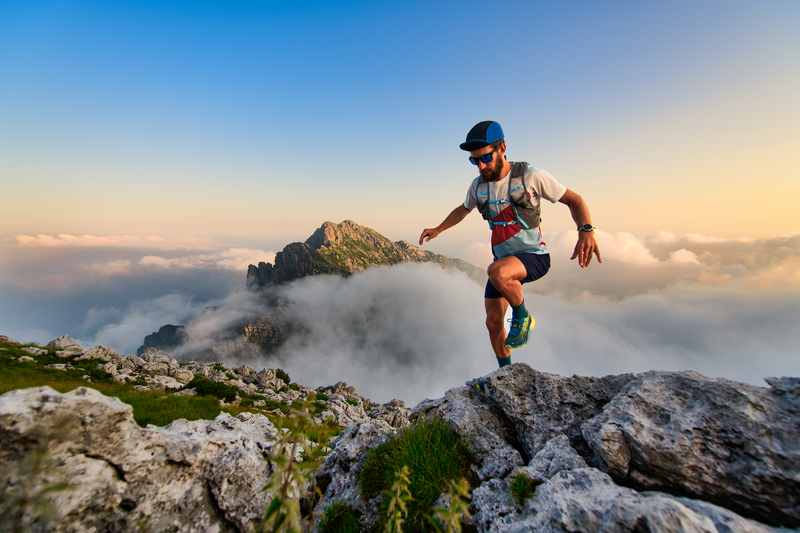
95% of researchers rate our articles as excellent or good
Learn more about the work of our research integrity team to safeguard the quality of each article we publish.
Find out more
MINI REVIEW article
Front. Neurol. , 14 February 2024
Sec. Neuro-Otology
Volume 15 - 2024 | https://doi.org/10.3389/fneur.2024.1361747
This article is part of the Research Topic Function and dysfunction of sensory hair cells and supporting cells View all 12 articles
In addition to hearing loss, damage to the cochlea can lead to gain of function pathologies such as hyperacusis. It has been proposed that painful hyperacusis, noxacusis, may be carried to the central nervous system by type II cochlear afferents, sparse, unmyelinated neurons that share morphological and neurochemical traits with nociceptive C-fibers of the somatic nervous system. Also like in skin, damage elicits spreading calcium waves within cochlear epithelia. These are mediated by extracellular ATP combined with IP3-driven release from intracellular calcium stores. Type II afferents are excited by ATP released from damaged epithelia. Thus, the genesis and propagation of epithelial calcium waves is central to cochlear pathology, and presumably hyperacusis. Damage-evoked signals in type II afferents and epithelial cells have been recorded in cochlear explants or semi-intact otic capsules. These efforts have included intracellular electrical recording, use of fluorescent calcium indicators, and visualization of an activity-dependent, intrinsic fluorescent signal. Of relevance to hyperacusis, prior noise-induced hearing loss leads to the generation of prolonged and repetitive activity in type II neurons and surrounding epithelia.
The mammalian cochlea has a problem. The evolution of sensitive hearing over a wide frequency range has produced a biomechanically complex sense organ, but one vulnerable to irreparable damage. Adding insult to injury, the associated pathology of hyperacusis can undermine quality of life as much or even more than partial hearing loss per se. Thus, efforts continue to identify the underlying pathological mechanisms of hyperacusis. A single row of inner hair cells and their associated type I afferents encode and transmit acoustic information to the brain. Three rows of outer hair cells are inhibited by cholinergic efferent neurons. A much smaller number (5% of the total) of unmyelinated type II afferents extend hundreds of microns along the cochlear length to contact dozens of outer hair cells. Previous work has examined the hypothesis that type II cochlear afferents may be not only acoustic sensors (1–3), but additionally respond to tissue damage as potential inner ear nociceptors (4–6). Their role in activation of the medial olivocochlear (MOC) neurons remains to be delineated fully (7–9), although type II afferents are unlikely to provide the dynamic range and frequency selectivity of the MOC efferents (10, 11). Of equal importance, it is known that local damage in cochlear explants can trigger intercellular calcium signals that propagate through surrounding epithelia (12, 13). These calcium waves may share features with the epithelial waves (14, 15) that modulate hair cell driven afferent activity (16–18) prior to the onset of hearing (19). This mini-review describes the propagation of damage-evoked epithelial calcium waves and relates them to the activity of type II cochlear afferents.
Experiments on cochlear damage can be informed by the extensive studies of neural and epithelial mechanisms of somatic pain (20). Relevant to this review are those that have examined the propagation of damage-evoked calcium waves in skin epithelia (21, 22) that modulate or activate pain-sensing C-fibers (20). A particularly informative in vivo study was carried out on earlobe skin of mice expressing a genetically-encoded calcium indicator (23). Calcium waves triggered by laser ablation of single keratinocytes were recorded, subjected to pharmacological blockade and used to inform a model of propagation. This model combined ATP release through connexin hemi-channels with IP3-evoked release of calcium from internal stores. The model accurately described experimentally-observed calcium waves whose velocity fell with time and distance from the site of lesion, with initial speeds averaging ~25 μm/s, then falling to zero within ~13 s elapsed time, or 70 μm traveled. Calcium imaging also has been used to characterize damage-evoked epithelial waves in cochlear explants (12, 13, 24, 25). As in skin, these intercellular calcium waves depend on released ATP as an extracellular signal, and IP3-dependent release of calcium from cytoplasmic stores (26). Similarly to those in living skin, ex vivo cochlear calcium waves slow from an initial velocity of 10–15 μm/s with distance from the site of lesion (13, 24). These commonalities suggest that cochlear epithelial waves may activate type II afferent neurons to drive painful percepts, by analogy to the role of C-fibers in skin. Thus it would be informative to record damage-evoked activity simultaneously in both epithelial and type II afferents in cochlear explants.
Type II cochlear afferents have small caliber, unmyelinated processes that extend hundreds of microns toward the cochlear base, contacting dozens of outer hair cells enroute (27–29). Type IIs make up only a small fraction (~5%) of cochlear afferents, the great majority being myelinated type I afferents postsynaptic to inner hair cells (30, 31). Given the scarcity and small size of type II afferents, it is not surprising that only few extracellular recordings have been obtained in vivo, showing limited acoustic sensitivity (2, 3); although c-FOS labeling of brainstem targets of type II afferents did find activity induced by loud, non-damaging, sound (1). Whatever the acoustic sensitivity of type II afferents, they must be far less able to support discriminative hearing than type I afferents whose activity accounts for acoustic intensity, frequency and timing (32). Intracellular tight-seal recordings from type II afferents in cochlear explants revealed that glutamatergic transmission from one OHC was orders of magnitude weaker than that provided by each IHC to type I afferent ribbon synapse (4, 33, 34). But in addition, type II afferents are strongly depolarized by direct application of ATP (4), a known algogenic mediator in skin (35). Acute mechanical damage to OHCs (produced by a glass probe) caused a large, long-lasting depolarization of the type II afferent that could trigger bursts of action potentials (5). The underlying inward current was ATP and connexin dependent and resulted from the activation of both P2X and P2Y purinergic receptors. The proposed hypothesis was that ATP released from surrounding supporting cells activated the type II afferent. Testing this hypothesis further requires a tissue-wide method for recording type II activity from mature animals.
Dissection damage and tissue degradation make intracellular recording from type II afferents limited largely to explants from the apical turn of early postnatal cochleas. Thus, subsequent studies have turned to fluorescence imaging in semi-intact cochlear capsules (‘half-shells’) (24). Using multi-photon microscopy, it is possible to interrogate any region of cochleas removed from adult mice (36). Several transgenic mouse lines have been developed to provide expression of the calcium indicator GCaMP6f in type II cochlear afferents (37–39). Calcium indicators can be expressed in apical type II afferents employing a tyrosine hydroxylase promoter (39). The dopamine type 2 receptor promoter functions preferentially in basal type II afferents (38). A third line utilizing the Tac1 promoter drives expression in type II afferents throughout the cochlea, combined with a tonotopic gradient of expression in epithelial cells (36). For the velocity measurements described below, the floxed GCaMP6f mouse (C57BL/6N-Gt(ROSA)26Sortm1(CAG-GCaMP6f)Khakh/J IMSR catalog #JAX:029626, RRID:IMSR_JAX:029626) was crossed with a tyrosine hydroxylase promoter Cre-recombinase mouse, Th2ACreEr (40). Following laser ablation of one to three OHCs, type II afferents had increased GCAMP6f fluorescence that decayed over 40–50 s, similar to the time course of damage-evoked inward current observed in tight-seal, whole-cell recordings (5). This time course also is similar to that of calcium signals evoked in neonatal cochlear and vestibular tissue by pulsed application of ACh or ATP (41). The ATP receptor antagonist PPADs reduced the spatial spread of the type II afferent GCaMP6f response, accounting for the reduced duration of ATP-evoked current observed in voltage clamp of type II afferents (5) (i.e., less delayed excitation from distant regions). These observations are consistent with an hypothesis whereby ATP released during damage-evoked epithelial calcium waves drives the type II afferent response.
How does the type II afferent response relate to previously described epithelial calcium waves? It is not possible to answer this question by GCaMP expression alone, since epithelial cell signals would obscure the type II neuron’s response (but see Tac1 below). Fortunately, there is a much weaker but still visible damage-evoked fluorescence signal in epithelia not expressing GCaMP (as confirmed by negative immunolabeling, and presence in mice without GCaMP expression) that could serve as an indicator of epithelial damage. The possible genesis of Non-GCaMP Associated Fluorescence (NGAF) will be discussed below (36). The validity of NGAF measures was supported by the Tac1 mouse model that drives GCaMP expression in most, if not all type II neurons, but in a declining gradient of epithelial cells from apex to base, providing direct comparison of GCaMP fluorescence from type II afferents and NGAF in epithelial cells stochastically-expressing GCaMP in middle cochlear segments. These showed similar durations for the neuronal/epithelial GCaMP responses and NGAF. The occurrence of NGAF and neuronal responses was strongly correlated across all mouse models and experimental conditions (36). Moreover, PPADS reduced the integrated magnitude of the NGAF response as well as the spatial spread of the type II afferent GCaMP6f response (36). Thus, the NGAF signal was used as a proxy for damage-evoked epithelial waves described previously using calcium indicators (12, 13, 24, 25), and in particular whether these activities were altered by noise-induced hearing loss.
Everyone has type II afferents, but not everyone has hyperacusis. Hyperacusis can be associated with a number of factors ranging from traumatic brain injury to autoimmune disease. Most commonly, it is related to prior acoustic trauma.1 Thus, any pathogenic mechanism should reflect this sensitization by previous trauma, akin to allodynia in skin. To that end, the cochlear damage response was examined in adult mice suffering noise-induced hearing loss (NIHL) (36). A striking feature of these NIHL cochleas was the occurrence of substantially delayed signals in both type II afferents and epithelial cells (NGAF). In both neurons and epithelia these could be repetitive, on the order of 1–2 per minute. These delayed damage responses were absent from immature (pre-hearing) cochlear tissue, and four times more likely in noise-exposed, compared to control adult tissue. Taken together these results support a hypothesis whereby tissue damage or stress activates ATP release from cochlear epithelia that can activate type II afferent neurons. Furthermore, prior trauma sensitizes the tissue leading to longer-lasting activity.
An unresolved issue concerns the use of NGAF for comparison to calcium waves. While there are a number of correspondences to support that assumption, further quantitative analysis is helpful. The velocity of calcium waves measured in both skin and cochlear tissue (see section 1, “calcium waves”) provides a useful measure for comparison to NGAF waves in the cochlea. Wave velocity following laser ablation was measured by first visualizing a standard deviation image of the entire time sequence to establish regions of interest (ROIs) for local measurement (Figure 1A1).
Figure 1. Analysis of autofluorescence – (Non-GCaMP Associated Fluorescence [NGAF]) in damaged cochlear epithelium; images from Th2ACreEr x Gcamp6ffl/fl mouse tissue of Nowak et al. (36). (A1) Standard deviation image of time series z-projection spanning 5.5 min in total (80 ms/slice). A central region of initial damage (“burn”), and a type II neuron are visible. (A2) A single 80 ms slice 14 s after the focused laser ablation (central bright green circle) shows one time point of spreading NGAF fluorescence. Red outline shows approximate initial response area (burn plus 9 s). Regions of interest (ROIs) from A1 overlaid. Colored asterisks denote exemplar ROIs used to determine wave velocity. (B) Average pixel intensity from Z projection for asterisked ROIs in A2.
Average pixel intensity throughout the time series was recorded for exemplar ROIs then displayed as a function of distance and time from the initial lesion (Figure 1B). The change in distance with time between wave fronts (measured at the transition to the steepest part of the rising signal in each ROI, approximately 10% of the peak amplitude) was used to calculate the velocity as a function of distance and time (Figure 2). Initial wave speed could reach 13 μm/s but then fell to 2–4 μm/s with distance and time from the initial response to damage. Wave speed was negatively correlated with both distance (Spearman’s r = −0.39) and time (Spearman’s r = −0.56). Camera saturation prevented imaging for the first 9 s after laser ablation so the very first (fastest) waves were not captured, weakening the correlation. (The first NGAF images 9 s after damage were large, averaging 2,647 ± 1,302 SD μm2 in area. Enclosed by a red line in Figure 1A2) The overall pattern is like that of damage-evoked calcium waves in skin in vivo that fall from a high of 25 to 0 μm/s over 13 s (23), or the slow waves in ex vivo cochlear Deiters cells that slow from ~12 to 3 μm/s over ~100 μm (24). Thus, allowing for experimental differences, NGAF waves behave similarly to damage-evoked calcium waves in skin and cochlea, presumably by similar mechanisms involving extracellular ATP and IP3-dependent release from internal calcium stores.
Figure 2. NGAF wave speed (38 ROIs, 10 trials on 6 cochleas) obtained as in Figure 1. (A) Wave speed with distance from leading edge of the initial response area (Spearman’s correlation = −0.39, p = 0.014), single exponential fit. (B) Wave speed with time (same ROIs as in A) >9 s after lesion, single exponential fit (Spearman’s correlation = −0.56, p = 0.003).
But what is NGAF, if not ‘GAF’? What other activity-dependent fluorescence changes in the cells could be calcium associated? One possibility is the intrinsic fluorescence of the reduced form of the redox cofactor, nicotinamide adenine dinucleotide, NADH, that absorbs at 340 ± 30 nm and emits at 460 ± 50 nm wavelength (42). This spectral characteristic is lost when oxidized to NAD. This change in fluorescence has made NAD/NADH fluorescence a useful indicator of the redox state and so the metabolism of living cells (43). Of relevance here, a rise in cytoplasmic calcium enters mitochondria via the calcium uniporter to increase NADH production (44) and so fluorescence. Indeed, NADH fluorescence, cytoplasmic and mitochondrial calcium are tightly correlated in other tissues (44) and can rise and fall with a half cycle duration of ~10 s (45). Similarly, spontaneous NGAF transients within individual, isolated cochlear epithelial cells (i.e., not part of a propagated wave) had an average duration of 9.5 s (±3.4 SD; 14 isolated epithelial cells from 8 experimental trials in 4 explants) on the same time scale as rapid NADH dynamics in other tissues.
Ex vivo imaging of semi-intact cochlear capsules using a genetically-encoded calcium sensor, GCaMP6f, enabled study of the tissue response to laser ablation of one to three outer hair cells. Serendipitously, the sensitive camera also revealed much weaker changes in fluorescence that did not rely on expression of the calcium sensor. This Non-GCaMP-Associated Fluorescence, NGAF, behaved similarly to damaged-evoked calcium waves studied previously in the cochlea and in skin. The NGAF waves propagated like those of previously published calcium waves, and like those, were diminished by purinoceptor blockade. Also, NGAF transients in isolated epithelial cells had a time course similar to the half-cycle duration of NADH oscillations found in other tissues (44, 45). As modeled for damage-evoked calcium waves in skin, these observations suggest that both intrinsic (intracellular oscillations of calcium, IP3 and NADH) and extrinsic (release of ATP through connexin hemi-channels) factors contribute to the damage-evoked signals in cochlear epithelia, as suggested previously (26).
Other studies of cochlear damage have identified additional molecular pathways that may be involved. Calcium homeostasis in small, electrically active hair cells is undoubtedly central to function and dysfunction (46, 47), including the generation of spontaneous calcium transients and synaptic maturation (48). Mitochondria serve as an important sink for cytoplasmic calcium, but additionally as a source of damaging reactive oxygen species during calcium overload (49, 50). It also has been shown that the damage-evoked calcium wave triggers activation of mitogen-activated kinases (ERK1/2) (51). These and stress-activated protein kinases (JNK and P38) have been implicated in the cochlear response to damage (52). Future studies can explore the impact of each of these pathways on the activation, speed and spread of epithelial calcium waves, and type II afferent activity.
The observations presented here provide a correlation, but do not establish causality. Are the type II afferents merely reporters of the epithelial signal, or do they contribute to its generation? The present data suggest that epithelial cells are the generators of the damage response. But in addition, as for somatic C-fibers, type II afferents could become ‘sensitized’ by prior trauma. Type II neurons express KCNQ potassium channels that contribute to the resting membrane conductance. ATP opens cation-selective P2X receptor channels, but also closes KCNQ channels via metabotropic P2Y receptors, further depolarizing the neuron (5). This increased input resistance of the type II afferent will make it more sensitive to glutamate release from OHCs, which, combined with the P2X-driven depolarization, could lower the threshold for making loud sound noxious. Presynaptically, ribbons of the OHCs increase in number and size after acoustic trauma (53), potentially providing stronger synaptic excitation to rouse previously silent type II contacts (27). In short, type II afferents may become better reporters of tissue damage after acoustic trauma. Whether this alters the probability, spread or persistence of epithelial waves remains to be determined.
Undoubtedly the pathogenesis of hyperacusis must involve still other processes.; especially considering that acoustically-evoked ear pain can continue for hours to days after sound exposure2 far beyond the minutes-long phenomena described to date. Such a prolonged time course suggests inflammation. Future research directions should include whether type II afferent signaling impacts immune cell activity in the cochlea. Type II afferents express neuropeptides such as CGRP (37, 38, 54), which in somatic C-fibers (55) modulates the activity of immune cells that proliferate after trauma (56). Thus, type II afferents could play a role in neuro-immune signaling as well. The cochlea provides another illustration of how neurons, epithelia and immune cells mediate the response to damage.
MW: Conceptualization, Data curation, Formal analysis, Funding acquisition, Investigation, Methodology, Project administration, Visualization, Writing – review & editing. NN: Conceptualization, Data curation, Formal analysis, Investigation, Methodology, Visualization, Writing – review & editing. PF: Formal analysis, Funding acquisition, Project administration, Resources, Supervision, Writing – original draft, Writing – review & editing.
The author(s) declare financial support was received for the research, authorship, and/or publication of this article. This study was supported by R01 DC016559 (PF) from the National Institutes for Deafness and Communication Disorders, the David M. Rubenstein Professorship and Fund for Hearing Research at Johns Hopkins University School of Medicine and an Emerging Research Grant from the Hearing Health Foundation to MW.
With thanks to F. Chakir for mouse-keeping and genotyping, and PFVY Vincent for guidance on use of the two-photon microscope.
The authors declare that the research was conducted in the absence of any commercial or financial relationships that could be construed as a potential conflict of interest.
All claims expressed in this article are solely those of the authors and do not necessarily represent those of their affiliated organizations, or those of the publisher, the editors and the reviewers. Any product that may be evaluated in this article, or claim that may be made by its manufacturer, is not guaranteed or endorsed by the publisher.
1. Weisz, CJC, Williams, SG, Eckard, CS, Divito, CB, Ferreira, DW, Fantetti, KN, et al. Outer hair cell glutamate signaling through type II spiral ganglion afferents activates neurons in the Cochlear nucleus in response to nondamaging sounds. J Neurosci. (2021) 41:2930–43. doi: 10.1523/JNEUROSCI.0619-20.2021
2. Robertson, D. Horseradish peroxidase injection of physiologically characterized afferent and efferent neurones in the guinea pig spiral ganglion. Hear Res. (1984) 15:113–21. doi: 10.1016/0378-5955(84)90042-X
3. Brown, MC. Antidromic responses of single units from the spiral ganglion. J Neurophysiol. (1994) 71:1835–47. doi: 10.1152/jn.1994.71.5.1835
4. Weisz, C, Glowatzki, E, and Fuchs, P. The postsynaptic function of type II cochlear afferents. Nature. (2009) 461:1126–9. doi: 10.1038/nature08487
5. Liu, C, Glowatzki, E, and Fuchs, PA. Unmyelinated type II afferent neurons report cochlear damage. Proc Natl Acad Sci USA. (2015) 112:14723–7. doi: 10.1073/pnas.1515228112
6. Brown, MC, Liberman, MC, Benson, TE, and Ryugo, DK. Brainstem branches from olivocochlear axons in cats and rodents. J Comp Neurol. (1988) 278:591–603. doi: 10.1002/cne.902780410
7. Froud, KE, Wong, AC, Cederholm, JM, Klugmann, M, Sandow, SL, Julien, JP, et al. Type II spiral ganglion afferent neurons drive medial olivocochlear reflex suppression of the cochlear amplifier. Nat Commun. (2015) 6:1–9. doi: 10.1038/ncomms8115
8. Cederholm, JME, Parley, KE, Perera, CJ, von Jonquieres, G, Pinyon, JL, Julien, JP, et al. Noise-induced hearing loss vulnerability in type III intermediate filament peripherin gene knockout mice. Front Neurol. (2022) 13:962227. doi: 10.3389/fneur.2022.962227
9. Maison, S, Liberman, LD, and Liberman, MC. Type II Cochlear ganglion neurons do not drive the Olivocochlear reflex: re-examination of the Cochlear phenotype in Peripherin Knock-out mice. eNeuro. (2016) 3:ENEURO.0207-16.2016. doi: 10.1523/ENEURO.0207-16.2016
10. Robertson, D, and Gummer, M. Physiological and morphological characterization of efferent neurones in the guinea pig cochlea. Hear Res. (1985) 20:63–77. doi: 10.1016/0378-5955(85)90059-0
11. Liberman, MC, and Brown, MC. Physiology and anatomy of single olivocochlear neurons in the cat. Hear Res. (1986) 24:17–36. doi: 10.1016/0378-5955(86)90003-1
12. Gale, JE, Piazza, V, Ciubotaru, CD, and Mammano, F. A mechanism for sensing noise damage in the inner ear. Curr Biol. (2004) 14:526–9. doi: 10.1016/j.cub.2004.03.002
13. Lahne, M, and Gale, JE. Damage-induced cell-cell communication in different cochlear cell types via two distinct ATP-dependent ca waves. Purinergic Signal. (2010) 6:189–200. doi: 10.1007/s11302-010-9193-8
14. Tritsch, NX, Yi, E, Gale, JE, Glowatzki, E, and Bergles, DE. The origin of spontaneous activity in the developing auditory system. Nature. (2007) 450:50–5. doi: 10.1038/nature06233
15. Tritsch, NX, and Bergles, DE. Developmental regulation of spontaneous activity in the mammalian cochlea. J Neurosci. (2010) 30:1539–50. doi: 10.1523/JNEUROSCI.3875-09.2010
16. Johnson, SL, Kuhn, S, Franz, C, Ingham, N, Furness, DN, Knipper, M, et al. Presynaptic maturation in auditory hair cells requires a critical period of sensory-independent spiking activity. Proc Natl Acad Sci USA. (2013) 110:8720–5. doi: 10.1073/pnas.1219578110
17. Tritsch, NX, Rodriguez-Contreras, A, Crins, TT, Wang, HC, Borst, JG, and Bergles, DE. Calcium action potentials in hair cells pattern auditory neuron activity before hearing onset. Nat Neurosci. (2010) 13:1050–2. doi: 10.1038/nn.2604
18. Carlton, AJ, Jeng, JY, Grandi, FC, de Faveri, F, Ceriani, F, de Tomasi, L, et al. A critical period of prehearing spontaneous ca(2+) spiking is required for hair-bundle maintenance in inner hair cells. EMBO J. (2023) 42:e112118. doi: 10.15252/embj.2022112118
19. Mammano, F. ATP-dependent intercellular Ca2+ signaling in the developing cochlea: facts, fantasies and perspectives. Semin Cell Dev Biol. (2013) 24:31–9. doi: 10.1016/j.semcdb.2012.09.004
20. Talagas, M, Lebonvallet, N, Berthod, F, and Misery, L. Lifting the veil on the keratinocyte contribution to cutaneous nociception. Protein Cell. (2020) 11:239–50. doi: 10.1007/s13238-019-00683-9
21. Boitano, S, Dirksen, ER, and Sanderson, MJ. Intercellular propagation of calcium waves mediated by inositol trisphosphate. Science. (1992) 258:292–5. doi: 10.1126/science.1411526
22. Handly, LN, and Wollman, R. Wound-induced ca(2+) wave propagates through a simple release and diffusion mechanism. Mol Biol Cell. (2017) 28:1457–66. doi: 10.1091/mbc.e16-10-0695
23. Donati, V, Peres, C, Nardin, C, Scavizzi, F, Raspa, M, Ciubotaru, CD, et al. Calcium signaling in the photodamaged skin: in vivo experiments and mathematical modeling. Function (Oxf). (2022) 3:zqab064. doi: 10.1093/function/zqab064
24. Sirko, P, Gale, JE, and Ashmore, JF. Intercellular ca(2+) signalling in the adult mouse cochlea. J Physiol. (2019) 597:303–17. doi: 10.1113/JP276400
25. Anselmi, F, Hernandez, VH, Crispino, G, Seydel, A, Ortolano, S, Roper, SD, et al. ATP release through connexin hemichannels and gap junction transfer of second messengers propagate Ca2+ signals across the inner ear. Proc Natl Acad Sci USA. (2008) 105:18770–5. doi: 10.1073/pnas.0800793105
26. Piazza, V, Ciubotaru, CD, Gale, JE, and Mammano, F. Purinergic signalling and intercellular Ca2+ wave propagation in the organ of Corti. Cell Calcium. (2007) 41:77–86. doi: 10.1016/j.ceca.2006.05.005
27. Martinez-Monedero, R, Liu, C, Weisz, C, Vyas, P, Fuchs, PA, and Glowatzki, E. GluA2-containing AMPA receptors distinguish ribbon-associated from Ribbonless afferent contacts on rat Cochlear hair cells. eNeuro. (2016) 3:ENEURO.0078-16.2016. doi: 10.1523/ENEURO.0078-16.2016
28. Berglund, AM, and Ryugo, DK. Hair cell innervation by spiral ganglion neurons in the mouse. J Comp Neurol. (1987) 255:560–70. doi: 10.1002/cne.902550408
29. Brown, MC. Morphology of labeled afferent fibers in the guinea pig cochlea. J Comp Neurol. (1987) 260:591–604. doi: 10.1002/cne.902600411
30. Spoendlin, H. Anatomy of cochlear innervation. Am J Otolaryngol. (1985) 6:453–67. doi: 10.1016/S0196-0709(85)80026-0
32. Young, ED. Neural representation of spectral and temporal information in speech. Philos Trans R Soc Lond Ser B Biol Sci. (2008) 363:923–45. doi: 10.1098/rstb.2007.2151
33. Weisz, CJ, Glowatzki, E, and Fuchs, PA. Excitability of type II cochlear afferents. J Neurosci. (2014) 34:2365–73. doi: 10.1523/JNEUROSCI.3428-13.2014
34. Weisz, CJ, Lehar, M, Hiel, H, Glowatzki, E, and Fuchs, PA. Synaptic transfer from outer hair cells to type II afferent fibers in the rat cochlea. J Neurosci. (2012) 32:9528–36. doi: 10.1523/JNEUROSCI.6194-11.2012
36. Nowak, N, Wood, MB, Glowatzki, E, and Fuchs, PA. Prior acoustic trauma alters type II afferent activity in the mouse cochlea. eNeuro. (2021) 8:ENEURO.0383-21.2021. doi: 10.1523/ENEURO.0383-21.2021
37. Sherry, WJ, Vyas, P, Glowatzki, E, and Fuchs, PA. Opposing expression gradients of calcitonin-related polypeptide alpha (Calca/Cgrpalpha) and tyrosine hydroxylase (Th) in type II afferent neurons of the mouse cochlea. J Comp Neurol. (2018) 526:1073. doi: 10.1002/cne.24380
38. Vyas, P, Wu, JS, Jimenez, A, Glowatzki, E, and Fuchs, PA. Characterization of transgenic mouse lines for labeling type I and type II afferent neurons in the cochlea. Sci Rep. (2019) 9:5549. doi: 10.1038/s41598-019-41770-5
39. Vyas, P, Wu, JS, Zimmerman, A, Fuchs, P, and Glowatzki, E. Tyrosine hydroxylase expression in type II Cochlear afferents in mice. J Assoc Res Otolaryngol. (2017) 18:139–51. doi: 10.1007/s10162-016-0591-7
40. Abraira, VE, Kuehn, ED, Chirila, AM, Springel, MW, Toliver, AA, Zimmerman, AL, et al. The cellular and synaptic architecture of the Mechanosensory dorsal horn. Cell. (2017) 168:295–310.e19. doi: 10.1016/j.cell.2016.12.010
41. Rabbitt, RD, and Holman, HA. ATP and ACh evoked calcium transients in the neonatal mouse Cochlear and vestibular sensory epithelia. Front Neurosci. (2021) 15:710076. doi: 10.3389/fnins.2021.710076
42. Blacker, TS, Duchen, MR, and Bain, AJ. NAD(P)H binding configurations revealed by time-resolved fluorescence and two-photon absorption. Biophys J. (2023) 122:1240–53. doi: 10.1016/j.bpj.2023.02.014
43. Chance, B, Cohen, P, Jobsis, F, and Schoener, B. Intracellular oxidation-reduction states in vivo. Science. (1962) 137:499–508. doi: 10.1126/science.137.3529.499
44. Voronina, S, Sukhomlin, T, Johnson, PR, Erdemli, G, Petersen, OH, and Tepikin, A. Correlation of NADH and Ca2+ signals in mouse pancreatic acinar cells. J Physiol. (2002) 539:41–52. doi: 10.1113/jphysiol.2001.013134
45. Porat-Shliom, N, Chen, Y, Tora, M, Shitara, A, Masedunskas, A, and Weigert, R. In vivo tissue-wide synchronization of mitochondrial metabolic oscillations. Cell Rep. (2014) 9:514–21. doi: 10.1016/j.celrep.2014.09.022
46. Fettiplace, R, and Nam, JH. Tonotopy in calcium homeostasis and vulnerability of cochlear hair cells. Hear Res. (2019) 376:11–21. doi: 10.1016/j.heares.2018.11.002
47. Mammano, F, and Bortolozzi, M. Ca(2+) signaling, apoptosis and autophagy in the developing cochlea: milestones to hearing acquisition. Cell Calcium. (2018) 70:117–26. doi: 10.1016/j.ceca.2017.05.006
48. Yang, Y, Murtha, K, Climer, LK, Ceriani, F, Thompson, P, Hornak, AJ, et al. Oncomodulin regulates spontaneous calcium signalling and maturation of afferent innervation in cochlear outer hair cells. J Physiol. (2023) 601:4291–308. doi: 10.1113/JP284690
49. O'Sullivan, JDB, Bullen, A, and Mann, ZF. Mitochondrial form and function in hair cells. Hear Res. (2023) 428:108660. doi: 10.1016/j.heares.2022.108660
50. Mann, ZF, Duchen, MR, and Gale, JE. Mitochondria modulate the spatio-temporal properties of intra- and intercellular Ca2+ signals in cochlear supporting cells. Cell Calcium. (2009) 46:136–46. doi: 10.1016/j.ceca.2009.06.005
51. Lahne, M, and Gale, JE. Damage-induced activation of ERK1/2 in cochlear supporting cells is a hair cell death-promoting signal that depends on extracellular ATP and calcium. J Neurosci. (2008) 28:4918–28. doi: 10.1523/JNEUROSCI.4914-07.2008
52. Wong, AC, and Ryan, AF. Mechanisms of sensorineural cell damage, death and survival in the cochlea. Front Aging Neurosci. (2015) 7:58. doi: 10.3389/fnagi.2015.00058
53. Wood, MB, Nowak, N, Mull, K, Goldring, A, Lehar, M, and Fuchs, PA. Acoustic trauma increases ribbon number and size in outer hair cells of the mouse cochlea. J Assoc Res Otolaryngol. (2021) 22:19–31. doi: 10.1007/s10162-020-00777-w
54. Shrestha, BR, Chia, C, Wu, L, Kujawa, SG, Liberman, MC, and Goodrich, LV. Sensory neuron diversity in the inner ear is shaped by activity. Cell. (2018) 174:1229–1246.e17. doi: 10.1016/j.cell.2018.07.007
55. Assas, BM, Pennock, JI, and Miyan, JA. Calcitonin gene-related peptide is a key neurotransmitter in the neuro-immune axis. Front Neurosci. (2014) 8:23. doi: 10.3389/fnins.2014.00023
Keywords: cochlea, type II afferent, epithelia, trauma, calcium waves, hyperacusis
Citation: Wood MB, Nowak N and Fuchs PA (2024) Damage-evoked signals in cochlear neurons and supporting cells. Front. Neurol. 15:1361747. doi: 10.3389/fneur.2024.1361747
Received: 26 December 2023; Accepted: 31 January 2024;
Published: 14 February 2024.
Edited by:
Walter Marcotti, The University of Sheffield, United KingdomReviewed by:
Anthony John Ricci, Stanford University, United StatesCopyright © 2024 Wood, Nowak and Fuchs. This is an open-access article distributed under the terms of the Creative Commons Attribution License (CC BY). The use, distribution or reproduction in other forums is permitted, provided the original author(s) and the copyright owner(s) are credited and that the original publication in this journal is cited, in accordance with accepted academic practice. No use, distribution or reproduction is permitted which does not comply with these terms.
*Correspondence: Paul Albert Fuchs, cGZ1Y2hzMUBqaG1pLmVkdQ==
†Present address: Nate Nowak, Caruso Department of Otolaryngology Head & Neck Surgery, Zilkha Neurogenetics Institute, Hearing and Communications Neuroscience Training Program, University of Southern California Keck School of Medicine, Los Angeles, CA, United States
Disclaimer: All claims expressed in this article are solely those of the authors and do not necessarily represent those of their affiliated organizations, or those of the publisher, the editors and the reviewers. Any product that may be evaluated in this article or claim that may be made by its manufacturer is not guaranteed or endorsed by the publisher.
Research integrity at Frontiers
Learn more about the work of our research integrity team to safeguard the quality of each article we publish.