- 1Department of Biomedical Engineering, Case Western Reserve University, Cleveland, OH, United States
- 2Department of Physical Therapy and Human Movement Sciences, Northwestern University, Chicago, IL, United States
- 3Department of Biomedical Engineering, Northwestern University, Evanston, IL, United States
- 4Department of Anesthesiology, Northwestern University, Chicago, IL, United States
- 5Cleveland FES Center, Louis Stokes Cleveland Veterans Affairs Medical Center, Cleveland, OH, United States
Introduction: Hand opening is reduced by abnormal wrist and finger flexor activity in many individuals with stroke. This flexor activity also limits hand opening produced by functional electrical stimulation (FES) of finger and wrist extensor muscles. Recent advances in electrical nerve block technologies have the potential to mitigate this abnormal flexor behavior, but the actual impact of nerve block on hand opening in stroke has not yet been investigated.
Methods: In this study, we applied the local anesthetic ropivacaine to the median and ulnar nerve to induce a complete motor block in 9 individuals with stroke and observed the impact of this block on hand opening as measured by hand pentagonal area. Volitional hand opening and FES-driven hand opening were measured, both while the arm was fully supported on a haptic table (Unloaded) and while lifting against gravity (Loaded). Linear mixed effect regression (LMER) modeling was used to determine the effect of Block.
Results: The ropivacaine block allowed increased hand opening, both volitional and FES-driven, and for both unloaded and loaded conditions. Notably, only the FES-driven and Loaded condition’s improvement in hand opening with the block was statistically significant. Hand opening in the FES and Loaded condition improved following nerve block by nearly 20%.
Conclusion: Our results suggest that many individuals with stroke would see improved hand-opening with wrist and finger flexor activity curtailed by nerve block, especially when FES is used to drive the typically paretic finger and wrist extensor muscles. Such a nerve block (potentially produced by aforementioned emerging electrical nerve block technologies) could thus significantly address prior observed shortcomings of FES interventions for individuals with stroke.
1 Introduction
An estimated 9.4 million Americans 20 years of age or older self-reported having had a stroke, with projections suggesting that an additional 3.4 million Americans may join them by 2030 (1). Moderately impaired individuals have a reduced ability to open their hands, while severely impaired individuals are often unable to open their impaired hand at all—especially due to involuntary flexion forces at wrist and fingers linked to increasing abduction load at the shoulder (2) and flexor hypertonia (3). While motor impairments at the paretic hand are due to multiple factors (4), of particular importance are overactive wrist and finger flexors and simultaneous extensor weakness (5). In particular, the proportional reduction of hand opening in relation to shoulder abduction loading results largely from the expression of the “flexion synergy” (i.e., abnormal coupling between shoulder abduction and elbow/wrist and finger flexion) (6–8), thought to be due to greater reliance on reticulospinal projections following a hemiparetic stroke (9). Furthermore, the flexor hypertonia may be related to the possible upregulation of monoaminergic coeruleospinal projections (10). The presence of hyperactive stretch reflexes, in comparison, may not play a major role in stroke disability (5) compared to the expression of said flexion synergy (11). As passive muscle properties are also largely unchanged (4, 12), it is likely the overactive wrist and finger flexors (particularly the flexion synergy) and extensor weakness that limits hand use in stroke.
The reduction in hand opening while lifting, induced by said flexion synergy expression (2), persists even when assisted by functional electrical stimulation (FES), limiting the effectiveness of FES interventions (13–15). Limited ability to open one’s hand can also lead to the “learned disuse” of the whole paretic arm (16), potentially worsening patient outcomes over time. Without the useful end-effector necessary for many activities of daily living, the impact of reach-focused rehabilitation interventions (17, 18) can be reduced as well.
A possible method for improving hand opening during lifting is thus to inhibit the “over-activated” flexors. One of the most commonly utilized clinical methods for reducing hyperactive flexor activity is the use of botulinum toxin A, which temporarily reduces function at the neuromuscular junction (19, 20). This approach has been employed with initially encouraging results (21–23). However, the approach also has a number of limitations. While the “therapeutic effect” is reported to last 3 months, the magnitude of that effect varies significantly within this window with peak effect occurring around 5 weeks and gradual reduction of effect thereafter (24). Therefore, most patients require repeat injections, often in combination with physical therapies, every 3 to 4 months (25). A review article has shown strong evidence that botulinum toxins reduce hypertonia and spasticity (i.e., hyperactive stretch reflex), but its effect on improving hand and arm function is less compelling (26). Botulinum toxins further reduce the strength of the already paretic muscle, which may negatively impact function (27). Some individuals even develop neutralizing antibodies to the toxin, rendering the intervention ineffective with repeat injections (28). Finally, evidence also indicates potential long-term concerns related to increased muscle passive stiffness, possibly due to muscle extracellular matrix proliferation (12, 29–32). An alternative worth exploring is the use of FES-based methods that provide instantaneous, controllable, and reversible blocking of peripheral nerve transmission (33–35). However, these methods are still under development, and their feasibility in improving voluntary hand opening and/or FES-driven hand opening, with or without arm lifting, has yet to be evaluated.
In this study we temporarily relaxed the finger flexor muscles using an anesthesia block of the median and ulnar nerves as a proxy for future electrical block techniques. Specifically, ropivacaine was selected for perineural injection into both median and ulnar nerves to provide an adequate motor block duration (~8.7 h) (36, 37) with low required dosages (5 mL) (38). The efficacy of such a temporary nerve block approach was then assessed by measuring improvements in volitional- and FES-assisted hand opening after the application of the anesthesia nerve block, both when the arm was in a relaxed state and when participants had to raise their paretic arm against gravity by abducting at the shoulder.
2 Materials and methods
2.1 Participants
Having conducted a power analysis based on earlier hand opening data involving stroke participants (2), in which we assumed a nerve block effect size of a 20% increase in hand opening and similar variance, we enrolled a total of 10 individuals with chronic stroke (occurring more than 1 year ago) for this proof-of-concept study. The respective demographics of these participants are detailed in Table 1. Participants were recruited from the Shirley Ryan AbilityLab/Physical Therapy and Human Movement Sciences Clinical Research Registry and from the greater Chicago area. Other main inclusion/exclusion criteria included: (1) paresis confined to one side, with an ability to lift the arm up to the horizontal plane while maintaining 90 degrees elbow flexion; (2) no allergies to lidocaine or ropivacaine, and no use of contraindicated medications; (3) no recent or prior long-term use of other chemodenervation approaches, such as botulinum toxin, in the hand and wrist flexor muscles; (4) absence of any severe concurrent medical problems (such as cardiorespiratory impairment) or any acute/chronic pain conditions in the upper extremities or spine greater than 5 on the 10-point visual analog scale; (5) no use of a cardiac pacemaker, implanted defibrillator, neurostimulation device, or similar implanted electrical equipment.
All participants gave written informed consent for participation in this study and the publication of any potentially identifiable images or data included in this article, as approved by the Northwestern University Institutional Review Board (IRB #STU00213403).
2.2 Experimental setup
The experiment was performed on the Arm Coordination Training 3D (ACT3D) system (39, 40), which consists of a modified HapticMaster robot (Moog-FCR BV, the Netherlands) and a Biodex chair and T-Base support system (Biodex Medical Systems, Shirley, NY). The ACT3D was used to measure arm configuration and modulate shoulder abduction load. Under the “Unloaded” condition, a frictionless virtual haptic table was provided by the ACT3D, and under the “Loaded” condition, a shoulder abduction load of 100% of the participant’s limb weight (41) was imposed.
Participants were seated in the Biodex chair with the trunk and shoulder strapped securely to prevent compensatory movements. Following a short series of wrist and finger stretches, the participant’s impaired arm was attached to the forearm orthosis of the ACT3D and placed in a “Home Position” of 85° shoulder abduction (SABD), 90° elbow flexion (EF), 40° shoulder flexion (SF), and 0° wrist extension (WE) (see Figure 1). The participant’s fingers, thumb, and palm were placed around a cylinder attached to the distal end of the forearm orthosis. On the cylinder, a pressure sensor mat (Custom TactArray Sensing System, Pressure Profile Systems Inc., Los Angeles, CA) was mounted circumferentially. This pressure mat contained 27 by 21 sensors across a 6.4 by 5.1-inch surface area, with each sensor able to record up to 50 PSI with a pressure sensitivity of 0.15%. Furthermore, five Model 180 sensors from two linked trakSTAR systems (Northern Digital Company, Waterloo, ON, Canada) were placed on each of the tips of the 5 fingers to record the hand aperture to an accuracy of 1.4 mm Root Mean Square Error.
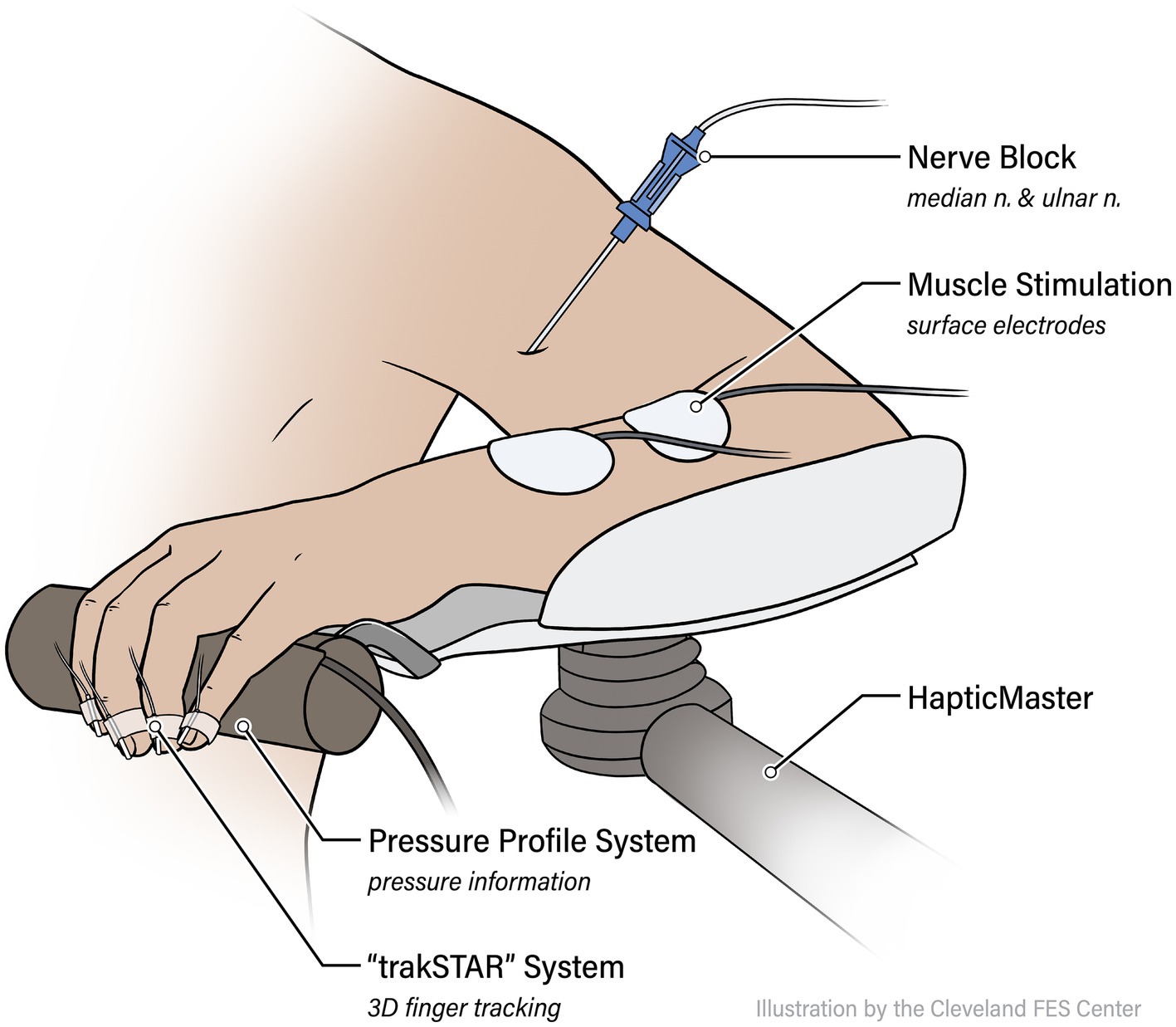
Figure 1. Experimental setup. Participants are attached via an orthosis to the ACT3D assistive/loading device, and instrumented with the trakSTAR position sensors and PPS pressure mat. Functional electrical stimulation (FES) electrodes are placed on forearm flexors (flexor digitorum superficialis, or FDS) and extensors (extensor digitorum communis, or EDC).
2.2.1 Assistive functional electrical stimulation parameters
A 2-channel E-Wave surface stimulator (Zynex Medical, Englewood, CO) was used to stimulate forearm compartment finger muscles, with one set of bipolar cutaneous electrodes placed over the flexor digitorum superficialis (FDS), and the other bipolar electrode pair placed over the extensor digitorum communis (EDC). The E-Wave was set up with a 200 μs pulse width duration and a 28 Hz biphasic stimulation paradigm that balances participant comfort with minimization of fatigue (42, 43). For each participant, each channel’s appropriate intensity was found by incrementally increasing the current amplitude until a visible plateau of effect was reached, or the participant expressed discomfort.
2.3 Protocol
2.3.1 Before anesthesia (Unblocked)
After the instrumentation setup, each participant performed a series of hand Opening and Closing tasks. These tasks were done as groups of at least 3 repetitions per set of conditions, under the following 2-by-2 conditions, themselves selected in random order: shoulder abduction loading condition (Loaded vs. Unloaded) and driving condition (Volitionally vs. FES driven).
All tasks were performed with the tested arm/hand at the “Home Position.” An auditory cue, 200 ms after the start of data collection, was used to trigger the participant to start the required task. Under the Unloaded condition, the participant opened or closed their hand volitionally (if a Volitional trial) or simply relaxed to let FES drive the task (if FES trial) for 6 s with the arm resting on the table. Under the Loaded condition, after hearing the auditory cue the participant first lifted their arm to the horizontal (90° SABD) level, then performed the open or close task Volitionally or with FES for 6 additional seconds. A rest period of at least 30 s was provided between trials to minimize fatigue.
2.3.2 Peripheral nerve anesthesia block
After the data collection for the Unblocked condition described above, the participant was prepped for applications of Ropivacaine to the ulnar and median nerves in the upper arm to induce a block of all wrist and finger flexors. After cleaning the skin with ChloraPrep (Becton, Dickinson and Company, Franklin Lakes, NJ), a trained anesthesiologist identified the position of each nerve via Ultrasound (GE LOGIQ e Ultrasound, 12L transducer, GE, Buc, France) and a SonoPlex echogenic nerve block needle (PAJUNK, Geisingen, Germany). Once a nerve was located, 5 mL of 0.5% Ropivacaine (Naropin, AstraZeneca, Wilmington, DE) was applied via perineural injection (see Figure 2). Throughout these injections, electrocardiogram, heart rate, and blood pressure were closely monitored (GE Carescape B105, GE Healthcare, Chicago, IL) for any adverse reactions.
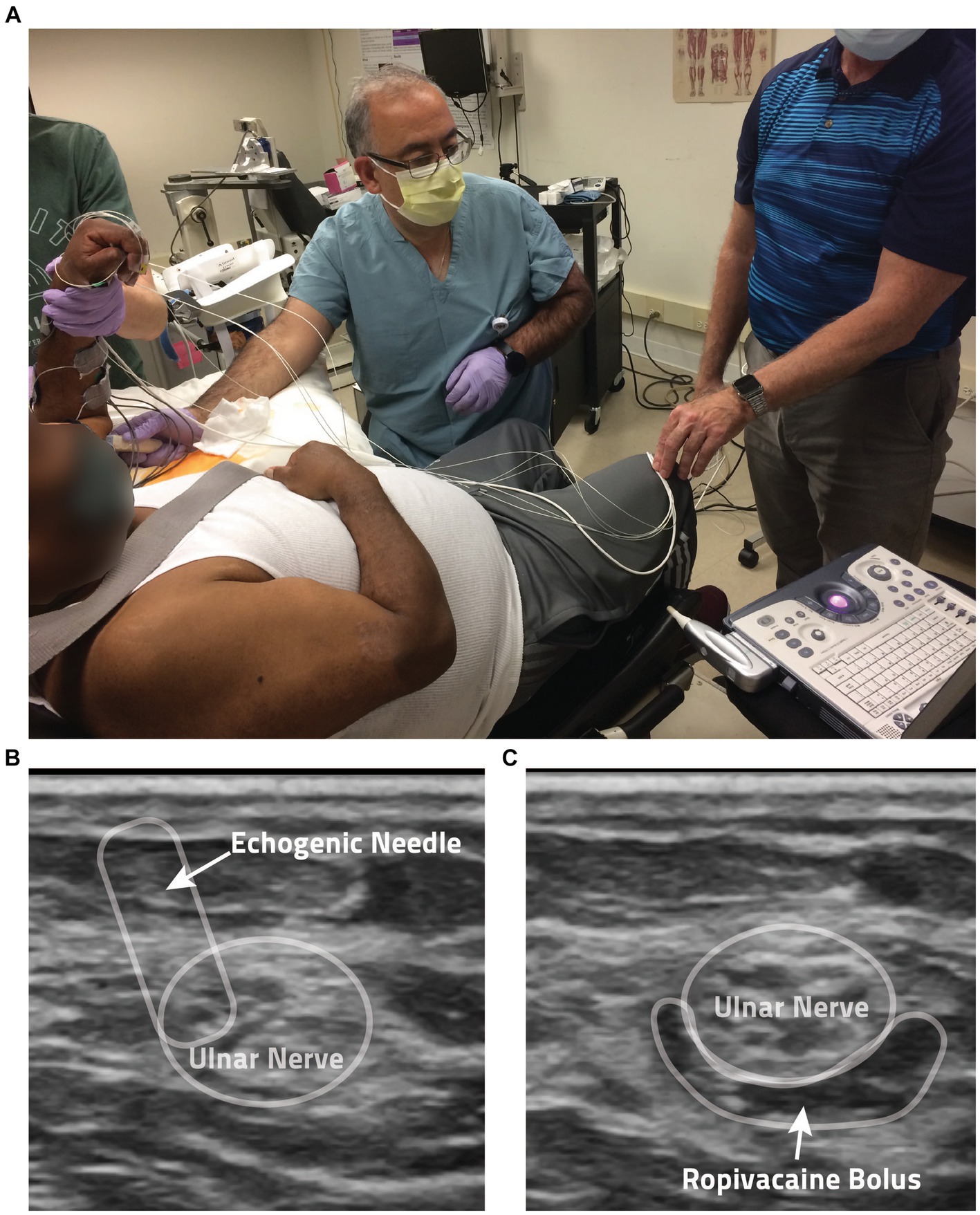
Figure 2. Anesthesia protocol. (A) Shown is a photo of the anesthesia application process. (B) Ultrasound guidance is used by the anesthesiologist in the administration of the local anesthesia ropivacaine. Shown here is the perineural injection method employed, with the echogenic needle noted by the arrow. (C) In this ultrasound image, you can see the anesthesia bolus surrounding a participant’s ulnar nerve following injection.
2.3.3 After anesthesia (Blocked condition)
After a 1.5 h rest period during which the block effect was allowed to plateau, the same data collection under all the various conditions described in the Unblocked condition was repeated for the Blocked condition.
2.4 Data collection
Data was recorded using a custom MATLAB program (Mathworks, Natick, MA) using API libraries from Pressure Profile Systems and Northern Digital. The flexion force was measured by the pressure sensor mat sampled at 16.5 Hz. The finger position was measured by the trakSTAR system sampled at 30 Hz.
2.5 Data analysis
2.5.1 Outcome measures/metrics
Hand pentagon area (HPA), shown to be an effective measure in evaluating hand opening ability (2), was used as the primary outcome measure when quantifying hand opening. As shown in Figure 3, this area (in mm2) was calculated as the sum of the surface area of three triangles formed by the participant’s fingertip sensor locations in 3D space: thumb-index-middle, thumb-middle-ring, and thumb-ring-pinky. The maximum HPA presented during each Hand Opening trial was calculated. The average of these max HPAs across trials of the same conditions was then normalized by each participant’s largest HPA (across all conditions), providing a 0–100% hand opening metric that could be readily compared across participants and between conditions.
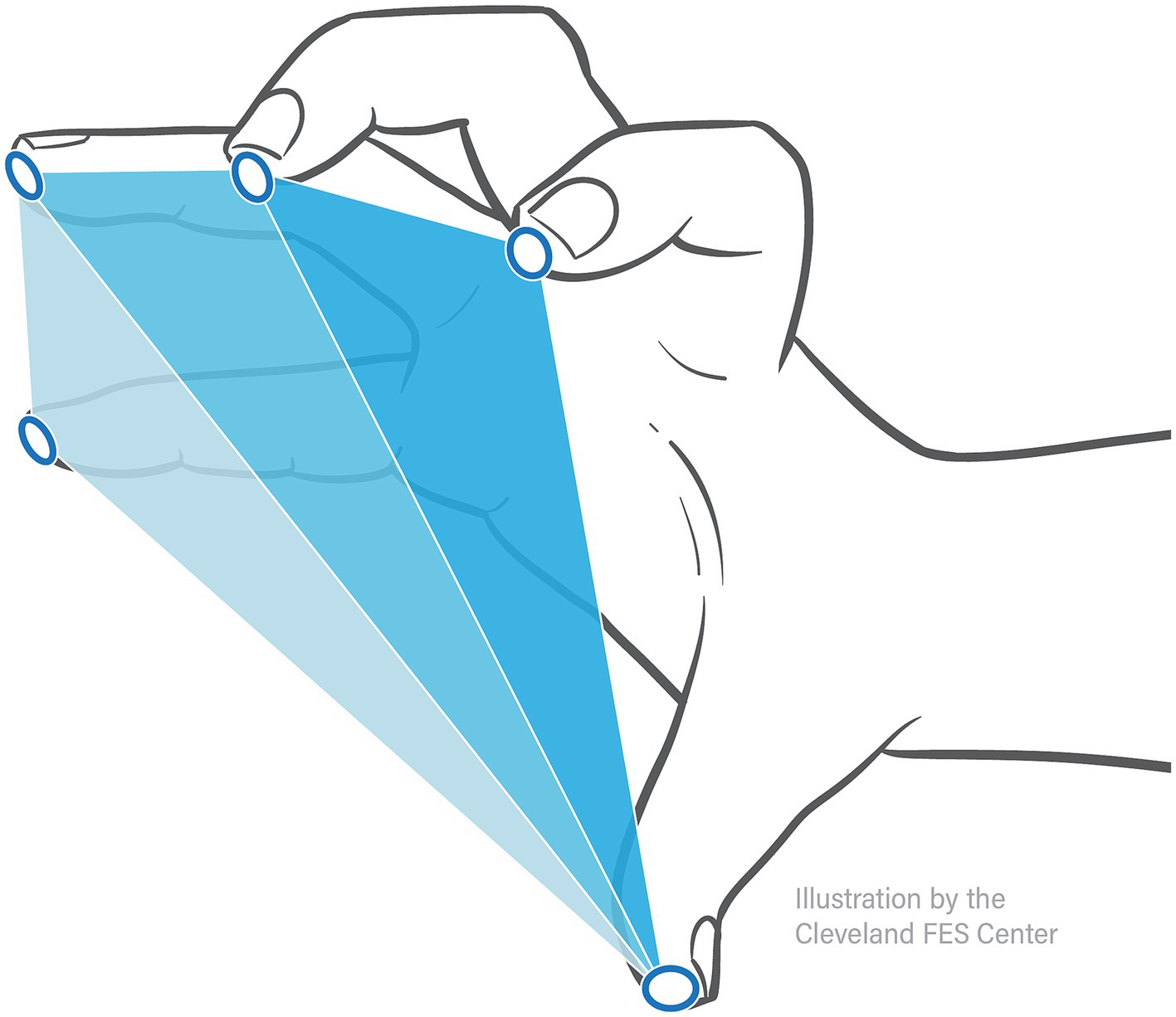
Figure 3. Hand pentagon area. Hand pentagon area, or HPA, is found using the trakSTAR sensor positions by calculating the surface area of three triangles made by the 5 fingertips: thumb-index-middle, thumb-middle-ring, and thumb-ring-pinky.
To determine block success and gauge the impact of nerve block on FES function, the total grasp force generated by each participant’s fingers and wrist in pounds (lbs) was calculated from pressure mat data by multiplying the PSI value of each sensor by each sensor’s size (6 by 6 mm). The maximum presented grasp force during a Hand Closing trial was determined, ensemble-averaged across each participant’s trials within the same condition, and finally normalized by each participant’s largest Volitional grasp force.
An example of the collected HPA of two Hand Opening trials (Unblocked and Blocked of the same conditions) and the grasp force values of two Hand Closing trials (Unblocked and Blocked of the same conditions) can be seen in Figure 4.
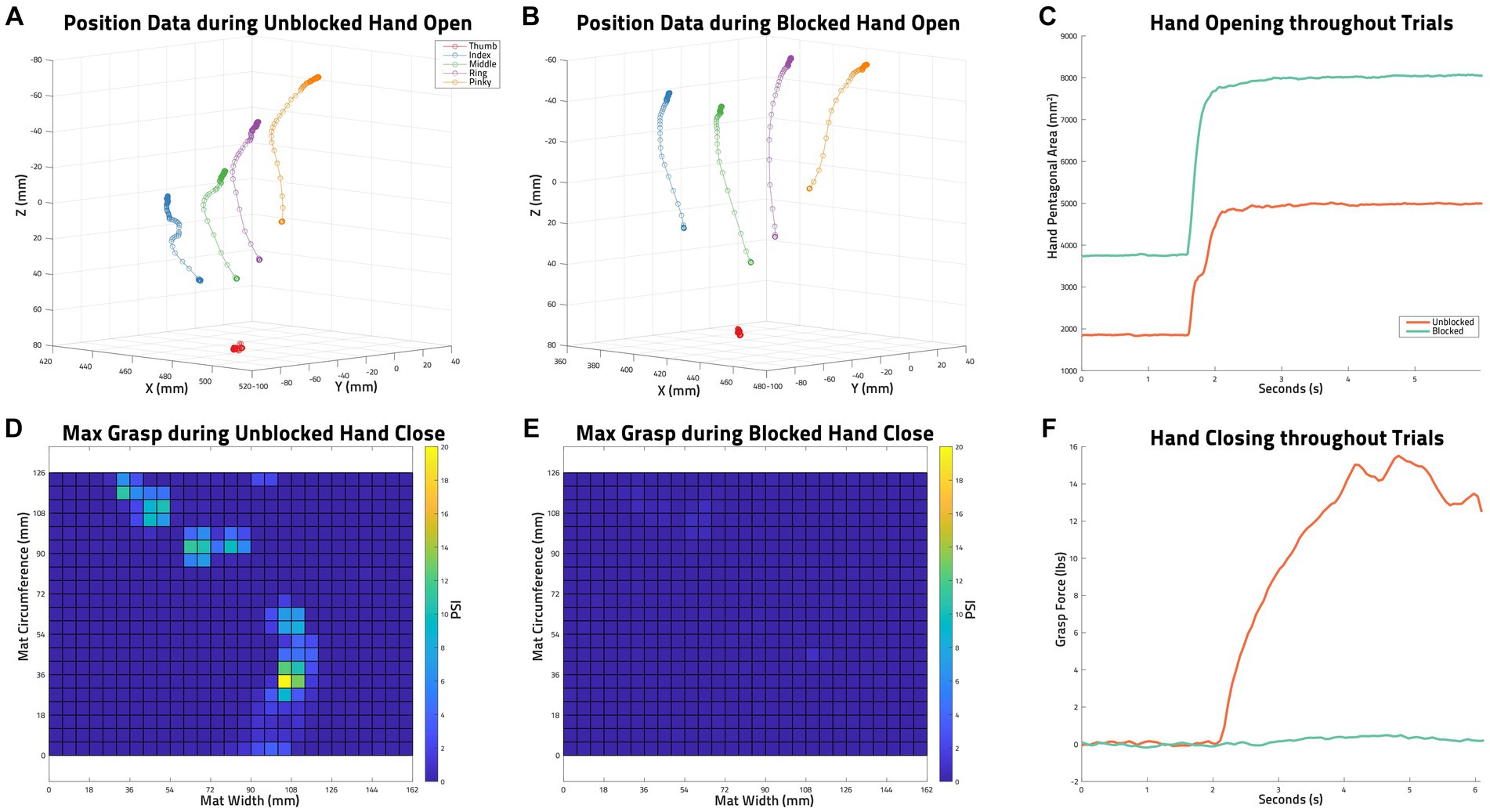
Figure 4. Example of Collected Data. (A) trakSTAR position data of all sensors during an unblocked hand opening task by S03: functional electrical stimulation (FES), unloaded, trial 3. (B) trakSTAR position data of all sensors during a blocked hand opening task by S03 (FES, unloaded, trial 2). (C) Hand pentagon area (HPA) as calculated from the sensor positions shown in (A,B) throughout the two mentioned trials. (D) The maximum grasp force measured with the PPS pressure mat during an unblocked volitional hand closing task by S03 (unloaded, trial 2). (E) The maximum grasp force measured with the PPS pressure mat during a blocked volitional hand closing task by s03 (unloaded, trial 1). (F) The grasp forces in lbs calculated from PPS pressure mat data (maxes of which are shown in D,E) throughout the two mentioned trials.
2.5.2 Statistical analysis
At the individual level, paired t-tests were used per participant for Volitionally-driven Close and for FES-induced Close, separately, to verify that the nerve block significantly reduced grasp forces while not having any impact on FES behavior.
A linear mixed effects regression (LMER) model was then created to determine if observed significant differences in FES-induced grasp force followed a consistent trend dependent on the Block condition.
LMER models were also used to determine the impact of Block on hand-opening. Four models were made for the following conditions: Volitional and Unloaded, Volitional and Loaded, FES-driven and Unloaded, and FES-driven and Loaded. All data used in these models were normally distributed (Shapiro–Wilk test) so as to satisfy LMER assumptions.
Statistical significance was set at p < 0.05. All statistical analyses were performed in R (The R Foundation, Indianapolis, IN).
3 Results
3.1 Determining block success
The ability of the anesthesia block of the median and ulnar nerves to reduce hand flexor muscle forces is illustrated in Figure 5, which shows flexion forces before and after the block for each of the participants in this study. To ensure a successful block, grasping forces were measured during (1) voluntary hand closing while the arm was supported (Unloaded) and (2) simultaneous voluntary and synergy-driven hand closing from lifting the arm against a load (Loaded). For all participants except one (S09), the anesthesia block produced large decreases (average 75%) in grasp force; S09 is denoted with an asterisk in all figures for this reason.
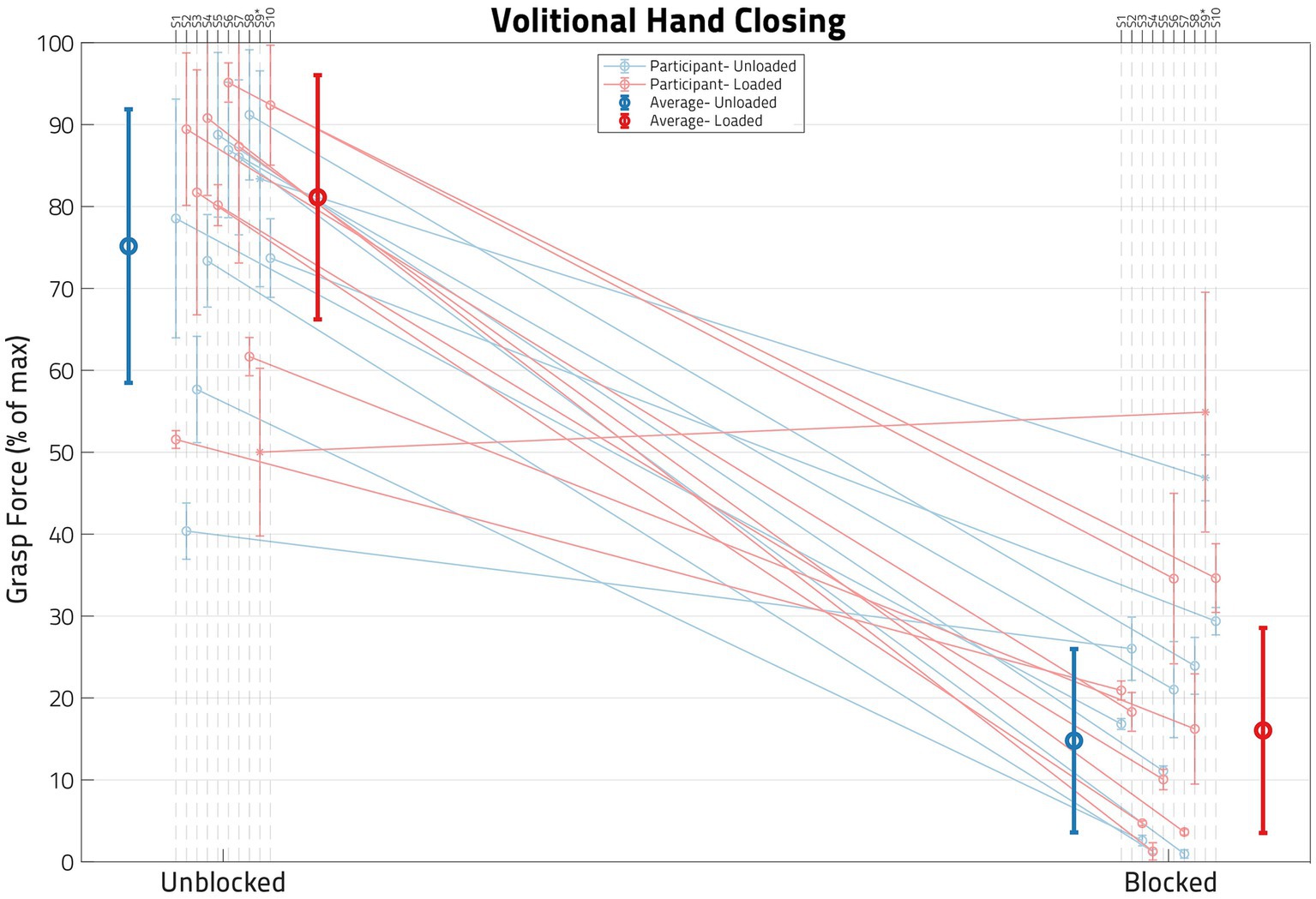
Figure 5. Impact of block on volitional hand closing. Each dot represents the normalized mean grasp force for a set of load and block conditions, with the error bars denoting standard deviation. Unblocked means are on the left and blocked means on the right. The blue lines represent Unloaded shoulder trials and the red lines represent Loaded shoulder trials. The blue and red circles on the left are the cross-participant averages for the Unblocked condition, while the corresponding circles on the right are for the Blocked condition. Note that, following block, grasp forces are reduced in most participants, verified by a paired t-test (p < 0.05). Only one participant, S09, failed to demonstrate statistical difference between pre- and post-nerve Block.
A paired t-test per participant (using all of their Volitional Close trials, both Unloaded and Loaded) indicated a significant (p < 0.05) drop in grasp force following the nerve block in all participants except S09 (whose means are represented by an asterisk in Figure 5 instead of a circle). Table 2 shows both the grasp forces of each participant and the percent drop in Volitional grasp force due to anesthesia nerve block per participant. Participant S09 had a much lower grasp force than any of the other participants, and the relative decrease (36.5%) in grasp force was significantly lower than the other participants. A successful nerve block was defined as a drop of at least 50% Volitional grasp forces following the application of anesthesia (4). Thus, S09 was not included in any subsequent statistical analyses.
3.2 FES behavior post nerve block
The impact of the anesthesia block on the hand flexion forces elicited by FES (applied at the forearm, below the elbow) is illustrated in Figure 6. The mean normalized FES-induced grasp forces before and after the nerve blocks are shown for the participants, along with their accompanying standard deviations. The average change in normalized FES-elicited grasp forces across participants before and after the block was very small—it is shown by the darker line, along with its associated standard deviation.
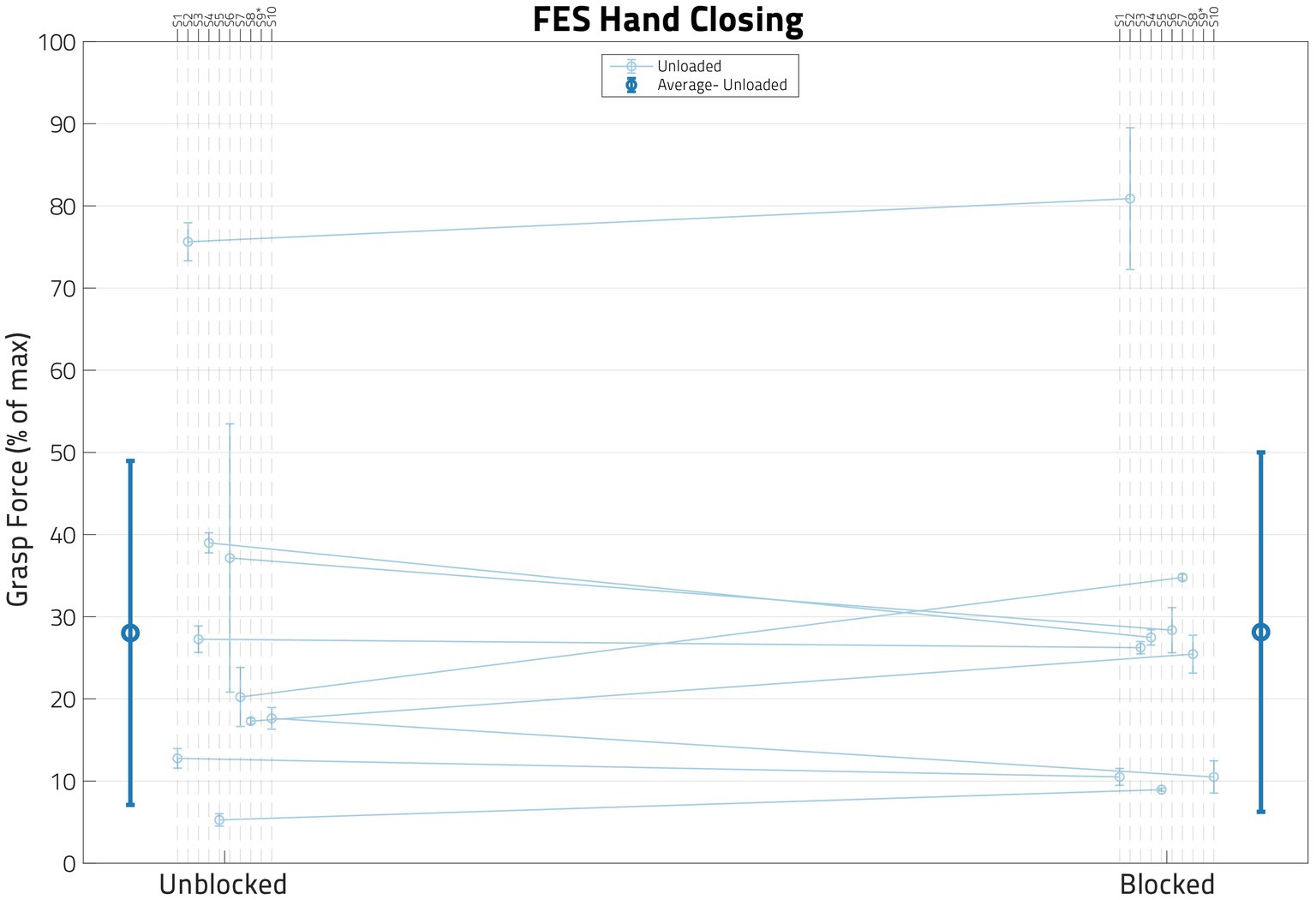
Figure 6. Impact of block on FES behavior. In this figure, each dot represents the normalized mean functional electrical stimulation (or FES) induced grasp force from the trials for one participant, with the error bars denoting standard deviations. While paired t-tests showed that these two populations were significantly different in some participants, a linear mixed effects regression (LMER) model found that there was no global trend. S09’s data is not shown in this figure as their FES grasp forces surpassed the volitional grasp force 0–100% range.
A paired t-test per participant using all FES Unloaded trial data indicated that the nerve block did change the FES-induced grasping force (p < 0.05) for 6 out of 9 eligible participants. Furthermore, an LMER model that included only Block as the Fixed Effect and Participant as the Random Effect was used to determine whether nerve block has any effect on FES-driven hand closing forces under the Unloaded condition (Table 3). The model did not find Block statistically significant (p ≫ 0.05).
3.3 Hand opening
Figure 7 shows the hand opening expressed as hand pentagon area (HPA) of all 10 participants, normalized per participant to their largest observed HPA. While most participants demonstrated an increase in hand opening following block for most conditions, this behavior was not ubiquitous in our 9-person sample (see S05 and S07 in Figure 7 and Table 2). The block had its largest and most consistent effect for FES-elicited contractions while supporting a load at the shoulder.
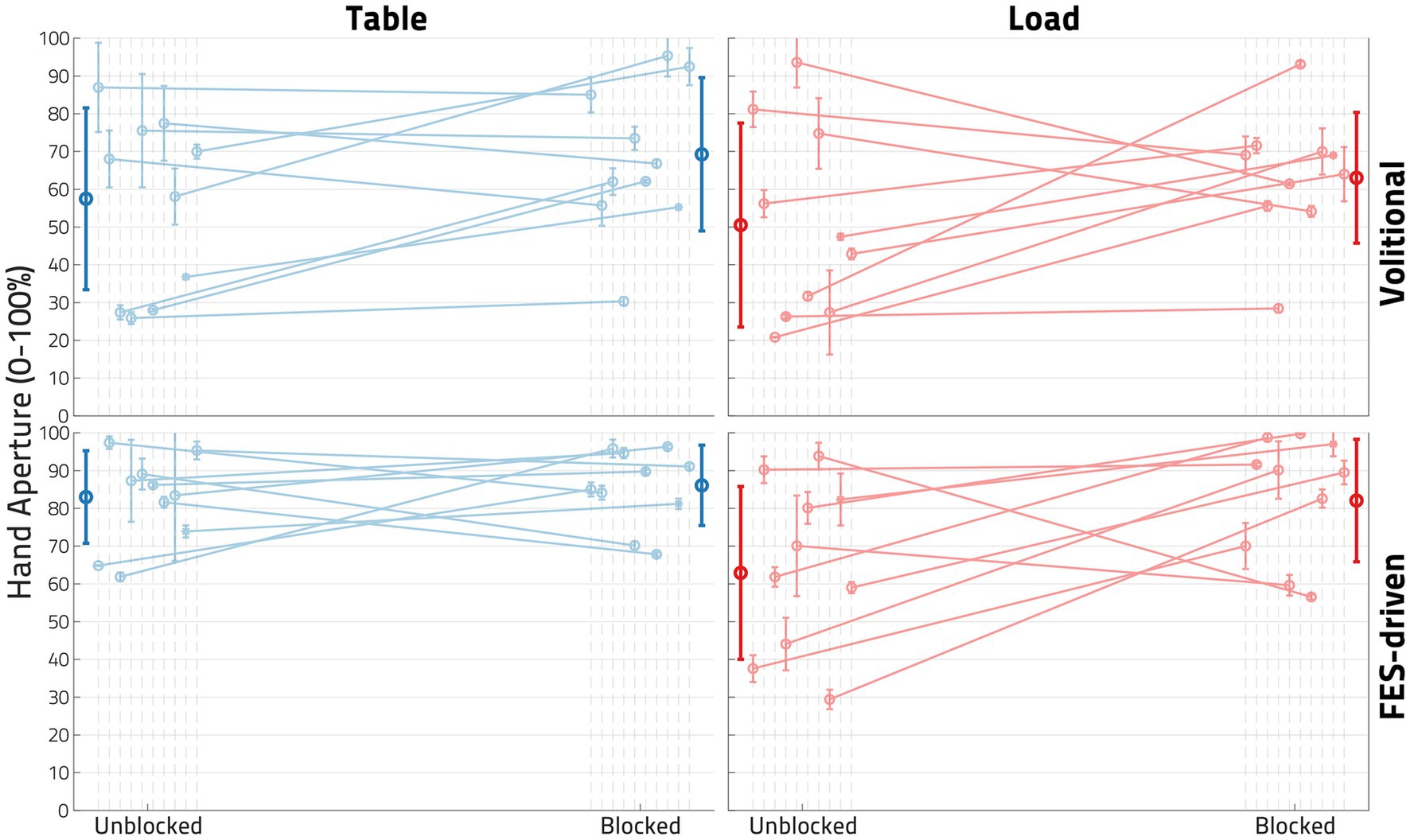
Figure 7. Impact of block on hand opening. In each plot, each dot represents the normalized mean hand pentagon area (HPA) from the trials of one participant in the denoted conditions, with the error bars denoting standard deviations. The left column of plots showcases the Unloaded (or table) condition, while the right represents the Loaded condition. The top row shows the Volitional condition, while the bottom row shows the functional electrical stimulation (FES)-driven condition. Four linear mixed effects regression (LMER) models were created for the four plots shown. In the bottom right plot-the FES-driven w/load condition- the Block term was found to be statistically significant (p < 0.05).
Four LMER models were created to determine the impact of Block on hand opening under the conditions of interest (Loaded vs. Unloaded and Volitional vs. FES). While all four models found that Block had, when viewed across the 9 participants included, a positive impact on hand opening, only in the FES-driven and shoulder-Loaded condition was the effect found to be significant (p < 0.05). In this model, the coefficient of block was found to be 19.431, or a roughly 20% increase in hand opening following the application of the nerve block.
To verify that the nerve block reduced the impact of shoulder loading on FES hand opening, we performed an additional one-tailed paired t-test on the change in FES hand opening brought on by loading, shown in Table 4. The drop in FES hand opening induced by Load was found to be significantly less in the Blocked case than in the Unblocked case (p = 0.02198).
4 Discussion
4.1 Summary of findings and previous research
Our results demonstrated that Ropivacaine injection in the median and ulnar nerves induced a block of hand grasp in 9 out of 10 participants with moderate to severe stroke, averaging 75% of their maximum hand grasp force. Furthermore, we demonstrated that FES was able to produce flexion forces distal of the flexor nerve block sites. The effectiveness of FES distal to the nerve block injection sites is critical as it allows for the possibility of performing a functional hand task with FES assistance following a block. Most importantly, this study has shown that blocking the median and ulnar nerves responsible for wrist and finger flexion can improve FES-assisted hand opening outcomes even during shoulder abduction loading conditions. Previous literature had demonstrated the potential of nerve block approaches to address abnormal passive and active torques at the first MCP joint (4). Our current study demonstrated the effect of nerve block on a more functionally relevant measurement of hand opening (HPA), and, for the first time, in reducing the detrimental impact of shoulder abduction loading induced flexion synergy on hand opening.
Assistive FES has been employed to improve hand opening in individuals with stroke (13), but its functionality has been significantly limited by flexion synergy expression. There have been multiple attempts to reduce this synergy expression to increase hand opening outcomes, such as by also utilizing FES for shoulder muscles to reduce synergy presentation (15), or designing arm support devices (44) such as the SaeboMAS. The combination of flexor nerve block and extensor FES shown here addresses these prior limitations with FES interventions and improves the feasibility of using modern FES approaches (14) to enhance hand function following stroke, even in the most severely impaired individuals with tremendous extensor weakness.
4.2 Study limitations and future work
4.2.1 Sample size
The original power analysis (G*Power, Heinrich-Heine-Universität, Dusseldorf, Germany) for this proof-of-concept study used an estimated effect size and standard deviation based on work that used an alternate measure of load at the shoulder (see 4.2.2). While 9 participants were sufficient to establish statistical significance in one set of conditions, a larger sample size could provide greater clarity on the impact of nerve block across the wide level of impairment encompassed by our participants. Of note is that the impact of nerve block on Volitional hand opening was still quite staggering in some individuals (such as S06), suggesting that some individuals could already benefit tremendously from nerve block alone without any assistance from FES. Determining which individuals may see such outcomes depends not only on a better understanding of mechanisms (4.2.5) but also on a larger sample size.
4.2.2 The effect of shoulder abduction loading
This study included trials with loading at the shoulder to evaluate the ability of nerve block to reduce the negative consequences of the flexion synergy on hand opening (2, 8). We studied two simple loading conditions: Unloaded, or when the participant was resting on a haptic table generated by the ACT3D, and Loaded, when the participant had to lift the full weight of their limb (100% limb weight, or 100%LW, as used in prior studies (41)). Future studies should more completely take into account the varying levels of participant impairment and shoulder strength so as to remove the variability introduced by relying upon participant limb weight. One such metric that better normalizes results between participants of varying levels of impairment, strength, and limb weight is percentage of maximum voluntary torque (MVT) expression at the shoulder (45, 46); using metrics such as these should reduce the variability in resulting data and may provide greater insight into the variation in efficacy of nerve block in hand opening across different levels of stroke impairment.
4.2.3 Block success and impairment levels
Block success was determined by measuring the drop in volitional hand closing forces following the administration of ropivacaine using a within-subject comparison t-test. Nine out of 10 participants indeed showed large drops in volitional hand closing forces, averaging 75%. Some participants had particularly low volitional grasp forces prior to the nerve block as compared to those generated by FES, which made determining block efficacy in these participants more difficult. We believe that S09’s failure to reach statistical significance and the 50% drop cutoff is due, in part, to the reduced dynamic range of the pressure mat at S09’s lower grasp force levels. Although ropivacaine did not result in significantly reduced hand closing in S09 (for which their data was excluded from further statistical analysis), we still observed a ~60% increase in hand opening from S09 following the nerve block, indicating that this block improved hand opening (S09’s data in the figures is denoted by an asterisk).
Regarding FES hand closing we observed minor, but statistically significant, variations in grasp forces before and after nerve block in 6 individuals (some increasing and others decreasing). The Unblock and Block FES Close cases have 2+ hours between them; many changes, such as fatigue and electrode site property changes, could occur during this period that can explain the change in grasp force. The statistical analysis based on the group data (see Table 3) did not show significant change in FES-induced flexion force. This result-that FES efficacy downstream of a nerve block site would perform similarly to the unblocked condition— was anticipated and supported by current understanding of the mechanisms of FES stimulation of muscle, but no prior scientific literature has technically demonstrated this. Now that this has been shown, FES assistance for flexors could arguably be applied alongside nerve block approaches in future interventions that might be unable to provide the required partial blocking or immediate on/off control of flexors necessary for an intervention useful for activities of daily living.
4.2.4 Mechanical side effects of nerve block
Though the LMER models showed a significant increase in FES hand opening ability during Load across our 9 participants, a few participants (S05 and S07) exhibited a decrease in FES or Volitional hand opening following the nerve block (Figure 7 and Table 2). We have considered two possible explanations for this: Firstly, ropivacaine nerve blocks affect not only motor, but also sensory nerve fibers, and the impact of this loss of afferent information in the spinal motor neuron loop on antagonist (extensor) behavior is not entirely clear. Secondly, loss of intrinsic hand muscles has often impaired FES hand outcomes in Spinal Cord Injury interventions, resulting in “claw hand” (47). This presents as strong finger flexion at the second and third MCP joint, reducing the HPA and thereby the potential for grasp functionality. We had occasionally observed such presentations in some of our participants, but our HPA metric did not take the orientation of the sensor into account. Future work could potentially omit ulnar nerve block or include FES of intrinsic hand muscles. Using implanted FES electrodes would, in general, provide precise, selective activation of the hand muscles needed to provide a more normal hand grasp pattern (48).
4.2.5 Disability mechanism contributions
Using electromyography (EMG) signals of relevant musculature as well as by calculating the purely flexion synergy-driven grasp forces exerted on the pressure mat during lifting, we next plan to analyze more directly the impact of the nerve block on certain known mechanisms of stroke disability. Of particular interest are the impacts of nerve block on the expression of hypertonia (tonic activation of wrist and finger flexors even while at rest), co-contraction (simultaneous activation of wrist and finger flexors and extensors during certain tasks (8)), and the expression of the flexion synergy (activation of wrist, finger, and elbow flexors proportional to the activity of shoulder abductors). This could help to explain why some participants improved in hand opening while others did not.
4.2.6 Electrical nerve block
There are a variety of electrically driven nerve block approaches currently in development (33) that could perform a similar function as Ropivacaine did in this study. KiloHertz frequency alternating current in particular (KHFAC) could provide a means for user-controlled, on/off, instant, and reversible flexor nerve block. Some studies have also demonstrated the potential for partial blocks using KHFAC (49). Regardless of whether managed by KHFAC block alone, or combined with newer emerging DC block approaches (50), a temporary and instant reduction of flexor activity combined with FES-assisted extension could provide a permanent solution to functional losses at the hand in individuals with moderate to severe chronic stroke.
4.2.7 Alternate hand ability metrics
More work needs to be done to evaluate the impact of a general increase in hand opening (as measured by HPA) on activities of daily living. The “clawhand” presentation we observed in some participants may limit functional gain for certain individuals. A possible means to better account for “clawhand” while measuring hand aperture could be a hand hexagon area (HHA), where an additional sensor on the center of the back of the hand could serve as a reference (ref) for four triangles: ref-thumb-index, ref-index-middle, ref-middle-ring, and ref-ring-pinky. Lastly, comparing HPA, HHA, or any other hand-opening metrics against functional tests such as box and blocks or clothespin task could provide greater insight into the true value of a combination nerve block and assistive FES approach.
5 Conclusion
Blocking undesirable and abnormal hand flexor contractions in individuals following hemiparetic stroke using local anesthesia of the median and ulnar nerves was shown to improve the ability of most individuals to open their hands using assistive functional electrical stimulation of the hand extensor muscles. These results indicate that controllable and deployable methods for blocking peripheral nerves, such as electrical block, may facilitate the deployment of better, proven FES methods for hand functional restoration for individuals with hemiparetic stroke.
Data availability statement
The raw data supporting the conclusions of this article will be made available by the authors, without undue reservation, upon request.
Ethics statement
The studies involving humans were approved by Northwestern University Institutional Review Board (IRB #STU00213403). The studies were conducted in accordance with the local legislation and institutional requirements. The participants provided their written informed consent to participate in this study. Written informed consent was obtained from the participants for the publication of any potentially identifiable images or data included in this article.
Author contributions
HD: Conceptualization, Data curation, Formal analysis, Investigation, Methodology, Project administration, Software, Writing – original draft, Writing – review & editing, Resources. JY: Data curation, Formal analysis, Methodology, Project administration, Supervision, Writing – review & editing. JD: Investigation, Methodology, Writing – review & editing, Conceptualization, Funding acquisition, Project administration, Resources, Supervision. AN: Conceptualization, Methodology, Writing – review & editing, Investigation. RK: Conceptualization, Formal analysis, Funding acquisition, Investigation, Methodology, Project administration, Resources, Supervision, Writing – review & editing.
Funding
The author(s) declare financial support was received for the research, authorship, and/or publication of this article. Research reported in this publication was supported by the Department of Veterans Affairs Office of Rehabilitation Research and Development grants B9281-S and B4853C, the National Institute of Child Health and Human Development grants R01-HD095187 and R01-HD039343, and the National Institute of Neurological Disorders and Stroke grant R01-NS105759. This research was also supported by funds from Case Western Reserve University’s Department of Biomedical Engineering and Northwestern University’s Department of Physical Therapy and Human Movement Sciences.
Acknowledgments
The authors would like to thank all the following individuals for their help. For helping with experiments: Albert Chan, Jane Gyarmaty, Rachel Elder, and Alex Samworth. For helping with information on botox use in the clinic: John Chae. For helping with recruitment and billing: Bessie Cofield, Brad Holubar, Carolina Carmona, Albert Chan, Drew Beauchamp, and Shirley Ryan AbilityLab Clinical Research Registry (Heidi Roth, Jennifer Kahn, and Carolyn Ostrowski). For helping with stats: Ana Maria Acosta, Hongchul Sohn, and Liangliang Zhang. For helping with figures: Emily Imka from Cleveland FES Center. And most importantly, endless thanks to our 10 participants.
Conflict of interest
The authors declare that the research was conducted in the absence of any commercial or financial relationships that could be construed as a potential conflict of interest.
The author declares that they were an editorial board member of Frontiers, at the time of submission. This had no impact on the peer review process and the final decision.
Publisher’s note
All claims expressed in this article are solely those of the authors and do not necessarily represent those of their affiliated organizations, or those of the publisher, the editors and the reviewers. Any product that may be evaluated in this article, or claim that may be made by its manufacturer, is not guaranteed or endorsed by the publisher.
References
1. Tsao, CW, Aday, AW, Almarzooq, ZI, Anderson, CAM, Arora, P, Avery, CL, et al. Heart disease and stroke Statistics-2023 update: a report from the American Heart Association. Circulation. (2023) 147:e93–e621. doi: 10.1161/CIR.0000000000001123
2. Lan, Y, Yao, J, and Dewald, JPA. The impact of shoulder abduction loading on volitional hand opening and grasping in chronic hemiparetic stroke. Neurorehabil Neural Repair. (2017) 31:521–9. doi: 10.1177/1545968317697033
3. Li, S, Kamper, DG, and Rymer, WZ. Effects of changing wrist positions on finger flexor hypertonia in stroke survivors. Muscle Nerve. (2006) 33:183–90. doi: 10.1002/mus.20453
4. Kamper, DG, Harvey, RL, Suresh, S, and Rymer, WZ. Relative contributions of neural mechanisms versus muscle mechanics in promoting finger extension deficits following stroke. Muscle Nerve. (2003) 28:309–18. doi: 10.1002/mus.10443
5. Kamper, DG, Fischer, HC, Cruz, EG, and Rymer, WZ. Weakness is the primary contributor to finger impairment in chronic stroke. Arch Phys Med Rehabil. (2006) 87:1262–9. doi: 10.1016/j.apmr.2006.05.013
6. Brunnstrom, S. (1970). Movement therapy in hemiplegia: a neurophysiological approach. New York: Harper & Row.
7. Dewald, JP, Pope, PS, Given, JD, Buchanan, TS, and Rymer, WZ. Abnormal muscle coactivation patterns during isometric torque generation at the elbow and shoulder in hemiparetic subjects. Brain. (1995) 118:495–510. doi: 10.1093/brain/118.2.495
8. McPherson, LM, and Dewald, JPA. Abnormal synergies and associated reactions post-hemiparetic stroke reflect muscle activation patterns of brainstem motor pathways. Front Neurol. (2022) 13:934670. doi: 10.3389/fneur.2022.934670
9. McPherson, JG, Chen, A, Ellis, MD, Yao, J, Heckman, CJ, and Dewald, JPA. Progressive recruitment of contralesional cortico-reticulospinal pathways drives motor impairment post stroke. J Physiol. (2018a) 596:1211–25. doi: 10.1113/JP274968
10. Beauchamp, J, Hassan, A, Mcpherson, L, Negro, F, Pearcey, G, Cummings, M, et al. (2023) Motor unit firing rate modulation is more impaired during flexion synergy-driven contractions of the biceps brachii in chronic stroke. medRxiv. Available at: https://doi.org/10.1101/2023.11.22.23298905. [Epub ahead of preprint]
11. Ellis, MD, Schut, I, and Dewald, JPA. Flexion synergy overshadows flexor spasticity during reaching in chronic moderate to severe hemiparetic stroke. Clin Neurophysiol. (2017) 128:1308–14. doi: 10.1016/j.clinph.2017.04.028
12. Binder-Markey, BI, Murray, WM, and Dewald, JPA. Passive properties of the wrist and fingers following chronic hemiparetic stroke: Interlimb comparisons in persons with and without a clinical treatment history that includes botulinum neurotoxin. Front Neurol. (2021) 12:687624. doi: 10.3389/fneur.2021.687624
13. Chae, J, and Hart, R. Intramuscular hand neuroprosthesis for chronic stroke survivors. Neurorehabil Neural Repair. (2003) 17:109–17. doi: 10.1177/0888439003017002005
14. Knutson, JS, Chae, J, Hart, RL, Keith, MW, Hoyen, HA, Harley, MY, et al. Implanted neuroprosthesis for assisting arm and hand function after stroke: a case study. J Rehabil Res Dev. (2012) 49:1505–16. doi: 10.1682/JRRD.2011.09.0171
15. Makowski, NS, Knutson, JS, Chae, J, and Crago, PE. Functional electrical stimulation to augment poststroke reach and hand opening in the presence of voluntary effort: a pilot study. Neurorehabil Neural Repair. (2014) 28:241–9. doi: 10.1177/1545968313505913
16. Hunter, SM, and Crome, P. Hand function and stroke. Rev Clin Gerontol. (2002) 12:68–81. doi: 10.1017/S0959259802012194
17. Ellis, MD, Carmona, C, Drogos, J, and Dewald, JPA. Progressive abduction loading therapy with horizontal-plane viscous resistance targeting weakness and flexion synergy to treat upper limb function in chronic hemiparetic stroke: a randomized clinical trial. Front Neurol. (2018) 9:71. doi: 10.3389/fneur.2018.00071
18. Ellis, MD, Sukal-Moulton, T, and Dewald, JP. Progressive shoulder abduction loading is a crucial element of arm rehabilitation in chronic stroke. Neurorehabil Neural Repair. (2009) 23:862–9. doi: 10.1177/1545968309332927
19. Sommerfeld, DK, Gripenstedt, U, and Welmer, AK. Spasticity after stroke: an overview of prevalence, test instruments, and treatments. Am J Phys Med Rehabil. (2012) 91:814–20. doi: 10.1097/PHM.0b013e31825f13a3
20. Ward, AB, Aguilar, M, De Beyl, Z, Gedin, S, Kanovsky, P, Molteni, F, et al. Use of botulinum toxin type a in the management of adult spasticity—a European consensus statement. J Rehabil Med. (2003) 35:98–9. doi: 10.1080/16501970306112
21. Foley, N, Pereira, S, Salter, K, Fernandez, MM, Speechley, M, Sequeira, K, et al. Treatment with botulinum toxin improves upper-extremity function post stroke: a systematic review and meta-analysis. Arch Phys Med Rehabil. (2013) 94:977–89. doi: 10.1016/j.apmr.2012.12.006
22. Sampaio, C, Ferreira, JJ, Pinto, AA, Crespo, M, Ferro, JM, and Castro-Caldas, A. Botulinum toxin type a for the treatment of arm and hand spasticity in stroke patients. Clin Rehabil. (1997) 11:3–7. doi: 10.1177/026921559701100102
23. Shaw, LC, Price, CI, van Wijck, FM, Shackley, P, Steen, N, Barnes, MP, et al. Botulinum toxin for the upper limb after stroke (BoTULS) trial: effect on impairment, activity limitation, and pain. Stroke. (2011) 42:1371–9. doi: 10.1161/STROKEAHA.110.582197
24. Ojardias, E, Ollier, E, Lafaie, L, Celarier, T, Giraux, P, and Bertoletti, L. Time course response after single injection of botulinum toxin to treat spasticity after stroke: systematic review with pharmacodynamic model-based meta-analysis. Ann Phys Rehabil Med. (2022) 65:101579. doi: 10.1016/j.rehab.2021.101579
25. Turner-Stokes, L, Jacinto, J, Fheodoroff, K, Brashear, A, Maisonobe, P, and Lysandropoulos, A. Longitudinal goal attainment with integrated upper limb spasticity management including repeat injections of botulinum toxin a: findings from the prospective, observational upper limb international spasticity (ULIS-III) cohort study. J Rehabil Med. (2021) 53:jrm00157. doi: 10.2340/16501977-2801
26. Levy, J, Molteni, F, Cannaviello, G, Lansaman, T, Roche, N, and Bensmail, D. Does botulinum toxin treatment improve upper limb active function? Ann Phys Rehabil Med. (2019) 62:234–40. doi: 10.1016/j.rehab.2018.05.1320
27. Chen, YT, Zhang, C, Liu, Y, Magat, E, Verduzco-Gutierrez, M, Francisco, GE, et al. The effects of botulinum toxin injections on spasticity and motor performance in chronic stroke with spastic hemiplegia. Toxins. (2020) 12:492. doi: 10.3390/toxins12080492
28. Rahman, E, Banerjee, PS, Asghar, A, Gupta, NK, and Mosahebi, A. Botulinum toxin type a immunogenicity across multiple indications: an overview systematic review. Plast Reconstr Surg. (2022) 149:837–48. doi: 10.1097/PRS.0000000000008904
29. Minamoto, VB, Suzuki, KP, Bremner, SN, Lieber, RL, and Ward, SR. Dramatic changes in muscle contractile and structural properties after 2 botulinum toxin injections. Muscle Nerve. (2015) 52:649–57. doi: 10.1002/mus.24576
30. Pingel, J, Wienecke, J, Lorentzen, J, and Nielsen, JB. Botulinum toxin injection causes hyper-reflexia and increased muscle stiffness of the triceps surae muscle in the rat. J Neurophysiol. (2016) 116:2615–23. doi: 10.1152/jn.00452.2016
31. Thacker, BE, Tomiya, A, Hulst, JB, Suzuki, KP, Bremner, SN, Gastwirt, RF, et al. Passive mechanical properties and related proteins change with botulinum neurotoxin a injection of normal skeletal muscle. J Orthop Res. (2012) 30:497–502. doi: 10.1002/jor.21533
32. Ward, SR, Minamoto, VB, Suzuki, KP, Hulst, JB, Bremner, SN, and Lieber, RL. Recovery of rat muscle size but not function more than 1 year after a single botulinum toxin injection. Muscle Nerve. (2018) 57:435–41. doi: 10.1002/mus.25707
33. Bhadra, N, Vrabec, TL, Bhadra, N, and Kilgore, KL. Reversible conduction block in peripheral nerve using electrical waveforms. Bioelectron Med. (2018) 1:39–54. doi: 10.2217/bem-2017-0004
34. Kilgore, KL, and Bhadra, N. Reversible nerve conduction block using kilohertz frequency alternating current. Neuromodulation. (2014) 17:242–55. doi: 10.1111/ner.12100
35. Vrabec, T, Bhadra, N, Wainright, J, and Kilgore, K. Non-damaging nerve conduction block using direct current. Int FES Soc. (2012):4–6.
36. Koraki, E, Stachtari, C, Kapsokalyvas, I, Stergiouda, Z, Katsanevaki, A, and Trikoupi, A. Dexmedetomidine as an adjuvant to 0.5% ropivacaine in ultrasound-guided axillary brachial plexus block. J Clin Pharm Ther. (2018) 43:348–52. doi: 10.1111/jcpt.12657
37. Fresenius Kabi (2018) Naropin ® (ropivacaine HCl) injection. Fresenius Kabi. Available at: https://www.accessdata.fda.gov/drugsatfda_docs/label/2018/020533s035lbl.pdf. (Accessed August 7, 2023)
38. Safa, B, Flynn, B, McHardy, PG, Kiss, A, Haslam, L, Henry, PD, et al. Comparison of the analgesic duration of 0.5% bupivacaine with 1:200,000 epinephrine versus 0.5% ropivacaine versus 1% ropivacaine for low-volume ultrasound-guided interscalene brachial plexus block: a randomized controlled trial. Anesth Analg. (2021) 132:1129–37. doi: 10.1213/ANE.0000000000005373
39. Dewald, JP, Ellis, MD, Acosta, AM, Sohn, MH, and Plaisier, TA. Implementation of impairment-based neurorehabilitation devices and technologies following brain injury In: Neurorehabilitation technology. Cham: Springer (2022). 89–112.
40. Sukal, TM, Ellis, MD, and Dewald, JPA. (2005). Dynamic characterization of upper limb discoordination following hemiparetic stroke. IEEE 9th International Conference on Rehabilitation Robotics. Chicago, IL
41. Sukal, TM, Ellis, MD, and Dewald, JP. Shoulder abduction-induced reductions in reaching work area following hemiparetic stroke: neuroscientific implications. Exp Brain Res. (2007) 183:215–23. doi: 10.1007/s00221-007-1029-6
42. Gregory, CM, Dixon, W, and Bickel, CS. Impact of varying pulse frequency and duration on muscle torque production and fatigue. Muscle Nerve. (2007) 35:504–9. doi: 10.1002/mus.20710
43. Wilson, RD, Page, SJ, Delahanty, M, Knutson, JS, Gunzler, DD, Sheffler, LR, et al. Upper-limb recovery after stroke: a randomized controlled trial comparing EMG-triggered, cyclic, and sensory electrical stimulation. Neurorehabil Neural Repair. (2016) 30:978–87. doi: 10.1177/1545968316650278
44. Ghassemi, M, and Kamper, DG. The hand after stroke and SCI: restoration of function with technology In: Neurorehabilitation technology. Cham: Springer (2022). 113–34.
45. McPherson, JG, Stienen, AH, Drogos, J, and Dewald, JPA. (2011). The relationship between the flexion synergy and stretch reflexes in individuals with chronic hemiparetic stroke. IEEE International Conference on Rehabilitation Robotics. Zurich, Switzherland. 1–7.
46. McPherson, JG, Stienen, AH, Drogos, JM, and Dewald, JP. Modification of spastic stretch reflexes at the elbow by flexion synergy expression in individuals with chronic hemiparetic stroke. Arch Phys Med Rehabil. (2018b) 99:491–500. doi: 10.1016/j.apmr.2017.06.019
47. Binder-Markey, BI, Dewald, JPA, and Murray, WM. The biomechanical basis of the claw finger deformity: a computational simulation study. J Hand Surg Am. (2019) 44:751–61. doi: 10.1016/j.jhsa.2019.05.007
48. Kilgore, KL, Anderson, KD, and Peckham, PH. Neuroprosthesis for individuals with spinal cord injury. Neurol Res. (2020) 45:893–905. doi: 10.1080/01616412.2020.1798106
49. Bhadra, N, and Kilgore, KL. High-frequency electrical conduction block of mammalian peripheral motor nerve. Muscle Nerve. (2005) 32:782–90. doi: 10.1002/mus.20428
50. Franke, M, Vrabec, T, Wainright, J, Bhadra, N, Bhadra, N, and Kilgore, K. Combined KHFAC + DC nerve block without onset or reduced nerve conductivity after block. J Neural Eng. (2014) 11:056012. doi: 10.1088/1741-2560/11/5/056012
Glossary of Acronyms
Keywords: nerve block, stroke, anesthesia, hand opening, grasp, FES, upper extremity synergies, paresis
Citation: Dewald HA, Yao J, Dewald JPA, Nader A and Kirsch RF (2024) Peripheral nerve blocks of wrist and finger flexors can increase hand opening in chronic hemiparetic stroke. Front. Neurol. 15:1284780. doi: 10.3389/fneur.2024.1284780
Edited by:
Marianna Semprini, Italian Institute of Technology (IIT), ItalyReviewed by:
Hewei Wang, Fudan University, ChinaJeanette Plantin, Karolinska Institutet (KI), Sweden
Copyright © 2024 Dewald, Yao, Dewald, Nader and Kirsch. This is an open-access article distributed under the terms of the Creative Commons Attribution License (CC BY). The use, distribution or reproduction in other forums is permitted, provided the original author(s) and the copyright owner(s) are credited and that the original publication in this journal is cited, in accordance with accepted academic practice. No use, distribution or reproduction is permitted which does not comply with these terms.
*Correspondence: Hendrik A. Dewald, aGFkMjdAY2FzZS5lZHU=