- 1Institute of Medical Science, University of Toronto, Toronto, ON, Canada
- 2Krembil Research Institute, University Health Network, Toronto, ON, Canada
- 3Division of Neurology, University of Toronto, Toronto, ON, Canada
Introduction: Patients with Neurofibromatosis type 1 (NF1), the most common neurocutaneous disorder, can develop several neurological manifestations that include cognitive impairments and epilepsy over their lifetime. It is unclear why certain patients with NF1 develop these conditions while others do not. Early-life immune activation promotes later-life seizure susceptibility, neurocognitive impairments, and leads to spontaneous seizures in some animal models of neurodevelopmental disorders, but the central nervous system immune profile and the enduring consequences of early-life immune activation on the developmental trajectory of the brain in NF1 have not yet been explored. We tested the hypothesis that early-life immune activation promotes the development of spatial memory impairments and epileptogenesis in a mouse model of NF1.
Methods: Male wild-type (WT) and Nf1+/− mice received systemic lipopolysaccharide (LPS) or saline at post-natal day 10 and were assessed in adulthood for learning and memory deficits in the Barnes maze and underwent EEG recordings to look for spontaneous epileptiform abnormalities and susceptibility to challenge with pentylenetetrazole (PTZ).
Results: Whereas early-life immune activation by a single injection of LPS acutely elicited a comparable brain cytokine signature in WT and Nf1+/− mice, it promoted spontaneous seizure activity in adulthood only in the Nf1+/− mice. Early-life immune activation affected susceptibility to PTZ-induced seizures similarly in both WT and Nf1+/−mice. There was no effect on spatial learning and memory regardless of mouse genotype.
Discussion: Our findings suggest second-hit environmental events such as early-life immune activation may promote epileptogenesis in the Nf1+/− mouse and may be a risk-factor for NF1-associated epilepsy.
1 Introduction
Neurofibromatosis type 1 (NF1) is a neurocutaneous disorder caused by autosomal dominant germline mutations to the NF1 gene (1, 2), a tumor suppressor gene on chromosome 17 encoding the protein neurofibromin. Neurofibromin is a multi-domain protein that is best characterized for its role as a Ras-guanosine triphosphate activation protein (Ras-GAP) (3). NF1-associated pathogenic mutations lead to reduced or complete absence of neurofibromin, promoting constitutive activation of GTP-Ras and overactivation of cell proliferation and survival pathways. NF1 is a progressive condition and patients often exhibit a wide range of dermatological, cardiovascular, and musculoskeletal comorbidities which present gradually over their lifetime, severely affecting their quality of life. Pigmentary lesions such as neurofibromas and café au lait macules (CALMs) are the most common clinical manifestations of the disease (4). Less understood are the neurological manifestations of NF1, which include cognitive impairments and epilepsy.
Epilepsy occurs at a higher incidence in NF1 patients (5–11%) (5, 7) than in the general population (1–2%) (8). Whereas structural abnormalities have been associated with seizures in some cases (5, 9, 10), they do not account for the manifestation of seizures in all NF1-associated epilepsy cases. For instance, only 50% of reported NF1-associated epilepsy cases are structural epilepsies resulting from brain abnormalities detectable on neuroimaging (5) and structural NF1-associated lesions on MRI do not always co-localize with epileptiform discharges on EEG (11). In fact, many reports of NF1-associated epilepsy lack detailed electroencephalographic (EEG), clinical, and brain imaging correlates that would confirm the epileptogenicity of a structural lesion (5, 12). This underscores an uncertainty around whether an underlying structural lesion is always the true epileptogenic basis of NF1 epilepsy, or whether there are molecular alterations, driven by disease-associated loss-of-function mutations in Nf1, that mediate NF1-associated hyperexcitability. Until very recently, research on seizure susceptibility and epileptogenesis in NF1 was lacking. Recent work from our group with the non-lesional Nf1+/− mouse model demonstrated increased seizure susceptibility and increased rates of epileptogenesis (13, 14).
In addition to epilepsy, cognitive impairments are also a common neurological manifestation of NF1, occurring in up to 65% of patients (15) and including learning disabilities, attention deficits, and social perception problems (16). Animal models of NF1, including the non-lesional Nf1+/− mouse, replicate these learning impairments in attention and spatial memory tests (17, 18), and have been shown to be mediated by RAS-hyperactivity downstream of neurofibromin, which promotes alterations in GABAergic and dopaminergic function (19–21). It is unclear why some patients with NF1 go on to develop cognitive impairments and epilepsy, while others do not.
There is an abundance of clinical and experimental evidence suggesting neuroinflammation is involved in the pathogenesis of seizures and may promote cognitive impairments in learning and memory (22, 23). In particular, epidemiological studies have suggested exposure of the developing fetus to immune challenges sustained in the mother during early to mid-pregnancy result in the greatest risk in the offspring for epilepsy (24, 25) and neurodevelopmental disorders, such as autism and schizophrenia (26, 27). Similarly, early-life in vivo studies suggest that activating the innate immune system during a critical window between postnatal day (P)7–14 (equivalent to early human infancy) with systemic lipopolysaccharide (LPS) is associated with an increased later-life susceptibility to chemo-convulsant induced seizure activity (28–30) and altered expression of NMDA receptor subunits (30), with mixed effects on hippocampal dependent memory (28, 31). Early-life systemic immune activation in a mouse model of autism spectrum disorder (ASD) has also been shown to promote spontaneous seizure activity in adulthood (32).
There are reports of an increased level of peripheral inflammation in patients with NF1 (33–36). It is therefore intriguing to determine if immune activation in NF1, particularly early in life, is associated with an increased susceptibility to cognitive impairments and seizures. In the present study we used the non-lesional Nf1+/− mouse model to determine whether early-life immune activation exacerbates the seizure susceptibility and spatial memory impairments associated with NF1.
2 Materials and methods
Experiments were performed in accordance with the policies and guidelines established by the Canadian Council on Animal Care and ethical approval from the University Health Network-Animal Resources Centre. Nf1+/− mice (strain #008192) were originally purchased from Jackson Laboratory (Bar Harbor, ME, United States), and bred in house. The mutation was originally generated on a 129S2/SvPas background and then backcrossed to a C57BL/6 background more than twelve times by the donating lab. Nf1+/+ littermates were designated as the WT controls and are henceforth referred to as such. Only male offspring mice were used in this study. The colony was maintained under standard specific pathogen-free conditions at 20–21°C on a 12 h light/dark cycle with food and water available ad libitum. To mitigate potential litter effects, 14 litters from a total of 13 different breeding pairs contributed pups to this study. An overview of the experimental design is presented in Figure 1.
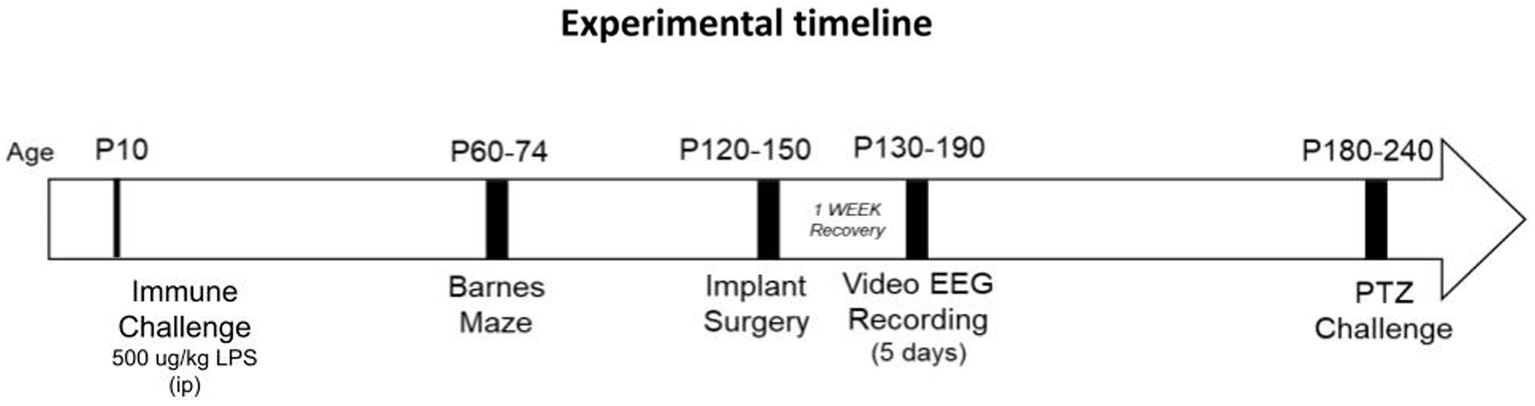
Figure 1. Experimental timeline. Postnatal day (p)10 mice were sacrificed four hours following LPS challenge for cytokine analysis on brain tissue. Some mice were allowed to mature after p10, for assessment of later-life spatial memory (between p60-p74), spontaneous recurrent seizure activity (p130-p190) and induced seizure activity (p180-p240).
2.1 Postnatal lipopolysaccharide challenge
P10 Nf1+/− and WT littermate male pups received a single intraperitoneal injection of 500 ug/kg lipopolysaccharide (Escherichia coli, serotype O26:B6, Sigma-Aldrich, Oakville, Canada) or a matched volume of 0.9% saline solution (sterile vehicle). Whenever possible, both Nf1+/− and WT males were used from each litter. Whereas a milder dose of 100 ug/kg LPS has been reported to induce early-life immune activation in rodents (29, 32), our own preliminary experiments with this dose did not reveal any changes in brain cytokine levels of LPS-challenged versus saline-treated WT and Nf1+/− controls. Therefore, to induce immune activation, the dose of LPS was increased to 500 ug/kg (100 ug/mL in sterile normal saline). Immediately following injection, all mice were returned to their dams with cages places on heating pads. Saline control and immune-challenged pups were included in each litter to minimize inter-litter variation in maternal care. A subset of mice were sacrificed four hours after saline or LPS injection for analysis of brain pro-inflammatory cytokine levels. The other subset was used for behavioral studies and EEG recordings in adulthood. These mice were weaned at P21 and housed with up to five same-sex littermates with different genotype and postnatal treatment combinations, at which point the experimenter remained blind to the experimental groups.
2.2 Neuroinflammatory cytokine profiling
Four hours following the immune challenge, whole-brain tissue (excluding cerebellum and olfactory accessories) was extracted on ice from P10 pups (n = 5 per group), flash frozen, and stored at −80°C. While we did not perform transcardial perfusion before harvesting the tissue, studies have shown this step does not affect brain cytokine concentrations (37). Brain tissue was mechanically homogenized by a cordless motor pellet mixer (#V8185-904; VWR, Mississauga, Canada) in a PBS-based cell lysis buffer complete with EDTA-free protease inhibitors (#04693159001; Roche, Mississauga, Canada) and 1 mmol/L phenylmethylsulfonyl fluoride (#PMSF123.5; BioShop, Toronto, Canada). Tissue lysates were analyzed for the concentration of total protein and assayed in a Mouse Magnetic Luminex Screening Assay (LXSAMSM, R&DSYSTEMS, Oakville, Canada) for the concentration of the following cytokines: TNF-α, IL-1β, IFN-γ, and IL-6. Refer to Appendix S1 for Supplementary methods.
2.3 Barnes maze
Between the P60-74 window, adolescent control and immune-challenged mice (n = 8–11 per group) were tested in the Barnes Maze to assess spatial learning and memory, as previously described (38). Mice were transported to the testing room 24 h before the first training day, and remained in their littermate cages, in order to habituate to the novel holding conditions. Mice were spatially trained for a total of 16 training trials over four days (acquisition phase), and short-term reference memory was tested once at 24 h following spatial acquisition. All groups were trained and tested during the light phase, and the maze was cleaned with 70% isopropyl alcohol between trials to prevent the transfer of olfactory cues. Smart TriWise video tracking system (Version 3.0.06, Panlab Harvard Apparatus, Massachusetts, United States) was used to record all trials and acquire the data. Refer to Appendix S1 for Supplementary methods outlining the experimental protocol and the acquired parameters.
2.4 Video-EEG monitoring
Mice were maintained in the vivarium after Barnes maze testing until a window between P120-150 for video-EEG monitoring, at which point five mice per group underwent implantation of intracranial electrodes. Briefly, mice were anesthetized with 2% isoflurane and fixed to a stereotaxic frame. The skull was exposed through a skin incision and small burr holes were drilled to implant a set of bipolar polyamide-insulated twisted stainless-steel wire electrodes (outer diameter 150 um; Plastics One, Roanoke, United States) in the CA3 region of the hippocampus (bregma: 2.5 mm posterior, 3.0 mm lateral, 3.0 mm depth) and the contralateral sensorimotor neocortex (2.0 mm posterior, 2.0 mm lateral, 1.5 mm depth). One reference electrode was implanted at a frontal area (1.0 mm anterior, 1.0 mm lateral, 0.5 mm depth). Following surgery, mice were allowed to recover for one week before video-EEG monitoring commenced, as previously described (13).
Video EEG-monitoring was conducted between P130-190. The implanted mice were placed individually in modified cages with food and water ad libitium. A slip-ring commutator was mounted on top of the cage and connected to the mouse through a flexible cable which was of sufficient length to allow the mouse to move freely throughout the cage. The EEG signal was sampled by one of two sets of MP160 amplifiers (BIOPAC Systems, Inc., California, United States) with a sampling rate of either 250 Hz, bandpass filtered with a high-pass of 1.0 Hz and low-pass of 3.0 K Hz (MP160-194D) or a sampling rate of 1,000 Hz, bandpass filtered with a high-pass of 0.5 Hz and low-pass of 100 Hz (MP160-1A88). A webcam (LINKIT security web cameras; BIOPAC Systems, Inc., California, United States) was anchored next to each cage to continuously monitor the animal’s behavior. Video-recordings were time-stamped to synchronize with the EEG recordings, and were acquired, stored and analyzed using the AcqKnowledge software (Version 5.0; BIOPAC Systems, Inc., California, United States). Continuous recordings were carried out 24 h daily for 5 consecutive days. A two-hour epoch from each of the light and dark cycles was visually inspected for interictal spikes. Entire recordings were analyzed for spontaneous recurrent seizure activity. EEG analyses were confirmed by two independent reviewers to minimize bias. An interictal spike was defined as a highly contoured waveform that appeared at least twice the baseline amplitude and lasted between 20–70 ms. A seizure was defined as rhythmic discharge events with an amplitude greater than four times the baseline and lasting a minimum of five seconds. Any ictal spikes (occurring as part of a seizure) were not included in the separate interictal spike counts.
2.5 Pentylenetetrazol-induced seizure susceptibility
Between the P180-240 window, seizure susceptibility to the GABA antagonist pentylenetetrazol (PTZ) was assessed (n = 6–8 per group). In order to determine a mildly convulsive dose of PTZ that would enable us to differentiate the seizure susceptibility between our groups, a number of doses of PTZ were tested on naïve (i.e., have not undergone early-life immune challenge and were not implanted with intracranial electrodes) Nf1+/− and WT animals, and in a subset of experimental Nf1+/− and WT animals. Doses of 30, 40, 50 and 60 mg/kg of PTZ were tested. Based on preliminary findings of no seizures at lower doses, and status epilepticus followed by death at higher doses, a dose of 40 mg/kg of PTZ (i.p.; P6500-100G, Sigma-Aldrich, Oakville, Canada) was selected as a sub-convulsive dose, and mice were challenged as previously described (39). Immediately following injection, every animal was monitored for one hour and video-EEG recordings were acquired and visually inspected as described above. The latency to the first spike, the first epileptiform discharge event (EDE), and the first induced-seizure were determined. Spikes and seizures were defined as described above, EDEs were defined as high amplitude rhythmic discharges containing bursts of slow wave, spike–wave and/or polyspike wave discharges and lasting less than five seconds. Additionally, the total count of spikes, total count of EDEs, and cumulative induced-seizure duration during the period of monitoring were determined.
2.6 Statistical analysis
For all experiments, data are reported as mean ± standard error of the mean (SEM). GraphPad Prism 8 statistical software (Version 8.4.3, California, United States) was used for statistical analyses. QQ plots and the Shapiro–Wilk distribution test were performed to assess normality of residuals for all data sets. All residuals passed the normality tests (p > 0.05, data not shown), therefore we proceeded to parametric comparisons. Cytokine concentrations were analyzed in a two-way analysis of variance (ANOVA) followed by Sidak’s post-hoc analysis for group comparisons. Barnes Maze acquisition data were analyzed using a two-way repeated measures ANOVA. Dunnett’s post-hoc was applied for within-group differences and Tukey’s post-hoc was applied for between group differences on any one day. The assumption of equal variances (sphericity) was not assumed, and the data were corrected by the Greenhouse–Geisser correction. Barnes maze probe data, spontaneous epileptiform abnormalities, and PTZ susceptibility data were compared in a two-way ANOVA followed by Sidak’s post-hoc analysis for group comparisons. The proportion of animals that did/did not develop a seizure in response to PTZ were compared with a Chi-Square test. p < 0.05 was considered significant for all analyses.
3 Results
3.1 Postnatal LPS increases levels of brain TNF-α and IL-6 In Nf1+/− and WT mice
Analysis of brain tissue harvested four hours after injection revealed a main effect of immune challenge, with higher cytokine levels in the LPS versus saline-treated mice [IL-1β (F(1,16) = 9.881, p = 0.0063); TNF-α (F(1,16) = 21.70, p = 0.0003); IL-6 (F(1,16) = 24.65, p = 0.0001)] (Figure 2). Post-hoc analyses showed increased expression of IL-6 and TNF-α in both Nf1+/− (IL-6 p = 0.0056; TNF-α p = 0.0034) and WT (IL-6 p = 0.006; TNF-α p = 0.0246) mice. Brain IL-1β levels were significantly elevated only in Nf1+/− + LPS mice (p = 0.031), remaining comparable in WT + LPS vs. WT + Saline mice (p = 0.193). No between-group differences or any significant treatment x genotype interaction effects were observed for any of the assessed cytokines.
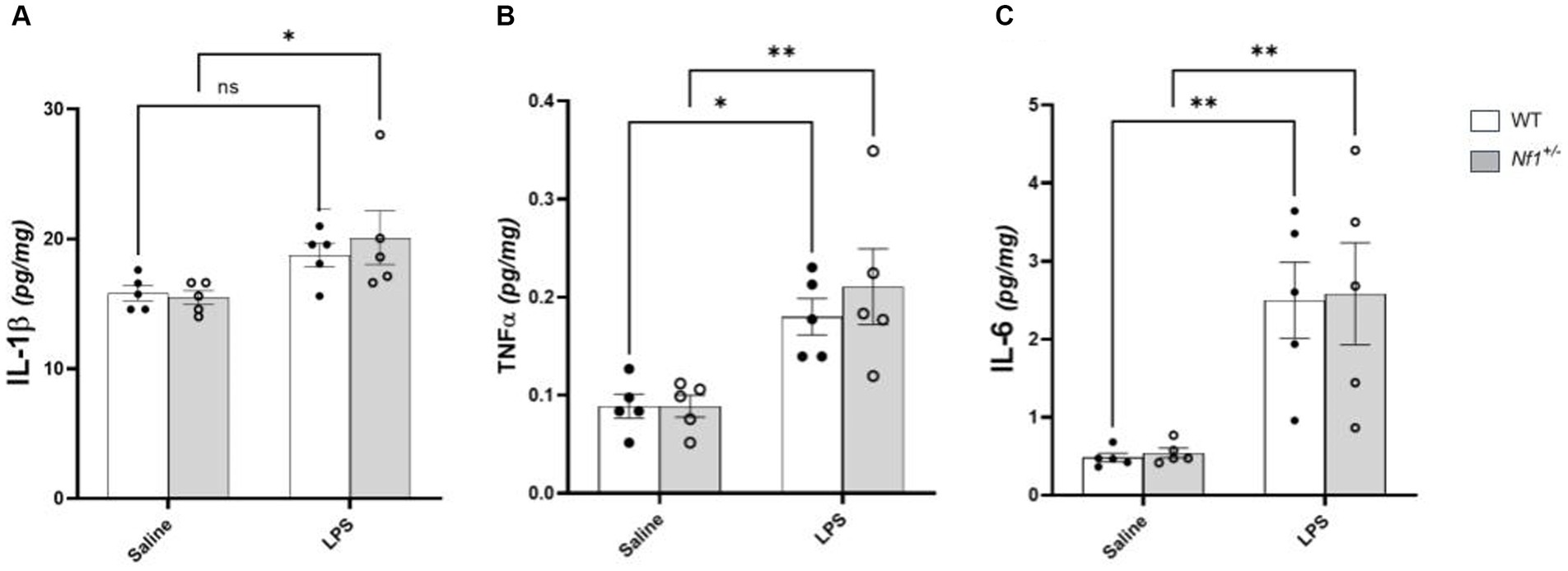
Figure 2. Postnatal LPS increases levels of brain TNF-α and IL-6 in Nf1+/− and WT mice. There was a significant increase in the concentration of the pro-inflammatory cytokines IL-1β (A), TNF-α (B), IL-6 (C) in whole-brain tissue collected four hours after LPS challenge in p10 Nf1+/− mice as compared to saline-treated controls. WT + LPS mice had similar increases in TNF-α (B) and IL-6 (C) compared with WT + Saline controls, but no significant increase in IL-1β concentration (A). No significant interaction effect of treatment x genotype for any cytokine was observed. Data represent mean concentrations (pg/mg) ± SEM. 2-WAY ANOVA, Sidak’s post-hoc,*p < 0.05 and **p < 0.01 compared to saline controls.
3.2 Early-life immune activation did not affect spatial learning
All groups demonstrated ability to learn the Barnes maze over a four day training period. WT + Saline and Nf1+/− + Saline mice had significantly shorter primary latencies (WT p = 0.003; Nf1+/− p = 0.001), shorter distances to the target (WT p = 0.002; Nf1+/− p = 0.009) and made fewer primary errors (WT p = 0.020; Nf1+/− p = 0.018) by Day 4 vs. Day 1 of training in the Barnes maze (Figure 3). Similarly, WT + LPS and Nf1+/− + LPS mice also exhibited shorter primary latencies (WT p < 0.001; Nf1+/− p = 0.001), fewer primary errors (WT p = 0.01; Nf1+/− p = 0.005) and shorter distance to the target (WT p = 0.002; Nf1+/− p = 0.002) by Day 4 vs. Day 1 of training (Figure 3). Lastly, all groups acquired the maze at a similar rate, regardless of genotype or treatment, considering that no significant between-group differences on any day of training in primary latency (Figure 3A), nor in distance to the target (Figure 3B) were noted. Still, Nf1+/− + LPS mice were observed to have made significantly more primary errors (Figure 3C) than WT + LPS mice on Day 2 of training (p = 0.022).
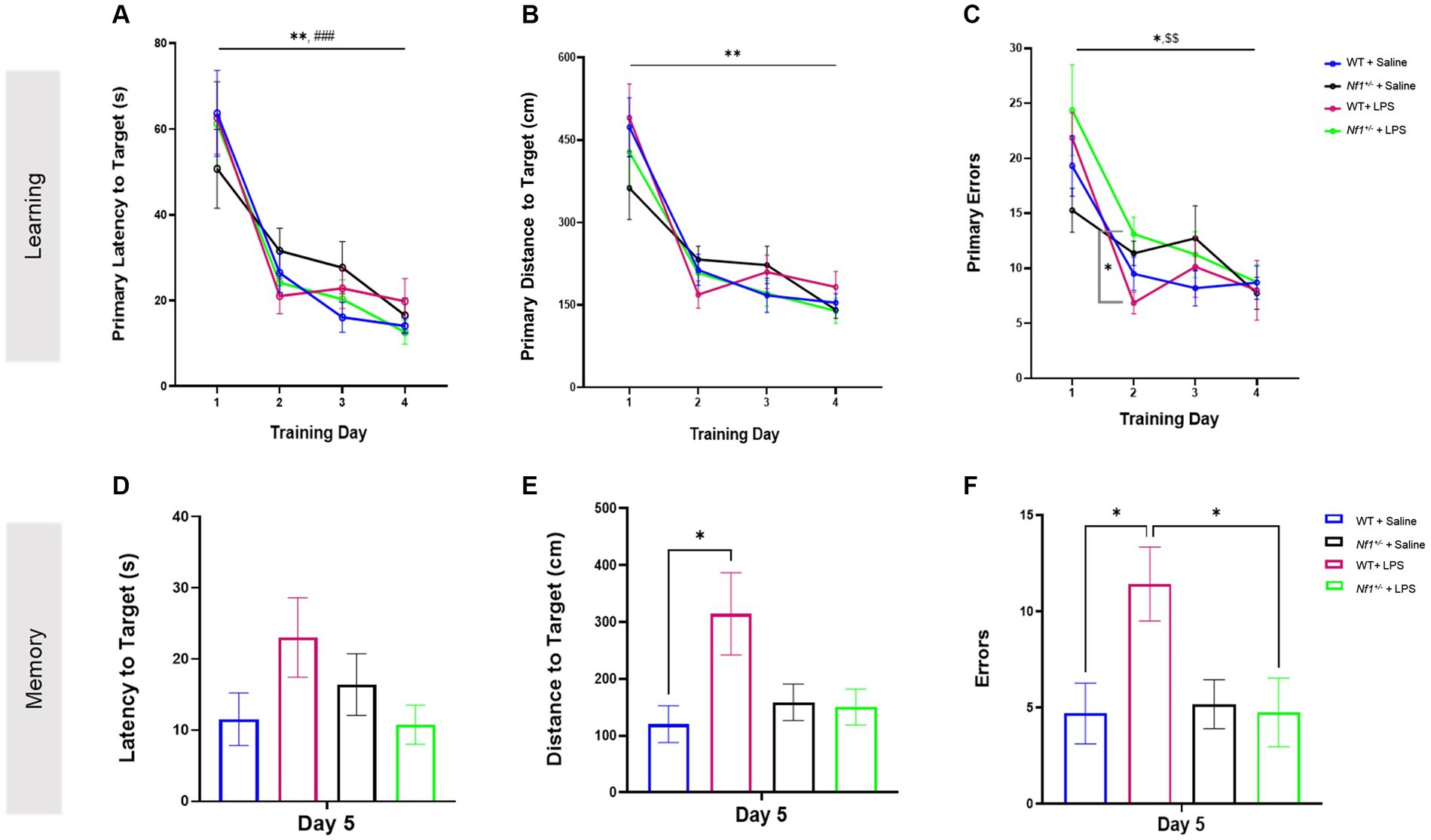
Figure 3. Early life inflammation does not affect spatial learning and memory in adult Nf1+/− mice. The WT + Saline, Nf1+/− + Saline, WT + LPS, and Nf1+/− + LPS groups all learned the Barnes maze as demonstrated by improvements in primary latency (s) to the target hole (A), primary distance travelled (CM) to the target hole (B), and number of primary errors (C) over the four training days. The training phase was followed by a probe trial to assess short-term spatial memory on Day 5. There were no differences in the latency to the target hole between any groups (D). The WT + LPS group travelled a significantly longer distance to reach the target hole (E) and made more errors (F) than the WT + Saline controls. Data are reported as the mean ± SEM. 2-WAY repeated measures ANOVA for analysis of the “Learning” phase. Dunnett’s post-hoc was applied for within-group differences and Tukey’s post-hoc was applied for between group differences on any one day. 2-WAY ANOVA, Sidak’s post-hoc for analysis of the “Memory” phase. [(*) was used to indicate that all groups were significantly different on Day 4 vs. Day 1: *p < 0.05, **p < 0.01, and ***p < 0.001 #p < 0 0.05. Specific within-group differences were represented with the following symbols: (#) for WT + Saline, ($) for Nf1+/− + Saline, (%) for Nf1+/− + LPS].
3.3 Early-life immune activation affected spatial memory in adult WT but not Nf1+/− mice
Short-term spatial reference memory was assessed 24 h after the last training trial (Day 5). There was no effect of genotype, and both WT + Saline and Nf1+/− + Saline mice demonstrated comparable short-term spatial memory evidenced by similar latency, distance, and total errors to the target hole (Figures 3D–F). There was an effect of early-life immune challenge in WT mice only, as WT + LPS mice traveled longer distances (p = 0.012) and made significantly more errors (p = 0.030) than WT + Saline mice. A similar effect of LPS was not seen in Nf1+/− mice. No between-group differences were observed in the latency to the target hole across all groups (Figure 3D). The time spent exploring the Target quadrant during the Barnes maze probe trial was also analyzed to capture other potential differences in performance. All groups demonstrated a degree of bias towards exploring the target quadrant over other maze quadrants, and the percentage of time mice spent exploring the Target quadrant was comparable across all groups regardless of genotype or treatment (Appendix S2; Supplementary Figure S1).
3.4 Early-life immune activation increased the number of spontaneous epileptiform spikes and promoted spontaneous seizure development in adult Nf1+/− but not WT mice
The number of spontaneous interictal epileptiform spikes was measured in epochs of EEG recordings including both light (Figure 4A) and dark cycles (Figure 4B). In the light cycle there was a significant effect of genotype with a greater number of total spikes in Nf1+/− mice (F(1,16) = 37.01, p < 0.0001) and a significant effect of early-life immune activation with a greater number of total spikes in LPS-treated mice (F(1,16) = 5.921, p = 0.0271). Multiple comparisons showed saline-treated Nf1+/− mice had significantly higher spike numbers than saline-treated WT mice (p = 0.0376). There was no effect of LPS in WT mice (p = 0.99940), whereas treatment with LPS significantly increased total spike number in Nf1+/− mice (p = 0.0284). There were also significantly more spikes in LPS-treated Nf1+/− versus WT mice (p = 0.0001). In the dark cycle, there was a significant effect of genotype with a greater number of average spikes in the Nf1+/− mice (F(1,16) = 13.63, p = 0.002). Post-hoc analysis revealed that LPS-challenged Nf1+/ mice had a significantly higher spike number than LPS-challenged WT mice (p = 0.0091).
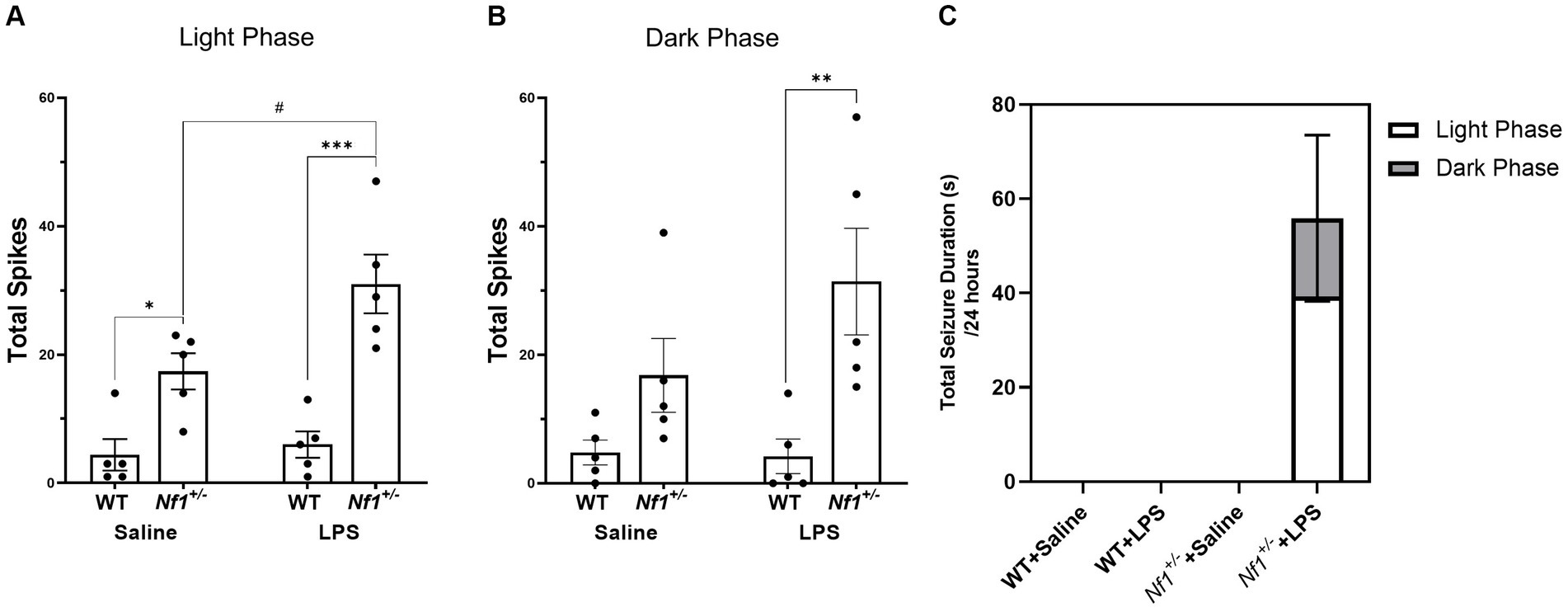
Figure 4. Early-life immune activation increases spike count and promotes spontaneous seizure activity in Nf1+/− mice. A two-hour epoch of EEG recording was analyzed during the light (10:00–12:00) (A) and dark cycle (22:00–24:00) (B) for each animal. The Nf1+/− + Saline group had significantly more interictal epileptiform spikes during the light cycle than the WT + Saline group (A). The Nf1+/− + LPS group had more spikes than the WT + LPS group in both the light and dark cycles (A,B). Spontaneous seizures were only detected in the Nf1+/− + LPS group (C). Data are presented as mean ± SEM. 2-WAY ANOVA, Sidak’s post-hoc,*p < 0.05, **p < 0.01, and ***p < 0.001 compared to another genotype group, #p < 0 0.05 compared to another treatment group.
Spontaneous seizure activity was not observed at any time in WT + Saline, WT + LPS, or Nf1+/+Saline mice. Spontaneous electrographic seizure activity was, however, observed in four out of five Nf1+/− + LPS mice (Figure 4C). In some cases, this was accompanied by freezing behaviour on video, but did not constitute convulsive behaviour. A greater proportion of seizures occurred during the light phase than the dark phase.
3.5 Early-life immune activation decreased the latency to the first spike after PTZ challenge
To assess hyperexcitability, we evaluated the proportion of animals that developed seizures in response to the PTZ challenge, as well as the latency to the first seizure, the cumulative seizure duration, the latency to the first spike, total spike count, the latency to the first EDE, and total EDE count in each group. An example of the different epileptiform events that were assessed is shown in Figure 5. As we used a subconvulsive dose of PTZ, not all animals developed electrographic seizures (i.e., seizures detected on EEG) following PTZ challenge, and animals that did develop electrographic seizures showed varying behavioral severity in seizure phenotype as assessed by the Racine scale. This ranged from stage 1 through stage 7 of behaviours, with two mice (one Nf1+/− + LPS and one WT + Saline) developing very severe seizures and dying from status epilepticus during testing. These were excluded from the analysis of the total number of spikes and EDEs but were included in the analysis of the latencies of these measures and any seizure-related parameters.
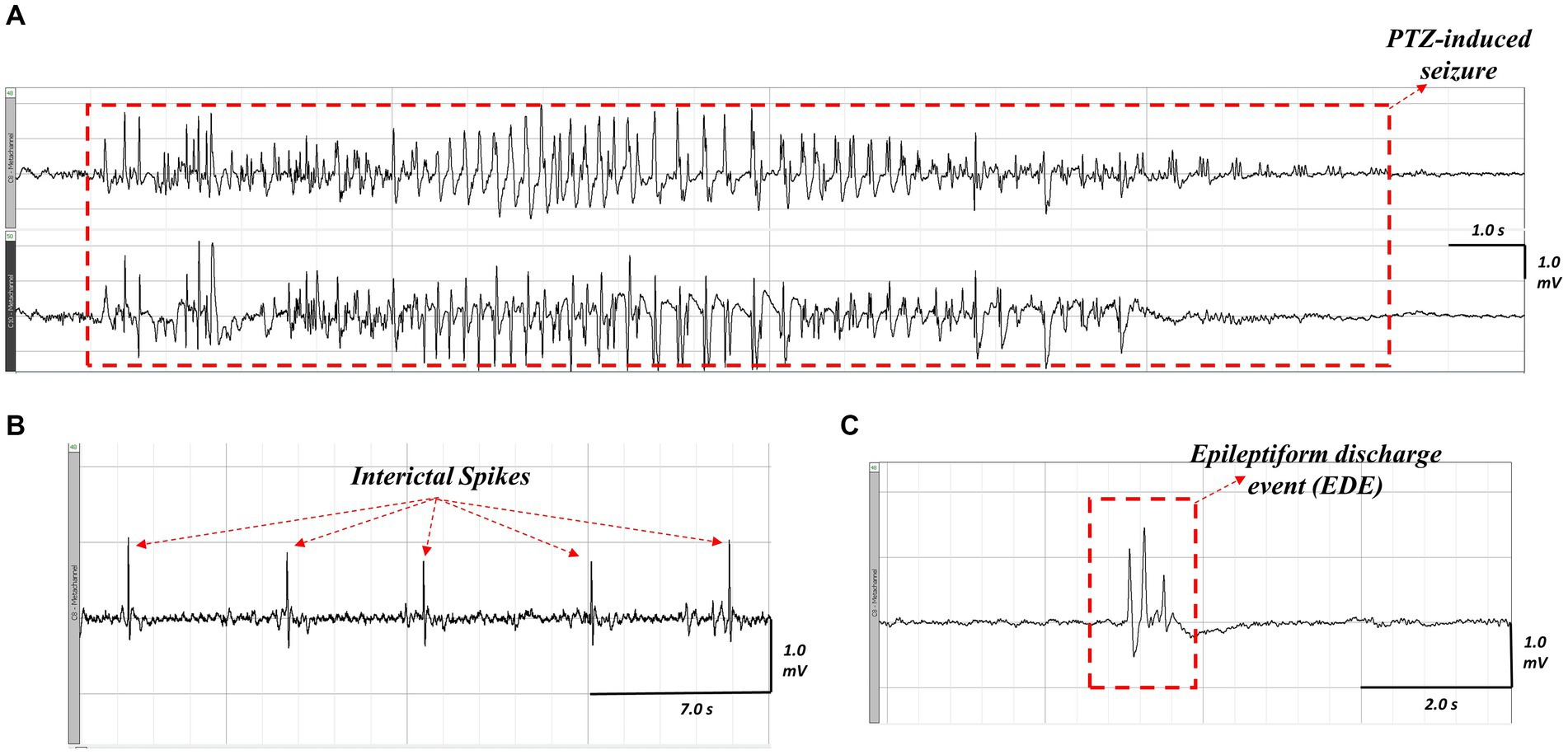
Figure 5. Representative ictal and interictal epileptiform activity observed on EEG following PTZ-challenge in an LPS-challenged Nf1+/− mouse. A seizure occurring after PTZ challenge (A), the top tracing is the neocortical electrode and the bottom tracing is the hippocampal electrode. Interictal spikes (B) appear as sharp, contoured, and fast waveforms that are a minimum twice the baseline amplitude. An epileptiform discharge event (C) appears as a complex waveform (e.g., a polyspike wave discharge) that lasts less than five seconds.
There was a significant effect of early-life immune activation on the latency to the first spike, with a lower latency in mice receiving LPS (p = 0.001), but no effect of genotype (p = 0.2482) and no interaction (p = 0.6042). Both WT + LPS (p = 0.0271) and Nf1+/− + LPS (p = 0.0151) groups had lower latencies to first spike than their respective saline controls (Figure 6A). No significant differences were found in the total spike count across all groups (p = 0.791) (Figure 6B), or in the latency to the first EDE or total EDE count (Appendix S2; Supplementary Figure S2).
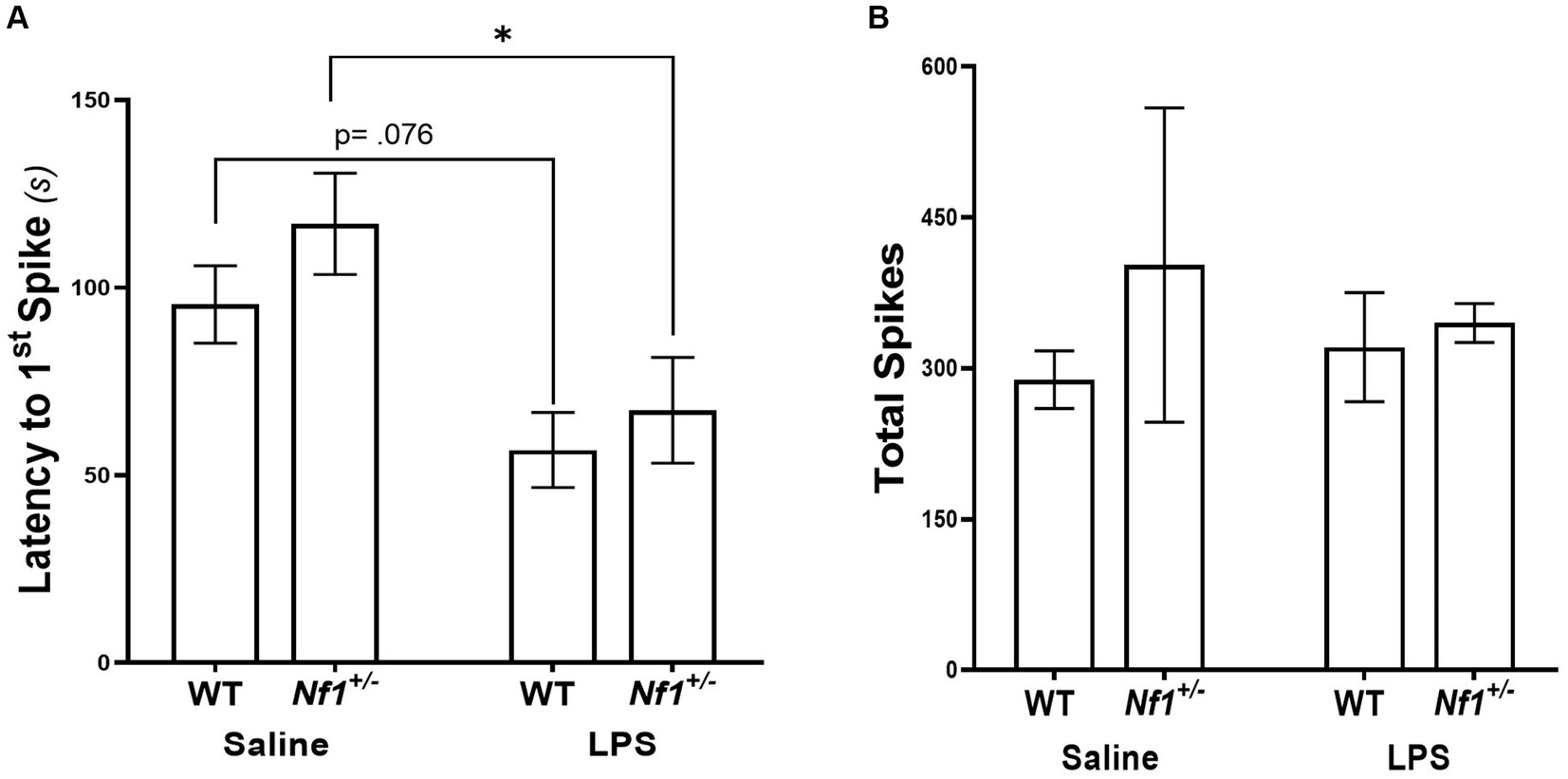
Figure 6. Early-life immune activation decreases the latency to the first spike, but does not affect the total spike count, following a PTZ challenge in both Nf1+/− and WT adult mice. WT + LPS and Nf1+/− + LPS groups had significantly shorter latencies to the first spike after PTZ challenge as compared to the WT + Saline and Nf1+/− + Saline groups (A). There were no differences in the total spike counts in the hour following PTZ challenge (B). Data are presented as the mean ± SEM, 2-WAY ANOVA, Sidak’s post-hoc,*p < 0.05.
The proportion of mice that developed seizures, the latency to the first PTZ-induced seizure and the cumulative seizure duration were also examined across groups. PTZ-induced seizures were detected in 2/8 WT + Saline mice, 2/6 Nf1+/− + Saline mice, 1/8 WT + LPS mice, and 2/6 Nf1+/− + LPS mice, with no statistical differences in the proportion of mice that developed PTZ-induced seizures (χ2 (3, N = 28) = 1.11; p = 0.774). There were no significant differences in the seizure latency or cumulative seizure duration (Appendix S2; Supplementary Figure S3).
4 Discussion
We set out to investigate whether early-life immune activation in NF1 further exacerbates the increased seizure susceptibility and learning and memory impairments associated with the disease. We found comparable basal neuroinflammation in P10 WT and Nf1+/− saline-treated mice as well as a comparable cytokine neuroimmune response to peripheral LPS challenge. We have shown for the first time that adult Nf1+/− mice have increased spontaneous epileptiform discharges compared to WT mice, as demonstrated in the saline control groups. We also demonstrate that early-life immune activation with LPS leads to a further increase in epileptiform abnormalities and promotes the development of spontaneous seizures in Nf1+/− but not WT mice. These results support a role for early-life inflammation in the development of seizures and epilepsy in NF1. However, a similar effect of early-life immune activation was not found in spatial learning and memory tasks, suggesting inflammation may not be a factor in NF1-associated cognitive deficits.
The present study is the first to assess levels of pro-inflammatory cytokines in the brain of Nf1+/− mice to determine the basal neuroimmune state of the CNS in NF1. There is evidence of increased concentrations of circulating inflammatory cytokines such as TGFβ (33), interferon-γ, TNF-α, IL-6 (33, 36) and IL-1β (35) in blood samples from patients with NF1. As such, we hypothesized that basal cytokine levels may be elevated in saline-treated Nf1+/− vs. WT mice. We found, however, comparable baseline cytokine levels (TNF-α, IL-6, IL-1β), as well as a comparable cytokine neuroimmune response to LPS challenge. We cannot exclude, however, differences in other inflammatory cytokines and chemokines between Nf1+/− and WT mice. We also cannot exclude any time-dependent differences in the brain cytokine response to the systemic LPS challenge, as has been previously described (40, 41). While we have shown an LPS-induced increase in inflammatory cytokines in whole brain samples from both WT and Nf1+/− mice, early-life exposure to LPS is known to cause inflammatory cytokine release, microglial activation, and neuronal dysfunction in a variety of brain regions, including the hippocampus, thalamus, neocortex, substantia nigra, and cerebellum (42–47). The exact brain region(s) responsible for increased seizure susceptibility and ictogenesis due to the genetic mutation in NF1 are unknown, but it is likely that one or more of these affected regions is involved.
This study is also the first to report spontaneous epileptiform abnormalities in Nf1+/− mice. We found a significantly higher number of interictal spikes in saline-treated Nf1+/− vs. WT mice, demonstrating for the first time that spontaneous interictal epileptiform activity occurs in adult Nf1+/− mice. These results build on previous work from our laboratory in which we have shown an increased susceptibility to various chemical convulsants (13) and an increased rate of epileptogenesis (14) in adult Nf1+/− mice. Although we did not detect any spontaneous seizures in Nf1+/− mice that were not challenged with LPS, we cannot rule out the possibility that spontaneous seizure activity may occur at a low frequency which would require analysis beyond the five days of EEG recording analyzed in the present study. Both the frequency of interictal spikes and the occurrence of spontaneous seizures were increased in adult Nf1+/− + LPS, but not in WT + LPS mice. Although we have only reported on male mice, we do not have reason to believe there would be sex difference in terms of the effect of LPS on seizures and epilepsy in NF1. For one, our previous studies on seizure susceptibility and epileptogenesis in NF1 were performed on both males and females (13, 14) with no sex differences reported. Similarly, sex differences have not been found in NF1 patients with epilepsy (6). In addition, reports of increased seizures after early-life LPS in a mouse model of autism spectrum disorder also did not find sex differences (32). Overall, our results support the hypothesis that the genetic mutation alone may not be sufficient to cause epilepsy in NF1, but that a second-hit from early-life inflammation may be a factor in promoting epileptogenesis.
We have previously reported an increased seizure susceptibility to pilocarpine and kainic acid in Nf1+/− mice (13), and predicted they would also have an increased susceptibility to PTZ. We were therefore surprised to find no differences in PTZ-induced seizure parameters between WT and Nf1+/− saline controls. The absence of an increased susceptibility of Nf1+/− mice to PTZ, as opposed to the increased susceptibility to kainic acid and pilocarpine we have previously reported12, may be attributed to the differences in the mechanism of action of these chemical convulsants. PTZ is a non-competitive antagonist of the GABAA receptor and elicits hyperexcitability by blocking GABA-mediated inhibition (48), while kainic acid is an L-glutamate analog of the ionotropic kainate receptors (49) and pilocarpine is a muscarinic acetylcholine receptor agonist (50). NF1 is associated with increased GABA tone in the hippocampus (20), which might negate some of the effects of PTZ and may explain why we did not find an increased susceptibility to PTZ in the Nf1+/− mouse as we previously reported with other chemical convulsants (13).
Early-life systemic inflammation models have been shown to promote an increased seizure susceptibility to chemical convulsants in adulthood (28, 29, 51). In the present study, early-life immune activation decreased the latency to first PTZ-induced spike in both WT and Nf1+/− mice, but did not affect PTZ-related additional parameters as previously reported by other groups. This may be related to different techniques in assessing PTZ seizure susceptibility, such as the use of continuous tail vein PTZ infusion (32, 51) versus the single systemic injection used here. However, for reasons mentioned above, PTZ may not be the most appropriate chemoconvulsant to use in NF1 studies going forward.
The mechanisms that underly NF1-associated seizure susceptibility have not been previously studied (52). Previous reports describe changes in voltage-gated ion channels associated with NF1 that may mediate hyperexcitability (53, 54). Furthermore, in addition to its interaction with Ras, neurofibromin is known to interact with NMDARs. While alterations in NMDAR have not been studied in the context of hyperexcitability in NF1, NMDAR activation has been shown to promote Ras-inactivation by neurofibromin and facilitate postsynaptic spine density and synaptic plasticity in pyramidal CA1 neurons (54). Enhanced ERK signaling has also been reported in the Nf1+/− mouse model (20) and enhanced ERK signaling has been previously shown to stimulate expression of NMDA receptors (17). If NMDAR trafficking is increased in NF1, then it is possible that an excitation/inhibition imbalance occurs, favoring hyperexcitability. The increased GABA tone previously described in NF1 may also promote hyperexcitability if limited to inhibitory neuronal circuits. In addition to these mechanisms, our results support the possibility that early-life immune activation can promote epileptogenesis in NF1 by perturbing the developmental trajectory of the brain. Previous reports have demonstrated that while LPS-stimulated in vitro cultures of Nf1+/− microglia secrete comparable levels of pro- and anti-inflammatory cytokines as WT microglia, the phagocytic activity and purinergic signaling in adult Nf1+/− microglia is significantly reduced (55). As microglia undergo morphological and functional changes throughout their developmental maturation (56), and given the role these cells play in fine tuning synaptic connections during neurodevelopment, it is possible for early-life immune activation to perturb the trajectory and function of these cells and affect the neuronal network in the NF1 brain. Although some cases of epilepsy in patients with NF1 are due to focal brain lesions, studies have reported a “normal” MRI in ~25–50% of patients with NF1 and epilepsy (6, 10, 58, 59), and structural NF1-associated lesions on MRI do not always co-localize with epileptiform discharges on EEG (11). This suggests the genetic mutation itself increases seizure susceptibility. However, considering the lifelong prevalence of epilepsy in NF1 is ~11% (58) the mutation alone is not sufficient to cause epilepsy. The results from our study suggest early-life inflammation is one of the secondary factors contributing to the development of epilepsy in NF1.
Nf1+/− mice were first described to have a spatial memory impairment in the Morris water maze (MWM) by Silva and colleagues, who demonstrated that the impairment is mild and can be overcome with additional acquisition training (18). Our data, the first describing the spatial learning and memory phenotype of Nf1+/− mice in the Barnes maze, did not demonstrate a spatial memory impairment in adult Nf1+/− mice. While we applied a common Bares Maze training regimen to what has previously been used in C57BL/6 mice (38), it is possible that the length of the acquisition phase promoted an overtraining of Nf1+/− mice. Additionally, Silva and colleagues have reported that the impairment in spatial reference memory in Nf1+/− mice is not fully penetrant and up to 30% of mice in their study had normal performance in the MWM. Taken together, while our data does not demonstrate a spatial memory impairment in the Nf1+/− mouse, our results serve to guide future studies for phenotyping the Nf1+/− mouse model in the Barnes maze. Early-life LPS challenge at P10 did increase the total distance travelled and the total number of errors in reaching the target hole in the probe trial in WT + LPS mice compared to WT + Saline mice, in contrast to the lack of effect of an early-life LPS challenge at P14 (31). It is unclear why there was an effect of early-life inflammation in WT and not Nf1+/− mice. It is possible that the kinetics of LPS differentially affect WT versus Nf1+/− mice, considering that the learning and memory impairments previously described in Nf1+/− mice are thought to be driven by GABAergic and dopaminergic circuit alterations (20, 21) (Costa et al., 2022). Lastly, we cannot exclude a delayed effect of LPS on the learning and memory phenotype in Nf1+/− mice which may have developed at a later timepoint beyond the window of postnatal day 60–74.
This is the first report describing the neuroinflammatory state and the long-term consequences of early-life immune activation in NF1. Our results have demonstrated that whereas a systemic early-life LPS challenge induces a comparable acute cytokine neuroimmune response in Nf1+/− and WT mice, it promotes increased seizure activity only in adult Nf1+/− mice. While Nf1+/− heterozygosity increases epileptiform abnormalities, our findings show that second-hit environmental events (such as early-life immune activation) may be required to promote epileptogenesis. This may explain in part why some patients with NF1 develop epilepsy over their lifetime while others do not.
Data availability statement
The raw data supporting the conclusions of this article will be made available by the authors, without undue reservation.
Ethics statement
The animal study was approved by University Health Network Animal Resources Committee. The study was conducted in accordance with the local legislation and institutional requirements.
Author contributions
RF: Data curation, Formal analysis, Investigation, Writing – original draft, Writing – review & editing. AR: Conceptualization, Formal analysis, Funding acquisition, Investigation, Resources, Supervision, Writing – original draft, Writing – review & editing.
Funding
The author(s) declare financial support was received for the research, authorship, and/or publication of this article. This work was supported by project funding to AR from the Toronto General & Western Hospital Foundation, and by scholarship awards to RF from the University of Toronto (Queen Elizabeth II Graduate Scholarship in Science and Technology, Margaret & Howard Gamble Neuroscience Research Grant, Unilever/Lipton Graduate Fellowship in Neuroscience, Institute of Medical Science Open Fellowship).
Acknowledgments
We would like to thank Dr. Chiping Wu for his assistance with electrode implantation, and Dr. Mingdong Yong for assistance with maintaining the breeding colonies.
Conflict of interest
The authors declare that the research was conducted in the absence of any commercial or financial relationships that could be construed as a potential conflict of interest.
Publisher’s note
All claims expressed in this article are solely those of the authors and do not necessarily represent those of their affiliated organizations, or those of the publisher, the editors and the reviewers. Any product that may be evaluated in this article, or claim that may be made by its manufacturer, is not guaranteed or endorsed by the publisher.
Supplementary material
The Supplementary material for this article can be found online at: https://www.frontiersin.org/articles/10.3389/fneur.2024.1284574/full#supplementary-material
References
1. Viskochil, D, Buchberg, AM, Xu, G, Cawthon, RM, Stevens, J, Wolff, RK, et al. Deletions and a translocation interrupt a cloned gene at the neurofibromatosis type 1 locus. Cell. (1990) 62:187–92. doi: 10.1016/0092-8674(90)90252-A
2. Wallace, MR, Marchuk, DA, Andersen, LB, Letcher, R, Odeh, HM, Saulino, AM, et al. Type 1 neurofibromatosis gene: identification of a large transcript disrupted in three NF1 patients. Science. (1990) 249:181–6. doi: 10.1126/science.2134734
3. Weiss, B, Bollag, G, and Shannon, K. Hyperactive Ras as a therapeutic target in neurofibromatosis type 1. Am J Med Genet. (1999) 89:14–22. doi: 10.1002/(SICI)1096-8628(19990326)89:1<14::AID-AJMG5>3.0.CO;2-4
4. Shofty, B, Constantini, S, and Ben-Shachar, S. Advances in molecular diagnosis of neurofibromatosis type 1. Semin Pediatr Neurol. (2015) 22:234–9. doi: 10.1016/j.spen.2015.10.007
5. Bernardo, P, Cinalli, G, and Santoro, C. Epilepsy in NF1: a systematic review of the literature. Childs Nerv Syst. (2020) 36:2333–50. doi: 10.1007/s00381-020-04710-7
6. Sorrentino, U, Bellonzi, S, Mozzato, C, Brasson, V, Toldo, I, Parrozzani, R, et al. Epilepsy in NF1: epidemiologic, genetic, and clinical features. A monocentric retrospective study in a cohort of 784 patients. Cancers (Basel). (2021) 13:6336. doi: 10.3390/cancers13246336
7. Hébert, J, De Santis, RJ, Daniyal, L, Mannan, S, Ng, E, Thain, E, et al. Epilepsy in neurofibromatosis type 1: Prevalence, phenotype, and genotype in adults. Epilepsy Res. (2024) 202:107336. doi: 10.1016/j.eplepsyres.2024.107336. [Epub ahead of print].
8. Hauser, WA . Epidemiology of epilepsy in children. Neurosurg Clin N Am. (1995) 6:419–30. doi: 10.1016/S1042-3680(18)30437-6
9. Hsieh, H-Y, Fung, H-C, Wang, C-J, Chin, S-C, and Wu, T. Epileptic seizures in neurofibromatosis type 1 are related to intracranial tumors but not to neurofibromatosis bright objects. Seizure. (2011) 20:606–11. doi: 10.1016/j.seizure.2011.04.016
10. Pecoraro, A, Arehart, E, Gallentine, W, Radtke, R, Smith, E, Pizoli, C, et al. Epilepsy in neurofibromatosis type 1. Epilepsy Behav. (2017) 73:137–41. doi: 10.1016/j.yebeh.2017.05.011
11. Serdaroglu, E, Konuskan, B, Karli Oguz, K, Gurler, G, Yalnizoglu, D, and Anlar, B. Epilepsy in neurofibromatosis type 1: diffuse cerebral dysfunction? Epilepsy Behav. (2019) 98:6–9. doi: 10.1016/j.yebeh.2019.06.022
12. Kulkantrakorn, K, and Geller, TJ. Seizures in neurofibromatosis 1. Pediatr Neurol. (1998) 19:347–50. doi: 10.1016/S0887-8994(98)00075-7
13. Rizwan, G, sabetghadam, A, Wu, C, Liu, J, Zhang, L, and Reid, AY. Increased seizure susceptibility in a mouse model of neurofibromatosis type 1. Epilepsy Res. (2019) 156:106190. doi: 10.1016/j.eplepsyres.2019.106190
14. Sabetghadam, A, Wu, C, Liu, J, Zhang, L, and Reid, AY. Increased epileptogenicity in a mouse model of neurofibromatosis type 1. Exp Neurol. (2020) 331:113373. doi: 10.1016/j.expneurol.2020.113373
15. Rosser, TL, and Packer, RJ. Neurocognitive dysfunction in children with neurofibromatosis type 1. Curr Neurol Neurosci Rep. (2003) 3:129–36. doi: 10.1007/s11910-003-0064-3
16. Hyman, SL, Shores, A, and North, KN. The nature and frequency of cognitive deficits in children with neurofibromatosis type 1. Neurology. (2005) 65:1037–44. doi: 10.1212/01.wnl.0000179303.72345.ce
17. Li, W, Cui, Y, Kushner, SA, Brown, RAM, Jentsch, JD, Frankland, PW, et al. The HMG-CoA reductase inhibitor lovastatin reverses the learning and attention deficits in a mouse model of neurofibromatosis type 1. Curr Biol. (2005) 15:1961–7. doi: 10.1016/j.cub.2005.09.043
18. Silva, AJ, Frankland, PW, Marowitz, Z, Friedman, E, Lazlo, G, Cioffi, D, et al. A mouse model for the learning and memory deficits associated with neurofibromatosis type I. Nat Genet. (1997) 15:281–4. doi: 10.1038/ng0397-281
19. Costa, RM, and Silva, AJ. Molecular and cellular mechanisms underlying the cognitive deficits associated with neurofibromatosis 1. J Child Neurol. (2002) 17:622–6. doi: 10.1177/088307380201700813
20. Cui, Y, Costa, RM, Murphy, GG, Elgersma, Y, Zhu, Y, Gutmann, DH, et al. Neurofibromin regulation of ERK signaling modulates GABA release and learning. Cell. (2008) 135:549–60. doi: 10.1016/j.cell.2008.09.060
21. Diggs-Andrews, KA, Tokuda, K, Izumi, Y, Zorumski, CF, Wozniak, DF, and Gutmann, DH. Dopamine deficiency underlies learning deficits in neurofibromatosis-1 mice. Ann Neurol. (2013) 73:309–15. doi: 10.1002/ana.23793
22. Maroso, M, Balosso, S, Ravizza, T, Liu, J, Aronica, E, Iyer, AM, et al. Toll-like receptor 4 and high-mobility group box-1 are involved in ictogenesis and can be targeted to reduce seizures. Nat Med. (2010) 16:413–9. doi: 10.1038/nm.2127
23. Ravizza, T, Terrone, G, Salamone, A, Frigerio, F, Balosso, S, Antoine, DJ, et al. High mobility group box 1 is a novel pathogenic factor and a mechanistic biomarker for epilepsy. Brain Behav Immun. (2018) 72:14–21. doi: 10.1016/j.bbi.2017.10.008
24. Sun, Y, Vestergaard, M, Christensen, J, Nahmias, AJ, and Olsen, J. Prenatal exposure to maternal infections and epilepsy in childhood: a population-based cohort study. Pediatrics. (2008) 121:e1100–7. doi: 10.1542/peds.2007-2316
25. Sun, Y, Vestergaard, M, Christensen, J, and Olsen, J. Prenatal exposure to elevated maternal body temperature and risk of epilepsy in childhood: a population-based pregnancy cohort study. Paediatr Perinat Epidemiol. (2011) 25:53–9. doi: 10.1111/j.1365-3016.2010.01143.x
26. Guma, E, Plitman, E, and Chakravarty, MM. The role of maternal immune activation in altering the neurodevelopmental trajectories of offspring: a translational review of neuroimaging studies with implications for autism spectrum disorder and schizophrenia. Neurosci Biobehav Rev. (2019) 104:141–57. doi: 10.1016/j.neubiorev.2019.06.020
27. Solek, CM, Farooqi, N, Verly, M, Lim, TK, and Ruthazer, ES. Maternal immune activation in neurodevelopmental disorders. Dev Dyn. (2018) 247:588–619. doi: 10.1002/dvdy.24612
28. Chen, Y-H, Kuo, TT, Chu, MT, Ma, HI, Chiang, YH, and Huang, EYK. Postnatal systemic inflammation exacerbates impairment of hippocampal synaptic plasticity in an animal seizure model. Neuroimmunomodulation. (2013) 20:223–32. doi: 10.1159/000348440
29. Galic, MA, Riazi, K, Heida, JG, Mouihate, A, Fournier, NM, Spencer, SJ, et al. Postnatal inflammation increases seizure susceptibility in adult rats. J Neurosci. (2008) 28:6904–13. doi: 10.1523/JNEUROSCI.1901-08.2008
30. Harré, EM, Galic, MA, Mouihate, A, Noorbakhsh, F, and Pittman, QJ. Neonatal inflammation produces selective behavioural deficits and alters N-methyl-D-aspartate receptor subunit mRNA in the adult rat brain. Eur J Neurosci. (2008) 27:644–53. doi: 10.1111/j.1460-9568.2008.06031.x
31. Dinel, A-L, Joffre, C, Trifilieff, P, Aubert, A, Foury, A, le Ruyet, P, et al. Inflammation early in life is a vulnerability factor for emotional behavior at adolescence and for lipopolysaccharide-induced spatial memory and neurogenesis alteration at adulthood. J Neuroinflammation. (2014) 11:155. doi: 10.1186/s12974-014-0155-x
32. Lewis, ML, Kesler, M, Candy, SA, Rho, JM, and Pittman, QJ. Comorbid epilepsy in autism spectrum disorder: implications of postnatal inflammation for brain excitability. Epilepsia. (2018) 59:1316–26. doi: 10.1111/epi.14440
33. Farschtschi, S, Park, SJ, Sawitzki, B, Oh, SJ, Kluwe, L, Mautner, VF, et al. Effector T cell subclasses associate with tumor burden in neurofibromatosis type 1 patients. Cancer Immunol Immunother. (2016) 65:1113–21. doi: 10.1007/s00262-016-1871-0
34. Lasater, EA, Li, F, Bessler, WK, Estes, ML, Vemula, S, Hingtgen, CM, et al. Genetic and cellular evidence of vascular inflammation in neurofibromin-deficient mice and humans. J Clin Invest. (2010) 120:859–70. doi: 10.1172/JCI41443
35. Park, S-J, Sawitzki, B, Kluwe, L, Mautner, VF, Holtkamp, N, and Kurtz, A. Serum biomarkers for neurofibromatosis type 1 and early detection of malignant peripheral nerve-sheath tumors. BMC Med. (2013) 11:109. doi: 10.1186/1741-7015-11-109
36. Torres, KCL, Lima, G, Simões e Silva, AC, Lubambo, I, Rodrigues, LO, Rodrigues, L, et al. Immune markers in the RASopathy neurofibromatosis type 1. J Neuroimmunol. (2016) 295-296:122–9. doi: 10.1016/j.jneuroim.2016.04.008
37. Walker, WH, Bumgarner, JR, Nelson, RJ, and Courtney, DVA. Transcardial perfusion is not required to accurately measure cytokines within the brain. J Neurosci Methods. (2020) 334:108601. doi: 10.1016/j.jneumeth.2020.108601
38. Sunyer, B, Patil, S, Höger, H, and Luber, G. Barnes maze, a useful task to assess spatial reference memory in the mice. Protoc exch. (2007). doi: 10.1038/nprot.2007.390
39. Van Erum, J, Van Dam, D, and De Deyn, PP. PTZ-induced seizures in mice require a revised Racine scale. Epilepsy Behav. (2019) 95:51–5. doi: 10.1016/j.yebeh.2019.02.029
40. Biesmans, S, Meert, TF, Bouwknecht, JA, Acton, PD, Davoodi, N, De Haes, P, et al. Systemic immune activation leads to neuroinflammation and sickness behavior in mice. Mediat Inflamm. (2013) 2013:271359. doi: 10.1155/2013/271359
41. Erickson, MA, and Banks, WA. Cytokine and chemokine responses in serum and brain after single and repeated injections of lipopolysaccharide: multiplex quantification with path analysis. Brain Behav Immun. (2011) 25:1637–48. doi: 10.1016/j.bbi.2011.06.006
42. Fan, L, Tien, L, Zheng, B, Pang, Y, Lin, R, Simpson, KL, et al. Dopaminergic neuronal injury in the adult rat brain following neonatal exposure to lipopolysaccharide and the silent neurotoxicity. Brain Behav Immun. (2011) 25:286–97. doi: 10.1016/j.bbi.2010.09.020
43. Heida, JG, Boissé, L, and Pittman, QJ. Lipopolysaccharide-induced febrile convulsions in the rat: short-term sequelae. Epilepsia. (2004) 45:1317–29. doi: 10.1111/j.0013-9580.2004.13704.x
44. Ulmer, AJ, Rietschel, ET, Zahringer, U, and Heine, H. Lipopolysaccharide: structure, and signal bioactivity. Transduct Trends Glycosci Glycotechnol. (2002) 14:53–68. doi: 10.4052/tigg.14.53
45. Pires, JM, Foresti, ML, Silva, CS, Rêgo, DB, Calió, ML, Mosini, AC, et al. Lipopolysaccharide-induced systemic inflammation in the neonatal period increases microglial density and oxidative stress in the cerebellum of adult rats. Front Cell Neurosci. (2020) 14:142. doi: 10.3389/fncel.2020.00142
46. Wang, K, Fan, L, Kaizaki, A, Pang, Y, Cai, Z, and Tien, L. Neonatal lipopolysaccharide exposure induces long-lasting learning impairment, less anxiety-like response and hippocampal injury in adult rats. Neuroscience. (2013) 234:146–57. doi: 10.1016/j.neuroscience.2012.12.049
47. Zhu, F, Zhang, L, Ding, IQ, Zhao, J, and Zheng, Y. Neonatal intrahippocampal injection of lipopolysaccharide induces deficits in social behavior and prepulse inhibition and microglial activation in rats: implication for a new schizophrenia animal model. Brain Behav Immun. (2014) 38:166–74. doi: 10.1016/j.bbi.2014.01.017
48. Ramanjaneyulu, R, and Ticku, MK. Interactions of pentamethylenetetrazole and tetrazole analogues with the picrotoxinin site of the benzodiazepine-GABA receptor-ionophore complex. Eur J Pharmacol. (1984) 98:337–45. doi: 10.1016/0014-2999(84)90282-6
49. Nadler, JV, Perry, BW, and Cotman, CW. Intraventricular kainic acid preferentially destroys hippocampal pyramidal cells. Nature. (1978) 271:676–7. doi: 10.1038/271676a0
50. Turski, WA, Cavalheiro, EA, Schwarz, M, Czuczwar, SJ, Kleinrok, Z, and Turski, L. Limbic seizures produced by pilocarpine in rats: behavioural, electroencephalographic and neuropathological study. Behav Brain Res. (1983) 9:315–35. doi: 10.1016/0166-4328(83)90136-5
51. Gomez, CD, Acharjee, S, Lewis, ML, Read, J, and Pittman, QJ. Increased excitatory synaptic transmission associated with adult seizure vulnerability induced by early-life inflammation in mice. J Neurosci. (2021) 41:4367–77. doi: 10.1523/JNEUROSCI.2667-20.2021
52. Stafstrom, CE, Staedtke, V, and Comi, AM. Epilepsy mechanisms in Neurocutaneous disorders: tuberous sclerosis complex, Neurofibromatosis type 1, and Sturge-weber syndrome. Front Neurol. (2017) 8:87. doi: 10.3389/fneur.2017.00087
53. Moutal, A, Dustrude, ET, and Khanna, R. Sensitization of ion channels contributes to central and peripheral dysfunction in neurofibromatosis type 1. Mol Neurobiol. (2017) 54:3342–9. doi: 10.1007/s12035-016-9907-1
54. Wang, Y, Brittain, JM, Wilson, SM, Hingtgen, CM, and Khanna, R. Altered calcium currents and axonal growth in NF1 haploinsufficient mice. Transl Neurosci. (2010) 1:106–14. doi: 10.2478/v10134-010-0025-8
55. Husi, H, Ward, MA, Choudhary, JS, Blackstock, WP, and Grant, SG. Proteomic analysis of NMDA receptor-adhesion protein signaling complexes. Nat Neurosci. (2000) 3:661–9. doi: 10.1038/76615
56. Elmadany, N, Logiacco, F, Buonfiglioli, A, Haage, VC, Wright-Jin, EC, Schattenberg, A, et al. Neurofibromatosis 1—mutant microglia exhibit sexually-dimorphic cyclic AMP-dependent purinergic defects. Neurobiol Dis. (2020) 144:105030. doi: 10.1016/j.nbd.2020.105030
57. Schwarz, JM, Sholar, PW, and Bilbo, SD. Sex differences in microglial colonization of the developing rat brain. J Neurochem. (2012) 120:948–63. doi: 10.1111/j.1471-4159.2011.07630.x
58. Hébert, J, De Santis, R, Kim, R, Bril, V, and Reid, AY. Epilepsy in neurofibromatosis: prevalence in adults, phenotype, and genotype. Epilepsy Res. (2024) 202:107336. doi: 10.1016/j.eplepsyres.2024.107336
Keywords: neurofibromatosis type 1, neuroinflammation, epileptogenesis, early-life, two-hit model, lipopolysaccharide
Citation: Faidi R and Reid AY (2024) Early-life immune activation is a vulnerability factor for adult epileptogenesis in neurofibromatosis type 1 in male mice. Front. Neurol. 15:1284574. doi: 10.3389/fneur.2024.1284574
Edited by:
Yi Li, Stanford University, United StatesReviewed by:
Meihua Yang, Army Medical University, ChinaOlagide Wagner Castro, Federal University of Alagoas, Brazil
Erfan Bahramnejad, University of Arizona, United States
Copyright © 2024 Faidi and Reid. This is an open-access article distributed under the terms of the Creative Commons Attribution License (CC BY). The use, distribution or reproduction in other forums is permitted, provided the original author(s) and the copyright owner(s) are credited and that the original publication in this journal is cited, in accordance with accepted academic practice. No use, distribution or reproduction is permitted which does not comply with these terms.
*Correspondence: Aylin Y. Reid, YXlsaW4ucmVpZEB1dG9yb250by5jYQ==