- 1Department of Otolaryngology – Head and Neck Surgery, Rutgers Robert Wood Johnson Medical School, New Brunswick, NJ, United States
- 2Rutgers Brain Health Institute, New Brunswick, NJ, United States
Background: Vestibular loss and dysfunction has been associated with cognitive deficits, decreased spatial navigation, spatial memory, visuospatial ability, attention, executive function, and processing speed among others. Superior semicircular canal dehiscence (SSCD) is a vestibular-cochlear disorder in humans in which a pathological third mobile window of the otic capsule creates changes to the flow of sound pressure energy through the perilymph/endolymph. The primary symptoms include sound-induced dizziness/vertigo, inner ear conductive hearing loss, autophony, headaches, and visual problems; however, individuals also experience measurable deficits in basic decision-making, short-term memory, concentration, spatial cognition, and depression. These suggest central mechanisms of impairment are associated with vestibular disorders; therefore, we directly tested this hypothesis using both an auditory and visual decision-making task of varying difficulty levels in our model of SSCD.
Methods: Adult Mongolian gerbils (n = 33) were trained on one of four versions of a Go-NoGo stimulus presentation rate discrimination task that included standard (“easy”) or more difficult (“hard”) auditory and visual stimuli. After 10 days of training, preoperative ABR and c+VEMP testing was followed by a surgical fenestration of the left superior semicircular canal. Animals with persistent circling or head tilt were excluded to minimize effects from acute vestibular injury. Testing recommenced at postoperative day 5 and continued through postoperative day 15 at which point final ABR and c+VEMP testing was carried out.
Results: Behavioral data (d-primes) were compared between preoperative performance (training day 8–10) and postoperative days 6–8 and 13–15. Behavioral performance was measured during the peak of SSCD induced ABR and c + VEMP impairment and the return towards baseline as the dehiscence began to resurface by osteoneogenesis. There were significant differences in behavioral performance (d-prime) and its behavioral components (Hits, Misses, False Alarms, and Correct Rejections). These changes were highly correlated with persistent deficits in c + VEMPs at the end of training (postoperative day 15). The controls demonstrated additional learning post procedure that was absent in the SSCD group.
Conclusion: These results suggest that aberrant asymmetric vestibular output results in decision-making impairments in these discrimination tasks and could be associated with the other cognitive impairments resulting from vestibular dysfunction.
Introduction
In adults, vestibular loss and dysfunction has been associated with cognitive deficits. Specifically, vestibular loss in adults is associated with decreased spatial navigation (1), spatial memory (2), visuospatial ability (3–6), attention (7, 8), executive function (5, 9), and processing speed (5), among others. In addition, individuals with chronic vestibular disorders have been reported to have accompanying cognitive dysfunction (9–13). These cognitive impairments can be observed in patients within a group of vestibular-cochlear disorders referred to as “Third Window Syndrome.” In this disorder a pathological third mobile window of the otic capsule creates changes to the flow of sound pressure energy between the oval and round window (see review 14). The nature and location of this third mobile window can occur at many different sites (or multiple sites); however, the most common third mobile window is superior semicircular canal dehiscence (SSCD), (9, 13, 15–18). The primary physiological symptoms include sound-induced dizziness/vertigo, inner ear/otic capsule conductive hearing loss, autophony (hearing internal sounds abnormally well [one-third can hear their eyes move or blink]), headaches, and visual problems (convergence disorders, skew deviation, nystagmus, oscillopsia). However, individuals also experience measurable deficits in basic decision-making, short-term memory, concentration, spatial cognition, and depression (9, 13). This can lead to significant decreases in quality of life, lower academic performance in children, and decreased job performance in adults. Even with surgical treatment (dehiscence plugging/resurfacing) these chronic symptoms can be persistent, and recovery can be prolonged. This suggests that central networks undergo maladaptive neural plasticity; however, there has been limited investigation into the vast vestibular projections that integrate with virtually every major cognitive behavioral and sensory system of the brain.
It has been suggested that the vestibular system can be considered a potential window for exploring brain function beyond that of maintenance of balance, and into areas of cognitive, affective and psychiatric symptomology (19). It is known that normal vestibular activity is important for accurate sound localization (20) and higher-order sensorimotor integration at the level of the cortex (21). Indeed, emerging research is also demonstrating that disruption of vestibular input can cause deficits to visuospatial processing, memory, navigation, attention, and executive function (7). To this end, neuropsychology studies before and after surgical management of third window syndrome, including SSCD, have shown that cognitive dysfunction can occur and improve over time (9). We have developed a gerbil model that is accompanied by peripheral measures of impairment (elevated ABR thresholds, increased c+VEMPs amplitudes) that manifest and then resolve as the surgically created SSCD closes by spontaneous osteoneogenesis; resulting in resurfacing of the canal (22). The current study’s scientific premise is that by utilizing this experimental model of SSCD we can design experiments that directly investigate the SSCD-induced changes to central plasticity along vestibular and auditory circuits that are associated with cognitive impairments, which then resolve after natural bone regrowth. This approach will allow us to explore the interactions between the vestibular nucleus and the vast integration of their long-range projections to the auditory system through neural recordings taken during behavioral paradigms that test higher order cognition such as decision-making. We found that animals were impaired on both an auditory and visual decision-making task shortly after SSCD, which corresponded with the peak of peripheral impairment that we previously reported (22). As these physiological symptoms return towards baseline, we find a reduction in the impairment; however, inter-animal variability in recovery rates shows that the vestibular component (c+VEMP amplitude) is directly correlated with behavioral impairment and recovery to preoperative levels. Together these results show that our model allows a unique timeline to investigate central cognitive components of vestibular induced impairment and recovery.
Materials and methods
Animals
A total of (33) adult male and female Mongolian gerbils Meriones unguiculatus met the inclusion criteria and were used in this study. All animals were housed in the same vivarium facility under a 12/12 dark cycle with ad libitum access to food and water. Surgical creation of a 1.5 mm fenestration of the superior (anterior) semicircular canal produced the SSCD. The details of this procedure have been published previously (22). Exclusion criteria included removing any animals with persistent circling or head tilt present at post SSCD day 3 from the study protocol. The Rutgers University IACUC reviewed and approved this research protocol (PROTO202000179).
Auditory brainstem response testing
Animals were anesthetized with isoflurane (1.0%) and placed in a small sound chamber (IAC, Sound Room Solutions, Inc., Glen Cove, NY). Auditory brainstem response (ABR) recordings were made by inserting pin electrodes subcutaneously at the vertex of the skull and just caudal to the right pinna; the ground electrode was inserted into the base of the tail. BioSigRZ software and the TDT ABR system (Tucker-Davis Technologies, Alachua, FL, United States) were used to collect ABR data. A 10-cm tube (closed field) was inserted into the ear and placed at the opening of the ear canal. The left ear of the animal was stimulated via multi-field speaker (MF1, Tucker-Davis Technologies) at 1, 2, 4, 8, and 16 kHz tones (90 to 20 dB SPL [10 dB steps]), 5 ms, 2 ms linear ramp rise-fall times at 25 Hz. Traces were averaged across 500 (threshold) sweeps. Thresholds for each frequency were measured as the last dB SPL, i.e., 10 dB SPL resolution stimulus level, that elicited a tone-induced ABR.
Sound-induced cervical positive potential vestibular evoked myogenic potentials
Sound-induced otolithic stimulation and evoked intramuscular excitatory potential recordings were made by inserting pin electrodes into the neck extensor muscles (splenius capitus m.) and the reference electrode in the vertex of the skull measured (positive cervical vestibular evoked potential [c+VEMP]). BioSigRZ software and the TDT ABR system were used to collect c+VEMP data. A 10-cm tube capable of delivering 100 dB SPL (see TDT specs, Closed Field) was inserted into the ear and placed at the opening of the ear canal. The left ear of the animal was stimulated via multi-field speaker (MF1, Tucker-Davis Technologies) at 2 kHz (100 to 80 dB SPL [5 dB steps], 5 ms, 2 ms linear ramp rise-fall times sampled at 25 kHz). Traces were averaged across 500 (threshold) sweeps. The c+VEMPs were recorded under low-isoflurane anesthesia (<1.5%), near conditions of wakefulness. The c+VEMP was measured when it appeared under the condition of stimulation of air-conducted sound at 2 kHz and 100 dB. Peak amplitudes were measured by subtracting the peak of the negative N1 wave (in μV) from the later positive P1 wave.
Auditory discrimination task
We assessed auditory perceptual skill in gerbils with a positive reinforcement “Go-NoGo” appetitive conditioning paradigm. Briefly, gerbils were placed on controlled access to food and trained to discriminate between amplitude-modulated (AM) broadband noise presented at 4 versus 12 Hz. Gerbils were placed in a behavioral arena test cage housed in a sound attenuation chamber (Med Associates, Inc., Fairfax, VT) and observed via a closed-circuit monitor. Auditory stimuli were presented from a calibrated multifield speaker (MF1, Tucker-Davis Technologies, Alachua, FL, United States) positioned 15 inches above the test cage floor. Sound calibration measurements were made with a 1/4-inch free-field condenser recording microphone (Brüel & Kjær, Nærum, Denmark). A modular pellet dispenser (Med Associates, Inc., [20 mg]) was connected to a trough type pellet receptacle (Med Associates, Inc.) placed within the test cage, and a cylindrical nose port with a 1-inch diameter hole (Med Associates, Inc.) was placed on the opposite side. Sensitive infrared sensors bisected the nose port and pellet receptacles to detect gerbil nose and head entry, respectively. Stimuli, food reward delivery, and behavioral data acquisition were controlled by an iPac computer system running iCon behavioral interfaces (Tucker-Davis Technologies). Gerbils self-initiated trials by placing their nose in the noseport. On each trial, one of two stimulus types were presented. The “Go” stimulus consisted of AM broadband noise (25 dB roll-off at 3.5 and 20 kHz) with a modulation rate of 4 Hz and a modulation depth of 100%. The “NoGo” stimulus for the less difficult (“easy”) auditory discrimination task consisted of AM broadband noise presented at a modulation rate of 12 Hz at 100% modulation depth. The NoGo stimulus for the more difficult (“hard”) auditory discrimination task consisted of AM broadband noise presented at a modulation rate of 6 Hz at 100% modulation depth. All stimuli were presented at a sound level of 70 dB SPL and was recalibrated daily with a sound level meter. Gerbils were shaped to approach the food troughs upon presentation of the Go stimulus, and received a 20-mg pellet reward. Once gerbils reached a criterion of three consecutive days of 100 trials with >90% Hit (correctly approaching the food trough during a Go trial), they were then trained to repoke upon presentation of the NoGo stimulus. During this phase, NoGo trials (20% probability) were randomly interleaved with Go trials. Gerbils performed the task for at least 120 trials per day, or until over 20 NoGo trials occurred. Typically, a session lasted 45 min to 1 h. This training continued for 10 days.
Trials were scored as Hit, Miss (failing to approach the food trough and repoking during a Go trial), Correct Reject (CR; correctly repoking during a NoGo trial), or False Alarm (FA; incorrectly approaching the food trough on a NoGo trial). Hit and FA rates were constrained to floor (0.05) and ceiling (0.95) values. A performance metric, d-prime was calculated for each session by performing a z-transform of both hit rate and false alarm rate: d-prime = z (Hit rate) − z (FA rate). Criterion was set at d-primes of 1.5. It typically took normal hearing animals 3–4 days to achieve the criterion of d-prime 1.5 when performing the easy auditory discrimination task. It typically takes normal hearing animals 5–6 days to achieve the criterion of d-prime 1.5 when performing the hard auditory discrimination ask.
Visual discrimination task
Visual perceptual skill was assessed with positive reinforcement “Go-NoGo” appetitive conditioning paradigm, similar to that as described for the AM rate auditory discrimination task above. In contrast to the AM rate auditory discrimination task, visual stimuli were delivered from a light-emitting diode (LED; Med Associates, Inc.). The Go stimulus consisted of a flashing LED presented at 4 Hz. The NoGo stimulus for the less difficult (“easy”) visual discrimination task consisted of a flashing LED presented at 24 Hz. The NoGo stimulus for the more difficult (“hard”) visual discrimination task consisted of a flashing LED presented at 12 Hz. Go and NoGo training were identical to the procedures described for the AM rate auditory discrimination task.
Control (sham surgery) experiments
Control (sham surgery) SSCD animals (n = 4, easy auditory discrimination task; n = 4, easy visual discrimination task) were anesthetized with isoflurane (1.0%) and prepared for stereotaxic surgery. An incision was made over the nuchal muscles on the left side of the head just posterior to the ear. Once exposed the nuchal muscles were sharply and then bluntly dissected to expose the left superior bulla. A 5.0 mm opening was made with a 1.5 mm diamond bur. The intact superior (anterior) semicircular canal was directly visualized but was not fenestrated. The open bulla was then sealed/partitioned with Sterile Silastic (Dow Chemical Company, Midland, MI) to partition the air-filled bulla from the overlying neck muscles thereby restoring the normal air-filled middle ear and avoiding a true conductive hearing loss. Condensation on the interior surface of the Silastic seal was deemed indicative of this restoration of function. Finally, the reattached muscles were glued to the skull with Medbond tissue glue (Stoelting Co., Wood Dale, IL) which allowed c+VEMP testing after the control (sham surgery) procedure. The incision was closed with a running locked 4-0 Vicryl suture (Ethicon US, LLC, New Brunswick, NJ) and topical antibiotic was applied to the wound. Preoperative c+VEMPs and ABRs were performed and repeated at post day 15. Behavioral training for the easy auditory and visual tasks was completed as described above. The easy auditory discrimination task and visual discrimination task was carried out for the same timeline as the SSCD group (post day 6–15).
Results
In this study we collected behavioral, ABR, and c+VEMP data from 33 male and female Mongolian gerbils. An auditory discrimination task and visual discrimination task was used, and each had two difficulty levels (easy auditory [n = 7], hard auditory [n = 8], easy visual [n = 11], hard visual [n = 7]). Of those animals included in this study, 85% (n = 28) had no head tilt or circling after surgical creation of the SSCD and 15% (n = 8) had head tilt that resolved by post day 3 and before electrophysiologic and behavioral testing beginning on post day 7.
In humans, mild cognitive impairments have been associated with third window syndrome including SSCD prior to surgical treatment. These impairments have been shown to improve after surgery repair. In our recent gerbil SSCD model publication we showed that the site of dehiscence was spontaneously resurfaced via osteoneogenesis in the weeks following fenestration which is accompanied by a peak in c+VEMP amplitude and ABR threshold shift at post day 7 that progressively returned towards baseline between post days 14 and 21 (22). The duration required for resurfacing was a function of fenestration size. Therefore, we tracked the relationship between behavioral performance, and physiological measures associated with SSCD diagnostic findings (ABRs, c+VEMPs), and recovery as the SSCD spontaneously resurfaced.
SSCD induced cognitive impairments in an auditory and visual Go-NoGo task
Because there is a mild hearing impairment associated with SSCD we ran an auditory and visual version of the discrimination task to test for modality specific effects on behavioral performance. For each modality (auditory/visual) a hard and easy version of the task was used to identify if task difficulty was a factor in SSCD cognitive impairment. Figure 1 shows the learning curves for both tasks and the behavioral impairments associated with the peak of the ABR threshold shift and c+VEMP amplitude increase (post day 6, 7, 8) and the progressive shift towards baseline as the dehiscence undergoes resurfacing (post day 13, 14, 15). Figure 1A shows the learning curves over 10 days of behavioral testing for both versions and difficulty levels of the task. Criterion was set at a d-prime of 1.5, thus both the easy auditory discrimination task and easy visual discrimination task were learned at the same rate, with a similar delay in learning the more difficult task.
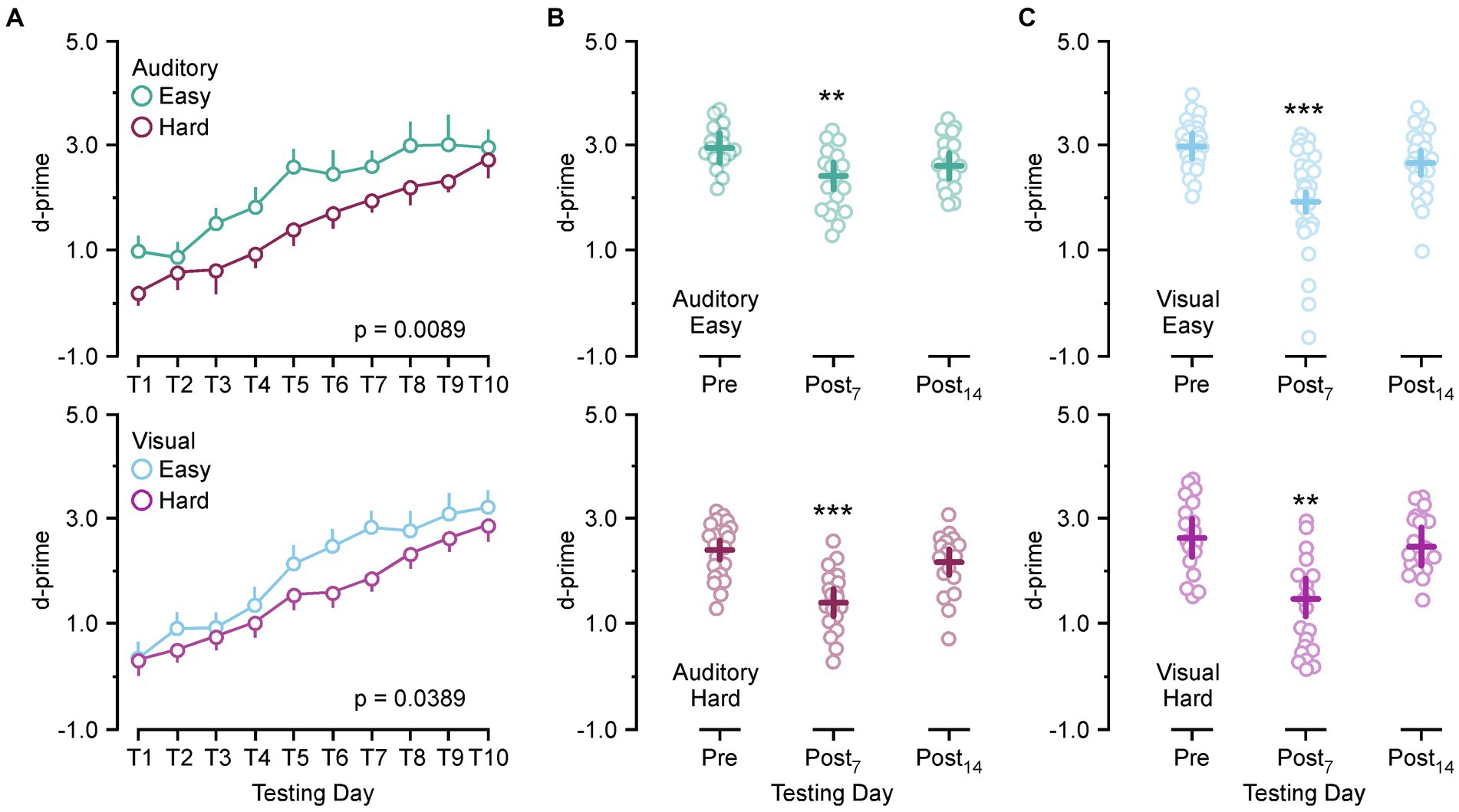
Figure 1. Behavioral impairments associated with postoperative days. (A) Line plot showing the group learning rates for the easy auditory discrimination task (green) and hard auditory discrimination task (purple) (top) and the easy visual discrimination task (blue) and hard visual discrimination task (purple) (bottom). (B) Scatter diagram showing comparisons between preoperative performance and postoperative day 7 (Postop7) and postoperative day 14 (Postop14) for the easy auditory discrimination task (green) (top) and hard auditory discrimination task (purple) (bottom). (C) Scatter diagram showing comparisons between preoperative performance and postoperative day 7 (Postop7) and postoperative day 14 (Postop14) for the easy visual discrimination task (blue) (top) and hard visual discrimination task (purple) (bottom). **p ≤ 0.001, ***p ≤ 0.0001.
There was no significant difference between the learning rate for the easy auditory discrimination task and easy visual discrimination task [F(1,16) = 0.57, p = 0.45] or the hard auditory discrimination task and the hard visual discrimination task [F(1,13) = 0.16, p = 0.68]. Within each task there was a significant difference in the learning rate between the easy and hard task (auditory, [F(1,13) = 9.43, p = 0.0089]; [F(1,16) = 5.06, p = 0.0389]). After 10 days of the testing the animals were given surgical fenestrations of the superior (anterior) semicircular canal and allowed to recover for 5 days prior to being returned to the behavioral paradigm. The animals then received 10 more days of testing to create statistical comparisons at the peak of the physiological impairment (post day 7) and during the recovery process (post day 14). Comparing preoperative d-prime performance (training day 8–10) to post day 7 (post days 6–8) and post day 14 performance (post days 13–15) showed a significant main effect of SSCD on behavior [F(2,294) = 42.28, p < 0.0001] with significant decreases at post day 7 (preoperative 2.77 ± 0.085 vs. post day 7, 1.83 ± 0.074, [p < 0.0001]) and post day 14 (preoperative 2.77 ± 0.085 vs. post day 14, 2.5 ± 0.048, [p = 0.0431]). When compared by task difficulty the significant decrease at post day 7 remained (easy: preoperative 2.99 ± 0.095 vs. post day 7, 2.15 ± 0.093, [p < 0.0001]; hard, preoperative 2.50 ± 0.105 vs. post day 7, 1.44 ± 0.109, [p < 0.0001]), while the main effect at post 14 was no longer significant (easy: preoperative 2.99 ± 0.095 vs. post day 14, 2.68 ± 0.094, [p = 0.059]; hard, preoperative 2.50 ± 0.105 vs. post day 14, 2.32 ± 0.104, [p = 0.453]). We next divided the data by task modality and difficulty for individual comparisons. Figures 1A–D shows the behavioral impairment associated with SSCD for the easy auditory discrimination task, hard auditory discrimination task, easy visual discrimination task, and hard visual discrimination task. There were significant differences between the preoperative behavior and postop day 7 for both tasks and difficulties (easy auditory, preoperative 2.96 ± 0.115 vs. post day 7, 2.45 ± 0.131, [p = 0.0049]; hard auditory, preoperative 2.40 ± 0.108 vs. post day 7, 1.41 ± 0.103, [p < 0.0001]; easy visual, preoperative 3.01 ± 0.132 vs. post day 7, 1.96 ± 0.123, [p < 0.0001]; hard visual, preoperative 2.60 ± 0.190 vs. post day 7, 1.47 ± 0.181, [p = 0.0003]). By post day 14 these impairments had largely resolved (easy auditory, preoperative 2.96 ± 0.115 vs. post day 14, 2.63 ± 0.111, [p = 0.098]; hard auditory, preoperative 2.40 ± 0.108 vs. post day 14, 2.17 ± 0.103, [p = 0.297]; easy visual, preoperative 3.01 ± 0.132 vs. post day 14, 2.72 ± 0.133, [p = 0.274]; hard visual, preoperative 2.60 ± 0.190 vs. post day 14, 2.45 ± 0.32, [p = 0.831]).
Behavioral metrics associated with cognitive impairments
As previously reported, there are individual variances associated with each animal concerning magnitude of the ABR threshold shifts and changes to c+VEMP amplitudes as the dehiscence is resurfaced via spontaneous osteoneogenesis. Therefore, we wanted to see if changes in ABR and c+VEMP properties were correlated with behavioral performance at post day 14. The behavioral measure d-prime is an expression of the ratio of the Hit rate to the FA rate. There are two main factors that can increase or decrease d-prime. Figure 2 shows the factors in this study that contributed to the impairments we report at post day 7 and the return towards terminal behavior scores at post day 14. Our results showed d-prime naturally increases for both the easy and hard task as the animals learn to respond correctly [F(1,35) = 14.72, p = 0.0006] (Figure 2A). A significantly smaller number of trials occurred in the harder task [F(1,35) = 14.85, p = 0.0005] (Figure 2B). Figure 2C shows that this leads to a smaller Hit rate in the harder task [F(1,35) = 10.05, p = 0.0032], but similarly decreasing FA rates [F(1,35) = 0.157, p = 0.693] (Figure 2D).
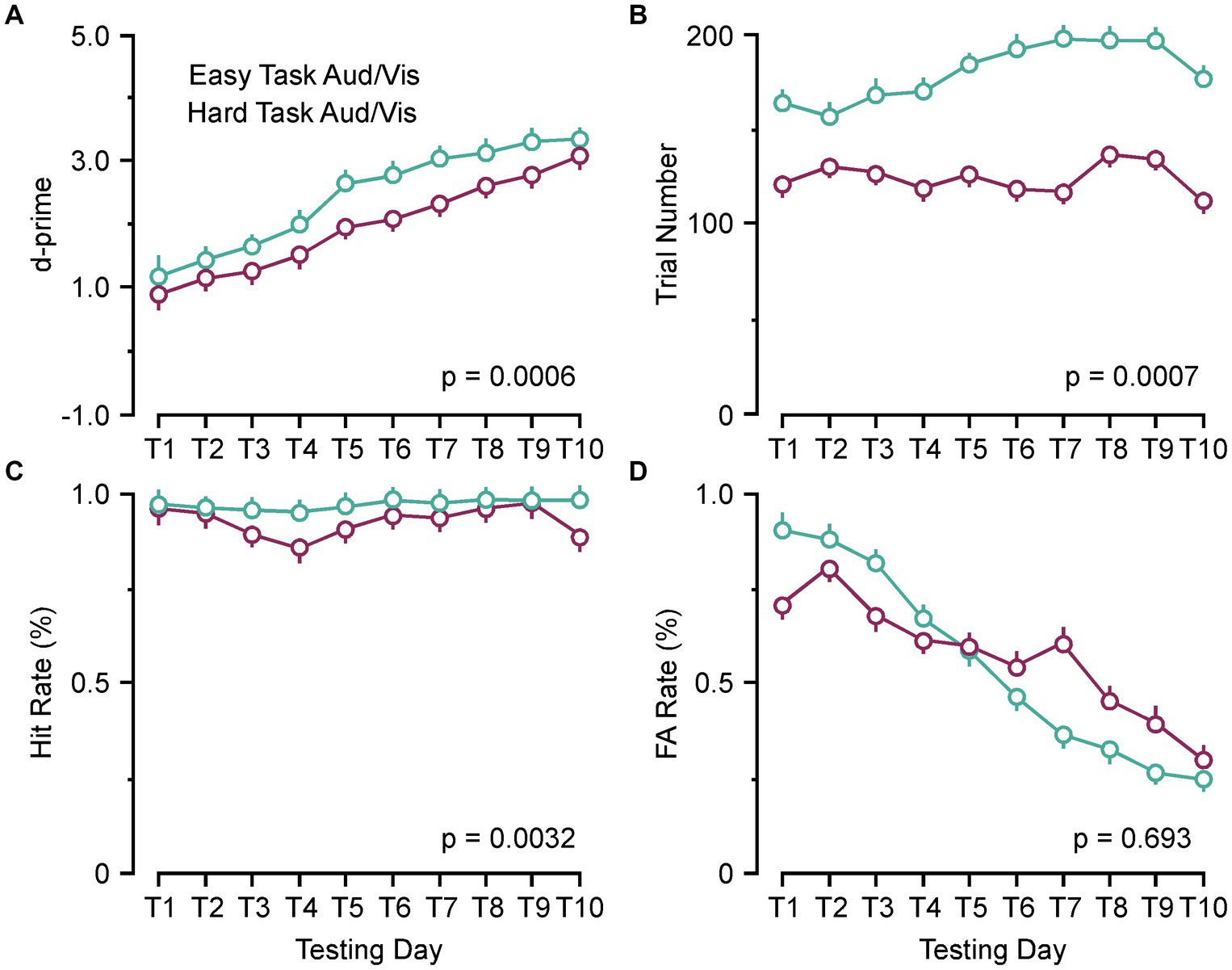
Figure 2. Behavioral parameters associated with performance (d-prime). (A) Line plots comparing the group learning rates for the pooled easy auditory and visual discrimination tasks (green) and hard auditory and visual discrimination (purple) tasks. (B) Line plot comparing the group trial numbers for the pooled easy auditory and visual discrimination tasks (green) and hard auditory and visual discrimination (purple) tasks. (C) Line plot comparing the group hit rates for the pooled easy auditory and visual discrimination tasks (green) and hard auditory and visual discrimination (purple) tasks. (D) Line plot comparing the group false alarm (FA) rates (%) for the pooled easy auditory and visual discrimination tasks (green) and hard auditory and visual discrimination (purple) tasks. Aud, auditory discrimination task; Vis, visual discrimination task.
Figure 3 compares the number of trials performed, Hit rate, and FA rate between preoperative behavior, post day 7, and post day 14 for the easy and hard versions of the auditory discrimination task and the visual discrimination task. In Figure 3A both the easy and the hard auditory discrimination task and the visual discrimination task show a significant reduction in overall trial number at post day 7 (easy, preoperative 202.3 ± 8.2 vs. post day 7, 133.2 ± 7.2, [p < 0.0001]; hard, preoperative 136.8 ± 7.53 vs. post day 7, 105.5 ± 5.77, [p = 0.0109]) that returned towards baseline at post day 14 (easy, preoperative 202.3 ± 8.2 vs. post day 14, 173.8 ± 8.30, [p = 0.054]; hard, preoperative 136.8 ± 7.53 vs. post day 14, 128.8 ± 8.35, [p = 0.735]). The same effect is present for the Hit rate in Figure 3B (easy, preoperative 0.97 ± 0.012 vs. post day 7, 0.91 ± 0.075, [p = 0.0015]; hard, preoperative 0.96 ± 0.013 vs. post day 7, 0.89 ± 0.035, [p = 0.0007]; easy, preoperative 0.97 ± 0.012 vs. post day 14, 0.94 ± 0.011, [p = 0.126]; hard, preoperative 0.96 ± 0.013 vs. post day 14, 0.93 ± 0.031, [p = 0.258]), and FA rate in Figure 3C (easy, preoperative 0.27 ± 0.019 vs. post day 7, 0.37 ± 0.061, [p = 0.0303]; hard, preoperative 0.36 ± 0.031 vs. post day 7, 0.49 ± 0.091, [p = 0.0141]; easy, preoperative 0.27 ± 0.012 vs. post day 14, 0.30 ± 0.018, [p = 0.720]; hard, preoperative 0.36 ± 0.031 vs. post day 14, 0.33 ± 0.027, [p = 0.847]). Overall, the SSCD lowered the number of trials, decreased the Hit rate, and increased the FA rate, suggesting that there was not a single factor driving the impairment.
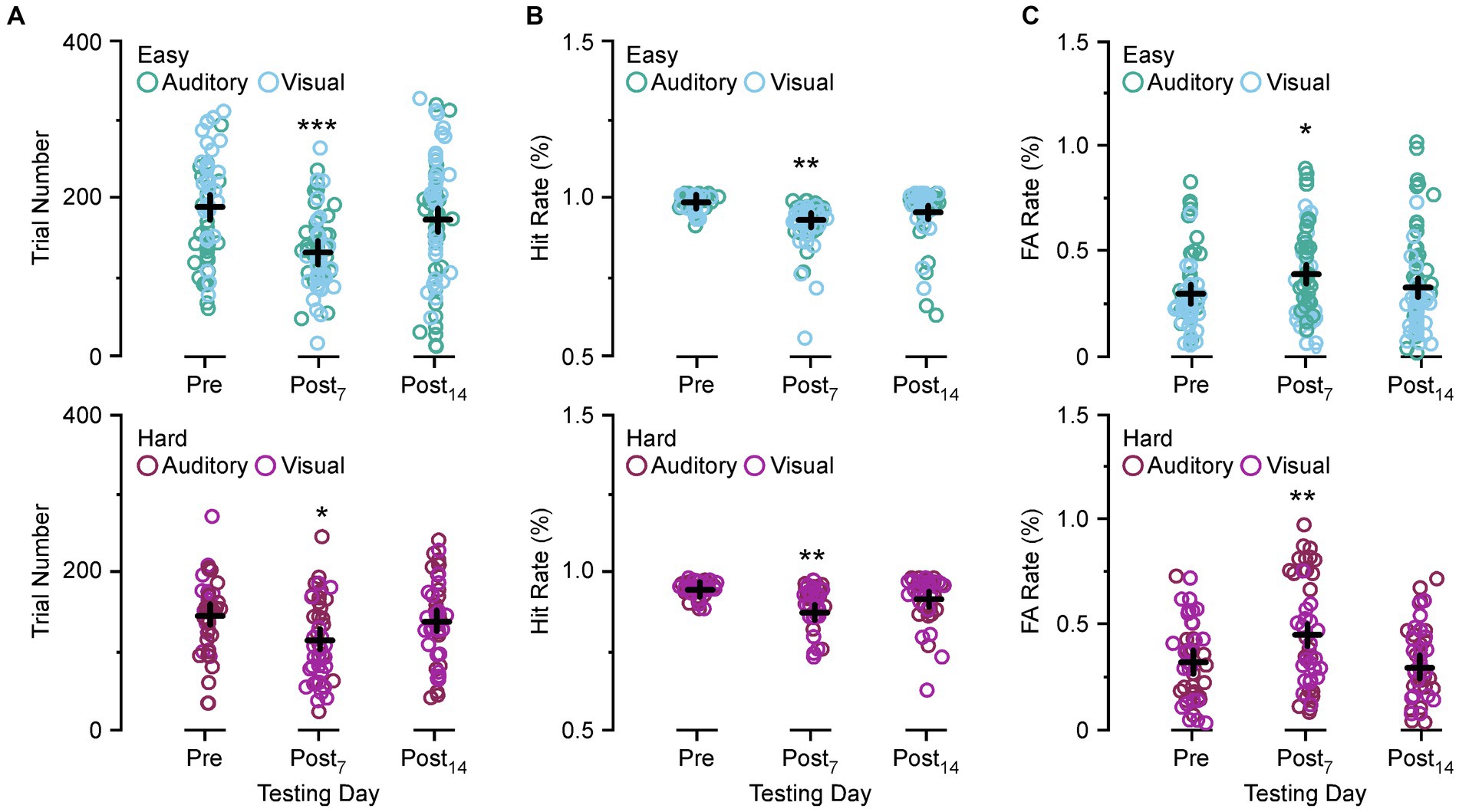
Figure 3. Comparison of the trial numbers performed, Hit rate, and false alarm (FA) rate between preoperative and postoperative days. (A) Scatter diagram showing group comparisons of average trial numbers performed between preoperative testing and postoperative day 7 and 14 for the easy auditory discrimination task (green) and easy visual discrimination task (blue) (top) and hard auditory discrimination task (red) and hard visual discrimination task (purple) (bottom). (B) Scatter diagram showing group comparisons of Hit rate between preoperative testing and postoperative day 7 (Postop7) and postoperative day 14 (Postop14) for the easy auditory discrimination task (green) and easy visual discrimination task (blue) (top) and hard visual discrimination task (purple) (bottom). (C) Scatter diagram showing group comparisons of average FA rate between preoperative testing and postoperative day 7 (Postop7) and postoperative day 14 (Postop14) for the easy auditory discrimination task (green) and easy visual discrimination task (blue) (top) and hard visual discrimination task (purple) (bottom). *p ≤ 0.01, **p ≤ 0.001, ***p ≤ 0.0001.
SSCD induced physiological factors associated with cognitive impairments
Due to the recovery phase of the animals after SSCD and from being food deprived, we did not collect ABR or c+VEMP data at post day 7; however, we did take final recordings at post day 15. Therefore, we tested whether there were specific correlations between ABR thresholds, c+VEMP amplitudes, Hit rates, or FA rates and the last few days of behavioral performance. Figure 4 shows the correlations for the behavioral data at post day 14. Figure 4A illustrates the correlation between ABR thresholds (top) c+VEMP amplitudes (bottom) and d-prime for the auditory and visual discrimination tasks. There was not a significant effect of threshold shift on performance (ABR Threshold [dB] = 0.39–0.05 * behavior (d-prime), adjusted R2 = 0.03, p = 0.146); however, there was a significant correlation of c+VEMP amplitude on behavior (c+VEMP amplitude [μV] = 0.75–0.20 * behavior [d-prime], adjusted R2 = 0.35, p ≤ 0.0001). Despite not having a straightforward group deficit at post day 14, individual differences in animals showed that higher c+VEMP amplitudes at post day 14 were associated with lower performance. Figure 4B shows the correlation between ABR thresholds (top), c+VEMP amplitudes (bottom) and Hit rate for the auditory and visual tasks. It should be noted that Hit rate was not affected by either threshold shifts (ABR Threshold [dB] = 0.40–0.146 * Hit Rate [%], adjusted R2 = −0.017, p = 0.513) or c+VEMP amplitudes (c+VEMP amplitude [μV] = 0.75–0.52 * Hit rate (%), adjusted R2 = 0.033, p = 0.156). However, FA rate was significantly affected by both the threshold shift (ABR Threshold [dB] = 0.182 + 0.287 * FA rate (%), adjusted R2 = 0.16, p = 0.011) and the c+VEMP amplitudes (c+VEMP amplitude [μV] = 0.068 + 0.610 * FA rate (%), adjusted R2 = 0.28, p = 0.0009; Figure 4C). This suggests that interactions between vestibular dysfunction associated with increased c+VEMP amplitudes can lead to behavioral impairments by influencing FA rates.
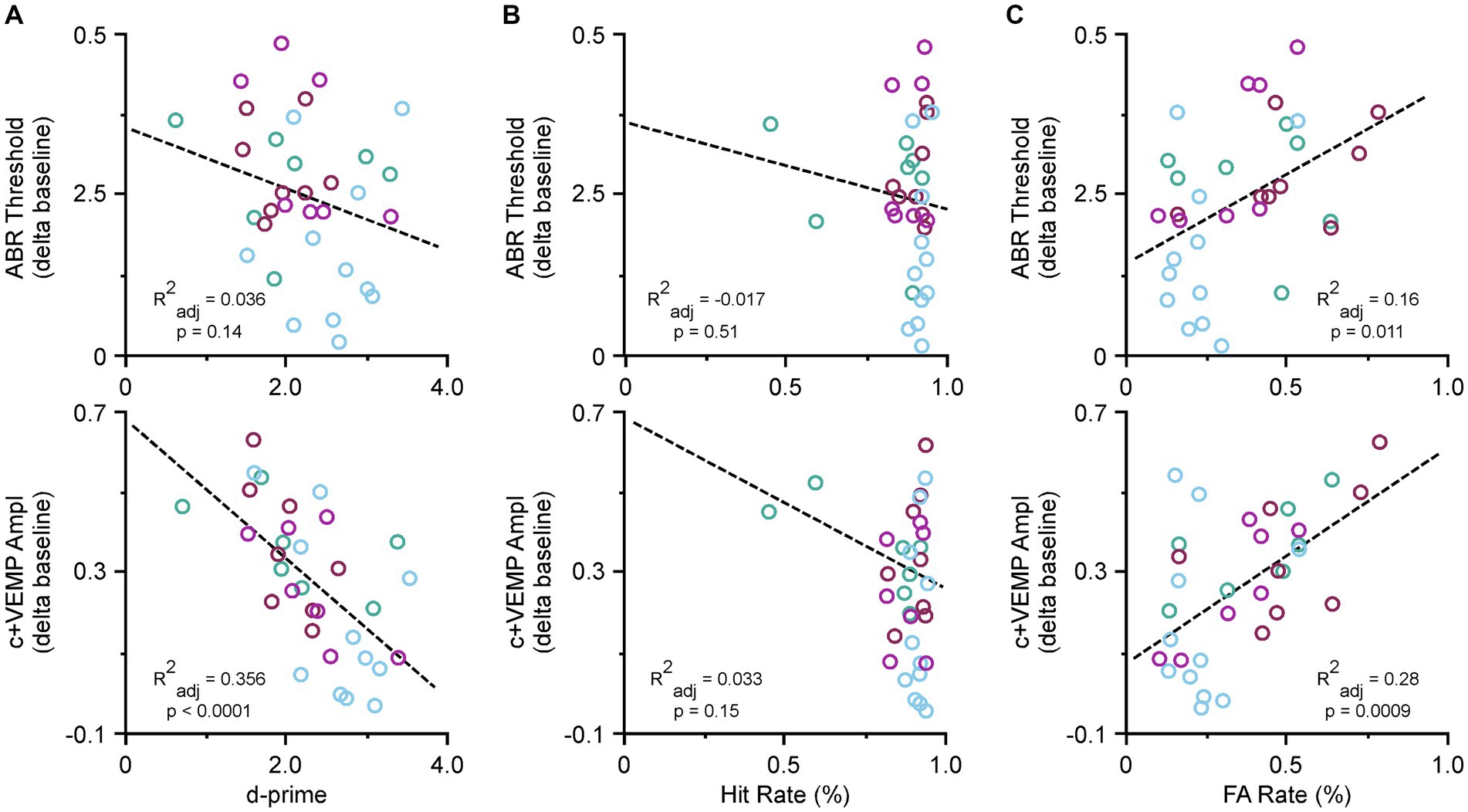
Figure 4. Correlations between ABR thresholds, c+VEMP amplitudes, d-primes, hit rates and false alarm (FA) rates. (A) Scatter plot showing correlations across all tasks for ABR thresholds and d-primes (easy auditory discrimination task [green] and easy visual discrimination task [blue], and hard auditory discrimination task [red] and hard visual discrimination task [purple]) (top) and c+VEMP amplitudes and d-primes (easy auditory discrimination task [green] and easy visual discrimination task [blue], and hard auditory discrimination task [red] and hard visual discrimination task [purple]) (bottom). (B) Scatter plot showing correlations across all tasks for ABR thresholds and hit rates (top) and c+VEMP amplitudes and hit rates (bottom). (C) Scatter plot showing correlations across all tasks for ABR thresholds and false alarm (FA) rates (%) (easy auditory discrimination task [green] and easy visual discrimination task [blue], and hard auditory discrimination task [red] and hard visual discrimination task [purple]) (top) and c+VEMP amplitudes and FA rates (easy auditory discrimination task [green] and easy visual discrimination task [blue], and hard auditory discrimination task [red] and hard visual discrimination task [purple]) (bottom).
Control (sham surgery) experiments
The results of the control (sham surgery) experiments are shown in Table 1; Figure 5. For these analyses we compared data from control (sham surgery) animals (n = 8) to SSCD animals at post day 7 (post days 6–8) and post day 14 (post days 13–15) that had completed 10 days of training followed by 10 days of postoperative training in the easy auditory task (n = 4) and the easy visual task (n = 4; Table 1; Figure 5). There was an additional learning phase in the sham group that led to a significant difference between the sham control and SSCD animals for both the easy discrimination auditory task [F(1,9) = 18.79, p = 0.0019] and the easy visual discrimination task [F(1,13) = 4.99, p = 0.0436]. This could suggest that even though the SSCD animals’ behavioral performance returns to preoperative levels, that the vestibular impairment might have delayed or possibly prevented the additional learning phase. This additional learning was more prominent in the easy auditory discrimination task compared to the easy visual discrimination task. Further evidence is presented in the statistical comparisons of the behavioral metrics and peripheral ear physiology at post day 7 and post day 14 (Table 1; Figure 5).
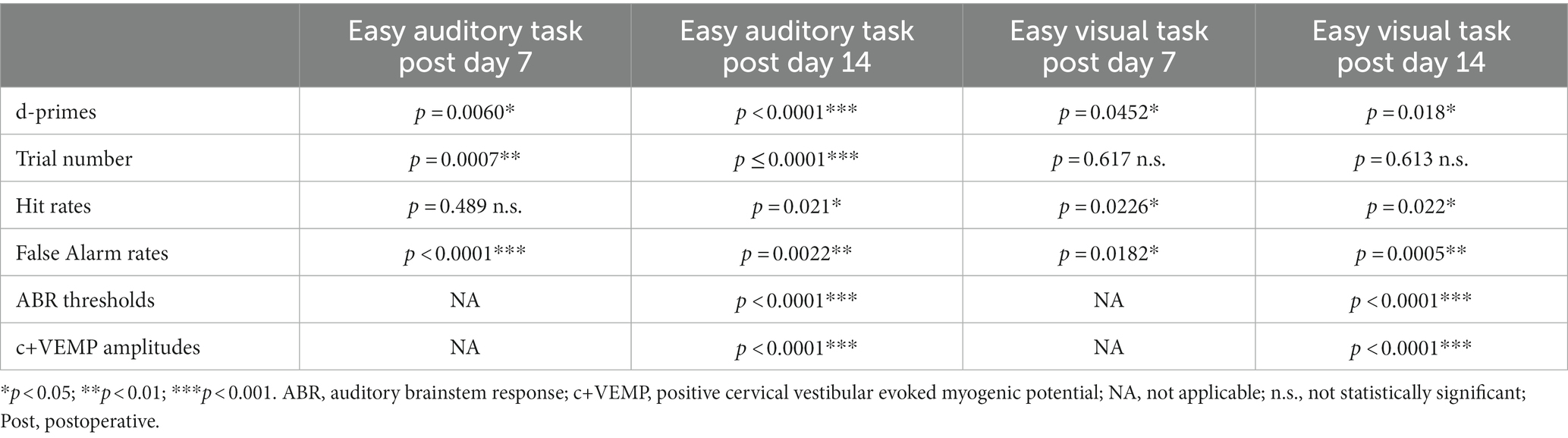
Table 1. Statistical comparisons between SSCD and sham controls for easy auditory task and easy visual task behavioral metrics and physiology.
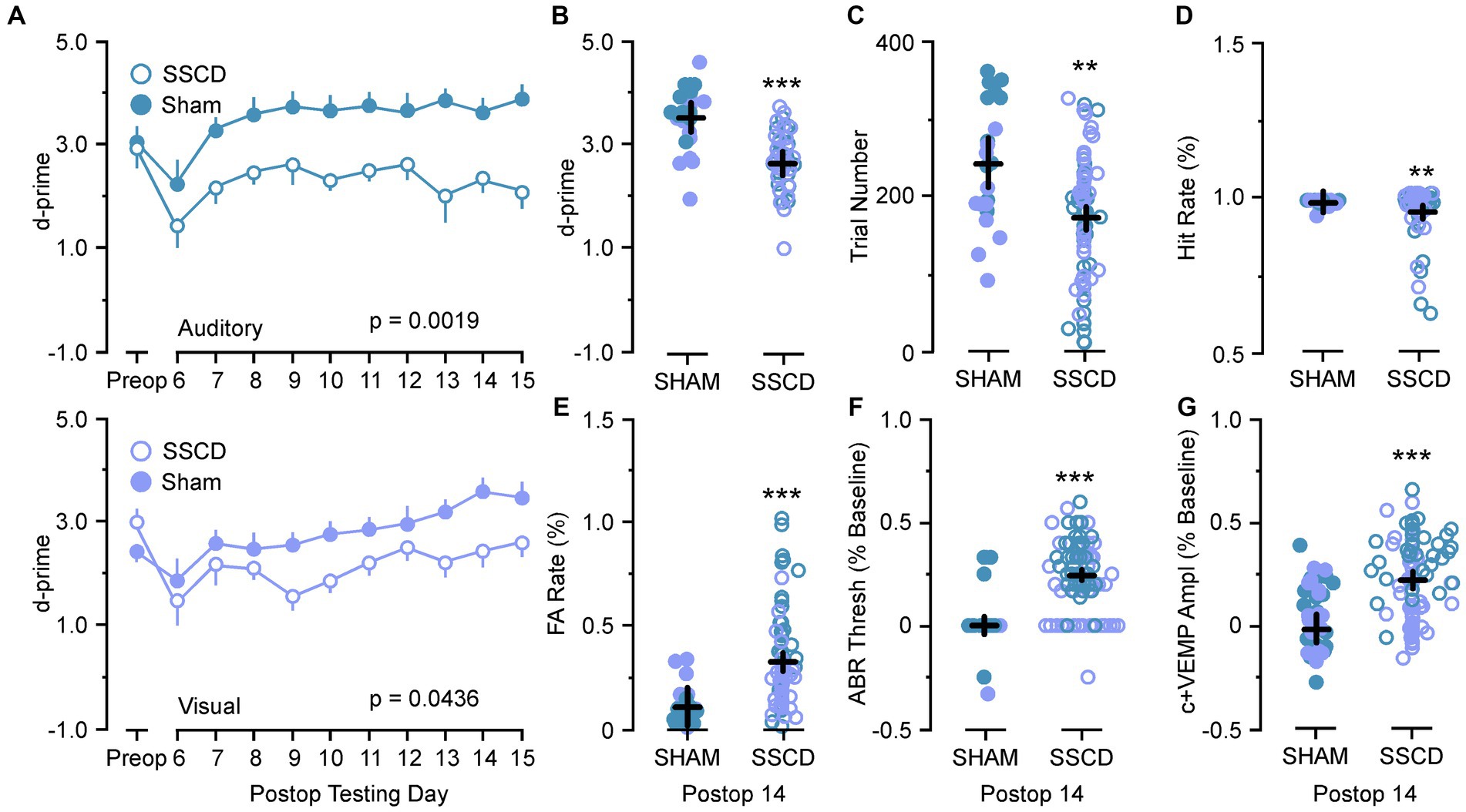
Figure 5. Statistical comparisons between SSCD and SSCD sham control animals. (A) Line graph comparing behavioral performance (d-prime) between SSCD (open blue) group and control (sham surgery) (solid blue) group for the easy auditory discrimination task across testing days (top). Line graph showing comparing behavioral performance (d-prime) between SSCD (open lavender) and control (sham surgery) (solid lavender) animals for the easy visual discrimination task across testing days (bottom). (B) Scatter plot showing the significant increase in d-primes in the control (sham surgery) group (solid colors) over the SSCD group (open colors) at postoperative day 14 (postoperative days 13–15) for both the easy auditory discrimination task (blue) and easy visual discrimination task (lavender). (C) Scatter plot showing that the control (sham surgery) group (solid colors) ran consistently more trials in both easy auditory discrimination task (blue) and easy visual discrimination task (lavender) compared to the SSCD group (open colors) at postoperative day 14. (D) Scatter plot showing the persistent slight decrease in Hit Rate for the SSCD group (open colors) compared to the control (sham surgery) group (solid colors) for both the easy auditory discrimination task (blue) and the easy visual discrimination task (lavender) at postoperative day 14. (E) Scatter plot showing the persistent elevation of false alarm (FA) rates on both the easy auditory discrimination task (blue) and the easy visual discrimination task (lavender) for the SSCD group (open colors) compared to the control (sham surgery) group (solid colors). (F) Scatter plot showing the persistent elevation of ABR thresholds for some SSCD animals (open colors) in both the easy auditory discrimination task (blue) and the easy visual discrimination task (lavender) at postoperative day 14 compared to the control (sham surgery) animals (solid colors). (G) Scatter plot showing the persistent elevation of c+VEMP amplitudes for some SSCD animals (open colors) in both the easy auditory discrimination task (blue) and the easy visual discrimination task (lavender) at postoperative day 14 compared to the control (sham surgery) animals (solid colors). ABR, auditory brainstem response; c+VEMP, positive cervical vestibular evoked myogenic potential; FA, false alarm; Postop, postoperative; Preop, preoperative; SHAM, control (sham surgery); SSCD, superior semicircular canal dehiscence. **p < 0.01, ***p < 0.001.
For the animals that were run through the easy auditory discrimination task there were highly significant differences in the behavioral metrics at post day 7 and post day 14 when compared to SSCD animals. Sham animals ran significantly more trials at both post day 7 (sham post day 7, 208 ± 15 vs. SSCD post day 7, 143 ± 9 [p = 0.0007]) and post day 14 (sham post day 14, 307 ± 24 vs. SSCD post day 14, 155 ± 15 [p < 0.0001]). Their d-primes were significantly higher as well at both time points (sham post day 7, 3.04 ± 0.18 vs. SSCD post day 7, 2.42 ± 0.11 [p = 0.0060]; sham post day 14, 3.79 ± 0.21 vs. SSCD post day 14, 2.31 ± 0.13 [p < 0.0001]). This was driven by significant differences in the FA rates (sham post day 7, 13.3 ± 0.04 vs. SSCD post day 7, 37.8 ± 0.03 [p < 0.0001]; sham post day 14, 7.6 ± 0.19 vs. SSCD post day 14, 22.3 ± 0.02 [p = 0.0022]) as opposed to the differences in Hit rates (sham post day 7, 94.4 ± 0.04 vs. SSCD post day 7, 91.1 ± 0.02 [p = 0.489]; sham post day 14, 91.99 ± 0.008 vs. SSCD post day 14, 96.5 ± 0.005 [p = 0.021]), which were only different at post day 14.
Performance in the easy visual discrimination task was also better in the sham group; however, the results were not as robust. These sham animals did not run more trials than the SSCD animals at either post day 7 (sham post day 7, 132 ± 17 vs. SSCD post day 7, 122 ± 10 [p = 0.617]) or post day 14 (sham post day 14, 192 ± 13 vs. SSCD post day 14, 178 ± 22 [p = 0.613]). There were slightly significant increases in d-primes at post day 7 (sham post day 7, 2.43 ± 0.25 vs. SSCD post day 7, 1.96 ± 0.15 [p = 0.0452]) and post day 14 (sham post day 14, 3.32 ± 0.20 vs. SSCD post day 14, 2.72 ± 0.12 [p = 0.018]), but not nearly as robustly significant as the easy auditory task sham animal d-primes. This was accompanied by slightly significant increases in Hit rate (sham post day 7, 97.3 ± 0.004 vs. SSCD post day 7, 95.2 ± 0.007 [p = 0.0226]; sham post day 14, 98.01 ± 0.03 vs. SSCD post day 14, 87.9 ± 0.02 [p = 0.022]) and lower FA rates (sham post day 7, 23.9 ± 0.02 vs. SSCD post day 7, 37.3 ± 0.04 [p = 0.0182]; sham post day 14, 14.7 ± 0.07 vs. SSCD post day 14, 49.0 ± 0.04 [p = 0.0005]) at both postoperative points.
Finally, significant increases in ABR thresholds remained in the SSCD group for both tasks, whereas sham animals showed no real changes from baseline at post day 14 (easy auditory discrimination task; sham post day 14, 3.3 ± 0.02 vs. SSCD post day 14, 31.0 ± 0.01 [p < 0.0001]; easy visual discrimination task; sham post day 14, 1.1 ± 0.03 vs. SSCD post day 14, 19.3 ± 0.02 [p < 0.0001]). The same trend was present for c+VEMP amplitude measurements (easy auditory task; sham post day 14, 1.1 ± 0.03 vs. SSCD post day 14, 19.3 ± 0.02 [p < 0.0001]; easy visual task; sham post day 14, −1.9 ± 0.05 vs. SSCD post day 14, 12.9 ± 0.03 [p < 0.0001]). As expected, this suggests that the sham surgery does not impose a change to cochlear or vestibular function.
Discussion
Vestibular function plays a crucial role in decision-making, as it provides ongoing real time feedback about body position, balance, and motion that integrates with auditory, visual, and somatosensory inputs (23–27) to make precise judgements regarding our orientation, velocity, and acceleration in relation to our head/world position in space (28). Together the integration of vestibular information across the central nervous system culminates in the emergence of cognitive processing (29). Conversely, dysfunction of the vestibular system leads to cognitive impairments (30). In this study we used a form of vestibular injury, i.e., SSCD, to ask how decision-making in an associative conditioning task is affected by aberrant asymmetric vestibular output. We found decreases in decision-making performance that were highly correlated with the peak and return towards baseline of ABR and c+VEMP measurements that we previously reported (22) and observed in the current study. The c+VEMP amplitude was specifically and highly correlated with variable recovery and lingering decision-making performance deficits. These were consistent across both a visual and auditory task of varying difficulty.
Human cognitive impairment in SSCD and third window syndrome
Previously, there has been controversy over whether there is a direct link between vestibular disorders and certain forms of cognitive impairment (31). However, recent research has shown that the relationship between vestibular function and cognition is incredibly intricate (32, 33), with a high incidence of some form of impairment present in patients with third window syndrome (13, 17, 34–44). In this context, the term “cognitive dysfunction” is being used broadly to refer to characteristics that are not directly influenced by vestibular sensorimotor coupling.
In patients with third window syndrome, including SSCD, cognitive dysfunction is almost always present due to otolithic asymmetry. This is not typically seen in disorders such as benign positional vertigo, vestibular neuronitis, or other rotational receptor dysfunctions (45). Patients with third window syndrome often report cognitive difficulties such as poor memory and concentration, trouble reading, forgetting words, and difficulty expressing themselves. To understand the intricate relationship between vestibular dysfunction and cognitive impairment, we must examine behavioral and anatomical studies in animals. Hitier et al. provide an excellent review of the neuroanatomical pathways from the vestibule to the central nervous system in rodents, cats, and non-human primates (29). Hitier et al. described five major pathways that integrate vestibular information throughout the brain, each with a specific function related to spatial learning and memory (29). These include: “(1) a vestibulo-thalamic-cortical pathway for environmental spatial integration, (2) a tegmental-thalamic-entorhinal pathway for calculating head direction, (3) a reticularis pontis oralis-supramammillary-septal pathway to the hippocampus involved with spatial memory and object recognition, (4) a cerebellar-thalamic-cortical pathway that supports spatial learning, and (5) a vestibular-thalamic-striatal pathway that supports spatial learning and memory.” The detailed anatomical pathways are beyond the scope of this discussion, but it is clear that vestibular dysfunctions, such as those seen in third window syndrome, will affect normal activity along these pathways, resulting in cognitive impairments. The complexity of the vestibular system’s non-classical sensory function has led to debates about the relationship between vestibular function and cognition. In contrast to classic sensory systems like vision, which have modal-specific inputs with straightforward pathways to the cortex via the thalamus, the vestibular system’s sensory inputs are more complex. The brain regions contain stable receptive fields where sensory stimuli are represented by external maps. These maps are retinotopic, tonotopic, and somatotopic, representing the peripheral receptors of the retina, cochlea, and skin throughout each modality specific neuraxis. Vestibular pathways heavily integrate with these modalities through direct vestibular nucleus projections and multisynaptic pathways to higher-order brain regions in the midbrain and thalamus. The core regions integrate environmental spatial auditory and visual information, proprioceptive somatotopic information, and vestibular information about head direction, angular velocity, and momentum. Vestibular information is continually updated and lacks a classical central topographical map, making it difficult to interpret and study. Although animal research investigating this topic is limited, human data offer interesting clues as to how vestibular dysfunction can cause cognitive impairment.
Behavioral features associated with vestibular function/dysfunction and cognitive impairment
To ask questions about cognition in lower animals such as rodents we turn to behavioral paradigms. Many previous animal studies have used unilateral labyrinthectomy and other forms of vestibular stimulation to probe the effect of vestibular dysfunction on various behaviors. The effects of vestibular manipulation on simple behaviors such as open field exploration suggest a definite role is spatial exploratory behavior (46, 47), while spatial navigation in the Morris water maze requires vestibular dependent cues (48). Reference memory in a radial arm maze task is significantly impaired by loss of vestibular information (49–51). Vestibular information even seems to be important for non-spatial processes such as object recognition (52). Studies using non-spatial associative conditioning have not been previously used to examine the effect of vestibular dysfunction on decision-making despite large input to the associative conditioning centers of the striatum (53–55). In this study we used a behavioral task with a decision-making component to ask whether superior semicircular canal dehiscence will produce measurable performance deficits. We found that at a postoperative timepoint associated with the peak of the auditory and vestibular physiological disruption (22), 7 days after SSCD, showed significant behavioral impairments to decision-making. Hit rates were lowered, misses were increased, FAs increased, and correct rejections decreased, leading to overall lowering of d-prime performance measures. These results were consistent across task modality (auditory/visual) and difficulty (easy/hard) suggesting a conserved source for the impairment. It further suggests that the raised auditory thresholds in the left ear were not the causative factor in the auditory task impairments. Observation of the animals showed that they were often confused and would go to an adjacent non-used food trough (misses) or would pause and then continue to the correct food trough (FA, NoGo trial). The increased FA rate was specifically correlated with the presence of a lingering increase in c+VEMP amplitude, which were also significantly correlated with lowered d-primes. Animals that had more recovery towards baseline, had lower c+VEMP amplitudes compared to baseline, lower FA rates, and thus higher d-primes. This suggests a clear connection between the decision-making errors which result in false alarms and the asymmetric vestibular dysfunction. This associative conditioning task has cortical dependent properties to it (56) that could suggest that errors in the decision-making could be due to cortical processing errors that are passed down to the striatum. There could also be hippocampal dependent components associated with spatial reference and navigation to the correct food trough, of which vestibular dysfunction disrupts the allocentric and egocentric reference required to navigate the behavioral arena (49, 50). Along these lines, there could be cerebellar aspects to the impairments, as this structure governs features of vestibular mediated spatial navigation (57).
Control (sham surgery) experiments
There was an additional learning phase in the control (sham surgery) group that led to a significant difference between the control (sham surgery) and SSCD groups for both the easy auditory discrimination task and the easy visual discrimination task. This could suggest that even though the SSCD animals’ behavioral performance returns to preoperative levels, the vestibular impairment might have delayed or possibly prevented the additional learning phase. This additional learning was more prominent in the easy auditory discrimination task compared to the easy visual discrimination task. Further evidence was presented in the statistical comparisons of the behavioral metrics at post day 7 and post day 14 and the peripheral ear physiology at post day 14 (Table 1; Figure 5). For the control (sham surgery) group that completed the easy auditory discrimination task there were highly significant differences in the behavioral metrics at post day 7 and post day 14 when compared to the SSCD group. Their d-primes were significantly higher as well at both time points (Table 1). This was driven by significant differences in the FA rates as opposed to the differences in Hit rates, which were only different at post day 14. Performance in the easy visual discrimination task was also better in the sham group; however, the results were not as robust. Finally, despite the lack of significance when comparing preoperative to post 14 ABR thresholds, and c+VEMPs in the SSCD animals as a group, the comparisons to control (sham surgery) animals revealed that peripheral physiology was still recovering in the SSCD group.
Gerbils as an ideal model to study SSCD central impairments
Altogether, this model of SSCD induced impairment in gerbils adds a powerful new scientific tool to study the effects of vestibular dysfunction on central circuits involved with learning, memory, behavior, and cognition. There is extensive literature that characterizes gerbil visual and auditory function. The gerbil is a diurnal rodent possessing a retina and cone density that is more similar to humans than those of mice and rats (58), providing excellent motion and depth perception (58–60). For auditory function, gerbils possess robust low frequency hearing, and its audiogram overlaps with the human audiogram (61). This contrasts with more standard rodent models, such as mice and rats, as they possess greater sensitivity over very high frequencies and are limited at low frequencies (62, 63). The gerbil also possesses robust auditory perceptual skill (56, 64–81) that is vulnerable to hearing impairment (71, 75, 76, 82–85). Furthermore, previous work in gerbils also demonstrated that cognitive variables, such as the ability to generalize a learned rule, are vulnerable to hearing loss (86). More recently, the gerbil has been utilized as a model to assess temporal integration, a hallmark of cognitive function, during auditory decision-making (80, 84, 87). Thus, the gerbil is a suitable animal model for assessing cognitive function, particularly across visual and auditory domains.
An important issue with this animal model, in the context of measuring cognitive dysfunction/impaired decision-making, is the question of when the acute vestibular injury induced by surgically creating the SSCD becomes a chronic intermittent vestibular asymmetry. With acquired SSCD in patients, at some point the dehiscence is an acute change and because of the longstanding presence of the dehiscence, patients experience a chronic intermittent vestibular asymmetry. While the timeline for transition from acute injury to chronic condition in our gerbil model that parallels the human experience is unknown, in general rodent timelines are more rapid than in humans, as is the development milestones, aging and shorter life expectancy. We do not know the how the gerbil development timeline maps to that of humans; however, we do know that in adult rats, every day of the animal is approximately equivalent to 34.8 human days (88). It is known that in gerbils with unilateral labyrinthectomy (a more severe acute vestibular injury), vestibular compensation with vision shows improved vestibulo-ocular reflex (VOR) just 24 h after labyrinthectomy (89). Thus, we expect that any acute vestibular injury had resolved by post day 7. To minimize effects due to acute vestibular injury, our exclusion criteria included removing animals from the protocol that had persistent circling or head tilt present at post SSCD day 3. Of those animals included in this study (n = 33), 85% (n = 28) had no head tilt or circling after surgical creation of the SSCD and 15% (n = 8) had head tilt that resolved by post day 3 and before electrophysiologic and behavioral testing beginning on post day 7. We know from previous studies that the surgically created SSCD resurfaces via spontaneous osteoneogenesis by post day 14 (22), and our electrophysiologic and behavioral results in this study suggest that the SSCD also closed by post day 14.
To enhance our comprehension of the effects of the vestibular system on behavior, cognition, and symptomatology in central brain processes, it is essential to systematically investigate analogous animal models of vestibular dysfunction. This approach will allow us to create experiments that reproduce the peripheral causes of vestibular dysfunction and explore central alterations along the five pathways outlined earlier (29). Recently, our team has developed a gerbil model of third window syndrome that will serve as a foundation for a thorough examination of the symptomatology patients with SSCD experience (22). This model involves creating a fenestration in the superior semicircular canal that produces an inner ear/otic capsule-conductive hearing loss and sound-evoked changes in vestibular evoked myogenic potentials (c+VEMPs) similar to what is seen in humans with third window syndrome due to SSCD. As reported herein, this model also displays substantial reversible impairments in decision-making, which we can use to investigate the maladaptive central plasticity resulting from vestibular injury that leads to cognitive dysfunction. This eliminates the need for a second surgical intervention to plug the SSCD, offering us an experimental window to carry out electrophysiological and behavioral studies to evaluate decision-making as a proxy for cognitive dysfunction. This model will also aid in determining the precise vestibular contributions of the five primary neuroanatomical pathways described earlier (29) and assist in understanding the central plasticity resulting from SSCD. Advanced tools such as adeno-associated viruses (e.g., optogenetics; ChR2, DREADDs; HM4Di), along with improvements in awake behaving neurophysiology and in vitro whole-cell recording, can be employed to isolate and manipulate specific brain circuits selectively. This will allow us to pose highly informative questions about the effect of vestibular function on physiology (e.g., balance), emotional states (e.g., anxiety, fear), and cognitive-behavioral processes. By unraveling the intricacies of vestibular influence on central brain function, we should obtain new insights into the etiology of symptomatology in humans that will hopefully result in new treatment approaches for chronic third window syndrome symptoms and other vestibular-related conditions in the years ahead.
Conclusion
Vestibular dysfunction in humans is associated with cognitive deficits that can lead to greatly reduced quality of life. Fortunately, treatments do exist for disorders such as SSCD, that involve surgical plugging or resurfacing the site of dehiscence. To enhance our understanding of how vestibular function affects behavior, cognition, and the central brain processes, it is necessary to systematically investigate vestibular disorders in animal models that are analogous to humans. Unlike humans who require surgical intervention, a particularly novel aspect of this SSCD model is the fenestration self-closure of the surgically created SSCD through spontaneous osteoneogenesis (22). This allows us the unique opportunity to study both impairment and recovery. We can also exploit this feature of the model to determine if there are persistent changes to central plasticity after recovery from injury. Herein, we report for the first-time, higher order associative decision-making impairments related to aberrant asymmetric vestibular output in an animal model of SSCD. These impairments resolve as the bone resurfaces via spontaneous osteoneogenesis; providing a unique window to study decision-making errors and their resolution. We can exploit this timeframe to design experiments that replicate the peripheral causes of vestibular dysfunction in SSCD and examine the central changes that underlie this dysfunction along many different brain circuits that receive vestibular inputs. We hope to expand these results in future experiments that study many of the circuits along which auditory, visual, and vestibular pathways integrate to drive learning, behavior, and higher-order cognition.
Data availability statement
The raw data supporting the conclusions of this article will be made available by the authors, without undue reservation.
Ethics statement
The animal study was approved by the Rutgers University IACUC reviewed and approved this research protocol (PROTO202000179). The study was conducted in accordance with the local legislation and institutional requirements.
Author contributions
TMM: Conceptualization, Data curation, Formal analysis, Funding acquisition, Investigation, Methodology, Project administration, Resources, Software, Supervision, Validation, Visualization, Writing – original draft, Writing – review & editing. PAW: Conceptualization, Data curation, Formal analysis, Funding acquisition, Investigation, Methodology, Project administration, Resources, Software, Supervision, Validation, Visualization, Writing – original draft, Writing – review & editing. JN: Data curation, Formal analysis, Writing – review & editing. ED: Data curation, Formal analysis, Writing – review & editing. RDS: Data curation, Formal analysis, Writing – review & editing. AT: Data curation, Formal analysis, Writing – review & editing. NLC: Data curation, Formal analysis, Writing – review & editing. MAB: Data curation, Formal analysis, Writing – review & editing. SSH: Writing – review & editing, Data curation, Formal analysis. JDY: Formal analysis, Validation, Writing – original draft, Writing – review & editing.
Funding
The author(s) declare financial support was received for the research, authorship, and/or publication of this article. The Rutgers Biomedical Health Sciences Chancellor Scholar Award to PAW provided funding to help support this work.
Conflict of interest
The authors declare that the research was conducted in the absence of any commercial or financial relationships that could be construed as a potential conflict of interest.
The author(s) declared that they were an editorial board member of Frontiers, at the time of submission. This had no impact on the peer review process and the final decision.
Publisher’s note
All claims expressed in this article are solely those of the authors and do not necessarily represent those of their affiliated organizations, or those of the publisher, the editors and the reviewers. Any product that may be evaluated in this article, or claim that may be made by its manufacturer, is not guaranteed or endorsed by the publisher.
References
1. Xie, Y, Bigelow, RT, Frankenthaler, SF, Studenski, SA, Moffat, SD, and Agrawal, Y. Vestibular loss in older adults associated with impaired spatial navigation: data from the triangle completion task. Front Neurol. (2017) 8:173. doi: 10.3389/fneur.2017.00173
2. Kremmyda, O, Hüfner, K, Flanagin, VL, Hamilton, DA, Linn, J, and Strupp, M. Beyond dizziness: virtual navigation, spatial anxiety and hippocampal volume in bilateral vestibulopathy. Front Hum Neurosci. (2016) 10:139. doi: 10.3389/fnhum.2016.00139
3. Guidetti, G, Guidetti, R, Manfredi, M, and Manfredi, M. Vestibular pathology and spatial working memory. Acta Otorhinolaryngol Ital. (2020) 40:72–8. doi: 10.14639/0392-100X-2189
4. Smith, L, Wilkinson, D, Bodani, M, Bicknell, R, and Surenthiran, SS. Short-term memory impairment in vestibular patients can arise independently of psychiatric impairment, fatigue, and sleeplessness. J Neuropsychol. (2019) 13:417–31. doi: 10.1111/jnp.12157
5. Popp, P, Wulff, M, Finke, K, Rühl, M, Brandt, T, and Dieterich, M. Cognitive deficits in patients with a chronic vestibular failure. J Neurol. (2017) 264:554–63. doi: 10.1007/s00415-016-8386-7
6. Ayar, DA, Kumral, E, and Celebisoy, N. Cognitive functions in acute unilateral vestibular loss. J Neurol. (2020) 267:153–9. doi: 10.1007/s00415-020-09829-w
7. Bigelow, RT, Semenov, YR, Trevino, C, Ferrucci, L, Resnick, SM, Simonsick, EM, et al. Association between visuospatial ability and vestibular function in the Baltimore longitudinal study of aging. J Am Geriatr Soc. (2015) 63:1837–44. doi: 10.1111/jgs.13609
8. Dobbels, B, Mertens, G, Gilles, A, Claes, A, Moyaert, J, van de Berg, R, et al. Cognitive function in acquired bilateral vestibulopathy: a cross-sectional study on cognition, hearing, and vestibular loss. Front Neurosci. (2019) 13:340. doi: 10.3389/fnins.2019.00340
9. Wackym, PA, Balaban, CD, Mackay, HT, Wood, SJ, Lundell, CJ, Carter, DM, et al. Longitudinal cognitive and neurobehavioral functional outcomes after repairing otic capsule dehiscence. Otol Neurotol. (2016) 37:70–82. doi: 10.1097/MAO.0000000000000928
10. Chari, DA, Liu, YH, Chung, JJ, and Rauch, SD. Subjective cognitive symptoms and dizziness handicap inventory (DHI) performance in patients with vestibular migraine and Menière's disease. Otol Neurotol. (2021) 42:883–9. doi: 10.1097/MAO.0000000000003081
11. Liu, YF, Locklear, TD, Sharon, JD, Lacroix, E, Nguyen, SA, and Rizk, HG. Quantification of cognitive dysfunction in dizzy patients using the neuropsychological vertigo inventory. Otol Neurotol. (2019) 40:e723–31. doi: 10.1097/MAO.0000000000002311
12. Eraslan Boz, H, Kırkım, G, Koçoğlu, K, Çakır Çetin, A, Akkoyun, M, Güneri, EA, et al. Cognitive function in Meniere's disease. Psychol Health Med. (2023) 28:1076–86. doi: 10.1080/13548506.2022.2144637
13. Wackym, PA, Balaban, CD, Zhang, P, Siker, DA, and Hundal, JS. Third window syndrome: surgical management of cochlea-facial dehiscence. Front Neurol. (2019) 10:1281. doi: 10.3389/fneur.2019.01281
14. Iversen, MM, and Rabbitt, RD. Biomechanics of third window syndrome. Front Neurol. (2020) 11:891. doi: 10.3389/fneur.2020.00891
15. Carey, JP, Minor, LB, and Nager, GT. Dehiscence or thinning of bone overlying the superior semicircular canal in a temporal bone survey. Arch Otolaryngol Head Neck Surg. (2000) 26:137–47. doi: 10.1001/archotol.126.2.137
16. Minor, LB . Clinical manifestations of superior semicircular canal dehiscence. Laryngoscope. (2005) 115:1717–27. doi: 10.1097/01.mlg.0000178324.55729.b7
17. Ward, BK, Carey, JP, and Minor, LB. Superior canal dehiscence syndrome: lessons from the first 20 years. Front Neurol. (2017) 8:177. doi: 10.3389/fneur.2017.00177
18. Wackym, PA, Agrawal, Y, Ikezono, T, and Balaban, CD. Editorial: third window syndrome. Front Neurol. (2021) 12:704095. doi: 10.3389/fneur.2021.704095
19. Gurvich, C, Maller, JJ, Lithgow, B, Haghgooie, S, and Kulkarni, J. Vestibular insights into cognition and psychiatry. Brain Res. (2013) 1537:244–59. doi: 10.1016/j.brainres.2013.08.058
20. Lewald, J, and Karnath, HO. Vestibular influence on human auditory space perception. J Neurophysiol. (2000) 84:1107–11. doi: 10.1152/jn.2000.84.2.1107
21. Oh, S-Y, Boegle, R, Ertl, M, Stephan, T, and Dieterich, M. Multisensory vestibular, vestibular-auditory, and auditory network effects revealed by parametric sound pressure stimulation. NeuroImage. (2018) 1:354–63. doi: 10.1016/j.neuroimage.2018.04.057
22. Wackym, PA, Balaban, CD, Van Osch, OJ, Morris, BT, Tamakloe, M-A, Salvatore, VL, et al. New model of superior semicircular canal dehiscence with reversible diagnostic findings characteristic of patients with the disorder. Front Neurol. (2023) 13:1035478. doi: 10.3389/fneur.2022.1035478
23. Bakker, RS, Selen, LPJ, and Medendorp, WP. Transformation of vestibular signals for the decisions of hand choice during whole body motion. J Neurophysiol. (2019) 121:2392–400. doi: 10.1152/jn.00470.2018
24. Genzel, D, Firzlaff, U, Wiegrebe, L, and Mac Neilage, PR. Dependence of auditory spatial updating on vestibular, proprioceptive, and efference copy signals. J Neurophysiol. (2016) 116:765–75. doi: 10.1152/jn.00052.2016
25. Rincon-Gonzalez, L, Selen, LP, Halfwerk, K, Koppen, M, Corneil, BD, and Medendorp, WP. Decisions in motion: vestibular contributions to saccadic target selection. J Neurophysiol. (2016) 116:977–85. doi: 10.1152/jn.01071.2015
26. Gao, W, Lin, Y, Shen, J, Han, J, Song, X, Lu, Y, et al. Diverse effects of gaze direction on heading perception in humans. Cereb Cortex. (2023) 2:bhac 541. doi: 10.1093/cercor/bhac541
27. Goel, R, De Dios, YE, Gadd, NE, Caldwell, EE, Peters, BT, Reschke, MF, et al. Assessing somatosensory utilization during unipedal postural control. Front Syst Neurosci. (2017) 11:21. doi: 10.3389/fnsys.2017.00021
28. Mac Neilage, PR, Banks, MS, DeAngelis, GC, and Angelaki, DE. Vestibular heading discrimination and sensitivity to linear acceleration in head and world coordinates. J Neurosci. (2010) 30:9084–94. doi: 10.1523/JNEUROSCI.1304-10.2010
29. Hitier, M, Besnard, S, and Smith, PF. Vestibular pathways involved in cognition. Front Integr Neurosci. (2014) 8:59. doi: 10.3389/fnint.2014.00059
30. Chari, DA, Madhani, A, Sharon, JD, and Lewis, RF. Evidence for cognitive impairment in patients with vestibular disorders. J Neurol. (2022) 269:5831–42. doi: 10.1007/s00415-022-11289-3
31. Gizzi, M, Zlotnick, M, Cicerone, K, and Riley, E. Vestibular disease and cognitive dysfunction: no evidence for a causal connection. J Head Trauma Rehabil. (2003) 18:398–407. doi: 10.1097/00001199-200309000-00002
32. Ferrè, ER, and Haggard, P. Vestibular cognition: state-of-the-art and future directions. Cogn Neuropsychol. (2020) 37:413–20. doi: 10.1080/02643294.2020.1736018
33. Smith, PF . The vestibular system and cognition. Curr Opin Neurol. (2017) 30:84–9. doi: 10.1097/WCO.0000000000000403
34. Wackym, PA . Right Perilymph Fistula Not Superior Canal Dehiscence. Patient Video Describing Symptoms Before and After Surgical Repair; (2023). Available at: https://www.youtube.com/watch?v=bDph0B0uLbg. (Accessed September 15, 2023)
35. Wackym, PA . Right Cochlea-Facial Nerve Dehiscence: 16 Year Old Thought to Have Conversion Disorder; (2019). Available at: https://youtu.be/fTjsnnUALBw. (Accessed September 15, 2023)
36. Wackym, PA . Perilymph Fistula; (2012). Available at: https://www.youtube.com/watch?v=jSAM6h-7Mwc. (Accessed September 15, 2023)
37. Wackym, PA . Right Superior Semicircular Canal Dehiscence Repair: Symptoms and Recovery; (2017). Available at: https://youtu.be/er4k8NZrG2I. (Accessed September 15, 2023)
38. Wackym, PA . Recurrent Third Window Syndrome Co-Morbidity: Functional Neurological Symptom Disorder; (2017). Available at: https://youtu.be/AgUy07QxTxo. (Accessed September 15, 2023)
39. Wackym, PA . Otic Capsule Dehiscence Syndrome in One Ear After a Car Accident; (2015). Available at: https://www.youtube.com/watch?v=1Nl9T6etxqM. (Accessed September 15, 2023)
40. Wackym, PA . Cochlea-Facial Nerve Dehiscence: Traumatic Third Window Syndrome After a Snowboarding Accident; (2019). Available at: https://youtu.be/NCDMD5FGf-w. (Accessed September 15, 2023)
41. Wackym, PA . Surgery for Cochlea-Facial Nerve Dehiscence: Symptoms and Tuning Fork Testing; (2019). Available at: https://youtu.be/lFR-zdYlIsY. (Accessed September 15, 2023)
42. Wackym, PA . Tuning Fork Testing in Otic Capsule Dehiscence Syndrome; (2015). Available at: https://www.youtube.com/watch?v=Szp_kO8oVos. (Accessed September 15, 2023)
43. Wackym, PA . Tuning Fork Testing Before and After Repair of Two Types of Otic Capsule Dehiscence; (2015). Available at: https://www.youtube.com/watch?v=NIauJPbvSpA. (Accessed September 15, 2023)
44. Wackym, PA . Vestibular Migraine. Patient Video Describing Symptoms Before and After Treatment with Topamax; (2012). https://www.youtube.com/watch?v=Zy7YjCDnLYM. (Accessed September 15, 2023)
45. Demirhan, MA, and Celebisoy, N. Cognitive functions in episodic vestibular disorders: Meniere's disease and vestibular migraine. J Vestib Res. (2023) 33:63–70. doi: 10.3233/VES-220025
46. Porter, JD, Pellis, SM, and Meyer, ME. An open-field activity analysis of labyrinthectomized rats. Physiol Behav. (1990) 48:27–30. doi: 10.1016/0031-9384(90)90255-3
47. Banovetz, MT, Lake, RI, Blackwell, AA, Oltmanns, JR, Schaeffer, EA, Yoder, RM, et al. Effects of acquired vestibular pathology on the organization of mouse exploratory behavior. Exp Brain Res. (2021) 239:1125–39. doi: 10.1007/s00221-020-06032-1
48. Clark, BJ, Hong, NS, Bettenson, DJ, Woolford, J, Horwood, L, and McDonald, RJ. Maintained directional navigation across environments in the Morris water task is dependent on vestibular cues. J Exp Psychol Anim Learn Cogn. (2015) 41:301–8. doi: 10.1037/xan0000066
49. Russell, NA, Horii, A, Smith, PF, Darlington, CL, and Bilkey, DK. Bilateral peripheral vestibular lesions produce long-term changes in spatial learning in the rat. J Vestib Res. (2003) 13:9–16. doi: 10.3233/VES-2003-13102
50. Russell, NA, Horii, A, Smith, PF, Darlington, CL, and Bilkey, DK. Long-term effects of permanent vestibular lesions on hippocampal spatial firing. J Neurosci. (2003) 23:6490–8. doi: 10.1523/JNEUROSCI.23-16-06490.2003
51. Ossenkopp, KP, and Hargreaves, EL. Spatial learning in an enclosed eight-arm radial maze in rats with sodium arsanilate-induced labyrinthectomies. Behav Neural Biol. (1993) 59:253–7. doi: 10.1016/0163-1047(93)91034-k
52. Zheng, Y, Darlington, CL, and Smith, PF. Bilateral labyrinthectomy causes long-term deficit in object recognition in rat. Neuroreport. (2004) 15:1913–6. doi: 10.1097/00001756-200408260-00016
53. Kato, S, Nishizawa, K, and Kobayashi, K. Thalamostriatal system controls the acquisition, performance, and flexibility of learning behavior. Front Syst Neurosci. (2021) 15:729389. doi: 10.3389/fnsys.2021.729389
54. Stiles, L, and Smith, PF. The vestibular–basal ganglia connection: balancing motor control. Brain Res. (2015) 1597:180–8. doi: 10.1016/j.brainres.2014.11.063
55. Sabzevar, FT, Vautrelle, N, Zheng, Y, and Smith, PF. Vestibular modulation of the tail of the rat striatum. Sci Reports. (2023) 13:4443. doi: 10.1038/s41598-023-31289-1
56. Paraouty, N, and Mowery, TM. Early sensory deprivation leads to differential inhibitory changes in the striatum during learning. Front Neural Circuits. (2021) 15:670858. doi: 10.3389/fncir.2021.670858
57. Zachou, A, and Bronstein, AM. Vestibulo-spatial navigation: pathways and sense of direction. J Neurophysiol. (2023) 129:672–84. doi: 10.1152/jn.00422.2022
58. Yang, S, Luo, X, Xiong, G, So, KF, Yang, H, and Xu, Y. The electroretinogram of Mongolian gerbil (Meriones unguiculatus): comparison to mouse. Neurosci Lett. (2015) 589:7–12. doi: 10.1016/j.neulet.2015.01.018
59. Baker, AG, and Emerson, VF. Grating acuity of the Mongolian gerbil (Meriones unguiculatus). Behav Brain Res. (1983) 8:195–209. doi: 10.1016/0166-4328(83)90054-2
60. Jacobs, GH, and Deegan, JF 2nd. Sensitivity to ultraviolet light in the gerbil (Meriones unguiculatus): characteristics and mechanisms. Vis Res. (1994) 34:1433–41. doi: 10.1016/0042-6989(94)90144-9
61. Garbers, C, Henke, J, Leibold, C, Wachtler, T, and Thurley, K. Contextual processing of brightness and color in Mongolian gerbils. J Vis. (2015) 15:13–4. doi: 10.1167/15.1.13
62. Kelly, JB, and Masterton, B. Auditory sensitivity of the albino rat. J Comp Psychol (Baltim). (1977) 91:930–6. doi: 10.1037/h0077356
63. Radziwon, KE, June, KM, Stolzberg, DJ, Xu-Friedman, MA, Salvi, RJ, and Dent, ML. Behaviorally measured audiograms and gap detection thresholds in CBA/CaJ mice. J Comp Physiol. (2009) 195:961–9. doi: 10.1007/s00359-009-0472-1
64. Ohl, FW, Wetzel, W, Wagner, T, Rech, A, and Scheich, H. Bilateral ablation of auditory cortex in Mongolian gerbil affects discrimination of frequency modulated tones but not of pure tones. Learning Memory. (1999) 6:347–62. doi: 10.1101/lm.6.4.347
65. Ohl, FW, Scheich, H, and Freeman, WJ. Change in pattern of ongoing cortical activity with auditory category learning. Nature. (2001) 412:733–6. doi: 10.1038/35089076
66. Wagner, E, Klump, GM, and Hamann, I. Gap detection in Mongolian gerbils (Meriones unguiculatus). Hear Res. (2003) 176:11–6. doi: 10.1016/S0378-5955(02)00643-3
67. Hamann, I, Gleich, O, Klump, GM, Kittel, MC, and Strutz, J. Age-dependent changes of gap detection in the Mongolian gerbil (Meriones unguiculatus). J Assoc Res Otolaryngol. (2004) 5:49–57. doi: 10.1007/s10162-003-3041-2
68. Maier, JK, and Klump, GM. Resolution in azimuth sound localization in the Mongolian gerbil (Meriones unguiculatus). J Acoust Soc Am. (2006) 119:1029–36. doi: 10.1121/1.2159429
69. Gleich, O, Hamann, I, Kittel, MC, Klump, GM, and Strutz, J. A quantitative analysis of psychometric functions for different auditory tasks in gerbils. Hear Res. (2006) 220:27–37. doi: 10.1016/j.heares.2006.06.014
70. Wetzel, W, Ohl, FW, and Scheich, H. Global versus local processing of frequency-modulated tones in gerbils: an animal model of lateralized auditory cortex functions. Proc Natl Acad Sci U S A. (2008) 105:6753–8. doi: 10.1073/pnas.0707844105
71. Buran, BN, von Trapp, G, and Sanes, DH. Behaviorally gated reduction of spontaneous discharge can improve detection thresholds in auditory cortex. J Neurosci. (2014) 34:4076–81. doi: 10.1523/JNEUROSCI.4825-13.2014
72. Buran, BN, Sarro, EC, Manno, FA, Kang, R, Caras, ML, and Sanes, DH. A sensitive period for the impact of hearing loss on auditory perception. J Neurosci. (2014) 34:2276–84. doi: 10.1523/JNEUROSCI.0647-13.2014
73. Sarro, EC, von Trapp, G, Mowery, TM, Kotak, VC, and Sanes, DH. Cortical synaptic inhibition declines during auditory learning. J Neurosci. (2015) 35:6318–25. doi: 10.1523/JNEUROSCI.4051-14.2015
74. von Trapp, G, Buran, BN, Sen, K, Semple, MN, and Sanes, DH. A decline in response variability improves neural signal detection during auditory task performance. J Neurosci. (2016) 36:11097–106. doi: 10.1523/JNEUROSCI.1302-16.2016
75. Caras, ML, and Sanes, DH. Top-down modulation of sensory cortex gates perceptual learning. Proc Natl Acad Sci U S A. (2017) 114:9972–7. doi: 10.1073/pnas.1712305114
76. Caras, ML, and Sanes, DH. Neural variability limits adolescent skill learning. J Neurosci. (2019) 39:2889–902. doi: 10.1523/JNEUROSCI.2878-18.2019
77. Tolnai, S, Beutelmann, R, and Klump, GM. Exploring binaural hearing in gerbils (Meriones unguiculatus) using virtual headphones. PLoS One. (2017) 12:e0175142. doi: 10.1371/journal.pone.0175142
78. Mowery, TM, Caras, ML, Hassan, SI, Wang, DJ, Dimidschstein, J, Fishell, G, et al. Preserving inhibition during developmental hearing loss rescues auditory learning and perception. J Neurosci. (2019) 39:8347–61. doi: 10.1523/JNEUROSCI.0749-19.2019
79. Eipert, L, and Klump, GM. Uncertainty-based informational masking in a vowel discrimination task for young and old Mongolian gerbils. Hear Res. (2020) 392:107959. doi: 10.1016/j.heares.2020.107959
80. Yao, JD, and Sanes, DH. Temporal encoding is required for categorization, but not discrimination. Cereb Cortex. (2021) 31:2886–97. doi: 10.1093/cercor/bhaa396
81. Jüchter, C, Beutelmann, R, and Klump, GM. Speech sound discrimination by Mongolian gerbils. Hear Res. (2022) 418:108472. doi: 10.1016/j.heares.2022.108472
82. Rosen, MJ, Sarro, EC, Kelly, JB, and Sanes, DH. Diminished behavioral and neural sensitivity to sound modulation is associated with moderate developmental hearing loss. PLoS One. (2012) 7:e41514. doi: 10.1371/journal.pone.0041514
83. Ihlefeld, A, Chen, YW, and Sanes, DH. Developmental conductive hearing loss reduces modulation masking release. Trends Hear. (2016) 20:2331216516676255. doi: 10.1177/2331216516676255
84. Yao, JD, and Sanes, DH. Developmental deprivation-induced perceptual and cortical processing deficits in awake-behaving animals. elife. (2018) 7:e33891. doi: 10.7554/eLife.33891
85. Anbuhl, KL, Yao, JD, Hotz, RA, Mowery, TM, and Sanes, DH. Auditory processing remains sensitive to environmental experience during adolescence in a rodent model. Nat Commun. (2022) 13:2872. doi: 10.1038/s41467-022-30455-9
86. von Trapp, G, Aloni, I, Young, S, Semple, MN, and Sanes, DH. Developmental hearing loss impedes auditory task learning and performance in gerbils. Hear Res. (2017) 347:3–10. doi: 10.1016/j.heares.2016.07.020
87. Yao, JD, Zemlianova, KO, Hocker, DL, Savin, C, Constantinople, CM, Chung, S, et al. Transformation of acoustic information to sensory decision variables in the parietal cortex. Proc Natl Acad Sci U S A. (2023) 120:e2212120120. doi: 10.1073/pnas.2212120120
88. Sengupta, P . The laboratory rat: relating its age with human's. Int J Prev Med. (2013) 4:624–30.
Keywords: cognitive dysfunction, decision-making, dizziness, headache, migraine, superior semicircular canal dehiscence, vestibular
Citation: Mowery TM, Wackym PA, Nacipucha J, Dangcil E, Stadler RD, Tucker A, Carayannopoulos NL, Beshy MA, Hong SS and Yao JD (2023) Superior semicircular canal dehiscence and subsequent closure induces reversible impaired decision-making. Front. Neurol. 14:1259030. doi: 10.3389/fneur.2023.1259030
Edited by:
Rick Friedman, University of California, San Diego, United StatesReviewed by:
Gerard Joseph Gianoli, The Ear and Balance Institute, United StatesQuinton Gopen, University of California, Los Angeles, United States
Copyright © 2023 Mowery, Wackym, Nacipucha, Dangcil, Stadler, Tucker, Carayannopoulos, Beshy, Hong and Yao. This is an open-access article distributed under the terms of the Creative Commons Attribution License (CC BY). The use, distribution or reproduction in other forums is permitted, provided the original author(s) and the copyright owner(s) are credited and that the original publication in this journal is cited, in accordance with accepted academic practice. No use, distribution or reproduction is permitted which does not comply with these terms.
*Correspondence: Todd M. Mowery, dG02OTJAcndqbXMucnV0Z2Vycy5lZHU=