2006.10.006
107. Prinster, A, Quarantelli, M, Orefice, G, Lanzillo, R, Brunetti, A, Mollica, C, et al. Grey matter loss in relapsing–remitting multiple sclerosis: a voxel-based morphometry study. NeuroImage. (2006) 29:859–67. doi: 10.1016/j.neuroimage.2005.08.034
108. Dolcetti, E, Bruno, A, Azzolini, F, Gilio, L, Moscatelli, A, de Vito, F, et al. The BDNF Val66Met polymorphism (rs6265) modulates inflammation and neurodegeneration in the early phases of multiple sclerosis. Genes. (2022) 13. doi: 10.3390/genes13020332
109. de Meo, E, Portaccio, E, Prestipino, E, Nacmias, B, Bagnoli, S, Razzolini, L, et al. Effect of BDNF Val66Met polymorphism on hippocampal subfields in multiple sclerosis patients. Mol Psychiatry. (2022) 27:1010–9. doi: 10.1038/s41380-021-01345-1
110. Blanco, Y, Moral, EA, Costa, M, Gómez-Choco, M, Torres-Peraza, JF, Alonso-Magdalena, L, et al. Effect of glatiramer acetate (Copaxone) on the immunophenotypic and cytokine profile and BDNF production in multiple sclerosis: a longitudinal study. Neurosci Lett. (2006b) 406:270–5. doi: 10.1016/j.neulet.2006.07.043
111. Chen, M, Valenzuela, R, and Dhib-Jalbut, S. Glatiramer acetate-reactive T cells produce brain-derived neurotrophic factor. J Neurol Sci. (2003) 215:37–44. doi: 10.1016/s0022-510x(03)00177-1
112. Ziemssen, T, Kümpfel, T, Schneider, H, Klinkert, WEF, Neuhaus, O, and Hohlfeld, R. Secretion of brain-derived neurotrophic factor by glatiramer acetate-reactive T-helper cell lines: implications for multiple sclerosis therapy. J Neurol Sci. (2005) 233:109. doi: 10.1016/j.jns.2005.03.010
113. Ruggieri, M, Avolio, C, Livrea, P, and Trojano, M. Glatiramer acetate in multiple sclerosis: a review. CNS Drug Rev. (2007) 13:178–91. doi: 10.1111/j.1527-3458.2007.00010.x
114. Arnon, R, and Aharoni, R. Mechanism of action of glatiramer acetate in multiple sclerosis and its potential for the development of new applications. Proc Natl Acad Sci USA. (2004) 101:14593–8. doi: 10.1073/pnas.0404887101
115. Ziemssen, T, and Schrempf, W. Glatiramer acetate: mechanisms of action in multiple sclerosis. Int Rev Neurobiol. (2007) 79:537–70. doi: 10.1016/S0074-7742(07)79024-4
116. Aharoni, R, Eilam, R, Domev, H, Labunskay, G, Sela, M, and Arnon, R. The immunomodulator glatiramer acetate augments the expression of neurotrophic factors in brains of experimental autoimmune encephalomyelitis mice. Proc Natl Acad Sci USA. (2005) 102:19045–50. doi: 10.1073/pnas.0509438102
117. Aharoni, R, Kayhan, B, Eilam, R, Sela, M, and Arnon, R. Glatiramer acetate-specific T cells in the brain express T helper 2/3 cytokines and brain-derived neurotrophic factor in situ. Proc Natl Acad Sci USA. (2003) 100:14157–62. doi: 10.1073/pnas.2336171100
118. Ziemssen, T, Kümpfel, T, Klinkert, WEF, Neuhaus, O, and Hohlfeld, R. Glatiramer acetate-specific T-helper 1- and 2-type cell lines produce BDNF: implications for multiple sclerosis therapy. Brain-derived neurotrophic factor. Brain. (2002) 125:2381–91. doi: 10.1093/brain/awf252
119. Gilgun-Sherki, Y, Panet, H, Holdengreber, V, Mosberg-Galili, R, and Offen, D. Axonal damage is reduced following glatiramer acetate treatment in C57/bl mice with chronic-induced experimental autoimmune encephalomyelitis. Neurosci Res. (2003) 47:201–7. doi: 10.1016/s0168-0102(03)00217-7
120. Lee, D-H, Geyer, E, Flach, A-C, Jung, K, Gold, R, Flügel, A, et al. Central nervous system rather than immune cell-derived BDNF mediates axonal protective effects early in autoimmune demyelination. Acta Neuropathol. (2012) 123:247–58. doi: 10.1007/s00401-011-0890-3
121. Golan, M, Mausner-Fainberg, K, Ibrahim, B, Benhamou, M, Wilf-Yarkoni, A, Kolb, H, et al. Fingolimod increases brain-derived neurotrophic factor level secretion from circulating T cells of patients with multiple sclerosis. CNS Drugs. (2019) 33:1229–37. doi: 10.1007/s40263-019-00675-7
122. Petereit, HF, Lindemann, H, and Schoppe, S. Effect of immunomodulatory drugs on in vitro production of brain-derived neurotrophic factor. Multiple Sclerosis. (2003) 9:16–20. doi: 10.1191/1352458503ms869oa
123. Kalinowska-Łyszczarz, A, Pawlak, MA, Wyciszkiewicz, A, Osztynowicz, K, Kozubski, W, and Michalak, S. Immune-cell BDNF expression in treatment-naïve relapsing-remitting multiple sclerosis patients and following one year of immunomodulation therapy. Neurol Neurochir Pol. (2018) 52:483–9. doi: 10.1016/j.pjnns.2018.03.006
124. Smith, PA, Schmid, C, Zurbruegg, S, Jivkov, M, Doelemeyer, A, Theil, D, et al. Fingolimod inhibits brain atrophy and promotes brain-derived neurotrophic factor in an animal model of multiple sclerosis. J Neuroimmunol. (2018) 318:103–13. doi: 10.1016/j.jneuroim.2018.02.016
125. Văcăraş, V, Major, Z, Buzoianu, AD, Zsigmond, A, and Dana, BA. Brain-derived neurotrophic factor levels under chronic natalizumab treatment in multiple sclerosis. A preliminary report. Neurol Neurochir Pol. (2017) 51:221–6. doi: 10.1016/j.pjnns.2017.03.002
126. Muraro, PA, Douek, DC, Packer, A, Chung, K, Guenaga, FJ, Cassiani-Ingoni, R, et al. Thymic output generates a new and diverse TCR repertoire after autologous stem cell transplantation in multiple sclerosis patients. J Exp Med. (2005) 201:805–16. doi: 10.1084/jem.20041679
127. Blanco, Y, Saiz, A, Costa, M, Torres-Peraza, JF, Carreras, E, Alberch, J, et al. Evolution of brain-derived neurotrophic factor levels after autologous hematopietic stem cell transplantation in multiple sclerosis. Neurosci Lett. (2005) 380:122–6. doi: 10.1016/j.neulet.2005.01.032
128. Giedraitiene, N, Gasciauskaite, G, and Kaubrys, G. Impact of autologous HSCT on the quality of life and fatigue in patients with relapsing multiple sclerosis. Sci Rep. (2022) 12:15404. doi: 10.1038/s41598-022-19748-7
129. Mancardi, GL, Sormani, MP, Di Gioia, M, Vuolo, L, Gualandi, F, Amato, MP, et al. Autologous haematopoietic stem cell transplantation with an intermediate intensity conditioning regimen in multiple sclerosis: the Italian multi-Centre experience. Multiple Sclerosis. (2012) 18:835–42. doi: 10.1177/1352458511429320
130. Burt, RK, Balabanov, R, Burman, J, Sharrack, B, Snowden, JA, Oliveira, MC, et al. Effect of nonmyeloablative hematopoietic stem cell transplantation vs continued disease-modifying therapy on disease progression in patients with relapsing-remitting multiple sclerosis: a randomized clinical trial. JAMA. (2019) 321:165–74. doi: 10.1001/jama.2018.18743
131. Dalgas, U, Langeskov-Christensen, M, Stenager, E, Riemenschneider, M, and Hvid, LG. Exercise as medicine in multiple sclerosis—time for a paradigm shift: preventive, symptomatic, and disease-modifying aspects and perspectives. Curr Neurol Neurosci Rep. (2019) 19:88. doi: 10.1007/s11910-019-1002-3
132. Begliuomini, S, Lenzi, E, Ninni, F, Casarosa, E, Merlini, S, Pluchino, N, et al. Plasma brain-derived neurotrophic factor daily variations in men: correlation with cortisol circadian rhythm. J Endocrinol. (2008) 197:429–35. doi: 10.1677/JOE-07-0376
133. Lu, Y, Christian, K, and Lu, B. BDNF: a key regulator for protein synthesis-dependent LTP and long-term memory? Neurobiol Learn Mem. (2008) 89:312–23. doi: 10.1016/j.nlm.2007.08.018
134. Thoenen, H . Neurotrophins and neuronal plasticity. Science. (1995) 270:593–8. doi: 10.1126/science.270.5236.593
135. Pedersen, BK . Physical activity and muscle-brain crosstalk. Nat Rev Endocrinol. (2019) 15:383–92. doi: 10.1038/s41574-019-0174-x
136. Sakuma, K, and Yamaguchi, A. The recent understanding of the neurotrophin’s role in skeletal muscle adaptation. J Biomed Biotechnol. (2011) 2011:201696. doi: 10.1155/2011/201696
137. Wrann, CD, White, JP, Salogiannnis, J, Laznik-Bogoslavski, D, Wu, J, Ma, D, et al. Exercise induces hippocampal BDNF through a PGC-1α/FNDC5 pathway. Cell Metab. (2013) 18:649–59. doi: 10.1016/j.cmet.2013.09.008
138. Gentile, A, Musella, A, de Vito, F, Rizzo, FR, Fresegna, D, Bullitta, S, et al. Immunomodulatory effects of exercise in experimental multiple sclerosis. Front Immunol. (2019) 10:2197. doi: 10.3389/fimmu.2019.02197
139. Guo, L, Lozinski, B, and Yong, VW. Exercise in multiple sclerosis and its models: focus on the central nervous system outcomes. J Neurosci Res. (2020) 98:509–23. doi: 10.1002/jnr.24524
140. Vaynman, S, Ying, Z, and Gomez-Pinilla, F. The select action of hippocampal calcium calmodulin protein kinase II in mediating exercise-enhanced cognitive function. Neuroscience. (2007) 144:825–33. doi: 10.1016/j.neuroscience.2006.10.005
141. Jørgensen, MLK, Kjølhede, T, Dalgas, U, and Hvid, LG. Plasma brain-derived neurotrophic factor (BDNF) and sphingosine-1-phosphat (S1P) are NOT the main mediators of neuroprotection induced by resistance training in persons with multiple sclerosis-a randomized controlled trial. Mult Scler Relat Disord. (2019) 31:106–11. doi: 10.1016/j.msard.2019.03.029
142. Abbaspoor, E, Zolfaghari, M, Ahmadi, B, and Khodaei, K. The effect of combined functional training on BDNF, IGF-1, and their association with health-related fitness in the multiple sclerosis women. J. Growth Hormone Res. Soc. Int. IGF Res. Soc. (2020) 52:101320. doi: 10.1016/j.ghir.2020.101320
143. Briken, S, Rosenkranz, S, Keminer, O, Patra, S, Ketels, G, Heesen, C, et al. Effects of exercise on Irisin, BDNF and IL-6 serum levels in patients with progressive multiple sclerosis. J Neuroimmunol. (2016) 299:53–8. doi: 10.1016/j.jneuroim.2016.08.007
144. Schulz, K-H, Gold, SM, Witte, J, Bartsch, K, Lang, UE, Hellweg, R, et al. Impact of aerobic training on immune-endocrine parameters, neurotrophic factors, quality of life and coordinative function in multiple sclerosis. J Neurol Sci. (2004) 225:11–8. doi: 10.1016/j.jns.2004.06.009
145. Waschbisch, A, Wenny, I, Tallner, A, Schwab, S, Pfeifer, K, and Mäurer, M. Physical activity in multiple sclerosis: a comparative study of vitamin D, brain-derived neurotrophic factor and regulatory T cell populations. Eur Neurol. (2012) 68:122–8. doi: 10.1159/000337904
146. Naghibzadeh, A, Mohammadi, G, Darlington, PJ, Rezaei Namjoo, F, and Rashidlamir, A. Aquatic exercise with Swedish massage increases neurotrophic factors and decreases interleukin-6 (IL-6) in relapsing remitting multiple sclerosis. Bio Exerc. (2019) 15:171–86.
147. Ozkul, C, Guclu-Gunduz, A, Irkec, C, Fidan, I, Aydin, Y, Ozkan, T, et al. Effect of combined exercise training on serum brain-derived neurotrophic factor, suppressors of cytokine signaling 1 and 3 in patients with multiple sclerosis. J Neuroimmunol. (2018) 316:121–9. doi: 10.1016/j.jneuroim.2018.01.002
148. Banitalebi, E, Ghahfarrokhi, M, Negaresh, R, Kazemi, A, Faramarzi, M, Motl, RW, et al. Exercise improves neurotrophins in multiple sclerosis independent of disability status. Mult Scler Relat Disord. (2020) 43:102143. doi: 10.1016/j.msard.2020.102143
149. Eftekhari, E, and Etemadifar, M. Interleukin-10 and brain-derived neurotrophic factor responses to the mat Pilates training in women with multiple sclerosis. Sci Med. (2018) 28:31668. doi: 10.15448/1980-6108.2018.4.31668
150. Khademosharie, M, Tadibi, V, Behpoor, N, and Hamedinia, MR. The effect of 12-weeks concurent training on the serum levels NGF, BDNF, and VDBP in women with multiple sclerosis (2018) 17:77–86. doi: 10.22631/ijaep.v7i1.228,
151. Mokhtarzade, M, Motl, R, Negaresh, R, Zimmer, P, Khodadoost, M, Baker, JS, et al. Exercise-induced changes in neurotrophic factors and markers of blood-brain barrier permeability are moderated by weight status in multiple sclerosis. Neuropeptides. (2018) 70:93–100. doi: 10.1016/j.npep.2018.05.010
152. Savšek, L, Stergar, T, Strojnik, V, Ihan, A, Koren, A, Špiclin, Ž, et al. Impact of aerobic exercise on clinical and magnetic resonance imaging biomarkers in persons with multiple sclerosis: an exploratory randomized controlled trial. J Rehabil Med. (2021) 53:jrm00178. doi: 10.2340/16501977-2814
153. Wens, I, Keytsman, C, Deckx, N, Cools, N, Dalgas, U, and Eijnde, BO. Brain derived neurotrophic factor in multiple sclerosis: effect of 24 weeks endurance and resistance training. Eur J Neurol. (2016) 23:1028–35. doi: 10.1111/ene.12976
154. Diechmann, MD, Campbell, E, Coulter, E, Paul, L, Dalgas, U, and Hvid, LG. Effects of exercise training on neurotrophic factors and subsequent neuroprotection in persons with multiple sclerosis-a systematic review and meta-analysis. Brain Sci. (2021) 11:111499. doi: 10.3390/brainsci11111499
155. Church, DD, Hoffman, JR, Mangine, GT, Jajtner, AR, Townsend, JR, Beyer, KS, et al. Comparison of high-intensity vs. high-volume resistance training on the BDNF response to exercise. J. Appl. Physiol. (2016) 121:123–8. doi: 10.1152/japplphysiol.00233.2016
156. Dinoff, A, Herrmann, N, Swardfager, W, and Lanctôt, KL. The effect of acute exercise on blood concentrations of brain-derived neurotrophic factor in healthy adults: a meta-analysis. Eur J Neurosci. (2017) 46:1635–46. doi: 10.1111/ejn.13603
157. Dinoff, A, Herrmann, N, Swardfager, W, Liu, CS, Sherman, C, Chan, S, et al. The effect of exercise training on resting concentrations of peripheral brain-derived neurotrophic factor (BDNF): a meta-analysis. PLoS One. (2016) 11:e0163037. doi: 10.1371/journal.pone.0163037
158. Ferris, LT, Williams, JS, and Shen, C-L. The effect of acute exercise on serum brain-derived neurotrophic factor levels and cognitive function. Med Sci Sports Exerc. (2007) 39:728–34. doi: 10.1249/mss.0b013e31802f04c7
159. Lippi, G, Mattiuzzi, C, and Sanchis-Gomar, F. Updated overview on interplay between physical exercise, neurotrophins, and cognitive function in humans. J Sport Health Sci. (2020) 9:74–81. doi: 10.1016/j.jshs.2019.07.012
160. Reycraft, JT, Islam, H, Townsend, LK, Hayward, GC, Hazell, TJ, and Macpherson, REK. Exercise intensity and recovery on circulating brain-derived neurotrophic factor. Med Sci Sports Exerc. (2020) 52:1210–7. doi: 10.1249/MSS.0000000000002242
161. Schmolesky, MT, Webb, DL, and Hansen, RA. The effects of aerobic exercise intensity and duration on levels of brain-derived neurotrophic factor in healthy men. J. Sports Sci. Med. (2013) 12:502–11.
162. Szuhany, KL, Bugatti, M, and Otto, MW. A meta-analytic review of the effects of exercise on brain-derived neurotrophic factor. J Psychiatr Res. (2015) 60:56–64. doi: 10.1016/j.jpsychires.2014.10.003
163. Zimmer, P, Bloch, W, Schenk, A, Oberste, M, Riedel, S, Kool, J, et al. High-intensity interval exercise improves cognitive performance and reduces matrix metalloproteinases-2 serum levels in persons with multiple sclerosis: a randomized controlled trial. Multiple Sclerosis. (2018) 24:1635–44. doi: 10.1177/1352458517728342
164. El-Sayes, J, Harasym, D, Turco, CV, Locke, MB, and Nelson, AJ. Exercise-induced neuroplasticity: a mechanistic model and prospects for promoting plasticity. Neuroscientist. (2019) 25:65–85. doi: 10.1177/1073858418771538
165. Bonfiglio, T, Olivero, G, Vergassola, M, Di Cesare Mannelli, L, Pacini, A, Iannuzzi, F, et al. Environmental training is beneficial to clinical symptoms and cortical presynaptic defects in mice suffering from experimental autoimmune encephalomyelitis. Neuropharmacology. (2019) 145:75–86. doi: 10.1016/j.neuropharm.2018.01.026
166. Mandolesi, G, Bullitta, S, Fresegna, D, de Vito, F, Rizzo, FR, Musella, A, et al. Voluntary running wheel attenuates motor deterioration and brain damage in cuprizone-induced demyelination. Neurobiol Dis. (2019) 129:102–17. doi: 10.1016/j.nbd.2019.05.010
167. Souza, PS, Gonçalves, ED, Pedroso, GS, Farias, HR, Junqueira, SC, Marcon, R, et al. Physical exercise attenuates experimental autoimmune encephalomyelitis by inhibiting peripheral immune response and blood-brain barrier disruption. Mol Neurobiol. (2017) 54:4723–37. doi: 10.1007/s12035-016-0014-0
168. Xie, Y, Li, Z, Wang, Y, Xue, X, Ma, W, Zhang, Y, et al. Effects of moderate- versus high- intensity swimming training on inflammatory and CD4+ T cell subset profiles in experimental autoimmune encephalomyelitis mice. J Neuroimmunol. (2019) 328:60–7. doi: 10.1016/j.jneuroim.2018.12.005
169. Tsuchimine, S, Sugawara, N, Ishioka, M, and Yasui-Furukori, N. Preanalysis storage conditions influence the measurement of brain-derived neurotrophic factor levels in peripheral blood. Neuropsychobiology. (2014) 69:83–8. doi: 10.1159/000358061
170. Gejl, A, Enevold, C, Bugge, A, Andersen, MS, Nielsen, CH, and Andersen, LB. Associations between serum and plasma brain-derived neurotrophic factor and influence of storage time and centrifugation strategy. Sci Rep. (2019) 9:9655. doi: 10.1038/s41598-019-45976-5
171. Wilson, DH, Rissin, DM, Kan, CW, Fournier, DR, Piech, T, Campbell, TG, et al. The Simoa HD-1 analyzer: a novel fully automated digital immunoassay analyzer with single-molecule sensitivity and multiplexing. J. Laborat. Autom. (2016) 21:533–47. doi: 10.1177/2211068215589580
172. Naegelin, Y, Dingsdale, H, Säuberli, K, Schädelin, S, Kappos, L, and Barde, Y-A. Measuring and validating the levels of brain-derived neurotrophic factor in human serum. eNeuro. (2018) 5:e419. doi: 10.1523/ENEURO.0419-17.2018
173. Nagahara, AH, and Tuszynski, MH. Potential therapeutic uses of BDNF in neurological and psychiatric disorders. Nat Rev Drug Discov. (2011) 10:209–19. doi: 10.1038/nrd3366
Glossary
Keywords: BDNF, brain-derived neurotrophic factor, multiple sclerosis, neuroplasticity, neuroprotection, remyelination, neuronal damage
Citation: Maiworm M (2024) The relevance of BDNF for neuroprotection and neuroplasticity in multiple sclerosis. Front. Neurol. 15:1385042. doi: 10.3389/fneur.2024.1385042
Edited by:
Eleonora Tavazzi, University at Buffalo, United StatesReviewed by:
Veronica Popescu, University of Hasselt, BelgiumCheryl F. Dreyfus, The State University of New Jersey, United States
Xavier Xifró, University of Girona, Spain
Jorge Matias-Guiu, Complutense University of Madrid, Spain
Copyright © 2024 Maiworm. This is an open-access article distributed under the terms of the Creative Commons Attribution License (CC BY). The use, distribution or reproduction in other forums is permitted, provided the original author(s) and the copyright owner(s) are credited and that the original publication in this journal is cited, in accordance with accepted academic practice. No use, distribution or reproduction is permitted which does not comply with these terms.
*Correspondence: Michelle Maiworm, TWFpd29ybUBtZWQudW5pLWZyYW5rZnVydC5kZQ==