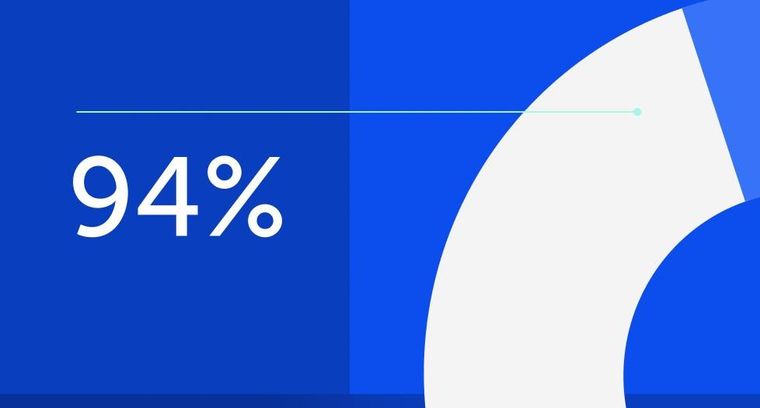
94% of researchers rate our articles as excellent or good
Learn more about the work of our research integrity team to safeguard the quality of each article we publish.
Find out more
CASE REPORT article
Front. Neurol., 18 May 2023
Sec. Neurorehabilitation
Volume 14 - 2023 | https://doi.org/10.3389/fneur.2023.1162149
Hereditary spastic paraplegia (HSP) is a heterogeneous group of inherited neurodegenerative disorders that currently have no cure. HSP type 11 (SPG11-HSP) is a complex form carrying mutations in the SPG11 gene. Neuropathological studies demonstrate that motor deficits in these patients are mainly attributed to axonal degeneration of the corticospinal tract (CST). Repetitive transcranial magnetic stimulation (rTMS) is a non-invasive technique that can induce central nervous system plasticity and promote neurological recovery by modulating the excitability of cortical neuronal cells. Although rTMS is expected to be a therapeutic tool for neurodegenerative diseases, no previous studies have applied rTMS to treat motor symptoms in SPG11-HSP. Here, we report a case of SPG11-HSP with lower extremity spasticity and gait instability, which were improved by applying high-frequency rTMS (HF-rTMS) at the primary motor cortex (M1). Clinical and physiological features were measured throughout the treatment, including the Modified Ashworth Scale (MAS), Berg Balance Scale (BBS), the timed up and go (TUG) test and the 10-meter walk test time (10 MWT). The structure and excitability of the CST were assessed by diffusion tensor imaging (DTI) and transcranial magnetic stimulation (TMS), respectively. After treatment, the patient gained 17 points of BBS, along with a gradual decrease in MAS scores of the bilateral lower extremity. In addition, the TUG test and 10 MWT improved to varying degrees. TMS assessment showed increased motor evoked potential (MEP) amplitude, decreased resting motor threshold (RMT), decreased central motor conduction time (CMCT), and decreased difference in the cortical silent period (CSP) between bilateral hemispheres. Using the DTI technique, we observed increased fractional anisotropy (FA) values and decreased mean diffusivity (MD) and radial diffusivity (RD) values in the CST. It suggests that applying HF-rTMS over the bilateral leg area of M1 (M1-LEG) is beneficial for SPG11-HSP. In this study, we demonstrate the potential of rTMS to promote neurological recovery from both functional and structural perspectives. It may provide a clinical rationale for using rTMS in the rehabilitation of HSP patients.
Hereditary spastic paraplegia (HSP) comprises a rare group of genetically heterogeneous neurological disorders characterized by lower extremity spasticity and slowly progressive abnormal gait (1). The most common neuroanatomical changes occurring in HSP are the loss of axons in the corticospinal tract (CST) and corpus callosum (CC) (2). Hereditary spastic paraplegia type 11 (SPG11-HSP) is a complex form of HSP mainly due to mutations in the SPG11 gene (3, 4). Although a broader range of neuropathological abnormalities has been reported in complicated HSP, it remains generally accepted that degenerative changes in the long axons of the CST are responsible for motor symptoms of the disease (2, 5).
To date, there is no specific treatment to prevent or reverse the neuron degeneration of HSP, nor is there a specific drug to cure it (3). Conventional therapies such as physical therapy, antispasticity medications and orthopedic braces are still mainstream treatments for motor symptoms in patients with all types of HSP (6). Unfortunately, the results are often less than satisfactory (4). Repetitive transcranial magnetic stimulation (rTMS) is a non-invasive neuromodulation technique that delivers repetitive magnetic pulses at specific cortical sites to modulate motor cortical excitability and improve motor performance through corticospinal projections (7, 8). It is expected to be a promising therapeutic tool for neurodegenerative diseases (9). However, no previous studies have applied rTMS to treat motor symptoms in SPG11-HSP.
Here, we report a case of SPG11-HSP with lower extremity spasticity and gait instability, which were improved by applying high-frequency rTMS (HF-rTMS) at the primary motor cortex (M1).
A 21-year-old Chinese female presented to Shanghai No. 3 Rehabilitation Hospital with a history of progressive lower extremity weakness and gait instability for over 6 years. She showed no abnormalities in motor learning or motor performance in early childhood. Only after age 15 did her motor deficits slowly manifest. The initial manifestation was a slight dragging of the left foot when walking with a tendency to fall, followed by weakness in both lower extremities. The patient was initially seen in pediatrics and underwent traditional physical rehabilitation, but her symptoms slowly worsened. When the patient arrived at our hospital, her balance and walking ability were so poor that she could not take care of herself well. Then, we performed a neurological examination. The mental status was normal (Mini-Mental State Examination score = 28). The examination revealed hyperreflexia of the knee, positive bilateral Babinski's sign. She had a spastic gait with marked lower extremities hypertonia, and her bilateral lower extremity muscle strength was level 4/5. She also had somatosensory deficits, evidenced by impairment of the two-point discrimination threshold and absence of vibration in the distal lower extremity.
Magnetic resonance imaging (MRI) of the brain revealed a markedly thin CC, particularly in the genu and anterior portion of the body, best seen on sagittal T1-weighted image (Figure 1A). Axial FLAIR image (Figure 1B) showed the focal thinning in the genu fibers of the CC, which is referred to as the “ears of the lynx” sign and is a highly correlated imaging presentation with SPG11-HSP (10). The genetic testing identified 2 heterozygous mutations on the SPG11 gene. The heterozygous mutations were located on 2 alleles, one from her mother (chr15:44888407, c.4307_4308del, p.Q1436fs) and the other from her father (chr15:44949428, c.733_734del, p.M245fs), constituting a compound heterozygous mutation. Therefore, the patient was diagnosed with SPG11-HSP.
Figure 1. Brain MRI in the patient and a healthy control subject (the same age girl). (A) Sagittal T1-weighted image showing thin corpus callosum (white arrow) in the patient, compared to normal corpus callosum morphology in a control subject. (B) Axial FLAIR image showing typical “ears of the lynx” sign (white arrow). (C) Brain image in the healthy control subject.
After signing an informed consent form, the patient received HF-rTMS treatment. Every session consisted of 40 trains of stimulation, each lasting 6 s and an intertrain interval of 8 s with a frequency of 5 Hz. There were 1,200 pulses in each session. The stimulation intensity was set at 90% of the resting motor threshold (RMT), and the stimulation sites were the bilateral leg area of M1 (M1-LEG). A total of 2,400 pulses (1,200 on the left side and 1,200 on the right side) were applied daily. We used a figure-8-shaped coil connected to the magnetic stimulator (M-100 Ultimate, Yingchi, Shenzhen, China). The location of the coil was determined by the international 10-20 system, with M1-LEG located ~1–2 cm posterior to the vertex (11). The center of the figure-8-shaped coil was placed 1 cm posterior to the vertex and 1–2 cm laterally on the target hemisphere (11). The coil handle was then pointing medially toward the non-target hemisphere to generate a medial-lateral current in M1-LEG (12). We used the medial-lateral coil orientation because it is proven more effective for targeting lower extremity muscles (12). The patient had undergone the treatment 5 days a week for 5 consecutive weeks without adverse events. During the rTMS treatment period, the existing conventional therapies (physical therapy, ankle-foot orthosis) were continuously used.
Clinical measures were conducted at baseline (T0) and weekly for 5 weeks during treatment. The Modified Ashworth Scale (MAS) scores were evaluated in 3 joints of the patient's lower extremity with a 6-point (0, 1, 1+, 2, 3, and 4) scale: ankle (dorsiflexion and plantar flexion), knee (extension and flexion), and hip (abduction and adduction). The scores for each joint were recorded, and the scores of the three joints were then summed to calculate a composite score for each leg. The Berg Balance Scale (BBS) consists of 14 tasks, including sitting, standing, and moving around. For the Timed Up and Go (TUG) test, we asked the patient to stand up from an armchair, walk 3 m, turn around, walk backwards, and sit down to the same chair. For performing the 10-meter Walk Test (10 MWT), the patient was instructed to walk in a straight line wearing shoes. The start, 2 m, 8 m and the end of this route were marked on the floor with brightly colored tape. We recorded only the middle 6 m in order to minimize the effect of acceleration and deceleration, disregarding the initial 2 m and the final 2 m of the path (13). Three consecutive measurements were done for the TUG test and 10 MWT, and the average time (in seconds) required to complete the measurements was calculated.
As shown in Table 1, the MAS scores of both legs gradually decreased, and the BBS scores gradually increased with prolonged treatment. Besides, the TUG test and 10 MWT were reduced to varying degrees after treatment.
Table 1. Comparison of MAS in the bilateral leg, BBS, 10 MWT and TUG test scores of the patient among 5 weeks.
Neurophysiological changes were assessed using single-pulse TMS (11). The patient was comfortably seated in a chair for the assessment. The assessor placed the coil tangentially on the M1 based on the international 10–20 system. Indicators related to the hand area of M1 (M1-HAND) and the M1-LEG were assessed separately. Electromyogram signals were acquired using Ag/AgCl surface electrodes (Kendall Medi-Trace) on the bilateral first dorsal interosseous (FDI) and tibialis anterior (TA) muscles, respectively. RMT was defined as the minimum TMS intensity evoking motor evoked potential (MEP) with amplitudes at least 50 μV in 5 out of 10 consecutive single-pulse stimuli while the target muscle was relaxed (14). RMT values were expressed as a percentage of the maximum stimulator output (%MSO). We consecutively evoked MEPs with 120% RMT and calculated the average latency values and peak-to-peak values of 10 MEPs. Thereafter, the peripheral motor latency from the 7th cervical magnetic stimulation was subtracted from the MEP cortical latency to obtain the central motor conduction time (CMCT) (15). Separately, we instructed the patient to maintain a tonic contraction of 20% maximal voluntary contraction of the FDI and measured cortical silent period (CSP) by stimulating the contralateral M1-HAND at 120% RMT intensity.
We evaluated the patient twice to compare the outcomes before and after HF-rTMS treatment. Table 2 shows an increase in MEP amplitude and a decrease in RMT in the upper and lower extremities compared to pre-treatment, a slight reduction in CMCT in the upper extremities, and a significant decline in the difference in CSP between the left and right hemispheres.
DTI data were collected using a 3-Tesla MRI scanner (SIEMENS VERIO, Erlangen, Germany) with an 8-channel head coil. To better understand the imaging changes, a healthy female volunteer of the same age was recruited as a control. The patient was scanned twice (pre-treatment and post-treatment) and the healthy control subject was only scanned once. The same DTI acquisition parameters were used for the patient and the healthy control subject as follows: acquisition matrix size = 128 × 128, field of view (FOV) = 240 mm × 240 mm, repetition time (TR) = 10,000 ms, echo time (TE) = 89 ms, flip angle = 90°, direction = 62, b = 0, 1,000 s/mm2, slice thickness = 2 mm and slice gap = 0.
All DTI data were preprocessed using FSL software (http://www.fmrib.ox.ac.uk/fsl). Then, whole-brain deterministic fiber tracking was processed based on fiber assignment by continuous tracking (FACT) algorithm and performed using Diffusion Toolkit and TrackVis (http://www.trackvis.org). The white matter fiber tracking was terminated when the maximum turning angle > 45° or fractional anisotropy (FA) < 0.2. After the whole-brain fiber tracts were obtained, the CST were traced with the fiber tracts passing through cerebral peduncles, the posterior extremity of the internal capsule and M1 as the regions of interest (ROIs) were obtained by manual segmentation. Finally, the mean value of FA, mean diffusivity (MD) and radial diffusivity (RD) were extracted from each CST. Besides, region-based analysis of the CC was applied using atlas-based predefined brain regions on the basis of the John Hopkins University (JHU) white matter tractography atlas (16).
As shown in Figure 2, DTI revealed thinning of the CSTs in bilateral hemispheres compared to those of a healthy control subject. The results showed a marked reduction of FA values and increases in MD and RD values in the CSTs, which indicated a loss of integrity and density of the CSTs. We also found a drastically reduced FA values and increased MD and RD values throughout the CC of the patient, following the observed extreme thinning of the CC areas in the brain MRI. Fortunately, the patient had increased FA values and decreased MD and RD values in the CST and CC after treatment. A detailed description of the FA, MD and RD values from CC and CST of the patient (pre-treatment and post-treatment) and the healthy subject, and the difference between pre-treatment vs. post-treatment and the difference between patient vs. healthy control as a percentage change in FA, MD and RD are provided in Supplementary material.
Figure 2. (A) The CSTs of the patient (pre-treatment and post-treatment) and a healthy subject (the same age girl). White arrows are used to highlight the differences. The DTI revealed thinning of the CSTs in bilateral hemispheres compared to those of a healthy control subject. (B) The FA values from CC and CST of the patient (pre-treatment and post-treatment) and the healthy subject. (C) The MD values from CC and CST of the patient (pre-treatment and post-treatment) and the healthy subject. (D) The RD values from CC and CST of the patient (pre-treatment and post-treatment) and the healthy subject. CC, corpus callosum; CST, corticospinal tract; FA, fractional anisotropy (10−3 mm2/s); MD, mean diffusivity (10−3 mm2/s); RD, radial diffusivity (10−3 mm2/s); R, right; L, left.
To our knowledge, this is the first report of HF-rTMS for SPG11-HSP and the first report of a Chinese patient. Before rTMS treatment, the patient received conventional therapies including physical therapy to stretch the spastic muscles and an ankle-foot orthosis to prevent foot drop. However, the patient's walking ability did not improve. In addition, the patient refused to take oral antispasticity medication due to the inability to tolerate the side effects. Therefore, rTMS, an approach targeting central nervous system modulation, played a crucial role in improving the symptoms. In this study, we revealed the recovery of CST and improvement of motor function after HF-rTMS treatment using TMS and DTI assessment. This disease is rare and incurable, and our findings have important implications for rehabilitation programs to treat SPG11-HSP.
The patient showed significant improvement in spasticity, according to MAS. In the lower extremity, the pathophysiology of spasticity is the reduction of reciprocal inhibition that plays a major role (17, 18). Previous studies demonstrated that the reduction of this spinal inhibitory mechanism is involved in the occurrence of spasticity in HSP patients (19, 20). Spinal cord stimulation can improve the spasticity of the lower extremity in patients with HSP (21). Another study found that rTMS enhances descending projections between the motor cortex and spinal inhibitory circuits, which contributes to the reduction of lower extremity spasticity (22). Perez et al. also demonstrated that rTMS at 5 Hz over M1-LEG could modulate transmission in specific spinal cord circuits through changes in the corticospinal drive (23). Therefore, we theorize that cortical and spinal cord neural remodeling associated with relief of spasticity has occurred in our patient, facilitated by rTMS.
In addition, our patient showed promising improvements in balance and walking ability according to BBS, TUG test and 10 MWT. It is not only due to reduced lower extremity spasticity but also associated with improved postural control. Behaviorally, the TUG test improves more significantly than 10 MWT. As we know, 10 MWT reflects walking speed, while the TUG test emphasizes postural control. Postural control and walking are generally considered voluntary motor functions mainly controlled by subcortical and spinal cord regions (24). However, it has been found that the motor cortex also participates in some aspects of postural control (25). The M1 plays a key role in motor control, including controlling body posture and planning and execution of movements (26). Previous study suggested that delayed postural responses and reduced MEP amplitude of the legs are responsible for balance impairments in HSP patients, and the mechanism may be diminished corticospinal drive onto spinal interneurons (27). Therefore, we believe that HF-rTMS may provide good modulation of postural control by enhancing corticospinal drive.
Other studies revealed that the ongoing modulation of corticospinal excitability and intracortical inhibition within M1 is critical for successful restraint and cancellation of actions (28, 29). In addition to activating corticospinal neurons, rTMS activates intracortical inhibitory and excitatory neural circuits in the M1 (30). GABAergic inhibitory brain circuits are important to motor control (31). TMS-invoked CSP is an index of GABAB-mediated intracortical inhibition within M1 (32), and the duration of CSP reflects individual inhibitory control capacities (33). Because of substantial interindividual variability, the relative right-to-left difference in CSP is generally considered clinically significant (11). Our patient had a significantly lower duration of CSP in the right hemisphere than in the left before treatment. It suggested that the inhibitory interneurons in the right hemisphere are more damaged, which is consistent with a high RMT in the right hemisphere. Therefore, the patient also presented clinically with weaker control in the left leg than in the right leg. After treatment, the improvement in postural control was accompanied by a significant reduction in CSP differences between the left and right hemispheres. It showed that the corticospinal excitatory and inhibitory regulation tends to be balanced, which may also be one of the favorable factors for the recovery of her balance and walking ability. However, the details of the corticospinal inhibition mechanisms affecting motor inhibition function cannot be determined by examining the silent period alone. Movement coordination relies on both the activation of each hemisphere and the communication between hemispheres mediated via the CC. Furthermore, the mechanism of walking is complex. In addition to central control, factors such as sensory and spatial cognitive function can also impact walking. The underlying mechanisms require further exploration.
The coordinated control of the upper and lower extremity is an important factor in adjusting posture and maintaining human balance during walking (34, 35). We considered that the function of the upper extremity also affects the recovery of walking ability. Thus, in addition to the neurophysiological assessment of the lower extremity, we also assessed the upper extremity. After treatment, the patient had an increased MEP amplitude and a decreased RMT for both upper and lower extremities. The decreased RMT reflects increased excitability of the most sensitive group of neurons in the stimulated area in M1 (36). The amplitude of MEP represents the global excitability of cortical interneurons, corticospinal neurons and spinal motoneurons (37). After HF-rTMS treatment, the neuronal excitability of M1-LEG increased, reaching the target muscle via the conduction pathway and facilitating the recovery of function in the patient's lower extremities. Meanwhile, the MEP amplitude measured through the FDI muscles of the upper extremity also increased, even though the patient did not exhibit signs of upper extremity dysfunction. It indicates that the motor neurons and motor pathways associated with the upper extremity muscles were activated, which may make the upper extremity motions more flexible and indirectly create favorable conditions for improving the patient's walking stability. Previous studies suggested that the activity motor cortical areas might improve gait by inducing activity modulation of spared descending motor pathways (38, 39). Furthermore, a slight decrease in CMCT in the upper extremities compared to the pre-treatment indicates an increase in the conduction velocity of upper motor neurons, which may reflect improved axonal performance in the fast-conducting fibers of corticospinal neurons (40). The above results indicate that the patient's motor cortex and the motor conduction pathways were activated bilaterally after a series of treatments, providing a basis for functional remodeling of the nervous system.
Moreover, we used the DTI metrics to analyze the microstructural alterations in the CSTs before and after treatment, including FA, RD and MD, which can characterize axonal injury and neuronal fiber myelination by quantifying white matter damage (41). A previous study has found that patients with HSP have lower FA values and higher RD values in the CST compared to healthy subjects (42), which is consistent with our results. It indicated the impaired structural integrity of the CST in the patient with axonal damage and neuronal fibers demyelination. However, we are pleased to observe an increase in FA values and a decrease in MD and RD values in the CST at the patient's second DTI scan (i.e., after treatment). Additionally, these indicators were similarly altered in the genu, body and splenium parts of the CC. A study confirmed that HF-rTMS promotes motor recovery while improving diffusion microstructures in motor-related white matter and gray matter brain regions, as evidenced by a significant increase in FA values of the CST (43). So, the changes in DTI metrics demonstrated that the restoration of white matter fiber connections occurred in the motor conduction pathways, providing a basis for the patient's functional recovery.
There are some limitations in generalizing the effects of rTMS based on this study. Since it is a case report, we can't completely rule out that other factors affected the results. Additionally, it is a qualitative report with no statistical analysis. In addition, the whole-brain effects induced by HF-rTMS should be considered in the future. Besides, there was a lack of follow-up in this case. Due to the health crisis of COVID-19, the patient did not return for a follow-up evaluation. We conducted a telephone interview after 4 months of treatment and learned that the patient's ambulation was maintained for 1–2 months after discharge and then tended to decline. Therefore, the long-term beneficial effects of HF-rTMS treatment could not be determined, and this should be investigated in depth in future studies.
In summary, this case report offers a new rehabilitation prescription. We demonstrate the potential of this strategy to promote motor nervous system recovery in SPG11-HSP patients from a functional and structural perspective. We expect researchers can provide additional clinical evidence of the benefits of HF-rTMS applied to the M1-LEG to improve walking ability and reduce spasticity in patients with HSP.
The original contributions presented in the study are included in the article/Supplementary material, further inquiries can be directed to the corresponding authors.
Written informed consent was obtained from the individual(s) for the publication of any potentially identifiable images or data included in this article.
SC and ZZ designed and conceptualized the study, treated the patient drafted, and finalized the manuscript. CS and WL supervised the progression and revised the manuscript. XC and SZ assisted in obtaining the TMS assessment. MR collected and sorted out the related materials. SX helped with DTI data processing. XS and XiaZ analyzed and interpreted the data. XinZ was responsible for the physical therapy. All authors read and approved the submitted version.
This work was supported by National Key Research and Development Program of China (Grant No. 2018YFC2001600/04), Shanghai Jing'an District Health Research Project (Grant No. 2021MS19), and Key Subjects Construction Program of the Health System in Jing'an District (Grant No. 2021PY04).
We gratefully thank the patient and her family for their participation. We also thank Yueyang Hospital of Integrated Traditional Chinese and Western Medicine, Shanghai University of Chinese Traditional Medicine for providing MRI equipment support.
The authors declare that the research was conducted in the absence of any commercial or financial relationships that could be construed as a potential conflict of interest.
All claims expressed in this article are solely those of the authors and do not necessarily represent those of their affiliated organizations, or those of the publisher, the editors and the reviewers. Any product that may be evaluated in this article, or claim that may be made by its manufacturer, is not guaranteed or endorsed by the publisher.
The Supplementary Material for this article can be found online at: https://www.frontiersin.org/articles/10.3389/fneur.2023.1162149/full#supplementary-material
1. de Souza P, de Rezende PW, de Rezende BG, Bortholin T, Oliveira A. Hereditary spastic paraplegia: clinical and genetic hallmarks. Cerebellum. (2017) 16:525–51. doi: 10.1007/s12311-016-0803-z
2. Mackay-Sim A. Hereditary spastic paraplegia: from genes, cells and networks to novel pathways for drug discovery. Brain Sci. (2021) 11:403. doi: 10.3390/brainsci11030403
3. Klebe S, Stevanin G, Depienne C. Clinical and genetic heterogeneity in hereditary spastic paraplegias: from SPG1 to SPG72 and still counting. Rev Neurol. (2015) 171:505–30. doi: 10.1016/j.neurol.2015.02.017
4. Murala S, Nagarajan E, Bollu PC. Hereditary spastic paraplegia. Neurol Sci. (2021) 42:883–94. doi: 10.1007/s10072-020-04981-7
5. Fowler PC, Garcia-Pardo ME, Simpson JC, O'Sullivan NC. Neurodegeneration: the central role for ER contacts in neuronal function and axonopathy, lessons from hereditary spastic paraplegias and related diseases. Front Neurosci. (2019) 13:1051. doi: 10.3389/fnins.2019.01051
6. Shribman S, Reid E, Crosby AH, Houlden H, Warner TT. Hereditary spastic paraplegia: from diagnosis to emerging therapeutic approaches. Lancet Neurol. (2019) 18:1136–46. doi: 10.1016/S1474-4422(19)30235-2
7. Bunday KL, Perez MA. Motor recovery after spinal cord injury enhanced by strengthening corticospinal synaptic transmission. Curr Biol. (2012) 22:2355–61. doi: 10.1016/j.cub.2012.10.046
8. Kumru H, Murillo N, Samso JV, Valls-Sole J, Edwards D, Pelayo R, et al. Reduction of spasticity with repetitive transcranial magnetic stimulation in patients with spinal cord injury. Neurorehabil Neural Repair. (2010) 24:435–41. doi: 10.1177/1545968309356095
9. Song C, Fang S, Lv G, Mei X. Gastrodin promotes the secretion of brain-derived neurotrophic factor in the injured spinal cord. Neural Regen Res. (2013) 8:1383–9.
10. Romagnoli ER, Akly MP, Miquelini LA, Funes JA, Besada CH. Hereditary spastic paraplegia: an “ears of the lynx” magnetic resonance imaging sign in a patient with recessive genetic type 11. Neuroradiol J. (2021) 34:42–4. doi: 10.1177/1971400920953820
11. Groppa S, Oliviero A, Eisen A, Quartarone A, Cohen LG, Mall V, et al. A practical guide to diagnostic transcranial magnetic stimulation: report of an IFCN committee. Clin Neurophysiol. (2012) 123:858–82. doi: 10.1016/j.clinph.2012.01.010
12. Smith MC, Stinear JW, Alan BP, Stinear CM. Effects of non-target leg activation, TMS coil orientation, and limb dominance on lower limb motor cortex excitability. Brain Res. (2017) 1655:10–6. doi: 10.1016/j.brainres.2016.11.004
13. de Baptista C, Vicente AM, Souza MA, Cardoso J, Ramalho VM, Mattiello-Sverzut AC. Methods of 10-meter walk test and repercussions for reliability obtained in typically developing children. Rehabil Res Pract. (2020) 2020:4209812. doi: 10.1155/2020/4209812
14. Rossini PM, Barker AT, Berardelli A, Caramia MD, Caruso G, Cracco RQ, et al. Non-invasive electrical and magnetic stimulation of the brain, spinal cord and roots: basic principles and procedures for routine clinical application. Report of an IFCN committee. Electroencephalogr Clin Neurophysiol. (1994) 91:79–92. doi: 10.1016/0013-4694(94)90029-9
15. Ugawa Y, Uesaka Y, Terao Y, Hanajima R, Kanazawa I. Magnetic stimulation of corticospinal pathways at the foramen magnum level in humans. Ann Neurol. (1994) 36:618–24. doi: 10.1002/ana.410360410
16. Hua K, Zhang J, Wakana S, Jiang H, Li X, Reich DS, et al. Tract probability maps in stereotaxic spaces: analyses of white matter anatomy and tract-specific quantification. Neuroimage. (2008) 39:336–47. doi: 10.1016/j.neuroimage.2007.07.053
17. Crone C, Nielsen J, Petersen N, Ballegaard M, Hultborn H. Disynaptic reciprocal inhibition of ankle extensors in spastic patients. Brain. (1994) 177:1161–8. doi: 10.1093/brain/117.5.1161
18. Mori F, Koch G, Foti C, Bernardi G, Centonze D. The use of repetitive transcranial magnetic stimulation (rTMS) for the treatment of spasticity. Prog Brain Res. (2009) 175:429. doi: 10.1016/S0079-6123(09)17528-3
19. Crone C, Petersen NT, Nielsen JE, Hansen NL, Nielsen JB. Reciprocal inhibition and corticospinal transmission in the arm and leg in patients with autosomal dominant pure spastic paraparesis (ADPSP). Brain. (2004) 127:2693–702. doi: 10.1093/brain/awh319
20. Mailis A, Ashby P. Alterations in group Ia projections to motoneurons following spinal lesions in humans. J Neurophysiol. (1990) 64:637–47. doi: 10.1152/jn.1990.64.2.637
21. Ardolino G, Bocci T, Nigro M, Vergari M, Di Fonzo A, Bonato S, et al. Spinal direct current stimulation (tsDSC) in hereditary spastic paraplegias (HSP): a sham-controlled crossover study. J Spinal Cord Med. (2021) 44:46–53. doi: 10.1080/10790268.2018.1543926
22. Nardone R, Holler Y, Thomschewski A, Brigo F, Orioli A, Holler P, et al. rTMS modulates reciprocal inhibition in patients with traumatic spinal cord injury. Spinal Cord. (2014) 52:831–5. doi: 10.1038/sc.2014.136
23. Perez MA, Lungholt BK, Nielsen JB. Short-term adaptations in spinal cord circuits evoked by repetitive transcranial magnetic stimulation: possible underlying mechanisms. Exp Brain Res. (2005) 162:202–12. doi: 10.1007/s00221-004-2144-2
24. Whelan PJ. The involvement of the motor cortex in postural control: a delicate balancing act. J Physiol. (2009) 587:3753. doi: 10.1113/jphysiol.2009.176750
25. Beauchet O, Annweiler C, Montero-Odasso M, Fantino B, Herrmann FR, Allali G. Gait control: a specific subdomain of executive function? J Neuroeng Rehabil. (2012) 9:12. doi: 10.1186/1743-0003-9-12
26. Rodriguez-Sabate C, Rodriguez M, Morales I. Studying the functional connectivity of the primary motor cortex with the binarized cross recurrence plot: the influence of Parkinson's disease. PLoS ONE. (2021) 16:e252565. doi: 10.1371/journal.pone.0252565
27. Nonnekes J, de Niet M, Oude NL, de Bot ST, van de Warrenburg BP, Bloem BR, et al. Mechanisms of postural instability in hereditary spastic paraplegia. J Neurol. (2013) 260:2387–95. doi: 10.1007/s00415-013-7002-3
28. Duque J, Greenhouse I, Labruna L, Ivry RB. Physiological markers of motor inhibition during human behavior. Trends Neurosci. (2017) 40:219–36. doi: 10.1016/j.tins.2017.02.006
29. van den Wildenberg WP, Burle B, Vidal F, van der Molen MW, Ridderinkhof KR, Hasbroucq T. Mechanisms and dynamics of cortical motor inhibition in the stop-signal paradigm: a TMS study. J Cogn Neurosci. (2010) 22:225–39. doi: 10.1162/jocn.2009.21248
30. Cash RF, Isayama R, Gunraj CA, Ni Z, Chen R. The influence of sensory afferent input on local motor cortical excitatory circuitry in humans. J Physiol. (2015) 593:1667–84. doi: 10.1113/jphysiol.2014.286245
31. Ding Q, Triggs WJ, Kamath SM, Patten C. Short intracortical inhibition during voluntary movement reveals persistent impairment post-stroke. Front Neurol. (2018) 9:1105. doi: 10.3389/fneur.2018.01105
32. Cardellicchio P, Dolfini E, Hilt PM, Fadiga L, D'Ausilio A. Motor cortical inhibition during concurrent action execution and action observation. Neuroimage. (2020) 208:116445. doi: 10.1016/j.neuroimage.2019.116445
33. Paci M, Di Cosmo G, Perrucci MG, Ferri F, Costantini M. Cortical silent period reflects individual differences in action stopping performance. Sci Rep. (2021) 11:15158. doi: 10.1038/s41598-021-94494-w
34. Cheron G, Duvinage M, De Saedeleer C, Castermans T, Bengoetxea A, Petieau M, et al. From spinal central pattern generators to cortical network: integrated BCI for walking rehabilitation. Neural Plast. (2012) 2012:375148. doi: 10.1155/2012/375148
35. Arya KN, Pandian S. Interlimb neural coupling: implications for poststroke hemiparesis. Ann Phys Rehabil Med. (2014) 57:696–713. doi: 10.1016/j.rehab.2014.06.003
36. Kim KM, Kim JS, Cruz-Diaz D, Ryu S, Kang M, Taube W. Changes in spinal and corticospinal excitability in patients with chronic ankle instability: a systematic review with meta-analysis. J Clin Med. (2019) 8:1037. doi: 10.3390/jcm8071037
37. Zhou R, Alvarado L, Kim S, Chong SL, Mushahwar VK. Modulation of corticospinal input to the legs by arm and leg cycling in people with incomplete spinal cord injury. J Neurophysiol. (2017) 118:2507–19. doi: 10.1152/jn.00663.2016
38. Pinto DSC, Coelho DB, Campos D, Dos SGM, de Oliveira VE, Gonzalez-Salazar C, et al. Spinal cord stimulation improves motor function and gait in spastic paraplegia type 4 (SPG4): clinical and neurophysiological evaluation. Parkinsonism Relat Disord. (2021) 83:1–5. doi: 10.1016/j.parkreldis.2020.12.008
39. Tufo T, Ciavarro M, Di Giuda D, Piccininni C, Piano C, Daniele A. Spinal cord stimulation may improve gait and cognition in hereditary spastic paraplegia with mental retardation: a case report. Neurol Sci. (2023) 44:961–6. doi: 10.1007/s10072-022-06487-w
40. Siow SF, Cameron SR, Ng K, Kumar KR, Sue CM. Motor evoked potentials in hereditary spastic paraplegia-a systematic review. Front Neurol. (2019) 10:967. doi: 10.3389/fneur.2019.00967
41. List J, Kohl Z, Winkler J, Marxreiter F, Doerfler A, Schmidt MA. Ascending axonal degeneration of the corticospinal tract in pure hereditary spastic paraplegia: a cross-sectional DTI study. Brain Sci. (2019) 9:268. doi: 10.3390/brainsci9100268
42. Aghakhanyan G, Martinuzzi A, Frijia F, Vavla M, Hlavata H, Baratto A, et al. Brain white matter involvement in hereditary spastic paraplegias: analysis with multiple diffusion tensor indices. Am J Neuroradiol. (2014) 35:1533–8. doi: 10.3174/ajnr.A3897
43. Guo Z, Jin Y, Peng H, Xing G, Liao X, Wang Y, et al. Ipsilesional high frequency repetitive transcranial magnetic stimulation add-on therapy improved diffusion parameters of stroke patients with motor dysfunction: a preliminary DTI study. Neural Plast. (2016) 2016:6238575. doi: 10.1155/2016/6238575
Keywords: hereditary spastic paraplegia, repetitive transcranial magnetic stimulation, diffusion tensor imaging, corticospinal tract, lower extremity spasticity, walking ability
Citation: Chen S, Zhou Z, Ren M, Chen X, Shi X, Zhang S, Xu S, Zhang X, Zhang X, Lin W and Shan C (2023) Case report: High-frequency repetitive transcranial magnetic stimulation for treatment of hereditary spastic paraplegia type 11. Front. Neurol. 14:1162149. doi: 10.3389/fneur.2023.1162149
Received: 09 February 2023; Accepted: 02 May 2023;
Published: 18 May 2023.
Edited by:
Giorgio Sandrini, Fondazione Cirna Onlus, ItalyReviewed by:
Marco Ciavarro, Mediterranean Neurological Institute Neuromed (IRCCS), ItalyCopyright © 2023 Chen, Zhou, Ren, Chen, Shi, Zhang, Xu, Zhang, Zhang, Lin and Shan. This is an open-access article distributed under the terms of the Creative Commons Attribution License (CC BY). The use, distribution or reproduction in other forums is permitted, provided the original author(s) and the copyright owner(s) are credited and that the original publication in this journal is cited, in accordance with accepted academic practice. No use, distribution or reproduction is permitted which does not comply with these terms.
*Correspondence: Chunlei Shan, c2hhbmNsaGFwcHlAMTYzLmNvbQ==; Wanlong Lin, MTMxNjI2MzgxNjVAMTYzLmNvbQ==
†These authors have contributed equally to this work
Disclaimer: All claims expressed in this article are solely those of the authors and do not necessarily represent those of their affiliated organizations, or those of the publisher, the editors and the reviewers. Any product that may be evaluated in this article or claim that may be made by its manufacturer is not guaranteed or endorsed by the publisher.
Research integrity at Frontiers
Learn more about the work of our research integrity team to safeguard the quality of each article we publish.