- 1Department of Neurological Surgery, UC Davis Medical Center, Sacramento, CA, United States
- 2Department of Neurology, UC Davis Medical Center, Sacramento, CA, United States
- 3Department of Neurology, Virginia Commonwealth University, Richmond, VA, United States
Given the complexity of cerebral pathology in patients with acute brain injury, various neuromonitoring strategies have been developed to better appreciate physiologic relationships and potentially harmful derangements. There is ample evidence that bundling several neuromonitoring devices, termed “multimodal monitoring,” is more beneficial compared to monitoring individual parameters as each may capture different and complementary aspects of cerebral physiology to provide a comprehensive picture that can help guide management. Furthermore, each modality has specific strengths and limitations that depend largely on spatiotemporal characteristics and complexity of the signal acquired. In this review we focus on the common clinical neuromonitoring techniques including intracranial pressure, brain tissue oxygenation, transcranial doppler and near-infrared spectroscopy with a focus on how each modality can also provide useful information about cerebral autoregulation capacity. Finally, we discuss the current evidence in using these modalities to support clinical decision making as well as potential insights into the future of advanced cerebral homeostatic assessments including neurovascular coupling.
Introduction
In patients with acute brain injury (ABI), there is a diverse and heterogenous range of pathologic processes that can often lead to irreversible neurologic insult. Following the primary injury, a cascade of maladaptive and deleterious physiologic processes can ensue in the form of edema, seizures, spreading cortical depolarization, metabolic failure, neuro-inflammation as well as impaired cerebrovascular reactivity, leading to cerebral injury and subsequent cellular death (1, 2). Prevention of secondary brain injury therefore is of paramount importance in neurocritical care with the goal of optimizing conditions to maximize the potential for recovery. Traditionally, management decisions have been guided by neurologic examination and neuroimaging; and while both provide invaluable clinical insights, in isolation, these approaches do not provide an understanding of the ongoing dynamic pathobiological processes, which when monitored can guide medical intervention in real time. As such, there has been an increased focus over the past few decades on the development and utilization of neuromonitoring techniques that allow for enhanced surveillance of cerebral physiologic parameters in order to detect early signs of secondary brain injury and allow for goal directed interventions to stave off irreversible damage (3).
Though advances in neuromonitoring represent a major breakthrough in the field of neurocritical care and provide new insights in the complexity of ABI pathophysiology, a single neuromonitoring device is insufficient in providing a comprehensive scope of the intricate and dynamic nature of impaired cerebral physiology (4, 5). Subsequently focus has shifted to bundling complementary neuromonitoring techniques, termed multimodality monitoring (MMM), to enhance the predictive value of the physiologic outputs and allow for individualized patient management decisions (4, 6). One of the advantages of the expanded use of MMM is the ability to characterize cerebral autoregulation (CA) capacity, which has been validated as an important prognostic indicator and may be useful to guide hemodynamic decisions, with the goal of optimizing cerebral perfusion (7, 8).
This multifaceted approach has begun to reshape the landscape of neurocritical care by moving away from standardized “one size fits all” treatment strategies to individualized precision medicine; however, questions still exist including how to best integrate and interpret the high dimensionality of signals and whether such an approach will result in improved patient outcomes (3, 9). Furthermore, when interpreting monitoring data, it is important to recognize potential limitations of each modality and specific characteristics including whether it provides continuous or intermittent assessment as well as if the device is measuring focal or global cerebral parameters (10, 11). Common neuromonitoring tools employed in the neurologic intensive care unit (ICU) are highlighted in Figure 1. This review will provide the most recent update on MMM with a focus on invasive and non-invasive monitoring strategies that can be used to simultaneously provide CA assessment. Modalities that do not possess capabilities for CA evaluation, such as quantitative electroencephalography (qEEG) and cerebral microdialysis, will not be covered.
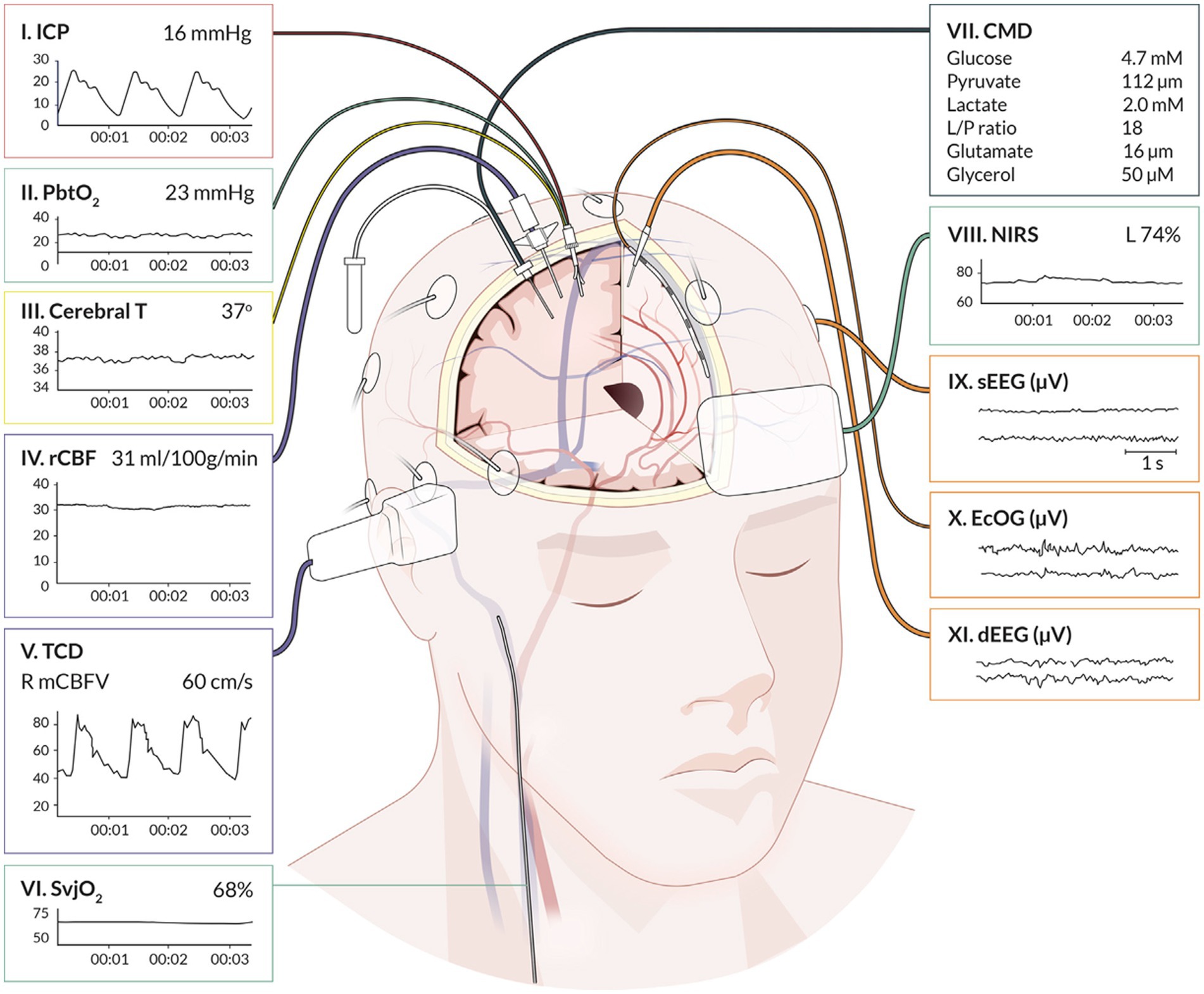
Figure 1. Graphical representation of cerebral multimodality monitoring modalities. Cerebral T, cerebral temperature; CMD, cerebral microdialysis; dEEG, depth electroencephalography; ECoG, electrocorticography; ICP, intracranial pressure; NIRS, near-infrared spectroscopy; PbtO2, partial pressure of brain tissue oxygenation; rCBF, regional cerebral blood flow; sEEG, surface electroencephalography; SvjO2, jugular bulb venous oximetry; TCD, transcranial Doppler. Reprinted with permission from open access publication corresponding author, Tas and colleagues (4). Professional illustration by Anna Sieben (Sieben Medical Art).
Cerebral physiology
Advances in neuromonitoring capabilities has allowed for enhanced understanding of complex cerebral physiologic relationships, both in normal homeostasis and with harmful derangements, with the potential to drive clinical management decisions. An understanding of major cerebral physiologic concepts is crucial for proper interpretation of MMM outputs and informed decision making for cerebral optimization.
Pressure-volume relationship
As the brain resides within the rigid and inflexible cranial vault, alterations in total volume result in corresponding changes in intracranial pressure (ICP). As described in the Monroe-Kellie doctrine, an increase in any of the components of the intracranial system, namely brain, blood and cerebrospinal fluid (CSF), must be accompanied by an equivalent volumetric reduction in another constituent to maintain homeostasis (12). Disproportionate increases in volume (such as cases of hematoma formation or acute hydrocephalus) are rapidly met with exhausted compliance and the pressure-volume relationship becomes non-linear where small alterations in volume induce large changes in ICP (12–14).
Examination of the ICP waveform can provide valuable insights into intracranial compliance and risk for deterioration (15, 16). In the absence of significant pathology, the ICP waveform has three notches corresponding to the systolic (P1), tidal (P2), and dicrotic waves (P3) and are of decreasing amplitude (16). As intracranial compliance decreases, P2 and P3 begin to exceed P1 and eventually P3 disappears leaving a sinusoidal morphology (12, 16). Elevation of P2 can predict risk for subsequent ICP crisis and during periods of severely elevated ICP there is often a reduction in ICP waveform complexity (15, 17).
Vasomotor reactivity
Under normal conditions, the brain requires a constant cerebral blood flow (CBF) of ~50–60 mL/100 g/min to maintain normal metabolic and physiologic conditions (18). Regulation of CBF involves a complex interplay between various cellular signaling pathways that act to modulate vascular tone to ensure optimal perfusion and homeostasis. The cerebrovasculature is particularly sensitive to changes in arterial blood carbon dioxide (PaCO2), pH, and to a lesser extent oxygen, through a process termed vasomotor reactivity (VMR) (19). PaCO2 acts as a fundamental regulator of CBF, with an ~3% increase in CBF for every 1 mmHg increase in PaCO2, through modulation of arteriolar tone by nitric oxide production and alterations in intracellular smooth muscle hydrogen and calcium ion concentrations (20, 21). Avoiding hypercarbia is particularly important for patients with reduced intracranial compliance and elevated ICP due to the potential for increase total cerebral blood volume (CBV) resulting from vasodilation (22). Excessive hyperventilation on the other hand, can dramatically reduce ICP through hypocapnic induced vasoconstriction, however may result in cerebral ischemia and secondary brain injury from neuronal ecotoxicity and diminished CBF (23). While not as potent a modulator of vascular tone under normal physiologic conditions as PaCO2, CBF is also impacted by changes in arterial blood oxygen (PaO2) with vasodilation occurring when arterial tension falls below 50 mmHg to protect against cerebral hypoxia (24).
Cerebral autoregulation
Autoregulatory status has gained recognition as a crucial protective homeostatic mechanism and an important determinant of mortality and functional outcome in patients with diffuse brain injury such as severe traumatic brain injury (TBI) and subarachnoid hemorrhage (SAH) (8, 25–28). First described in humans by Lassen and colleagues in 1959, CA describes the capacity of the cerebral pre-capillary arterioles to relax and constrict to changes in transmural pressure in order to ensure a constant cerebral blood flow (CBF) over a range of mean arterial pressures (MAP) (29). More recent investigations have demonstrated that the range of MAP during which CA remains intact is narrower than previously thought, even in otherwise healthy individuals, and is influenced by the rate of arterial pressure changes (Figure 2) (30). In the setting of ABI, the CA plateau may be further restricted, shifted or all together exhausted thereby predisposing patients to periods of ischemia or conversely hyperemia whenever MAP falls outside the lower and upper limits of autoregulation, respectively (31). In such patients cerebral ischemia may occur under ranges of MAP typically considered normal, thus highlighting the potential significance of individualized assessment and optimization (26, 31, 32).
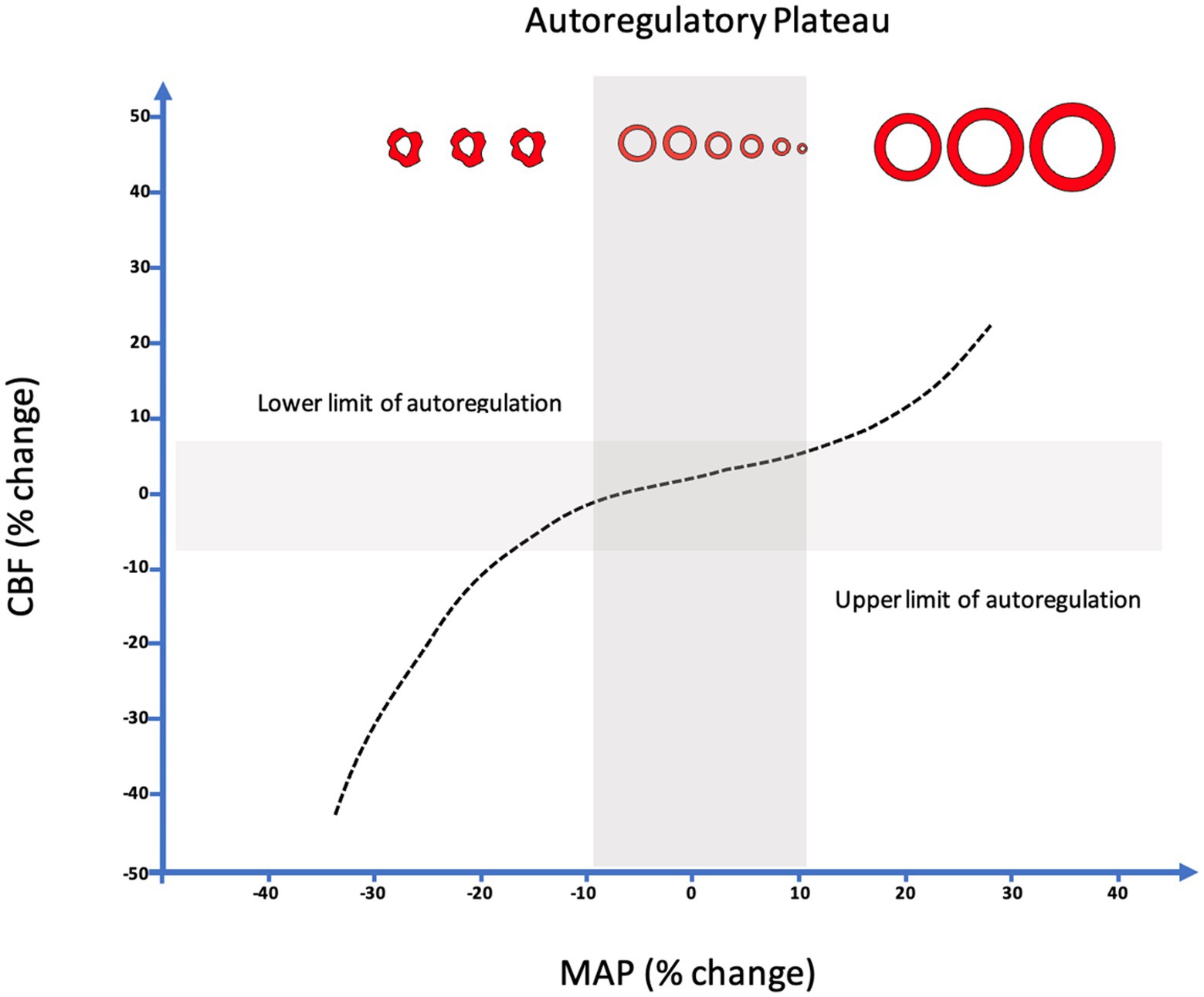
Figure 2. Relationship between changes in MAP from baseline (Δ%MAP) and concomitant relative changes in CBF (Δ%CBF). Upper and lower limits of autoregulation demonstrated by linear correlation between MAP and CBF indicative of pressure passive state. NB. The classic Lassen’s curve demonstrating a wide range of autoregulation (~50–150 mm hg) has been challenged. It is suggested that a narrow range of autoregulation (~15–200 mm hg) is likely to be more common, even in healthy individuals, and is influenced by the rate of arterial pressure changes.
Early studies evaluating CA capacity in ABI focused on intermittent methods for CA determination including various neuroimaging modalities such as positron emission tomography (PET) and computed tomographic xenon (XE-CT) as well as indirect CBF estimation with transcranial doppler (TCD) in response to alterations in MAP either with vasopressors or non-invasively with techniques such as thigh-cuff deflation and orthostatic hypotension provocation (10, 33). More recently, novel methods have been developed for continuous assessment of CA indices based on CBF responses to spontaneous changes in MAP or cerebral perfusion pressure CPP and are outlined in Table 1 (44). These advances have enhanced the clinical applicability of CA and provided a foundation to develop monitoring and treatment protocols based off CA capacity (45). While there is of yet no primary data from large scale randomized controlled trials to support the use of CA monitoring, growing evidence from retrospective and prospective analysis suggests that it may provide crucial insights into the complexities of deranged cerebral physiology inherent in ABI patients and serve as a vital tool to drive clinical management decisions (26, 46–48). This is the topic of several ongoing clinical investigations that will hopefully provide further evidence of how continuous CA assessment may be used to drive patient care decisions (NCT03987139, NCT05670028, NCT02351518).
Neurovascular coupling
While there is mounting evidence regarding the integral role of CA, both as a prognostic marker and foundation for individually targeted management, the brain possesses other homeostatic mechanisms of equal or potentially greater importance for cerebral reserve and guarding against irreversible injury (49). The brain is a highly metabolic organ, utilizing more than 20% of bodily oxygen and glucose despite comprising only 2% of body weight, and has limited capacity for intracellular energy storage thus rendering it dependent on a continuous and tightly regulated blood supply to regions with higher metabolic demand (50, 51). The governing mechanism regulating CBF in response to neuronal activity is termed neurovascular coupling (NVC) and is crucial for ensuring precise cerebral metabolic demands are met (52). NVC has been studied in a variety of conditions with evidence of impairment in chronic conditions such as hypertension, atrial fibrillation and Alzheimer’s Disease leading to blunted NVC response and oxidative stress (49, 53). Impaired NVC may also play a role in the development of delayed cerebral ischemia (DCI) with SAH animal models demonstrating impaired NVC throughout the acute period following aneurysm rupture, leading to an imbalance between metabolic demand and CBF, and rendering the brain vulnerable to ischemia in the face of spreading cortical depressions (54). In acute ischemic stroke (AIS) animal models, early impairment of NVC is associated with reduction in neuronally mediated feed-forward regulation of CBF as well as evidence of prolonged NVC disruption involving territories outside the zone of ischemia that does not resolve with the restoration of CBF, thus contributing to ongoing cerebral dysfunction and injury accumulation (52). Similar findings have been reported in human subjects, where global NVC dysfunction is described following AIS and correlated with the degree of vessel stenosis in addition to functional outcomes (34, 55, 56).
The majority of NVC research has focused on outpatient methods of assessment that rely on patient participation to perform cognitive, verbal or motor tasks with evaluation of a CBF response measured by non-invasive modalities such as TCD and advanced perfusion imaging (50, 52). While these methods are generally not suitable for patients in the ICU environment, several physiologic approaches leveraging MMM have been developed and allow for NVC assessment and recognition of disturbed cerebral physiology in critically ill patients. These methods often involve fast Fourier transformation of EEG signal into frequency bands to represent neuronal activity combined with a method of CBF estimation including ICP pulse-waveform, near infrared spectroscopy (NIRS) and TCD alterations in response to electrocortical activity (57–61). Though these approaches have largely been investigated in small cohorts as proof of principle studies, a recent investigation involving nine comatose patients with various forms of ABI were studied using NIRS-EEG and found normalization of NVC predicted the recovery of consciousness with >99% accuracy (62). Though these results need to be validated in a larger and more diverse cohort, they highlight the promising role NVC may play in improving our understanding of disturbed cerebral physiology across a spectrum of neurologic disorders including disorders of consciousness. It remains to be seen whether enhanced processes for monitoring and identifying disturbed NVC will provide a foundation to develop timely targeted interventions to ameliorate pathologic processes or refine our approach to prognostication.
Intracranial pressure monitoring
ICP monitoring was first described by Lundberg and colleagues in 1964 and has since become the most widely employed invasive monitoring technique with the largest amount of supporting data (4, 63). Elevations in ICP are directly related to excess mortality and poor neurologic outcomes, likely through destructive mechanical forces as well as reduced cerebral perfusion leading to ischemia and oligemia within vulnerable brain parenchyma (32, 64–66). The two most utilized invasive modalities for ICP monitoring are intraventricular catheters and parenchymal monitors, both of which can provide continuous ICP data whereas the former also allows for therapeutic CSF diversion. In general, these modalities are considered monitors of global ICP given pressure equilibration in the intracranial vault, however, in certain conditions such a unilateral masses with associated large midline shift or posterior fossa or infratentorial lesions, there may be an associated pressure gradient leading to differential ICP measurements depending on catheter tip placement (67).
ICP monitoring is a central focus in the management of severe TBI and recommended (Level IIB) by the Brain Trauma Foundation (BTF) for all patients with a Glasgow Coma Scale (GCS) 3–8 and abnormal CT scan as well as for patients who have a normal CT head but meet at least two of the three following criteria: age >40 years, unilateral or bilateral motor posturing, or systolic blood pressure (SBP) <90 mmHg (68). Furthermore, the International Multidisciplinary Consensus Conference on MMM in Neurocritical Care recommends ICP monitoring to better contextualize and interpret data generated from other monitoring devices given the robust level of evidence supporting its use (3). Adherence to a protocolized ICP management strategy in severe TBI has been demonstrated to decrease 2-week adjusted mortality and the recently published multinational SYNAPSE-ICU study found that ICP monitoring and management in a mixed cohort of ABI patients was associated with increased therapeutic intensity as well as improved neurologic outcome and 6-month mortality, with the largest magnitude of benefit in patients with more severe grade injury (69–71).
While it seems evident that avoidance of elevated ICP would be beneficial in ABI patients, efforts to define a precise treatment threshold for ICP have yielded variable results (66, 72, 73). In the 2000 BTF guidelines, an ICP threshold of 20–25 mmHg was recommended and later refined to less than or equal to 20 mmHg in 2007 (74). In most recent 2016 published guidelines, further adjusted the recommended threshold to <22 mmHg, largely based on a single retrospective analysis of 459 severe TBI patients where 22 mmHg best predicted the intersection between mortality and favorable outcome (26, 68). Subgroup analysis in this study found a threshold of 18 mmHg was more accurate for female and elderly patients, highlighting the heterogeneity present in subpopulations, and further studies have demonstrated that ICP values as low as 10 mmHg may still confer harm in some patients raising the question if a single “one size fits all” threshold is suitable (26, 75). These questions are further magnified by the results of the BEST TRIP trial where a management strategy solely focused on maintaining ICP at 20 mmHg or less was not shown to be superior to care based on imaging and clinical examination (76). More recently, the concept of a pressure-dose model has arisen which weighs both the magnitude and duration of pathological ICP to help inform treatment decisions (Figure 3) (32, 64, 77). According to this model, even ICP values below guideline recommended treatment thresholds (15–20 mmHg) can induce injury if sufficiently sustained whereas in one study, ICP values above 20 mmHg and 30 mmHg correlate with worse outcome after 37 and 8 min, respectively (32). These findings suggest that ICP should not be viewed as a dichotomous parameter with values below a particular threshold regarded as safe and those above considered dangerous in all patient populations. Moreover, this research has illuminated how vulnerability to ICP-related injury largely depends on CA status where even small elevations are poorly tolerated when CA is compromised (32, 78).
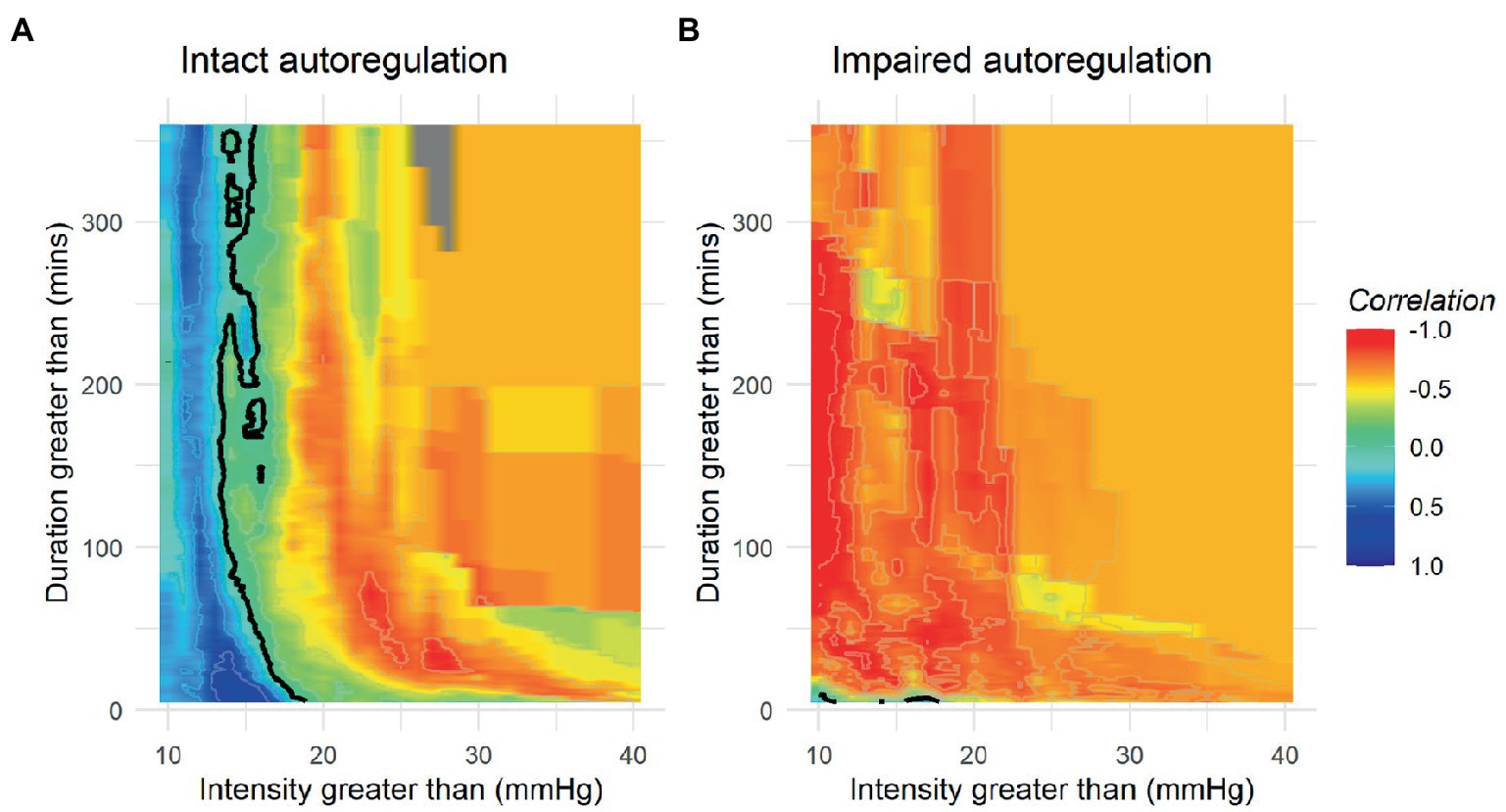
Figure 3. Population based incracranial pressure (ICP) intensity map stratified by autoregulatory status. Red areas indicate areas where ICP intensity is associated with poor outcome, while blue areas indicate good outcomes. Patients with intact autoregulation tolerate longer durations and intensities of ICP elevation compared to those with impaired autoregulation where no safe zone could be identified. (A) Intact autoregulation (mean PRx <= 0.3), (B) Impaired autoregulation (mean PRx > 0.3). Reprinted with permission from open access publication corresponding author, Akerlund and associates (52).
Cerebral perfusion pressure
Another important physiologic parameter indirectly measured from ICP monitoring is CPP, expressed by MAP-ICP, and reflects the driving pressure to the brain parenchyma. The BTF recommends maintaining CPP between 60 and 70 mmHg based on historical observational data demonstrating worse outcomes with lower thresholds as well as evidence of heightened risk for acute respiratory distress syndrome (ARDS), likely due to increased vasopressor use and fluid balance, when uniformly targeting CPP above 70 mmHg (8, 79, 80). Modest elevations in CPP have been shown to have a protective effect against ICP insults. However, recent investigations have demonstrated the ideal range of individualized CPP appears to be quite variable and highly dependent on CA status with evidence of average CPP <70 mmHg being poorly tolerated in those with impaired CA (26, 31, 32).
Pressure reactivity index
CA capacity can be assessed by the Pressure Reactivity Index (PRx), which is a moving Pearson’s correlation that expresses the relationship between slow-wave changes in ICP, a surrogate marker of pulsatile CBV, in response to alterations in MAP/CPP where positive values denote impaired CA and negative values suggest intact cerebrovascular reactivity (13). Multiple studies have validated the prognostic importance of PRx in TBI populations with thresholds of +0.05 and +0.25 for poor neurologic outcome and mortality, respectively (7, 26, 81). Certain TBI populations appear particularly susceptible to impaired CA following TBI as measured by PRx including the elderly as well as those with diffuse injury patterns (47, 82). Additionally, elevated PRx has been correlated with impaired cerebral metabolism and energy utilization in addition to expansion of cerebral edema following parenchymal contusion (83, 84). Trending continuous PRx as a function of ICP fluctuations recently has been used to assign individual ICP thresholds, where ICP values at which PRx is >+0.2, was found to have superior predictive value compared to static thresholds for mortality and functional outcomes (85, 86). Using a similar methodology, plotting PRx against CPP allows for the determination of optimal CPP (CPPOPT), where CPP values with the lowest associated PRx are interpreted as most ideal for preservation of autoregulatory status and a target for hemodynamic management (Figure 4) (7, 8). TBI patients with a median CPP that more closely approximates CPPOPT have been found to have improved cerebral oxygenation, more favorable markers of cerebral energy metabolism, and better clinical outcomes (25, 87–90). Recently, the Phase II COGITATE study published their results and highlighted the safety and feasibility of a CPPOPT guided protocol compared to BTF guideline recommendations of CPP 60–70 mmHg (46). Though not powered for outcomes, fewer patients died in the intervention arm (23% vs. 44%) with no increased in therapeutic intensity level or adverse events suggesting that such a strategy is achievable and safe in clinical practice and may improve functional recovery in TBI by ensuring adherence to individualized optimal physiologic targets.
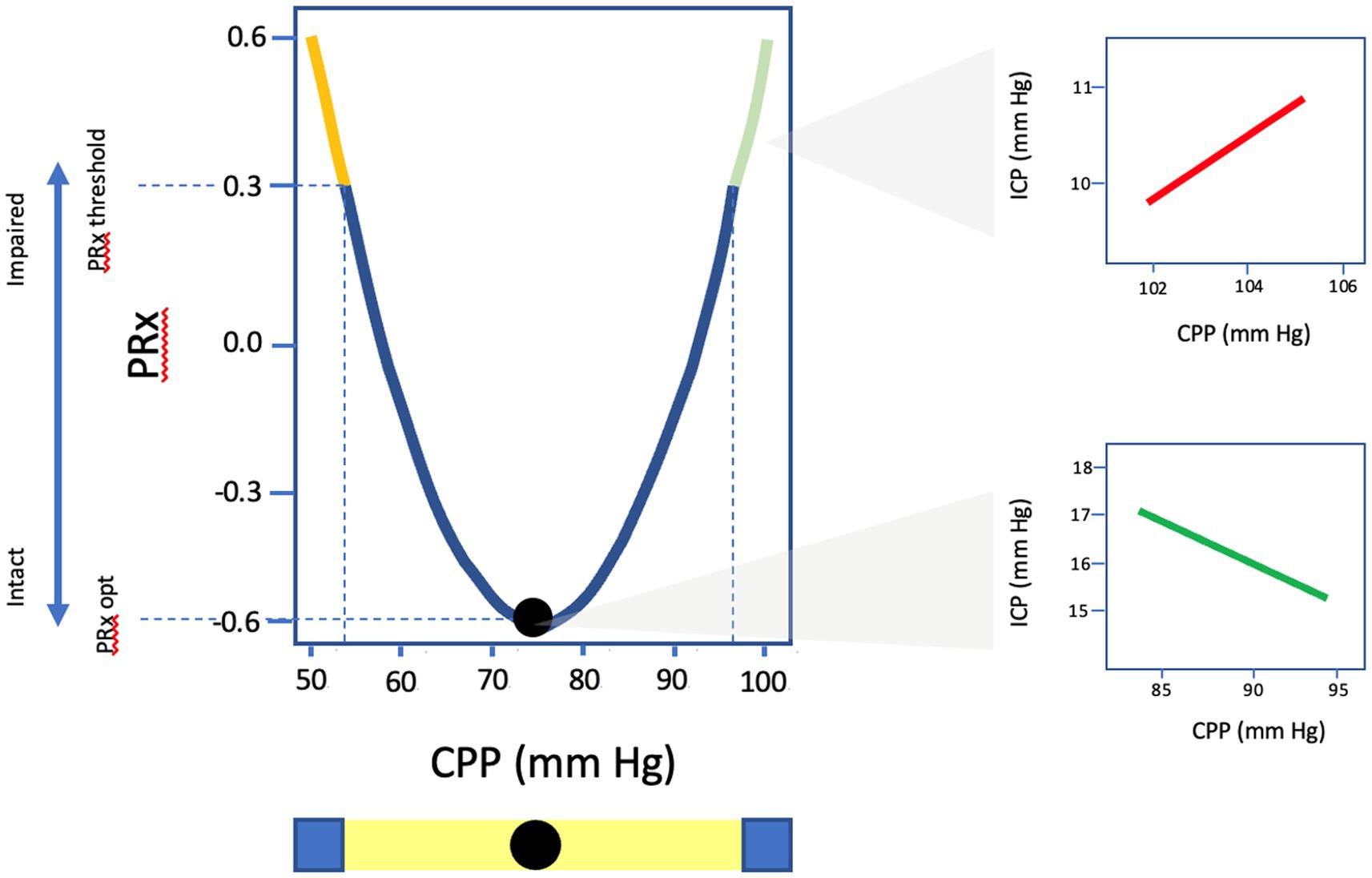
Figure 4. Graphic representation of U-shaped curve of PRx values as a function of CPP. CPPopt identified as the CPP value with the lowest associated PRx and most optimal autoregulatory state. PRx, pressure reactivity; CPP, cerebral perfusion pressure; ICP, intracranial pressure.
Though PRx and CPPOPT targeted therapy hold promising potential to transform the landscape of neurocritical care management and are the most well-established of all the continuous CA indices, there are several important limitations and questions that must be acknowledged. PRx requires exhausted intracranial compliance in order to visualize changes in ICP in response to minimal alterations in CBV and therefore may provide inconclusive results in patients lacking significant mass effect or with highly compliant cranial compartments such as seen in age-associated atrophy or following decompressive hemicraniectomy (35, 47, 91). Just as ICP monitoring represents a global evaluation of pressure, PRx represents a global estimator of CA and therefor is unable to characterize heterogeneous patterns of disturbed CA (90, 92). Furthermore, PRx requires high-frequency signal processing, on the level of second-by-second data acquisition which is labor intensive, expensive, and not widely available for clinical monitoring outside of several specialized academic centers. As a result, CPPOPT can be challenging to estimate in a significant number of individuals, particularly in the elderly, with 16–45% of monitored 4–6 h epochs in retrospective analysis incapable of generating a CPPOPT (25, 35, 89, 91). To address this issue, several studies have explored the use of minute-by-minute low-resolution PRx compared to standard PRx, finding that it is comparable in regards to outcome prediction and CPPOPT determination albeit with slightly lower precision (81, 93). These advances have the potential to expand the adoption of these monitoring approaches across a broader range of clinical settings lacking high-resolution signal monitoring however, widespread implementation cannot be expected until clinical efficacy is proven (90). Furthermore, innovations in non-invasive approaches to neuromonitoring such as automated robotic TCD has the potential to provide analogous and complementary information in ABI populations (94).
Cerebral oxygen monitoring
Though ICP/CPP monitoring plays a crucial role in the management of ABI, it does not provide information about the adequacy of cerebral perfusion and the underlying metabolic demand (CMRO2). Following ABI, derangements in CBF and oxidative metabolism are common and contribute to secondary brain injury in the form of cerebral hypoxia and metabolic crisis, independent of ICP and CPP (95–97). PET studies in patients with TBI reveals evidence of regional microvascular collapse leading to decreased diffusion capacity and ischemia occurring both in areas with damage and those that appear structurally normal on imaging (98). In clinical studies, the magnitude and duration of cerebral hypoxia has been shown to correlate with worse neurologic outcomes and increased mortality making it an attractive target for neuromonitoring (97, 99). The two most widely used modalities for cerebral oxygenation include jugular venous oxygen saturation (SjvO2), which measures the balance between global CBF and CMRO2, and brain tissue oxygen partial pressure (PbtO2) that provides information regarding CBF in addition to oxygen diffusion and delivery (100). As SjvO2 monitoring is not capable of continuous CA assessment and has largely fallen out of favor in most centers, this section will focus exclusively on PbtO2 (101).
Brain tissue oxygen partial pressure
Monitoring PbtO2 requires surgical placement, often into the subcortical white matter, and detects focal changes in oxygen tension (102, 103). PbtO2 is a complex and multidimensional physiologic parameter that reflects CBF in addition to oxygen delivery, diffusion and consumption (100). Normal values are between 23 and 35 mmHg though when <15–20 mmHg and <10 mmHg are considered moderate and severe hypoxia, respectively, with proportionate risk for irreversible cerebral ischemia (103–105). Given the focal nature of PbtO2 monitoring, regional variability is common and there is debate on whether monitors should be placed in healthy appearing parenchyma or in perilesional tissue that theoretically is most vulnerable to secondary insults (103, 105). Though values generated from perilesional tissue may provide the best discrimination for neurologic outcome, precise placement of probes is technically challenging and PbtO2 response to treatment is often blunted compared to healthy tissue (106, 107). In keeping with this notion, the protocol for the ongoing randomized controlled trial, “Brain Tissue Oxygen Monitoring and Management in Traumatic Brain Injury (BOOST-III)” includes placement of monitors 2 cm from the cortical surface in the least trauma-affected frontal lobe to promote consistency of practice and data interpretation (108). Probe position should always be confirmed on imaging and either FiO2 or MAP challenge should be utilized to ensure appropriate function before attempting to interpret data.
Low PbtO2 recordings are frequent following ABI, occurring in up to 87% of individuals during monitoring, and influenced by a variety of clinical insults including elevated ICP and suboptimal CPP leading to impaired CBF (65, 95, 109). Multiple studies have also demonstrated the importance of CA in maintaining normal PbtO2 with evidence that cerebral hypoxia is more common when CPP falls below PRx derived CPPOPT (88, 110, 111). Ensuring adequate systemic oxygenation is imperative to improving cerebral hypoxia and under usual conditions PbtO2 is very responsive to increases in FiO2. However, if PbtO2 is not responsive to increase in FiO2, other contributory factors should be assessed such as hemoglobin level, temperature, brain edema and ICP. It is also important to note that prolonged periods of hyperoxia may increase the risk of excitotoxicity (112, 113). In practice, maintaining a minimum PaO2 > 90 mmHg or SpO2 > 94–98% appears pragmatic to avoid cerebral hypoxia and recently the concept of a brain oxygen (BOx) ratio (PbtO2/PaO2) was introduced to assist in recognizing altered cerebral physiology even in the setting of normal PbtO2 due to overtreatment with FiO2 (114, 115). Optimization of PbtO2 requires an multidimensional tiered approach involving various measures aimed at correcting hypoxemia, reducing ICP, augmenting CPP and decreasing CMRO2 (113, 116).
Treatment response to subthreshold PbtO2 is predictive for survival whereas the duration and magnitude of cerebral hypoxia are strongly correlated with increased mortality and poor neurologic outcome (97, 99, 113, 117). In aneurysmal SAH, low PbtO2 can predict symptomatic vasospasm though the sensitivity depends heavily on whether the probe location corresponds to the affected vascular territory (118, 119). PbtO2 represents the second most commonly used invasive neuromonitor after ICP and the two together are the most widely published MMM combination (4). Bundling PbtO2 and ICP/CPP compared to managing ICP/CPP in isolation has been explored in multiple retrospective analyses, both in TBI and SAH populations, and found improved mortality and functional outcomes associated with combined therapy with no increase in length of stay or serious adverse events including ARDS (95, 99, 120–123). Though large prospective trial data is lacking, a 2015 small multicenter study found that PbtO2 and ICP/CPP guided therapy was associated with more aggressive ICP control and higher CPP with a trend toward improved functional outcomes at 6 months compared to ICP management alone (124). More recently the BOOST-II trial demonstrated that a protocolized PbtO2 and ICP/CPP combined management strategy was safe, feasible and associated with a lower burden of cerebral hypoxia and while not powered for outcome, demonstrated a trend toward lower mortality and better neurologic outcomes (108). Though the data at this time remains too limited to recommend widespread implementation of PbtO2 monitoring, based on the promising preliminary studies presented there are several Phase III multicenter randomized controlled trials currently underway, including BOOST-III, BONANZA, and OXY-TC, which will hopefully provide further insights into the role of PbtO2 based therapy in severe TBI management (36, 95).
Brain tissue oxygen pressure reactivity index
Intact CA function appears to exert a protective force in preventing cerebral hypoxia though low PbtO2 itself has also been implicated in the development of disturbed cerebrovascular reactivity (37, 110). Several studies have explored the relationship between PbtO2 and changes in CPP, termed Brain Tissue Oxygen Pressure Reactivity Index (ORx), which is a moving linear correlation coefficient of PbtO2 and CPP data obtained in 10–30 s intervals (37, 125). ORx has been postulated as an index of CA, similar to PRx, with negative values and those near 0 considered intact while disturbed CA is assumed as ORx approaches +1 (27). ORx values were correlated with unfavorable outcomes in a single study with a threshold of +0.3–0.4 and other investigations have demonstrated higher values associated with vasospasm and DCI in SAH patient populations (27, 28). Agreement between ORx and PRx has yielded mixed results with several studies demonstrating concordance between the two indices, particularly when PbtO2 is low, though it diverges when PbtO2 exceeds 40 mmHg with an increase in ORx despite stable PRx (27, 37). Subsequent investigations have yielded poor correlations between the two indices and an absence of a clear threshold for prognosticating outcomes or ability to determine a CPPOPT based on ORx (125–127). PbtO2 is a complex physiologic parameter influenced by a multitude of different systemic and cerebral factors, with CPP being only one aspect, likely hindering precise estimation of CA (116). In a high-resolution TBI dataset, PbtO2 failed to demonstrate a reliable response to slow-wave fluctuations in MAP or ICP as would be expected in a surrogate of CA, further calling into question whether PbtO2 is an appropriate parameter for CA derivation (125). Furthermore, PbtO2 involves monitoring a relatively small focal area of parenchyma, which can exhibit significant regional variability, thereby reducing generalizability and the ability to infer overall CA capacity (105, 106). At present, the data supporting ORx as a reliable surrogate of CA is limited and caution should be exercised before using it clinically. Further investigation is warranted to better understand circumstances when it may be of utility.
Transcranial Doppler
Transcranial Doppler (TCD) is a non-invasive neuromonitoring technique that has been used in clinical practice since first described by Aaslid and colleagues in 1982 to examine CBF in the basal cerebral arteries (128). CBF velocity (CBFV) and directionality can be determined from the degree of doppler shift created by a moving column of red blood cells through the proximal cerebral vasculature (129). TCD utilizes a low frequency probe (2 MHz) to penetrate the skull with the most common windows for insonation being the transtemporal and suboccipital approaches in which CBFV can be measured in the anterior and posterior circulations, respectively (130). Furthermore, the ophthalmic artery and carotid siphon can be insonated through the transorbital approach and the submandibular window allows for evaluation of the cervical portion of the internal carotid artery (129). While CBFV is proportional to CBF, TCD is unable to accurately account for vessel diameter, making it impossible to draw direct conclusions about CBF as vasodilation and vasoconstriction can also produce reciprocal changes in CBFV (130). Similar to the arterial waveform, the TCD flow velocity (FV) waveform is pulsatile, though lower resistance with continuous flow throughout the cardiac cycle, and allows for quantification of peak systolic velocity (PSV), end diastolic velocity (EDV) and mean flow velocity (MFV) parameters (131).
MFV is very sensitive to corresponding alterations in vessel caliber, making TCD a valuable screening tool for large vessel vasospasm in aneurysmal SAH and TBI (132, 133). Multiple thresholds for grading vasospasm have been proposed with the strongest level of evidence and predictive value found in MFV obtained from the middle cerebral arteries (MCA) (134, 135). MFV exceeding 160–200 cm/s in the MCA has a high positive predictive value (PPV) for moderate to severe angiographic vasospasm while those <120 cm/s are unlikely to have clinically significant spasm (136, 137). The Lindegaard Ratio reflects the MFV of the MCA relative to the ipsilateral ICA and is a useful tool to contextualize elevated velocities more related to vasospasm or hyperemia (138). The rate of vasospasm evolution and overall severity diagnosed on TCD, are established risk factors for the development of DCI, with a 90% sensitivity and 92% negative predictive value (NPV), thus allowing for early targeted intervention to prevent irreversible cerebral infarction and further supports the role of TCD for routine surveillance monitoring in this setting (40, 133, 138). In addition to vasospasm monitoring, TCD is a valuable modality in AIS evaluation with high sensitivity for microembolic signals which can be used for risk stratification of future ischemic events in the setting of carotid stenosis, blunt cerebrovascular injury, as well as cardiac disease and may be useful for guiding decisions for anticoagulation (38, 41, 139, 140).
Furthermore, FV waveform morphology is sensitive to alterations in CBF and provides valuable insights regarding perfusion status in different vascular beds. For example, elevated ICP characteristically leads to a more pronounced systolic upstroke and loss of the Windkessel notch due to external compression as well as reduced EDV signifying impaired diastolic flow (Figure 5) (131, 141, 142). When ICP exceeds the diastolic closing pressure, a reversal of diastolic CBFV is observed and may eventually progress to cerebrocirculatory arrest characterized by oscillating flow patterns, systolic spikes or absent flow and can be used as an acillary confirmatory test in brain death determination (143). Conversely, in patients with hyperemia due to disturbed CA or arteriovenous malformations, waveforms are typically low-resistance with high EDV relative to PSV and a diminished dicrotic notch (39).
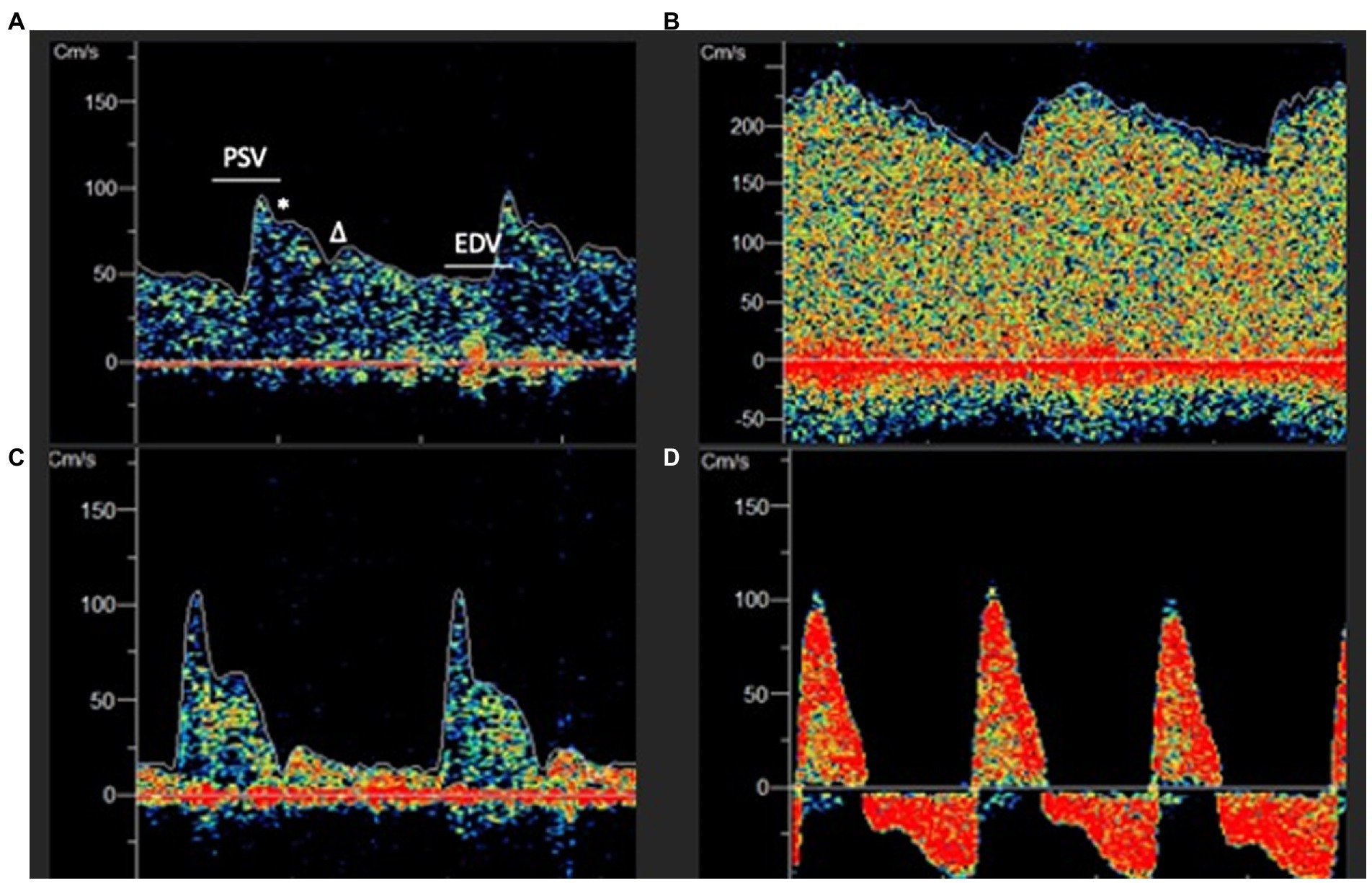
Figure 5. (A) Normal appearing low resistance TCD waveform. PSV, Peak systolic velocity; EDV, End Diastolic Velocity; * Windkessel Notch; Dicrotic Notch. (B) Hyperemic appearing waveform with elevated EDV, absent dicrotic notch and low pulsatility index (PI). (C) High resistance waveform with low EDV and elevated PI. (D) Extremely elevated intracranial pressure with peaked systolic upstroke and reversal of diastolic flow in a patient with cerebral circulatory arrest.
While these point of care applications are very useful in clinical practice, TCD is often performed in an isolated or intermittent fashion which limits the ability to capture dynamic alterations in the FV waveform and longitudinal changes in cerebrovascular hemodynamics (94). Furthermore, TCD signal acquisition is highly operator dependent and technically challenging with ~10% of patients lacking adequate temporal acoustic windows (144). Despite these limitations, recent innovations in automated robotic technology have made prolonged monitoring with TCD more suitable for the ICU environment to derive flow based indices for risk stratification and goal directed therapies (Figure 6) (145).
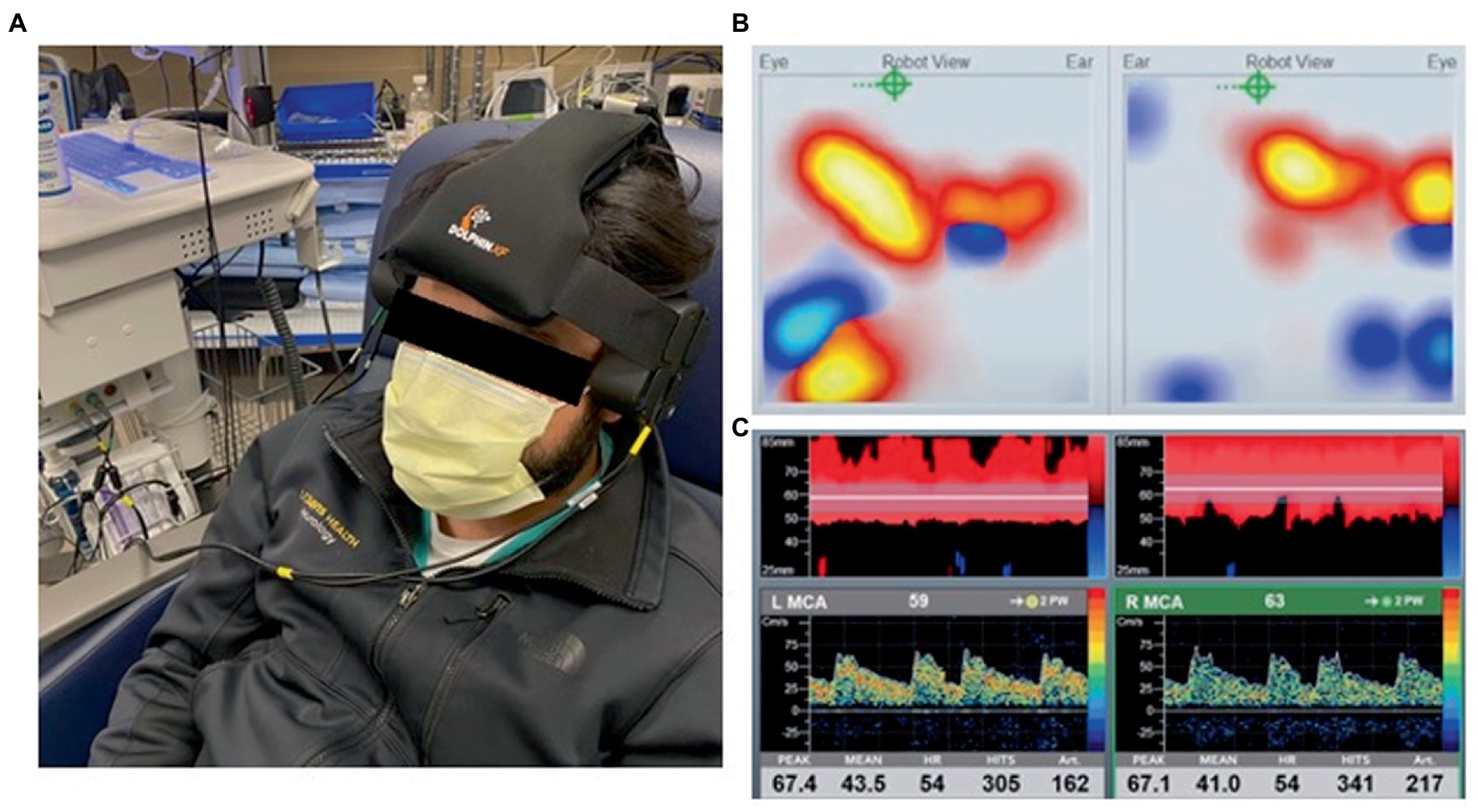
Figure 6. (A) Transcranial doppler (TCD) robotic headset placement. (B) Automated robotic scanning for intracranial vessel. (C) Continuous bilateral middle cerebral artery recordings with power M-mode and spectral doppler. Viasonix Dolphin/XF TCD Robotic Probe, Imaging Monitoring United States®.
Pulsatility index, FV thresholds and prognosis
The degree of waveform resistance can be quantified by the Gosling pulsatility index (PI) [(PSV-EDV)/MFV] which has gained particular interest due to its association with ICP and inverse relationship with CPP in the setting of brain injury (146, 147). Though this correlation has been validated in several investigations, particularly in the extremes of ICP and CPP, PI is a complex parameter influenced by multiple different factors including distal cerebrovascular resistance (CVR), CPP, arterial blood pressure (ABP), pulse amplitude, heart rate and cerebral artery compliance, making direct parallels with ICP/CPP challenging (148–150). This shifting relationship is highlighted during a hyperventilation challenge where an expected increase in CPP occurs due to a drop in ICP however PI paradoxically increases as a result of arteriolar vasoconstriction and increased CVR (148). Notwithstanding these limitations, elevated PI above 1.3–1.5 in patients with TBI has been linked to increased risk of neurologic decline and worse outcome, particularly if measured within the first 24 h of admission, and predict the development of malignant cerebral edema, herniation and midline shift in patients with MCA territory ischemic stroke (151–153).
TCD derived CBFV measurements also provide useful information regarding the competency of cerebral perfusion, with low values suggestive of oligemia, and have been linearly correlated with CBF using computed tomography perfusion (CTP) in patients with diffuse traumatic injury patterns (154). A low-flow state defined as MCA MFV below 40 cm/s, occurs in over half of TBI patients during the first 24 h after injury, most often ipsilateral to focal pathology and correlates with the burden of cerebral hypoxia (PbtO2 <20 mmHg) (155, 156). The combination of elevated PI (suggesting increased resistance) with MFV 35–40 cm/s or EDV <20–25 cm/s (signifying impaired CBF) identifies TBI patients with particularly high risk of poor outcome and has even been validated in a mixed ICU population of patients with coma for mortality (152, 153, 157, 158). In addition to prognostication, TCD based thresholds may be used to recognize patients at risk for early deterioration and guide targeted therapy in hopes of preventing cerebral hypoperfusion. This has been exemplified in single center studies where mannitol administration in patients with hemispheric injury led to improvement in dangerously low MFV as well as aggressively treating abnormal TCD findings with hyperosmolar therapy, vasopressors and surgical intervention leading to normalization of TCD parameters in over 80% of patients (159, 160). Though external validation is required to better understand optimal treatment thresholds and the impact of TCD goal-directed therapy on outcomes, these studies illustrate how non-invasive TCD may provide insights into cerebral physiology at the bedside and inform personalized management decisions to limit secondary brain injury.
Non-invasive ICP and CPP
The TCD waveform is responsive to changes in vascular tone and CBF with corresponding alterations in morphology in the setting of fluctuations in ICP and ABP (161, 162). Given this close relationship, there has been significant emphasis placed on developing non-invasive quantitative methods for ICP and CPP estimation (nICP and nCPP) based on TCD waveform morphological features (161). Initial efforts focused on PI as a quantitative measure of non-invasive ICP (nICP) given the strong correlation between the two parameters during periods of ICP elevation (148). The most favorable results were published by Bellner in 2014, expressed as nICP = 10.927 × PI – 1.284 with a 95% confidence interval of ±4.2 mmHg and strong correlation coefficient (R = 0.94), however these results could not be replicated in other patient populations (149, 163). Subsequent nICP investigations using PI have yielded less promising results with a wide range of confidence intervals, likely owing to factors other than ICP that impact PI, and suggest a limited role for PI in nICPP determination (161).
Several other quantitative approaches have focused on nCPP. This allows for nICP estimation using the mathematical relationship nICP = ABP – nCPP. These methodologies rely on TCD and ABP waveform analysis and include the diastolic flow velocity model (nICP_FVd), which leverages the close relationship between EDV and CPP, and the critical closing pressure (CrCP) model (nICP_CrCP) based on the concept of the minimum ABP required to prevent microvascular circulation and cessation of blood flow (161, 164, 165). A 2016 study from Cambridge United Kingdom compared these approaches in addition to PI derived nICP (nICP_PI) and a mathematical “black box (BB)” model (nICP_BB). Using a prospective TBI cohort with invasive ICP monitoring, researchers found that nICP_FVd, nICP_CrCP and nICP_BB generally performed well in ICP estimation with nICP_BB providing the most accurate ICP prediction with the least bias (166). In contrast, nICP_PI was the most sensitive to changes in ICP however proved to be the worst estimator of absolute ICP values.
Subsequent investigations using these non-invasive methods in different populations has produced mixed results with evidence of good correlation, though less accurate prediction, of absolute nICP and nCPP values (167, 168). The multicenter IMPRESSIT-2 study found nICP estimation based on nICP_FVd resulted in a high NPV for ICP >20 mmHg and >25 mmHg, 91.3 and 98.6%, respectively, though a concordance correlation between nICP and invasive ICP of only 33.3% (169). Conversely, in a recent study involving 100 TBI patients, nICP_FVd had essentially no agreement with invasive ICP (R = −0.17; 95% CI: −0.35, 0.03; p = 0.097) and a sensitivity of 0% for detecting ICP >20 mmHg, though notably few patients had elevations in ICP in this cohort (170). Similar findings have been reported in nCPP estimation in children with TBI using nICP_CrCP where the ability to identify CPP values below 70 mmHg was excellent (AUC = 0.91; 95% CI: 0.83–0.99) though overestimated true CPP by about 20 mmHg and was less precise for more clinically relevant thresholds of CPP < 60 mmHg and <50 mmHg (167). In another pediatric TBI study, both nICP_FVd and nICP_CrCP were compared against invasive monitoring and while there was a strong correlation between both models and CPP, each were also associated with wide limits of agreement and unable to discriminate CPP values <50–60 mmHg (171). Although the performance characteristics of current non-invasive TCD models preclude the ability to precisely measure ICP and CPP, they may still serve as a primary assessment tool in the acute stages of management or in patients who are not candidates for invasive monitoring. Furthermore, combining TCD parameters with other noninvasive non-invasive indices such as optic nerve sheath diameter and pupillometry may improve the diagnostic accuracy to identify patients with elevated ICP (172, 173).
TCD derived autoregulatory indices
Given the close relationship of the TCD waveform to fluctuations in perfusion, evaluating for CA is made possible by way of a moving correlation coefficient to model the relationship between alterations in CBFV in response to slow wave changes in CPP. These indices including Mx, Sx, and Dx which correspond to TCD derived MFV, PSV, and EDV, respectively (174). In TBI patients, Mx is the most well-established index and appears to accurately capture dynamic CA fluctuations with evidence of a pressure passive state when significantly elevated and exhibits a moderate correlation to ICP-derived PRx (48, 162, 175). In particular, during elevations in ICP above 30 mmHg, there is an observed divergence which may be explained in part by the fundamental differences in how the two indexes relate to cerebrovascular physiology (48, 175, 176). In contrast, Sx appears to more closely approximate PRx, potentially related to increased systolic peaks and arterial pulsatility in the setting of elevated ICP, while Dx appears to possess limited clinical utility (45, 48). Both Mx and Sx correlate with mortality as well as neurologic outcome in TBI populations with evidence from several reports suggesting that Sx may possess superior outcome prediction characteristics (44, 48, 174). Using a small TBI cohort, Mx and Sx were found to have a parabolic relationship with CPP, thus allowing for determination of CPPOPT with overall good agreement compared to PRx (42). Furthermore, by substituting ABP for CPP, Mx_a, and Sx_a can be determined with the potential to estimate PRx and CPPOPT without the need for an ICP monitor and may provide a path forward for use of TCD-based advanced cerebral monitoring and goal directed therapy in a wider population of critically ill patients without invasive monitors (145, 174, 177).
Other potential advantages of TCD rely on the fact that it can interrogate different vascular beds and provide a better understanding of physiologic asymmetries, including CA capacity, compared to other global monitors. CBF is highly heterogeneous in patients following TBI with more frequent loss of CA ipsilateral to the site of injury and the overall magnitude of TCD-derived hemispheric CA asymmetry has been demonstrated to confer an increased risk of death and poor neurologic outcome (43, 178). In patients presenting with MCA stroke, the degree of CA dysregulation, as measured by the ipsilateral Mx_a, correlates with worse functional outcomes and larger overall final infarct volumes (179). Similar findings have been described in patients presenting with spontaneous intracerebral hemorrhage (ICH) where a gradual decline in CA capacity ipsilateral to the hemorrhage location over the first 5 days was associated with a decline in clinical exam and worse 90-day outcomes (180). In SAH, increases in Mx_a and Sx_a have been associated with the development of vasospasm in the ipsilateral hemisphere and combining radiographic vasospasm with increasing Mx_a from baseline over the first 7 days from ictus has been correlated with the development of DCI (181, 182).
Given advances in TCD technology, it is conceivable that CA could be monitored simultaneously in different hemispheres to allow for more precise evaluation of heterogeneous cerebral physiology and estimation of regional CPPOPT. Furthermore, unlike PRx, which is based on changes in ICP as a surrogate of CBV and relies on the intracranial pressure-volume relationship, TCD more directly measures CBF and can provide insights into dynamic CA changes even in the setting of highly compliant intracranial systems where PRx may not demonstrate significant fluctuations (35, 47). Despite these reasons for optimism, much work still needs to be done to validate TCD as a feasible continuous or semi-continuous monitoring technique as the majority of published studies report only snap-shots in time with no study reporting monitoring durations longer than 4 h (183). Furthermore, the vast majority of TCD derived CA reports are from data generated at the Addenbrooke’s Hospital in Cambridge United Kingdom, highlighting the need for external validation in different hospital settings and patient populations (183). Recently, a proposed protocol was published for a prospective multicenter study in severe TBI patients utilizing TCD with ICP/PbtO2 monitors with the goal of obtaining high-fidelity continuous data and correlate TCD based indices such as Mx/Mx_a, Sx/Sx_a, and CrCP with invasive parameters (94). This study and others like it may enhance our understanding of how TCD based indices may complement or alternatively serve as a surrogate for other MMM outputs and identify clinically relevant targets for future interventions.
Near-infrared spectroscopy
Near-infrared spectroscopy (NIRS) is a non-invasive monitoring modality that can provide real-time information about regional cerebral oxygenation and CBF. The basis of this technology involves the emission of a near-infrared light source, in the range of 700–1,100 nm, to penetrate through the skin, cranium and most superficial few centimeters of brain (184). Depending on different tissue characteristics and cellular interfaces, the emitted light can either be scattered, reflected back to the detector, or absorbed by different chromophores such as protein, lipids and water (185). Hemoglobin is one such important organic macromolecule that exhibits differential absorption spectra characteristics depending on oxygenation status, thus allowing for determination of relative concentrations of oxyhemoglobin (HbO) and deoxyhemoglobin (HHb) in tissue according to the Modified Beer–Lambert law (186, 187). This technique provides estimates of total hemoglobin concentration (HbT) as well as the ratio of HbO to HbT called regional cerebral oxygen saturation (rSO2) or Tissue Oxygenation Index (TOI) depending on the manufacturer (185, 188). Similar to PbtO2, rSO2, and TOI reflect the balance between oxygen supply and demand within the distal arterial, venous and capillary territory and has demonstrated good correlation with invasively obtained absolute CBF values in critically ill patients (189–191). The majority of NIRS applications involve placing optodes over the forehead and measure signal over the frontal lobe gray matter and watershed zone of the anterior and middle cerebral arteries with the normal range of rSO2 being 55–80% (192, 193).
Low rSO2/TOI values, defined as <50–60% is concerning for ischemic insult in a single study demonstrating TOI thresholds of ≥75 and <55% correlating well with CPP values above and below 70 mmHg, respectively (192, 194). Though these thresholds serve as a reference, intra-patient optical measurement can vary significantly depending on cranial geometry and it is often more useful to monitor for relative changes in rSO2/TOI to detect cerebral ischemia (195). In TBI patients monitored with NIRS, cerebral hypoxia and hypoperfusion events are common even in the presence of accepted normal CPP range and has been correlated with more severe grade injury and higher likelihood of mortality (196, 197). NIRS has also been studied extensively in comparison to other modalities for cerebral oxygen assessment including to SjvO2, which is a global measure of cerebral oxygen supply and utilization, and exhibits a modest correlation with conflicting findings regarding sensitivity to changes in ABP, PaCO2, and CPP (191, 198, 199). Similarly when compared to PbtO2, NIRS has demonstrated variable levels of concordance with one study reporting an inability to detect clinically significant episodes of low PbtO2 with a high failure rate due to poor sensor-skin contact, scalp hematoma and subdural air after cranial procedures (185, 200). Conversely in a study with 42 TBI patients, NIRS and TCD demonstrated parallel findings with PbtO2, though more rapidly detected alterations in ABP and ICP, supporting the concept that no single bedside monitor represents the “gold standard” for cerebral oxygenation, with each device appearing to monitor different components of cerebral oxygenation, and may enhance diagnostic utility when used in complementary overlapping approaches (201, 202).
Autoregulation indices
Multiple studies have explored the capacity of NIRS for non-invasive CA determination by correlating slow wave oscillations in NIRS derived parameters, such as rSO2, TOI, or HbT, with alterations in ABP or CPP resulting in the Cerebral oximetry index (COx), Tissue Oxygen Reactivity Index (TOx) and Total Hemoglobin Reactivity Index (THx), respectively (203, 204). These indices have been validated in piglet animal models, where they demonstrate a strong agreement with laser-doppler flow measurements and were capable of detecting both upper and lower limits of autoregulation during controlled titration of ABP (205–208). In clinical studies, NIRS based indices have been compared to ICP derived PRx with good overall correlation however a less robust agreement has been described in the presence of insufficient slow wave power or during periods of significant NIRS interhemispheric variance (203, 209).
TOx and COx are the most widely used parameters for CA estimation and demonstrate a strong correlation with TCD-derived Mx/Mxa (r = 0.55–0.81) in both operative and ICU based studies with thresholds of ~0.2–0.3 denoting loss of CA (210–213). Furthermore, combining Sx_a and TOx in SAH patients appears to strengthen the predictive capabilities for detection of DCI, likely due to measurement of different, though complementary, anatomic components of the cerebral vascular system for enhanced surveillance (214, 215). In mixed ICU populations, impaired CA as determined by TOx/COx has been correlated with the development of ICU delirium and all-cause mortality at 3 months presumably due to CBF dysregulation (216–218). Multiple investigations have also assessed the utility of NIRS in cardiac arrest patients, who often do not have invasive neuromonitoring and guidelines for hemodynamic management have traditionally focused on static thresholds (219). In these studies, higher TOx/COx values over the first 3 days is independently associated with an excess in mortality (220). Additionally several studies have demonstrated the feasibility of determining optimal MAP based on COx with evidence of a wide diversity of optimal thresholds across individuals, likely reflecting different cerebral injury patterns and impact of preexisting hypertension, with more favorable outcomes noted when the actual MAP more closely aligns with NIRS derived thresholds (219, 221, 222). These findings highlight the potential utility of NIRS technology to provide a foundation for non-invasive individualized hemodynamic management in patients with hypoxic ischemic brain injury. Similar findings have been reported in TBI patients using COx derived CPPOPT, which when compared to ORx, more strongly correlates with PRx derived thresholds with evidence that deviations between actual CPP and CPPOPT of >10 mmHg are more likely to be associated with adverse outcomes (223).
Other studies have focused on THx, alternatively referred to as Hemoglobin Volume Index (HVx), which, in contrast to the CBF weighted TOx/COx, may be more reflective of CBV-based measurements in a similar fashion to PRx (193, 203). In a cohort of 40 TBI patients, THx demonstrated a significant association with PRx in individual recordings with ~50% of recordings suitable to determine optimal CPP and MAP with good agreement compared to PRx derived thresholds (204). In another TBI study, while correlation with PRx was similar between THx and TOx, albeit with large limits of agreement, THx demonstrated poor correlation with Mx supporting the notion that NIRS may provide information about distinctive features of CBF compared to TCD based parameters with the potential for complementary assessment in clinical situations where invasive monitoring is not otherwise indicated or safe (203).
Similar to TCD, NIRS has the advantage of being completely non-invasive, portable and capable of providing regional assessment of cerebral physiology and oxygenation. In contrast to TCD however, NIRS has the added benefit of being non-operator dependent, easily applied in the operative and ICU environments, without the need for frequent calibration and capable of generating continuous monitoring data (185, 187). Multiple different NIRS techniques have been developed with particular strengths and limitations in addition to proprietary algorithms and indices. For example, depending on the manufacturer, the ratio of HbO relative to HbT can be described by rSO2 as well as TOI, ScO2, and SpO2 with similar, though not completely interchangeable, values (188). The most common techniques include fixed wavelength, spatially-resolved, frequency-resolved, time-resolved and diffuse correlation spectroscopy techniques which are beyond the scope of this review to individually detail though differ in their associated cost, depth of measurement, precision and ability to provide complementary information about absolute CBF as well as CMRO2 (187).
Though advances in NIRS technology provides grounds for optimism regarding the development of non-invasive assessment of cerebral hemodynamics, oxygenation and metabolism, there are several limitations that must be addressed. First, NIRS requires a close spatial relationship between the cortex and cranium and is prone to inaccurate readings in the setting of post-operative pneumocephalus, skin pigmentation, scalp edema, extracranial hemodynamics, frontal contusions, and hemorrhage which are all common in neuro-ICU populations (224–226). As a regional monitor, it is only sensitive for changes in the superficial cortical structures, often restricted to the frontal brain region, and is unable to detect distant ischemic events (204). As a complex physiologic parameter, NIRS-based cerebral oxygenation is also influenced by a multitude of physiologic factors thus limiting attempts to draw direct conclusions about cerebral respiration and CBF. Efforts to establish clear prognostic thresholds are hindered by a multitude of different proprietary indices and conflicting reports across diverse pathologies and patient populations (185, 195, 211, 227). While the assortment of different NIRS based technologies allows for focused investigation and novel insights into aspects of cerebral physiology, the reporting of multiple similar yet distinct parameters limits the generalizability of scientific findings across different devices (228). The role of NIRS monitoring in neurocritical care populations has yet to be verified in large, multicenter trials, and at this time should continue to be explored under the context of clinical research. Further investigation is required to determine optimal NIRS based technology, monitoring parameters, and thresholds to optimize CA and potentially improve neurologic outcomes across a spectrum of ABI patients with particular focus in populations where invasive monitoring is not routinely performed or is otherwise contraindicated.
Current limitations and future directions
While it is evident that MMM allows for improved characterization of complex and often interrelated neurophysiologic determinants, considerable heterogeneity exists in the application and utilization of advanced neuromonitoring due to lack of familiarity, cost of high-resolution data acquisition and uncertainty regarding clinical efficacy (4, 9). Though there is accumulating data to suggest that targeted management of cerebral parameters such as pressure, blood flow and metabolism may prevent neurologic deterioration, there is a lack of high-quality prospective data to support widespread implementation of protocolized monitoring strategies. Even when considering CA and the advances in continuous assessment capabilities, current therapeutic interventions appear entirely inadequate to correct dysregulated CA thus underscoring the gap in our understanding of molecular and cellular pathways involved in cerebral homeostasis (229–231). Future research will need to focus on improved methods of data collection with more clearly defined pathologies, clinically meaningful outcomes, and assessment of secondary neurologic injury. As the number of MMM strategies expands, integrative approaches with a focus on standardized methods of real-time data visualization and analysis will be needed to translate these approaches effectively from research into clinical practice (232). Additionally, leveraging machine learning technology to provide automated detection of multidimensional physiologic patterns may help forecast deleterious events and provide an opportunity to intervene before there is risk of permanent injury thereby engaging in preventive rather than reactionary treatment approaches (233–235).
Conclusion
There is ample mounting evidence that invasive and non-invasive neuromonitoring techniques provide instrumental information regarding the cerebral physiome that otherwise would not be possible with standard bedside clinical assessment and imaging alone. Integration of various neuromonitoring modalities using a central interface at the bedside allows clinicians to monitor physiologic data in real time, which, not only facilitates improved understanding of complex neurophysiologic processes but can also guide targeted interventions to restore cerebral homeostasis. Goal directed therapy using neuromonitoring tools can however, only be realized when multimodal data is collected and integrated using high-resolution time-synchronized data collection and archiving system. Such technological advances have the potential to transition neurocritical care from standardized “one size fits all” treatment paradigm based on epidemiologic studies, to a focus on precision medicine centered on continuously adjusted individualized thresholds with the goal of improving patient outcomes. Substantial need still exists for innovative methods of enhanced data integration and interpretation as well as high-quality outcome-based studies before widespread application and acceptance can be expected.
Author contributions
JV designed and conceptualized the manuscript, literature review, interpretation and summarization of data, drafting of the manuscript, and final approval of the manuscript. NL substantial contribution in the design and draft of the initial manuscript, literature review, interpretation and summarization of data, and approval of the final manuscript. SM designed and conceptualized the manuscript, literature review, interpretation and summarization of data, critical revision of the manuscript for important intellectual content, and final approval of the manuscript. All authors contributed to the article and approved the submitted version.
Conflict of interest
The authors declare that the research was conducted in the absence of any commercial or financial relationships that could be construed as a potential conflict of interest.
Publisher’s note
All claims expressed in this article are solely those of the authors and do not necessarily represent those of their affiliated organizations, or those of the publisher, the editors and the reviewers. Any product that may be evaluated in this article, or claim that may be made by its manufacturer, is not guaranteed or endorsed by the publisher.
References
1. Roh, D, and Park, S. Brain multimodality monitoring: updated perspectives. Curr Neurol Neurosci Rep. (2016) 16:56. doi: 10.1007/s11910-016-0659-0
2. Sinha, S, Hudgins, E, Schuster, J, and Balu, R. Unraveling the complexities of invasive multimodality neuromonitoring. Neurosurg Focus. (2017) 43:E4. doi: 10.3171/2017.8.FOCUS17449
3. Le Roux, P, Menon, DK, Citerio, G, Vespa, P, Bader, MK, Brophy, GM, et al. Consensus summary statement of the international multidisciplinary consensus conference on multimodality monitoring in Neurocritical care: a statement for healthcare professionals from the Neurocritical care society and the European Society of Intensive Care Medicine. Neurocrit Care. (2014) 21:1–26. doi: 10.1007/s12028-014-0041-5
4. Tas, J, Czosnyka, M, van der Horst, ICC, Park, S, van Heugten, C, Sekhon, M, et al. Cerebral multimodality monitoring in adult neurocritical care patients with acute brain injury: a narrative review. Front Physiol. (2022) 13:1071161. doi: 10.3389/fphys.2022.1071161
5. Chen, HI, Stiefel, MF, Oddo, M, Milby, AH, Maloney-Wilensky, E, Frangos, S, et al. Detection of cerebral compromise with multimodality monitoring in patients with subarachnoid hemorrhage. Neurosurgery. (2011) 69:53–63. doi: 10.1227/NEU.0b013e3182191451
6. Meyfroidt, G, Bouzat, P, Casaer, MP, Chesnut, R, Hamada, SR, Helbok, R, et al. Management of moderate to severe traumatic brain injury: an update for the intensivist. Intensive Care Med. (2022) 48:649–66. doi: 10.1007/s00134-022-06702-4
7. Steiner, LA, Czosnyka, M, Piechnik, SK, Smielewski, P, Chatfield, D, Menon, DK, et al. Continuous monitoring of cerebrovascular pressure reactivity allows determination of optimal cerebral perfusion pressure in patients with traumatic brain injury. Crit Care Med. (2002) 30:733–8. doi: 10.1097/00003246-200204000-00002
8. Donnelly, J, Czosnyka, M, Adams, H, Robba, C, Steiner, LA, Cardim, D, et al. Individualizing thresholds of cerebral perfusion pressure using estimated limits of autoregulation. Crit Care Med. (2017) 45:1464–71. doi: 10.1097/CCM.0000000000002575
9. Sivakumar, S, Taccone, FS, Rehman, M, Hinson, H, Naval, N, and Lazaridis, C. Hemodynamic and neuro-monitoring for neurocritically ill patients: an international survey of intensivists. J Crit Care. (2017) 39:40–7. doi: 10.1016/j.jcrc.2017.01.005
10. Zeiler, FA, Aries, M, Czosnyka, M, and Smielewski, P. Cerebral autoregulation monitoring in traumatic brain injury: an overview of recent advances in personalized medicine. J Neurotrauma. (2022) 39:1477–94. doi: 10.1089/neu.2022.0217
11. Czosnyka, M, and Miller, C. Monitoring of cerebral autoregulation. Neurocrit Care. (2014) 21:95–102. doi: 10.1007/s12028-014-0046-0
12. Kawoos, U, McCarron, R, Auker, C, and Chavko, M. Advances in intracranial pressure monitoring and its significance in managing traumatic brain injury. IJMS. (2015) 16:28979–97. doi: 10.3390/ijms161226146
13. Czosnyka, M. Monitoring and interpretation of intracranial pressure. J Neurol Neurosurg Psychiatry. (2004) 75:813–21. doi: 10.1136/jnnp.2003.033126
14. Calviello, L, Donnelly, J, Cardim, D, Robba, C, Zeiler, FA, Smielewski, P, et al. Compensatory-reserve-weighted intracranial pressure and its association with outcome after traumatic brain injury. Neurocrit Care. (2018) 28:212–20. doi: 10.1007/s12028-017-0475-7
15. Fan, JY, Kirkness, C, Vicini, P, Burr, R, and Mitchell, P. Intracranial pressure waveform morphology and intracranial adaptive capacity. Am J Crit Care. (2008) 17:545–54. doi: 10.4037/ajcc2008.17.6.545
16. Nucci, CG, De Bonis, P, Mangiola, A, Santini, P, Sciandrone, M, Risi, A, et al. Intracranial pressure wave morphological classification: automated analysis and clinical validation. Acta Neurochir. (2016) 158:581–8. doi: 10.1007/s00701-015-2672-5
17. Lu, CW, Czosnyka, M, Shieh, JS, Smielewska, A, Pickard, JD, and Smielewski, P. Complexity of intracranial pressure correlates with outcome after traumatic brain injury. Brain. (2012) 135:2399–408. doi: 10.1093/brain/aws155
18. Rostrup, E, Knudsen, GM, Law, I, Holm, S, Larsson, HBW, and Paulson, OB. The relationship between cerebral blood flow and volume in humans. Neuro Image. (2005) 24:1–11. doi: 10.1016/j.neuroimage.2004.09.043
19. Ainslie, PN, Ashmead, JC, Ide, K, Morgan, BJ, and Poulin, MJ. Differential responses to CO2 and sympathetic stimulation in the cerebral and femoral circulations in humans: blood flow responses to sympathetic stimulation and CO2 in humans. J Physiol. (2005) 566:613–24. doi: 10.1113/jphysiol.2005.087320
20. Grüne, F, Kazmaier, S, Stolker, RJ, Visser, GH, and Weyland, A. Carbon dioxide induced changes in cerebral blood flow and flow velocity: role of cerebrovascular resistance and effective cerebral perfusion pressure. J Cereb Blood Flow Metab. (2015) 35:1470–7. doi: 10.1038/jcbfm.2015.63
21. Slupe, AM, and Kirsch, JR. Effects of anesthesia on cerebral blood flow, metabolism, and neuroprotection. J Cereb Blood Flow Metab. (2018) 38:2192–208. doi: 10.1177/0271678X18789273
22. van der Kleij, LA, De Vis, JB, de Bresser, J, Hendrikse, J, and Siero, JCW. Arterial CO2 pressure changes during hypercapnia are associated with changes in brain parenchymal volume. Eur Radiol Exp. (2020) 4:17. doi: 10.1186/s41747-020-0144-z
23. Laffey, HG, and Kavanagh, BP. Hypocapnea. N Engl J Med. (2002) 347:43–53. doi: 10.1056/NEJMra012457
24. Johnston, AJ, Steiner, LA, Gupta, AK, and Menon, DK. Cerebral oxygen Vasoreactivity and cerebral tissue oxygen reactivity. Surv Anesthesiol. (2003) 90:774–86. doi: 10.1093/bja/aeg104
25. Aries, MJH, Czosnyka, M, Budohoski, KP, Steiner, LA, Lavinio, A, Kolias, AG, et al. Continuous determination of optimal cerebral perfusion pressure in traumatic brain injury*. Crit Care Med. (2012) 40:2456–63. doi: 10.1097/CCM.0b013e3182514eb6
26. Sorrentino, E, Diedler, J, Kasprowicz, M, Budohoski, KP, Haubrich, C, Smielewski, P, et al. Critical thresholds for cerebrovascular reactivity after traumatic brain injury. Neurocrit Care. (2012) 16:258–66. doi: 10.1007/s12028-011-9630-8
27. Jaeger, M, Soehle, M, Schuhmann, MU, and Meixensberger, J. Clinical significance of impaired cerebrovascular autoregulation after severe aneurysmal subarachnoid hemorrhage. Stroke. (2012) 43:2097–101. doi: 10.1161/STROKEAHA.112.659888
28. Jaeger, M, Schuhmann, MU, Soehle, M, Nagel, C, and Meixensberger, J. Continuous monitoring of cerebrovascular autoregulation after subarachnoid hemorrhage by brain tissue oxygen pressure reactivity and its relation to delayed cerebral infarction. Stroke. (2007) 38:981–6. doi: 10.1161/01.STR.0000257964.65743.99
29. Lassen, NA. Cerebral blood flow and oxygen consumption in man. Physiol Rev. (1959) 39:183–238. doi: 10.1152/physrev.1959.39.2.183
30. Brassard, P, Labrecque, L, Smirl, JD, Tymko, MM, Caldwell, HG, Hoiland, RL, et al. Losing the dogmatic view of cerebral autoregulation. Physiol Rep. (2021) 9:e14982. doi: 10.14814/phy2.14982
31. Güiza, F, Meyfroidt, G, Piper, I, Citerio, G, Chambers, I, Enblad, P, et al. Cerebral perfusion pressure insults and associations with outcome in adult traumatic brain injury. J Neurotrauma. (2017) 34:2425–31. doi: 10.1089/neu.2016.4807
32. Güiza, F, Depreitere, B, Piper, I, Citerio, G, Chambers, I, Jones, PA, et al. Visualizing the pressure and time burden of intracranial hypertension in adult and paediatric traumatic brain injury. Intensive Care Med. (2015) 41:1067–76. doi: 10.1007/s00134-015-3806-1
33. Zeiler, FA, Donnelly, J, Calviello, L, Menon, DK, Smielewski, P, and Czosnyka, M. Pressure autoregulation measurement techniques in adult traumatic brain injury, part I: a scoping review of intermittent/semi-intermittent methods. J Neurotrauma. (2017) 34:3207–23. doi: 10.1089/neu.2017.5085
34. Liu, X, Pu, Y, Wu, D, Zhang, Z, Hu, X, and Liu, L. Cross-frequency coupling between cerebral blood flow velocity and EEG in ischemic stroke patients with large vessel occlusion. Front Neurol. (2019) 10:194. doi: 10.3389/fneur.2019.00194
35. Howells, T, Smielewski, P, Donnelly, J, Czosnyka, M, Hutchinson, PJA, Menon, DK, et al. Optimal cerebral perfusion pressure in centers with different treatment protocols. Crit Care Med. (2018) 46:e235–41. doi: 10.1097/CCM.0000000000002930
36. Leach, MR, and Shutter, LA. How much oxygen for the injured brain – can invasive parenchymal catheters help? Curr Opin Crit Care. (2021) 27:95–102. doi: 10.1097/MCC.0000000000000810
37. Jaeger, M, Schuhmann, MU, Soehle, M, and Meixensberger, J. Continuous assessment of cerebrovascular autoregulation after traumatic brain injury using brain tissue oxygen pressure reactivity*. Crit Care Med. (2006) 34:1783–8. doi: 10.1097/01.CCM.0000218413.51546.9E
38. Salem, MK, Butt, HZ, Watts, APW, Sayers, RD, Bown, MJ, and Naylor, AR. Spontaneous cerebral embolisation in asymptomatic and recently symptomatic patients with TIA/minor stroke. Eur J Vasc Endovasc Surg. (2011) 41:720–5. doi: 10.1016/j.ejvs.2011.01.013
39. Chan, KH, Dearden, NM, Miller, JD, Midgley, S, and Piper, IR. Transcranial doppler waveform differences in hyperemic and nonhyperemic patients after severe head injury. Surg Neurol. (1992) 38:433–6. doi: 10.1016/0090-3019(92)90111-Y
40. Rabinstein, AA, Friedman, JA, Weigand, SD, McClelland, RL, Fulgham, JR, Manno, EM, et al. Predictors of cerebral infarction in aneurysmal subarachnoid hemorrhage. Stroke. (2004) 35:1862–6. doi: 10.1161/01.STR.0000133132.76983.8e
41. Zuromskis, T, Wetterholm, R, Lindqvist, JF, Svedlund, S, Sixt, C, Jatuzis, D, et al. Prevalence of micro-emboli in symptomatic high grade carotid artery disease: a transcranial Doppler study. Eur J Vasc Endovasc Surg. (2008) 35:534–40. doi: 10.1016/j.ejvs.2008.01.001
42. Zeiler, FA, Czosnyka, M, and Smielewski, P. Optimal cerebral perfusion pressure via transcranial Doppler in TBI: application of robotic technology. Acta Neurochir. (2018) 160:2149–57. doi: 10.1007/s00701-018-3687-5
43. Marion, DW, Darby, J, and Yonas, H. Acute regional cerebral blood flow changes caused by severe head injuries. J Neurosurg. (1991) 74:407–14. doi: 10.3171/jns.1991.74.3.0407
44. Lang, EW, Lagopoulos, J, Griffith, J, Yip, K, Mudaliar, Y, Mehdorn, HM, et al. Noninvasive cerebrovascular autoregulation assessment in traumatic brain injury: validation and utility. J Neurotrauma. (2003) 20:69–75. doi: 10.1089/08977150360517191
45. Zeiler, FA, Donnelly, J, Menon, DK, Smielewski, P, Zweifel, C, Brady, K, et al. Continuous autoregulatory indices derived from multi-modal monitoring: each one is not like the other. J Neurotrauma. (2017) 34:3070–80. doi: 10.1089/neu.2017.5129
46. Tas, J, Beqiri, E, van Kaam, RC, Czosnyka, M, Donnelly, J, Haeren, RH, et al. Targeting autoregulation-guided cerebral perfusion pressure after traumatic brain injury (COGiTATE): a feasibility randomized controlled clinical trial. J Neurotrauma. (2021) 38:2790–800. doi: 10.1089/neu.2021.0197
47. Petkus, V, Preiksaitis, A, Chaleckas, E, Chomskis, R, Zubaviciute, E, Vosylius, S, et al. Optimal cerebral perfusion pressure: targeted treatment for severe traumatic brain injury. J Neurotrauma. (2020) 37:389–96. doi: 10.1089/neu.2019.6551
48. Zeiler, FA, Cardim, D, Donnelly, J, Menon, DK, Czosnyka, M, and Smielewski, P. Transcranial Doppler systolic flow index and ICP-derived cerebrovascular reactivity indices in traumatic brain injury. J Neurotrauma. (2018) 35:314–22. doi: 10.1089/neu.2017.5364
49. Girouard, H, and Iadecola, C. Neurovascular coupling in the normal brain and in hypertension, stroke, and Alzheimer disease. J Appl Physiol. (2006) 100:328–35. doi: 10.1152/japplphysiol.00966.2005
50. Phillips, AA, Chan, FH, Zheng, MMZ, Krassioukov, AV, and Ainslie, PN. Neurovascular coupling in humans: physiology, methodological advances and clinical implications. J Cereb Blood Flow Metab. (2016) 36:647–64. doi: 10.1177/0271678X15617954
51. Pinti, P, Siddiqui, MF, Levy, AD, Jones, EJH, and Tachtsidis, I. An analysis framework for the integration of broadband NIRS and EEG to assess neurovascular and neurometabolic coupling. Sci Rep. (2021) 11:3977. doi: 10.1038/s41598-021-83420-9
52. Beishon, LC, and Minhas, JS. Cerebral autoregulation and neurovascular coupling in acute and chronic stroke. Front Neurol. (2021) 12:720770. doi: 10.3389/fneur.2021.720770
53. Junejo, RT, Braz, ID, Lucas, SJ, van Lieshout, JJ, Phillips, AA, Lip, GY, et al. Neurovascular coupling and cerebral autoregulation in atrial fibrillation. J Cereb Blood Flow Metab. (2020) 40:1647–57. doi: 10.1177/0271678X19870770
54. Balbi, M, Vega, MJ, Lourbopoulos, A, Terpolilli, NA, and Plesnila, N. Long-term impairment of neurovascular coupling following experimental subarachnoid hemorrhage. J Cereb Blood Flow Metab. (2020) 40:1193–202. doi: 10.1177/0271678X19863021
55. Salinet, AS, Silva, NC, Caldas, J, de Azevedo, DS, de Lima-Oliveira, M, Nogueira, RC, et al. Impaired cerebral autoregulation and neurovascular coupling in middle cerebral artery stroke: influence of severity? J Cereb Blood Flow Metab. (2019) 39:2277–85. doi: 10.1177/0271678X18794835
56. Salinet, ASM, Haunton, VJ, Panerai, RB, and Robinson, TG. A systematic review of cerebral hemodynamic responses to neural activation following stroke. J Neurol. (2013) 260:2715–21. doi: 10.1007/s00415-013-6836-z
57. Connolly, M, Vespa, P, Pouratian, N, Gonzalez, NR, and Hu, X. Characterization of the relationship between intracranial pressure and electroencephalographic monitoring in burst-suppressed patients. Neurocrit Care. (2015) 22:212–20. doi: 10.1007/s12028-014-0059-8
58. Connolly, M, Liou, R, Vespa, P, and Hu, X. Identification of an intracranial pressure (ICP) response function from continuously acquired electroencephalographic and ICP signals in burst-suppressed patients In: BT Ang, editor. Intracranial pressure and brain monitoring XV, vol. 122. Cham: Springer International Publishing (2016). 225–8. (Acta Neurochirurgica Supplement)
59. Sanz-García, A, Pérez-Romero, M, Pastor, J, Sola, RG, Vega-Zelaya, L, Monasterio, F, et al. Identifying causal relationships between EEG activity and intracranial pressure changes in neurocritical care patients. J Neural Eng. (2018) 15:066029. doi: 10.1088/1741-2552/aadeea
60. Yang, J, Ruesch, A, Schmitt, S, Smith, MA, and Kainerstorfer, JM. Correlation of EEG with Intercranial pressure and cerebral hemodynamics during burst-Supression. In: Biophotonics congress: biomedical optics congress 2018 (Microscopy/Translational/Brain/OTS). Hollywood, Florida: OSA. (2018). JTu3A.40.
61. Wu, D, Liu, X, Gadhoumi, K, Pu, Y, Hemphill, JC, Zhang, Z, et al. Causal relationship between neuronal activity and cerebral hemodynamics in patients with ischemic stroke. J Neural Eng. (2020) 17:026006. doi: 10.1088/1741-2552/ab75af
62. Othman, MH, Bhattacharya, M, Møller, K, Kjeldsen, S, Grand, J, Kjaergaard, J, et al. Resting-state NIRS–EEG in unresponsive patients with acute brain injury: a proof-of-concept study. Neurocrit Care. (2021) 34:31–44. doi: 10.1007/s12028-020-00971-x
63. Lundberg, N, Troupp, H, and Lorin, H. Continuous recording of the ventricular-fluid pressure in patients with severe acute traumatic brain injury: a preliminary report. J Neurosurg. (1965) 22:581–90. doi: 10.3171/jns.1965.22.6.0581
64. Åkerlund, CA, Donnelly, J, Zeiler, FA, Helbok, R, Holst, A, Cabeleira, M, et al. Impact of duration and magnitude of raised intracranial pressure on outcome after severe traumatic brain injury: a CENTER-TBI high-resolution group study. PLoS ONE. (2020) 15:e0243427. doi: 10.1371/journal.pone.0243427
65. Zeiler, FA, Ercole, A, Cabeleira, M, Stocchetti, N, Hutchinson, PJ, Smielewski, P, et al. Descriptive analysis of low versus elevated intracranial pressure on cerebral physiology in adult traumatic brain injury: a CENTER-TBI exploratory study. Acta Neurochir. (2020) 162:2695–706. doi: 10.1007/s00701-020-04485-5
66. Miller, JD, Becker, DP, Ward, JD, Sullivan, HG, Adams, WE, and Rosner, MJ. Significance of intracranial hypertension in severe head injury. J Neurosurg. (1977) 47:503–16. doi: 10.3171/jns.1977.47.4.0503
67. Zacchetti, L, Magnoni, S, Di Corte, F, Zanier, ER, and Stocchetti, N. Accuracy of intracranial pressure monitoring: systematic review and meta-analysis. Crit Care. (2015) 19:420. doi: 10.1186/s13054-015-1137-9
68. Carney, N, Totten, AM, O’Reilly, C, Ullman, JS, Hawryluk, GWJ, Bell, MJ, et al. Guidelines for the management of severe traumatic brain injury, fourth edition. Neurosurgery. (2017) 80:6–15. doi: 10.1227/NEU.0000000000001432
69. Farahvar, A, Gerber, LM, Chiu, YL, Carney, N, Härtl, R, and Ghajar, J. Increased mortality in patients with severe traumatic brain injury treated without intracranial pressure monitoring: clinical article. JNS. (2012) 117:729–34. doi: 10.3171/2012.7.JNS111816
70. Gerber, LM, Chiu, YL, Carney, N, Härtl, R, and Ghajar, J. Marked reduction in mortality in patients with severe traumatic brain injury: clinical article. JNS. (2013) 119:1583–90. doi: 10.3171/2013.8.JNS13276
71. Robba, C, Graziano, F, Rebora, P, Elli, F, Giussani, C, Oddo, M, et al. Intracranial pressure monitoring in the intensive care unit: an international prospective observational stud Y on iNtrAcranial pre Ssur E in intensive care (SYNAPSE-ICU). Lancet Neurol. (2021) 20:548–58. doi: 10.1016/S1474-4422(21)00138-1
72. Marmarou, A, Anderson, RL, Ward, JD, Choi, SC, Young, HF, Eisenberg, HM, et al. Impact of ICP instability and hypotension on outcome in patients with severe head trauma. J Neurosurg. (1991) 75:S59–66. doi: 10.3171/sup.1991.75.1s.0s59
73. Balestreri, M, Czosnyka, M, Hutchinson, P, Steiner, LA, Hiler, M, Smielewski, P, et al. Impact of intracranial pressure and cerebral perfusion pressure on severe disability and mortality after head injury. NCC. (2006) 4:008–13. doi: 10.1385/NCC:4:1:008
74. Bratton, SL, Chestnut, RM, Ghajar, J, McConnell Hammond, FF, Harris, OA, Hartl, R, et al. VIII. Intracranial pressure thresholds. J Neurotrauma. (2007) 24:S-55–8. doi: 10.1089/neu.2007.9988
75. Hawryluk, GWJ, Nielson, JL, Huie, JR, Zimmermann, L, Saigal, R, Ding, Q, et al. Analysis of normal high-frequency intracranial pressure values and treatment threshold in neurocritical care patients: insights into normal values and a potential treatment threshold. JAMA Neurol. (2020) 77:1150. doi: 10.1001/jamaneurol.2020.1310
76. Chesnut, RM, Temkin, N, Carney, N, Dikmen, S, Rondina, C, Videtta, W, et al. A trial of intracranial-pressure monitoring in traumatic brain injury. N Engl J Med. (2012) 367:2471–81. doi: 10.1056/NEJMoa1207363
77. Vik, A, Nag, T, Fredriksli, OA, Skandsen, T, Moen, KG, Schirmer-Mikalsen, K, et al. Relationship of “dose” of intracranial hypertension to outcome in severe traumatic brain injury: clinical article. JNS. (2008) 109:678–84. doi: 10.3171/JNS/2008/109/10/0678
78. Lee, HJ, Kim, H, Kim, YT, Won, K, Czosnyka, M, and Kim, DJ. Prediction of life-threatening intracranial hypertension during the acute phase of traumatic brain injury using machine learning. IEEE J Biomed Health Inform. (2021) 25:3967–76. doi: 10.1109/JBHI.2021.3085881
79. Robertson, C, Valadka, A, Hannay, H, Contant, C, Gopinath, S, Cormio, M, et al. Prevention of secondary ischemic insults after severe head injury. Crit Care Med. 27:2086–95. doi: 10.1097/00003246-199910000-00002
80. Eker, C, Asgeirsson, B, Grande, P, Schalen, W, and Nordstrom, C. Improved outcome after severe head injury with a new therapy based on principles for brain volume regulation and preserved microcirculation. Crit Care Med. (1998) 26:1881–6. doi: 10.1097/00003246-199811000-00033
81. Depreitere, B, Güiza, F, Van den Berghe, G, Schuhmann, MU, Maier, G, Piper, I, et al. Pressure autoregulation monitoring and cerebral perfusion pressure target recommendation in patients with severe traumatic brain injury based on minute-by-minute monitoring data: clinical article. JNS. (2014) 120:1451–7. doi: 10.3171/2014.3.JNS131500
82. Zeiler, FA, Mathieu, F, Monteiro, M, Glocker, B, Ercole, A, Beqiri, E, et al. Diffuse intracranial injury patterns are associated with impaired cerebrovascular reactivity in adult traumatic brain injury: a CENTER-TBI validation study. J Neurotrauma. (2020) 37:1597–608. doi: 10.1089/neu.2019.6959
83. Steiner, LA. Cerebrovascular pressure reactivity is related to global cerebral oxygen metabolism after head injury. J Neurol Neurosurg Psychiatry. (2003) 74:765–70. doi: 10.1136/jnnp.74.6.765
84. Mathieu, F, Zeiler, FA, Whitehouse, DP, Das, T, Ercole, A, Smielewski, P, et al. Relationship between measures of cerebrovascular reactivity and intracranial lesion progression in acute TBI patients: an exploratory analysis. Neurocrit Care. (2020) 32:373–82. doi: 10.1007/s12028-019-00885-3
85. Lazaridis, C, DeSantis, SM, Smielewski, P, Menon, DK, Hutchinson, P, Pickard, JD, et al. Patient-specific thresholds of intracranial pressure in severe traumatic brain injury: clinical article. JNS. (2014) 120:893–900. doi: 10.3171/2014.1.JNS131292
86. Zeiler, FA, Ercole, A, Cabeleira, M, Beqiri, E, Zoerle, T, Carbonara, M, et al. Patient-specific ICP epidemiologic thresholds in adult traumatic brain injury: a CENTER-TBI validation study. Journal of Neurosurgical Anesthesiology. (2021) 33:28–38.
87. Svedung Wettervik, T, Howells, T, Hillered, L, Rostami, E, Lewén, A, and Enblad, P. Autoregulatory or fixed cerebral perfusion pressure targets in traumatic brain injury: determining which is better in an energy metabolic perspective. J Neurotrauma. (2021) 38:1969–78. doi: 10.1089/neu.2020.7290
88. Jaeger, M, Dengl, M, Meixensberger, J, and Schuhmann, MU. Effects of cerebrovascular pressure reactivity-guided optimization of cerebral perfusion pressure on brain tissue oxygenation after traumatic brain injury*. Crit Care Med. (2010) 38:1343–7. doi: 10.1097/CCM.0b013e3181d45530
89. Kramer, AH, Couillard, PL, Zygun, DA, Aries, MJ, and Gallagher, CN. Continuous assessment of “optimal” cerebral perfusion pressure in traumatic brain injury: a cohort study of feasibility, reliability, and relation to outcome. Neurocrit Care. (2019) 30:51–61. doi: 10.1007/s12028-018-0570-4
90. Needham, E, McFadyen, C, Newcombe, V, Synnot, AJ, Czosnyka, M, and Menon, D. Cerebral perfusion pressure targets individualized to pressure-reactivity index in moderate to severe traumatic brain injury: a systematic review. J Neurotrauma. (2017) 34:963–70. doi: 10.1089/neu.2016.4450
91. Weersink, CSA, Aries, MJH, Dias, C, Liu, MX, Kolias, AG, Donnelly, J, et al. Clinical and physiological events that contribute to the success rate of finding “optimal” cerebral perfusion pressure in severe brain trauma patients. Crit Care Med. (2015) 43:1952–63. doi: 10.1097/CCM.0000000000001165
92. Zeiler, FA, Donnelly, J, Calviello, L, Smielewski, P, Menon, DK, and Czosnyka, M. Pressure autoregulation measurement techniques in adult traumatic brain injury, part II: a scoping review of continuous methods. J Neurotrauma. (2017) 34:3224–37. doi: 10.1089/neu.2017.5086
93. Riemann, L, Beqiri, E, Smielewski, P, Czosnyka, M, Stocchetti, N, Sakowitz, O, et al. Low-resolution pressure reactivity index and its derived optimal cerebral perfusion pressure in adult traumatic brain injury: a CENTER-TBI study. Crit Care. (2020) 24:266. doi: 10.1186/s13054-020-02974-8
94. Mainali, S, Cardim, D, Sarwal, A, Merck, LH, Yeatts, SD, Czosnyka, M, et al. Prolonged automated robotic TCD monitoring in acute severe TBI: study design and rationale. Neurocrit Care. (2022) 37:267–75. doi: 10.1007/s12028-022-01483-6
95. Gouvea Bogossian, E, Diaferia, D, Ndieugnou Djangang, N, Menozzi, M, Vincent, JL, Talamonti, M, et al. Brain tissue oxygenation guided therapy and outcome in non-traumatic subarachnoid hemorrhage. Sci Rep. (2021) 11:16235. doi: 10.1038/s41598-021-95602-6
96. Sarrafzadeh, A, Kiening, K, Callsen, TA, and Unterberg, A. Metabolic changes during impending and manifest cerebral hypoxia in traumatic brain injury. Br J Neurosurg. (2003) 17:340–6. doi: 10.1080/02688690310001601234
97. Chang, JJJ, Youn, TS, Benson, D, Mattick, H, Andrade, N, Harper, CR, et al. Physiologic and functional outcome correlates of brain tissue hypoxia in traumatic brain injury*. Crit Care Med. (2009) 37:283–90. doi: 10.1097/CCM.0b013e318192fbd7
98. Veenith, TV, Carter, EL, Geeraerts, T, Grossac, J, Newcombe, VFJ, Outtrim, J, et al. Pathophysiologic mechanisms of cerebral ischemia and diffusion hypoxia in traumatic brain injury. JAMA Neurol. (2016) 73:542. doi: 10.1001/jamaneurol.2016.0091
99. Spiotta, AM, Stiefel, MF, Gracias, VH, Garuffe, AM, Kofke, WA, Maloney-Wilensky, E, et al. Brain tissue oxygen–directed management and outcome in patients with severe traumatic brain injury: clinical article. JNS. (2010) 113:571–80. doi: 10.3171/2010.1.JNS09506
100. Rosenthal, G, Hemphill, JC, Sorani, M, Martin, C, Morabito, D, Obrist, WD, et al. Brain tissue oxygen tension is more indicative of oxygen diffusion than oxygen delivery and metabolism in patients with traumatic brain injury*. Crit Care Med. (2008) 36:1917–24. doi: 10.1097/CCM.0b013e3181743d77
101. Stocchetti, N, Magnoni, S, and Zanier, ER. My paper 20 years later: cerebral venous oxygen saturation studied with bilateral samples in the internal jugular veins. Intensive Care Med. (2015) 41:412–7. doi: 10.1007/s00134-015-3650-3
102. Goyal, K, Khandelwal, A, and Kedia, S. Multimodal Neuromonitoring: current scenario in neurocritical care. J Neuroanaesthesiology Crit Care. (2019) 06:062–71. doi: 10.1055/s-0039-1692863
103. Oddo, M, and Bösel, J, the Participants in the International Multidisciplinary Consensus Conference on Multimodality Monitoring. Monitoring of brain and systemic oxygenation in neurocritical care patients. Neurocrit Care. (2014) 21:103–20. doi: 10.1007/s12028-014-0024-6
104. Pennings, FA, Schuurman, PR, van den Munckhof, P, and Bouma, GJ. Brain tissue oxygen pressure monitoring in awake patients during functional neurosurgery: the assessment of normal values. J Neurotrauma. (2008) 25:1173–7. doi: 10.1089/neu.2007.0402
105. Longhi, L, Pagan, F, Valeriani, V, Magnoni, S, Zanier, ER, Conte, V, et al. Monitoring brain tissue oxygen tension in brain-injured patients reveals hypoxic episodes in normal-appearing and in peri-focal tissue. Intensive Care Med. (2007) 33:2136–42. doi: 10.1007/s00134-007-0845-2
106. Ponce, LL, Pillai, S, Cruz, J, Li, X, Julia, H, Gopinath, S, et al. Position of probe determines prognostic information of brain tissue PO2 in severe traumatic brain injury. Neurosurgery. (2012) 70:1492–503. doi: 10.1227/NEU.0b013e31824ce933
107. Hawryluk, GWJ, Phan, N, Ferguson, AR, Morabito, D, Derugin, N, Stewart, CL, et al. Brain tissue oxygen tension and its response to physiological manipulations: influence of distance from injury site in a swine model of traumatic brain injury. JNS. (2016) 125:1217–28. doi: 10.3171/2015.7.JNS15809
108. Okonkwo, DO, Shutter, LA, Moore, C, Temkin, NR, Puccio, AM, Madden, CJ, et al. Brain oxygen optimization in severe traumatic brain injury phase-II: a phase II randomized trial*. Crit Care Med. (2017) 45:1907–14. doi: 10.1097/CCM.0000000000002619
109. Meixensberger, J. Brain tissue oxygen guided treatment supplementing ICP/CPP therapy after traumatic brain injury. J Neurol Neurosurg Psychiatry. (2003) 74:760–4. doi: 10.1136/jnnp.74.6.760
110. Zeiler, FA, Beqiri, E, Cabeleira, M, Hutchinson, PJ, Stocchetti, N, Menon, DK, et al. Brain tissue oxygen and cerebrovascular reactivity in traumatic brain injury: a collaborative European neuro trauma effectiveness research in traumatic brain injury exploratory analysis of insult burden. J Neurotrauma. (2020) 37:1854–63. doi: 10.1089/neu.2020.7024
111. Megjhani, M, Weiss, M, Ford, J, Terilli, K, Kastenholz, N, Nametz, D, et al. Optimal cerebral perfusion pressure and brain tissue oxygen in aneurysmal subarachnoid hemorrhage. Stroke. (2023) 54:189–97. doi: 10.1161/STROKEAHA.122.040339
112. Quintard, H, Patet, C, Suys, T, Marques-Vidal, P, and Oddo, M. Normobaric hyperoxia is associated with increased cerebral excitotoxicity after severe traumatic brain injury. Neurocrit Care. (2015) 22:243–50. doi: 10.1007/s12028-014-0062-0
113. Bohman, LE, Heuer, GG, Macyszyn, L, Maloney-Wilensky, E, Frangos, S, Le Roux, PD, et al. Medical management of compromised brain oxygen in patients with severe traumatic brain injury. Neurocrit Care. (2011) 14:361–9. doi: 10.1007/s12028-011-9526-7
114. O’Driscoll, BR, Howard, LS, Earis, J, and Mak, V. British Thoracic Society guideline for oxygen use in adults in healthcare and emergency settings. BMJ Open Resp Res. (2017) 4:e000170. doi: 10.1136/bmjresp-2016-000170
115. Dellazizzo, L, Demers, SP, Charbonney, E, Williams, V, Serri, K, Albert, M, et al. Minimal PaO2 threshold after traumatic brain injury and clinical utility of a novel brain oxygenation ratio. J Neurosurg. (2019) 131:1639–47. doi: 10.3171/2018.5.JNS18651
116. Chesnut, R, Aguilera, S, Buki, A, Bulger, E, Citerio, G, Cooper, DJ, et al. A management algorithm for adult patients with both brain oxygen and intracranial pressure monitoring: the Seattle international severe traumatic brain injury consensus conference (SIBICC). Intensive Care Med. (2020) 46:919–29. doi: 10.1007/s00134-019-05900-x
117. Oddo, M, Levine, JM, Mackenzie, L, Frangos, S, Feihl, F, Kasner, SE, et al. Brain hypoxia is associated with short-term outcome after severe traumatic brain injury independently of intracranial hypertension and low cerebral perfusion pressure. Neurosurgery. (2011) 69:1037–45. doi: 10.1227/NEU.0b013e3182287ca7
118. Ulrich, CT, Fung, C, Vatter, H, Setzer, M, Gueresir, E, Seifert, V, et al. Occurrence of vasospasm and infarction in relation to a focal monitoring sensor in patients after SAH: placing a bet when placing a probe? PLoS One. (2013) 8:e62754. doi: 10.1371/journal.pone.0062754
119. Veldeman, M, Albanna, W, Weiss, M, Park, S, Hoellig, A, Clusmann, H, et al. Invasive multimodal Neuromonitoring in aneurysmal subarachnoid hemorrhage: a systematic review. Stroke. (2021) 52:3624–32. doi: 10.1161/STROKEAHA.121.034633
120. Narotam, PK, Morrison, JF, and Nathoo, N. Brain tissue oxygen monitoring in traumatic brain injury and major trauma: outcome analysis of a brain tissue oxygen–directed therapy: clinical article. JNS. (2009) 111:672–82. doi: 10.3171/2009.4.JNS081150
121. Xie, Q, Wu, HB, Yan, YF, Liu, M, and Wang, ES. Mortality and outcome comparison between brain tissue oxygen combined with intracranial pressure/cerebral perfusion pressure–guided therapy and intracranial pressure/cerebral perfusion pressure–guided therapy in traumatic brain injury: a meta-analysis. World Neurosurg. (2017) 100:118–27. doi: 10.1016/j.wneu.2016.12.097
122. Komisarow, JM, Toro, C, Curley, J, Mills, B, Cho, C, Simo, GM, et al. Utilization of brain tissue oxygenation monitoring and association with mortality following severe traumatic brain injury. Neurocrit Care. (2022) 36:350–6. doi: 10.1007/s12028-021-01394-y
123. Nangunoori, R, Maloney-Wilensky, E, Stiefel, M, Park, S, Andrew Kofke, W, Levine, JM, et al. Brain tissue oxygen-based therapy and outcome after severe traumatic brain injury: a systematic literature review. Neurocrit Care. (2012) 17:131–8. doi: 10.1007/s12028-011-9621-9
124. Lin, CM, Lin, MC, Huang, SJ, Chang, CK, Chao, DP, Lui, TN, et al. A prospective randomized study of brain tissue oxygen pressure-guided management in moderate and severe traumatic brain injury patients. Bio Med Res Int. (2015) 2015:1–8. doi: 10.1155/2015/529580
125. Zeiler, FA, Cabeleira, M, Hutchinson, PJ, Stocchetti, N, Czosnyka, M, Smielewski, P, et al. Evaluation of the relationship between slow-waves of intracranial pressure, mean arterial pressure and brain tissue oxygen in TBI: a CENTER-TBI exploratory analysis. J Clin Monit Comput. (2021) 35:711–22. doi: 10.1007/s10877-020-00527-6
126. Radolovich, DK, Czosnyka, M, Timofeev, I, Lavinio, A, Hutchinson, P, Gupta, A, et al. Reactivity of brain tissue oxygen to change in cerebral perfusion pressure in head injured patients. Neurocrit Care. (2009) 10:274–9. doi: 10.1007/s12028-009-9190-3
127. Andresen, M, Donnelly, J, Aries, M, Juhler, M, Menon, D, Hutchinson, P, et al. Further controversies about brain tissue oxygenation pressure-reactivity after traumatic brain injury. Neurocrit Care. (2018) 28:162–8. doi: 10.1007/s12028-017-0438-z
128. Aaslid, R, Markwalder, TM, and Nornes, H. Noninvasive transcranial Doppler ultrasound recording of flow velocity in basal cerebral arteries. J Neurosurg. (1982) 57:769–74. doi: 10.3171/jns.1982.57.6.0769
129. Alexandrov, AV. Cerebrovascular ultrasound in stroke prevention and treatment. 2nd ed. Chichester, West Sussex: Wiley-Blackwell (2011). 280 p.
130. Rodríguez, CN, Baracchini, C, Mejia-Mantilla, JH, Czosnyka, M, Suarez, JI, Csiba, L, et al. Neurosonology in critical care: monitoring the neurological impact of the critical pathology. Cham: Springer International Publishing (2022).
131. Aggarwal, S, Brooks, DM, Kang, Y, Linden, PK, and Patzer, JF. Noninvasive monitoring of cerebral perfusion pressure in patients with acute liver failure using transcranial doppler ultrasonography. Liver Transpl. (2008) 14:1048–57. doi: 10.1002/lt.21499
132. Sørensen, PT, Nyborg, G, Lorentsen, T, Olasveengen, TM, Langerud, AK, Aarhus, M, et al. Vasospasm surveillance by a simplified transcranial Doppler protocol in traumatic brain injury. World Neurosurg. (2022) 164:e318–25. doi: 10.1016/j.wneu.2022.04.108
133. Kumar, G, Shahripour, RB, and Harrigan, MR. Vasospasm on transcranial Doppler is predictive of delayed cerebral ischemia in aneurysmal subarachnoid hemorrhage: a systematic review and meta-analysis. JNS. (2016) 124:1257–64. doi: 10.3171/2015.4.JNS15428
134. Lysakowski, C, Walder, B, Costanza, MC, and Tramèr, MR. Transcranial Doppler versus angiography in patients with vasospasm due to a ruptured cerebral aneurysm: a systematic review. Stroke. (2001) 32:2292–8. doi: 10.1161/hs1001.097108
135. Suarez, JI, Qureshi, AI, Yahia, AB, Parekh, PD, Tamargo, RJ, Williams, MA, et al. Symptomatic vasospasm diagnosis after subarachnoid hemorrhage: evaluation of transcranial Doppler ultrasound and cerebral angiography as related to compromised vascular distribution. Crit Care Med. (2002) 30:1348–55. doi: 10.1097/00003246-200206000-00035
136. Vora, Y, Suarez-Almazor, M, Steinke, D, Martin, M, and Findlay, JM. Role of transcranial Doppler monitoring in the diagnosis of cerebral vasospasm after subarachnoid hemorrhage. Neurosurgery. (1999) 44:1237–48.
137. Mascia, L, Fedorko, L, ter Brugge, K, Filippini, C, Pizzio, M, Ranieri, VM, et al. The accuracy of transcranial Doppler to detect vasospasm in patients with aneurysmal subarachnoid hemorrhage. Intensive Care Med. (2003) 29:1088–94. doi: 10.1007/s00134-003-1780-5
138. Snider, SB, Migdady, I, LaRose, SL, Mckeown, ME, Regenhardt, RW, Lai, PMR, et al. Transcranial-Doppler-measured vasospasm severity is associated with delayed cerebral infarction after subarachnoid hemorrhage. Neurocrit Care. (2022) 36:815–21. doi: 10.1007/s12028-021-01382-2
139. Markus, HS, King, A, Shipley, M, Topakian, R, Cullinane, M, Reihill, S, et al. Asymptomatic embolisation for prediction of stroke in the asymptomatic carotid emboli study (ACES): a prospective observational study. Lancet Neurol. (2010) 9:663–71. doi: 10.1016/S1474-4422(10)70120-4
140. Bonow, RH, Witt, CE, Mosher, BP, Mossa-Basha, M, Vavilala, MS, Rivara, FP, et al. Transcranial Doppler microemboli monitoring for stroke risk stratification in blunt cerebrovascular injury. Crit Care Med. (2017) 45:e1011–7. doi: 10.1097/CCM.0000000000002549
141. Khan, KS, and Wiersema, UF. Transcranial Doppler waveform changes due to increased cerebrovascular resistance and raised intracranial pressure in a patient with cirrhosis: a difference in shapes, not in numbers. J Clin Ultrasound. (2020) 48:59–63. doi: 10.1002/jcu.22799
142. Calviello, LA, Zeiler, FA, Donnelly, J, Czigler, A, Lavinio, A, Hutchinson, PJ, et al. Cerebrovascular consequences of elevated intracranial pressure after traumatic brain injury In: B Depreitere, G Meyfroidt, and F Güiza, editors. Intracranial pressure and Neuromonitoring XVII, vol. 131. Cham: Springer International Publishing (2021). 43–8 (Acta Neurochirurgica Supplement).
143. Greer, DM, Shemie, SD, Lewis, A, Torrance, S, Varelas, P, Goldenberg, FD, et al. Determination of brain death/death by neurologic criteria: the world brain death project. JAMA. (2020) 324:1078–97. doi: 10.1001/jama.2020.11586
144. Marinoni, M, Ginanneschi, A, Forleo, P, and Amaducci, L. Technical limits in transcranial DOPPLER recording: inadequate acoustic windows. Ultrasound Med Biol. (1997) 23:1275–7. doi: 10.1016/s0301-5629(97)00077-x
145. Zeiler, FA, Smielewski, P, Stevens, A, Czosnyka, M, Menon, DK, and Ercole, A. Non-invasive pressure reactivity index using Doppler systolic flow parameters: a pilot analysis. J Neurotrauma. (2019) 36:713–20. doi: 10.1089/neu.2018.5987
146. Voulgaris, S, Partheni, M, Kaliora, H, Haftouras, N, Pessach, I, and Polyzoidis, K. Early cerebral monitoring using the transcranial Doppler pulsatility index in patients with severe brain trauma. Med Sci Monit. (2005) 11:49–52.
147. Figaji, AA, Zwane, E, Fieggen, AG, Siesjo, P, and Peter, JC. Transcranial Doppler pulsatility index is not a reliable indicator of intracranial pressure in children with severe traumatic brain injury. Surg Neurol. (2009) 72:389–94. doi: 10.1016/j.surneu.2009.02.012
148. de Riva, N, Budohoski, KP, Smielewski, P, Kasprowicz, M, Zweifel, C, Steiner, LA, et al. Transcranial Doppler pulsatility index: what it is and what it Isn’t. Neurocrit Care. (2012) 17:58–66. doi: 10.1007/s12028-012-9672-6
149. Brandi, G, Béchir, M, Sailer, S, Haberthür, C, Stocker, R, and Stover, JF. Transcranial color-coded duplex sonography allows to assess cerebral perfusion pressure noninvasively following severe traumatic brain injury. Acta Neurochir. (2010) 152:965–72. doi: 10.1007/s00701-010-0643-4
150. Zweifel, C, Czosnyka, M, Carrera, E, de Riva, N, Pickard, JD, and Smielewski, P. Reliability of the blood flow velocity pulsatility index for assessment of intracranial and cerebral perfusion pressures in head-injured patients. Neurosurgery. (2012) 71:853–61. doi: 10.1227/NEU.0b013e3182675b42
151. Jaffres, P, Brun, J, Declety, P, Bosson, JL, Fauvage, B, Schleiermacher, A, et al. Transcranial Doppler to detect on admission patients at risk for neurological deterioration following mild and moderate brain trauma. Intensive Care Med. (2005) 31:785–90. doi: 10.1007/s00134-005-2630-4
152. Bouzat, P, Almeras, L, Manhes, P, Sanders, L, Levrat, A, David, JS, et al. Transcranial Doppler to predict neurologic outcome after mild to moderate traumatic brain injury. Anesthesiology. (2016) 125:346–54. doi: 10.1097/ALN.0000000000001165
153. Moreno, JA, Mesalles, E, Gener, J, Tomasa, A, Ley, A, Roca, J, et al. Evaluating the outcome of severe head injury with transcranial Doppler ultrasonography. FOC. (2000) 8:1–7. doi: 10.3171/foc.2000.8.1.1702
154. Trofimov, A, Kopylov, A, Dobrzeniecki, M, Sheludyakov, A, Martynov, D, Trofimova, K, et al. Comparative analysis of simultaneous transcranial Doppler and perfusion computed tomography for cerebral perfusion evaluation in patients with traumatic brain injury In: PD Ryu, LM JC, DK Harrison, and SS Lee, editors. Oxygen transport to tissue XLI, vol. 1232. Cham: Springer International Publishing (2020). 55–62 (Advances in Experimental Medicine and Biology).
155. van Santbrink, H, Schouten, JW, Steyerberg, EW, Avezaat, CJJ, and Maas, AIR. Serial transcranial Doppler measurements in traumatic brain injury with special focus on the early posttraumatic period. Acta Neurochir. (2002) 144:1141–9. doi: 10.1007/s00701-002-1012-8
156. Sokoloff, C, Williamson, D, Serri, K, Albert, M, Odier, C, Charbonney, E, et al. Clinical usefulness of transcranial Doppler as a screening tool for early cerebral hypoxic episodes in patients with moderate and severe traumatic brain injury. Neurocrit Care. (2020) 32:486–91. doi: 10.1007/s12028-019-00763-y
157. Trabold, F, Meyer, PG, Blanot, S, Carli, PA, and Orliaguet, GA. The prognostic value of transcranial Doppler studies in children with moderate and severe head injury. Intensive Care Med. (2004) 30:108–12. doi: 10.1007/s00134-003-2057-8
158. Calviello, LA, Cardim, D, Czosnyka, M, Preller, J, Smielewski, P, Siyal, A, et al. Feasibility of non-invasive neuromonitoring in general intensive care patients using a multi-parameter transcranial Doppler approach. J Clin Monit Comput. (2022) 36:1805–15. doi: 10.1007/s10877-022-00829-x
159. Vicenzini, E, Ricciardi, MC, Zuco, C, Sirimarco, G, Di Piero, V, and Lenzi, GL. Effects of a single mannitol bolus on cerebral hemodynamics in intracerebral hemorrhage: a transcranial Doppler study. Cerebrovasc Dis. (2011) 32:447–53. doi: 10.1159/000330639
160. Ract, C, Le Moigno, S, Bruder, N, and Vigué, B. Transcranial Doppler ultrasound goal-directed therapy for the early management of severe traumatic brain injury. Intensive Care Med. (2007) 33:645–51. doi: 10.1007/s00134-007-0558-6
161. Cardim, D, Robba, C, Bohdanowicz, M, Donnelly, J, Cabella, B, Liu, X, et al. Non-invasive monitoring of intracranial pressure using transcranial Doppler ultrasonography: is it possible? Neurocrit Care. (2016) 25:473–91. doi: 10.1007/s12028-016-0258-6
162. Varsos, GV, Budohoski, KP, Kolias, AG, Liu, X, Smielewski, P, Varsos, VG, et al. Relationship of vascular wall tension and autoregulation following traumatic brain injury. Neurocrit Care. (2014) 21:266–74. doi: 10.1007/s12028-014-9971-1
163. Bellner, J, Romner, B, Reinstrup, P, Kristiansson, KA, Ryding, E, and Brandt, L. Transcranial Doppler sonography pulsatility index (PI) reflects intracranial pressure (ICP). Surg Neurol. (2004) 62:45–51. doi: 10.1016/j.surneu.2003.12.007
164. Varsos, GV, Richards, H, Kasprowicz, M, Budohoski, KP, Brady, KM, Reinhard, M, et al. Critical closing pressure determined with a model of cerebrovascular impedance. J Cereb Blood Flow Metab. (2013) 33:235–43. doi: 10.1038/jcbfm.2012.161
165. Varsos, GV, Kolias, AG, Smielewski, P, Brady, KM, Varsos, VG, Hutchinson, PJ, et al. A noninvasive estimation of cerebral perfusion pressure using critical closing pressure. JNS. (2015) 123:638–48. doi: 10.3171/2014.10.JNS14613
166. Cardim, D, Robba, C, Donnelly, J, Bohdanowicz, M, Schmidt, B, Damian, M, et al. Prospective study on noninvasive assessment of intracranial pressure in traumatic brain-injured patients: comparison of four methods. J Neurotrauma. (2016) 33:792–802. doi: 10.1089/neu.2015.4134
167. Abecasis, F, Cardim, D, Czosnyka, M, Robba, C, and Agrawal, S. Transcranial Doppler as a non-invasive method to estimate cerebral perfusion pressure in children with severe traumatic brain injury. Childs Nerv Syst. (2020) 36:125–31. doi: 10.1007/s00381-019-04273-2
168. Rasulo, FA, Bertuetti, R, Robba, C, Lusenti, F, Cantoni, A, Bernini, M, et al. The accuracy of transcranial Doppler in excluding intracranial hypertension following acute brain injury: a multicenter prospective pilot study. Crit Care. (2017) 21:44. doi: 10.1186/s13054-017-1632-2
169. Rasulo, FA, Calza, S, Robba, C, Taccone, FS, Biasucci, DG, Badenes, R, et al. Transcranial Doppler as a screening test to exclude intracranial hypertension in brain-injured patients: the IMPRESSIT-2 prospective multicenter international study. Crit Care. (2022) 26:110. doi: 10.1186/s13054-022-03978-2
170. Cardim, D, Robba, C, Czosnyka, M, Savo, D, Mazeraud, A, Iaquaniello, C, et al. Noninvasive intracranial pressure estimation with transcranial Doppler: a prospective observational study. J Neurol Anesthesia. (2020) 32:349–53. doi: 10.1097/ANA.0000000000000622
171. O’Brien, NF, Lovett, ME, Chung, M, and Maa, T. Non-invasive estimation of cerebral perfusion pressure using transcranial Doppler ultrasonography in children with severe traumatic brain injury. Childs Nerv Syst. (2020) 36:2063–71. doi: 10.1007/s00381-020-04524-7
172. Robba, C, Cardim, D, Tajsic, T, Pietersen, J, Bulman, M, Donnelly, J, et al. Ultrasound non-invasive measurement of intracranial pressure in neurointensive care: a prospective observational study. PLoS Med. (2017) 14:e1002356. doi: 10.1371/journal.pmed.1002356
173. Robba, C, Pozzebon, S, Moro, B, Vincent, JL, Creteur, J, and Taccone, FS. Multimodal non-invasive assessment of intracranial hypertension: an observational study. Crit Care. (2020) 24:379. doi: 10.1186/s13054-020-03105-z
174. Budohoski, KP, Reinhard, M, Aries, MJH, Czosnyka, Z, Smielewski, P, Pickard, JD, et al. Monitoring cerebral autoregulation after head injury. Which component of transcranial Doppler flow velocity is optimal? Neurocrit Care. (2012) 17:211–8. doi: 10.1007/s12028-011-9572-1
175. Steiner, LA, Coles, JP, Johnston, AJ, Chatfield, DA, Smielewski, P, Fryer, TD, et al. Assessment of cerebrovascular autoregulation in head-injured patients: a validation study. Stroke. (2003) 34:2404–9. doi: 10.1161/01.STR.0000089014.59668.04
176. Budohoski, KP, Czosnyka, M, de Riva, N, Smielewski, P, Pickard, JD, Menon, DK, et al. The relationship between cerebral blood flow autoregulation and cerebrovascular pressure reactivity after traumatic brain injury. Neurosurgery. (2012) 71:652–61. doi: 10.1227/NEU.0b013e318260feb1
177. Zeiler, FA, Smielewski, P, Donnelly, J, Czosnyka, M, Menon, DK, and Ercole, A. Estimating pressure reactivity using noninvasive Doppler-based systolic flow index. J Neurotrauma. (2018) 35:1559–68. doi: 10.1089/neu.2017.5596
178. Schmidt, EA, Czosnyka, M, Steiner, LA, Balestreri, M, Smielewski, P, Piechnik, SK, et al. Asymmetry of pressure autoregulation after traumatic brain injury. J Neurosurg. (2003) 99:991–8. doi: 10.3171/jns.2003.99.6.0991
179. Reinhard, M, Rutsch, S, Lambeck, J, Wihler, C, Czosnyka, M, Weiller, C, et al. Dynamic cerebral autoregulation associates with infarct size and outcome after ischemic stroke: cerebral autoregulation in ischemic stroke. Acta Neurol Scand. (2012) 125:156–62. doi: 10.1111/j.1600-0404.2011.01515.x
180. Reinhard, M, Neunhoeffer, F, Gerds, TA, Niesen, WD, Buttler, KJ, Timmer, J, et al. Secondary decline of cerebral autoregulation is associated with worse outcome after intracerebral hemorrhage. Intensive Care Med. (2010) 36:264–71. doi: 10.1007/s00134-009-1698-7
181. Soehle, M, Czosnyka, M, Pickard, JD, and Kirkpatrick, PJ. Continuous assessment of cerebral autoregulation in subarachnoid hemorrhage. Anesth Analg. (2004) 98:1133–9. doi: 10.1213/01.ANE.0000111101.41190.99
182. Calviere, L, Nasr, N, Arnaud, C, Czosnyka, M, Viguier, A, Tissot, B, et al. Prediction of delayed cerebral ischemia after subarachnoid hemorrhage using cerebral blood flow velocities and cerebral autoregulation assessment. Neurocrit Care. (2015) 23:253–8. doi: 10.1007/s12028-015-0125-x
183. Gomez, A, Froese, L, Sainbhi, AS, Batson, C, and Zeiler, FA. Transcranial Doppler based cerebrovascular reactivity indices in adult traumatic brain injury: a scoping review of associations with patient oriented outcomes. Front Pharmacol. (2021) 12:690921. doi: 10.3389/fphar.2021.690921
184. Jöbsis, FF. Noninvasive, infrared monitoring of cerebral and myocardial oxygen sufficiency and circulatory parameters. Science. (1997) 198:1264–7. doi: 10.1126/science.929199
185. Weigl, W, Milej, D, Janusek, D, Wojtkiewicz, S, Sawosz, P, Kacprzak, M, et al. Application of optical methods in the monitoring of traumatic brain injury: a review. J Cereb Blood Flow Metab. (2016) 36:1825–43. doi: 10.1177/0271678X16667953
186. Vretzakis, G, Georgopoulou, S, Stamoulis, K, Stamatiou, G, Tsakiridis, K, Zarogoulidis, P, et al. Cerebral oximetry in cardiac anesthesia. J Thorac Dis. (2014):6. doi: 10.3978/j.issn.2072-1439.2013.10.22
187. Gomez, A, Sainbhi, AS, Froese, L, Batson, C, Alizadeh, A, Mendelson, AA, et al. Near infrared spectroscopy for high-temporal resolution cerebral Physiome characterization in TBI: a narrative review of techniques, applications, and future directions. Front Pharmacol. (2021) 12:719501. doi: 10.3389/fphar.2021.719501
188. Zweifel, C, Dias, C, Smielewski, P, and Czosnyka, M. Continuous time-domain monitoring of cerebral autoregulation in neurocritical care. Med Eng Phys. (2014) 36:638–45. doi: 10.1016/j.medengphy.2014.03.002
189. Collette, SL, Venema, AM, Eleveld, N, Absalom, AR, Scheeren, TW, Verhoeve, S, et al. Near-infrared spectroscopy monitoring during endovascular treatment for acute ischaemic stroke. Eur Stroke J. (2022) 7:384–92. doi: 10.1177/23969873221107824
190. Keller, E, Froehlich, J, Baumann, D, Böcklin, C, Sikorski, C, Oberle, M, et al. Detection of delayed cerebral ischemia (DCI) in subarachnoid Haemorrhage applying near-infrared spectroscopy: elimination of the Extracerebral signal by transcutaneous and Intraparenchymatous measurements in parallel In: J Fandino, S Marbacher, AR Fathi, C Muroi, and E Keller, editors. Neurovascular events after subarachnoid hemorrhage. Cham: Springer International Publishing (2015). 243–7.
191. Kirkpatrick, PJ, Smielewski, P, Czosnyka, M, Menon, DK, and Pickard, JD. Near-infrared spectroscopy use in patients with head injury. J Neurosurg. (1995) 83:963–70. doi: 10.3171/jns.1995.83.6.0963
192. Scheeren, TWL, Schober, P, and Schwarte, LA. Monitoring tissue oxygenation by near infrared spectroscopy (NIRS): background and current applications. J Clin Monit Comput. (2012) 26:279–87. doi: 10.1007/s10877-012-9348-y
193. Rivera-Lara, L, Zorrilla-Vaca, A, Geocadin, R, Ziai, W, Healy, R, Thompson, R, et al. Predictors of outcome with cerebral autoregulation monitoring: a systematic review and meta-analysis. Crit Care Med. (2017) 45:695–704. doi: 10.1097/CCM.0000000000002251
194. Dunham, C, Sosnowski, C, Porter, J, Siegal, J, Kohli, C, Dunham, C, et al. Correlation of noninvasive cerebral oximetry with cerebral perfusion in the severe head injured patient: a pilot study. J Trauma. (2002) 52:40–6. doi: 10.1097/00005373-200201000-00009
195. Al-Rawi, PG, and Kirkpatrick, PJ. Tissue oxygen index: thresholds for cerebral ischemia using near-infrared spectroscopy. Stroke. (2006) 37:2720–5. doi: 10.1161/01.STR.0000244807.99073.ae
196. Dunham, CM, Ransom, KJ, Flowers, LL, Siegal, JD, and Kohli, CM. Cerebral hypoxia in severely brain-injured patients is associated with admission Glasgow coma scale score, computed tomographic severity, cerebral perfusion pressure, and survival. J Trauma. (2004) 56:482–91. doi: 10.1097/01.ta.0000114537.52540.95
197. Vilkė, A, Bilskienė, D, Šaferis, V, Gedminas, M, Bieliauskaitė, D, Tamašauskas, A, et al. Predictive value of early near-infrared spectroscopy monitoring of patients with traumatic brain injury. Medicina. (2014) 50:263–8. doi: 10.1016/j.medici.2014.10.001
198. Minassian, AT, Poirier, N, Pierrot, M, Menei, P, Granry, JC, Ursino, M, et al. Correlation between cerebral oxygen saturation measured by near-infrared spectroscopy and jugular oxygen saturation in patients with severe closed head injury. Anesthesiology. (1999) 91:985–5. doi: 10.1097/00000542-199910000-00018
199. Kim, MB, Ward, DS, Cartwright, CR, Chlebowski, S, and Henson, LC. Estimation of jugular venous O2 saturation from cerebral oximetry or arterial O2 saturation during Isocapnic hypoxia. J Clin Monit Comput. (2000) 16:191–9. doi: 10.1023/a:1009940031063
200. Büchner, K, Meixensberger, J, Dings, J, and Roosen, K. Near-infrared spectroscopy – not useful to monitor cerebral oxygenation after severe brain injury. Cent Eur Neurosurg. (2000) 61:69–73. doi: 10.1055/s-2000-8262
201. Budohoski, KP, Zweifel, C, Kasprowicz, M, Sorrentino, E, Diedler, J, Brady, KM, et al. What comes first? The dynamics of cerebral oxygenation and blood flow in response to changes in arterial pressure and intracranial pressure after head injury. Br J Anaesth. (2012) 108:89–99. doi: 10.1093/bja/aer324
202. Forcione, M, Ganau, M, Prisco, L, Chiarelli, AM, Bellelli, A, Belli, A, et al. Mismatch between tissue partial oxygen pressure and near-infrared spectroscopy Neuromonitoring of tissue respiration in acute brain trauma: the rationale for implementing a multimodal monitoring strategy. IJMS. (2021) 22:1122. doi: 10.3390/ijms22031122
203. Highton, D, Ghosh, A, Tachtsidis, I, Panovska-Griffiths, J, Elwell, CE, and Smith, M. Monitoring cerebral autoregulation after brain injury: multimodal assessment of cerebral slow-wave oscillations using near-infrared spectroscopy. Anesth Analg. (2015) 121:198–205. doi: 10.1213/ANE.0000000000000790
204. Zweifel, C, Castellani, G, Czosnyka, M, Helmy, A, Manktelow, A, Carrera, E, et al. Noninvasive monitoring of cerebrovascular reactivity with near infrared spectroscopy in head-injured patients. J Neurotrauma. (2010) 27:1951–8. doi: 10.1089/neu.2010.1388
205. Brady, KM, Lee, JK, Kibler, KK, Easley, RB, Koehler, RC, and Shaffner, DH. Continuous measurement of autoregulation by spontaneous fluctuations in cerebral perfusion pressure: comparison of 3 methods. Stroke. (2008) 39:2531–7. doi: 10.1161/STROKEAHA.108.514877
206. Lee, JK, Yang, ZJ, Wang, B, Larson, AC, Jamrogowicz, JL, Kulikowicz, E, et al. Noninvasive autoregulation monitoring in a swine model of pediatric cardiac arrest. Anesth Analg. (2012) 114:825–36. doi: 10.1213/ANE.0b013e31824762d5
207. Lee, JK, Kibler, KK, Benni, PB, Easley, RB, Czosnyka, M, Smielewski, P, et al. Cerebrovascular reactivity measured by near-infrared spectroscopy. Stroke. (2009) 40:1820–6. doi: 10.1161/STROKEAHA.108.536094
208. Liu, X, Hu, X, Brady, KM, Koehler, R, Smielewski, P, Czosnyka, M, et al. Comparison of wavelet and correlation indices of cerebral autoregulation in a pediatric swine model of cardiac arrest. Sci Rep. (2020) 10:5926. doi: 10.1038/s41598-020-62435-8
209. Diedler, J, Zweifel, C, Budohoski, KP, Kasprowicz, M, Sorrentino, E, Haubrich, C, et al. The limitations of near-infrared spectroscopy to assess cerebrovascular reactivity: the role of slow frequency oscillations. Anesth Analg. (2011) 113:849–57. doi: 10.1213/ANE.0b013e3182285dc0
210. Steiner, LA, Pfister, D, Strebel, SP, Radolovich, D, Smielewski, P, and Czosnyka, M. Near-infrared spectroscopy can monitor dynamic cerebral autoregulation in adults. Neurocrit Care. (2009) 10:122–8. doi: 10.1007/s12028-008-9140-5
211. Zweifel, C, Castellani, G, Czosnyka, M, Carrera, E, Brady, KM, Kirkpatrick, PJ, et al. Continuous assessment of cerebral autoregulation with near-infrared spectroscopy in adults after subarachnoid hemorrhage. Stroke. (2010) 41:1963–8. doi: 10.1161/STROKEAHA.109.577320
212. Brady, K, Joshi, B, Zweifel, C, Smielewski, P, Czosnyka, M, Easley, RB, et al. Real-time continuous monitoring of cerebral blood flow autoregulation using near-infrared spectroscopy in patients undergoing cardiopulmonary bypass. Stroke. (2010) 41:1951–6. doi: 10.1161/STROKEAHA.109.575159
213. Pham, P, Bindra, J, Aneman, A, Chuan, A, Worthington, JM, and Jaeger, M. Noninvasive monitoring of dynamic cerebrovascular autoregulation and ‘optimal blood pressure’ in Normal adult subjects. Neurocrit Care. (2019) 30:201–6. doi: 10.1007/s12028-018-0600-2
214. Budohoski, KP, Czosnyka, M, Smielewski, P, Varsos, GV, Kasprowicz, M, Brady, KM, et al. Cerebral autoregulation after subarachnoid hemorrhage: comparison of three methods. J Cereb Blood Flow Metab. (2013) 33:449–56. doi: 10.1038/jcbfm.2012.189
215. Budohoski, KP, Czosnyka, M, Smielewski, P, Kasprowicz, M, Helmy, A, Bulters, D, et al. Impairment of cerebral autoregulation predicts delayed cerebral ischemia after subarachnoid hemorrhage: a prospective observational study. Stroke. (2012) 43:3230–7. doi: 10.1161/STROKEAHA.112.669788
216. Lee, KF, Wood, MD, Maslove, DM, Muscedere, JG, and Boyd, JG. Dysfunctional cerebral autoregulation is associated with delirium in critically ill adults. J Cereb Blood Flow Metab. (2019) 39:2512–20. doi: 10.1177/0271678X18803081
217. Nakano, M, Nomura, Y, Whitman, G, Sussman, M, Schena, S, Kilic, A, et al. Cerebral autoregulation in the operating room and intensive care unit after cardiac surgery. Br J Anaesth. (2021) 126:967–74. doi: 10.1016/j.bja.2020.12.043
218. Bindra, J, Pham, P, Chuan, A, Jaeger, M, and Aneman, A. Is impaired cerebrovascular autoregulation associated with outcome in patients admitted to the ICU with early septic shock? Crit Care Resusc. (2016) 18:95–101.
219. Ameloot, K, Genbrugge, C, Meex, I, Jans, F, Boer, W, Vander Laenen, M, et al. An observational near-infrared spectroscopy study on cerebral autoregulation in post-cardiac arrest patients: time to drop ‘one-size-fits-all’ hemodynamic targets? Resuscitation. (2015) 90:121–6. doi: 10.1016/j.resuscitation.2015.03.001
220. Pham, P, Bindra, J, Chuan, A, Jaeger, M, and Aneman, A. Are changes in cerebrovascular autoregulation following cardiac arrest associated with neurological outcome? Results of a pilot study. Resuscitation. (2015) 96:192–8. doi: 10.1016/j.resuscitation.2015.08.007
221. Hoiland, RL, Sekhon, MS, Cardim, D, Wood, MD, Gooderham, P, Foster, D, et al. Lack of agreement between optimal mean arterial pressure determination using pressure reactivity index versus cerebral oximetry index in hypoxic ischemic brain injury after cardiac arrest. Resuscitation. (2020) 152:184–91. doi: 10.1016/j.resuscitation.2020.03.016
222. Kirschen, MP, Majmudar, T, Beaulieu, F, Burnett, R, Shaik, M, Morgan, RW, et al. Deviations from NIRS-derived optimal blood pressure are associated with worse outcomes after pediatric cardiac arrest. Resuscitation. (2021) 168:110–8. doi: 10.1016/j.resuscitation.2021.09.023
223. Dias, C, Silva, MJ, Pereira, E, Monteiro, E, Maia, I, Barbosa, S, et al. Optimal cerebral perfusion pressure management at bedside: a single-center pilot study. Neurocrit Care. (2015) 23:92–102. doi: 10.1007/s12028-014-0103-8
224. Viderman, D, and Abdildin, YG. Near-infrared spectroscopy in neurocritical care: a review of recent updates. World Neurosurg. (2021) 151:23–8. doi: 10.1016/j.wneu.2021.04.054
225. Ogoh, S, Sato, K, Okazaki, K, Miyamoto, T, Secher, F, Sørensen, H, et al. A decrease in spatially resolved near-infrared spectroscopy-determined frontal lobe tissue oxygenation by phenylephrine reflects reduced skin blood flow. Anesth Analg. (2014) 118:823–9. doi: 10.1213/ANE.0000000000000145
226. Kahn, RA, and Anyanwu, A. Near-infrared spectroscopy in vegetables and humans: an observational study. Eur J Anaesthesiol. (2018) 35:907–10. doi: 10.1097/EJA.0000000000000855
227. Zavriyev, AI, Kaya, K, Farzam, P, Farzam, PY, Sunwoo, J, Jassar, AS, et al. The role of diffuse correlation spectroscopy and frequency-domain near-infrared spectroscopy in monitoring cerebral hemodynamics during hypothermic circulatory arrests. JTCVS Techniques. (2021) 7:161–77. doi: 10.1016/j.xjtc.2021.01.023
228. Bickler, PE, Feiner, JR, and Rollins, MD. Factors affecting the performance of 5 cerebral oximeters during hypoxia in healthy volunteers. Anesth Analg. (2013) 117:813–23. doi: 10.1213/ANE.0b013e318297d763
229. Zeiler, FA, Ercole, A, Beqiri, E, Cabeleira, M, Aries, M, Zoerle, T, et al. Cerebrovascular reactivity is not associated with therapeutic intensity in adult traumatic brain injury: a CENTER-TBI analysis. Acta Neurochir. (2019) 161:1955–64. doi: 10.1007/s00701-019-03980-8
230. Froese, L, Dian, J, Batson, C, Gomez, A, Unger, B, and Zeiler, FA. The impact of hypertonic saline on cerebrovascular reactivity and compensatory reserve in traumatic brain injury: an exploratory analysis. Acta Neurochir. (2020) 162:2683–93. doi: 10.1007/s00701-020-04579-0
231. Thorat, JD, Wang, EC, Lee, KK, Seow, WT, and Ng, I. Barbiturate therapy for patients with refractory intracranial hypertension following severe traumatic brain injury: its effects on tissue oxygenation, brain temperature and autoregulation. J Clin Neurosci. (2008) 15:143–8. doi: 10.1016/j.jocn.2006.08.014
232. Hemphill, JC, Andrews, P, and De Georgia, M. Multimodal monitoring and neurocritical care bioinformatics. Nat Rev Neurol. (2011) 7:451–60. doi: 10.1038/nrneurol.2011.101
233. Myers, RB, Lazaridis, C, Jermaine, CM, Robertson, CS, and Rusin, CG. Predicting intracranial pressure and brain tissue oxygen crises in patients with severe traumatic brain injury. Crit Care Med. (2016) 44:1754–61. doi: 10.1097/CCM.0000000000001838
234. Xiao, H, Peng, X, Asgari, S, Vespa, P, and Bergsneider, M. Forecasting ICP elevation based on prescient changes of intracranial pressure waveform morphology. IEEE Trans Biomed Eng. (2010) 57:1070–8. doi: 10.1109/TBME.2009.2037607
Keywords: neuromonitoring, transcranial Doppler, multimodal monitoring, near infrared spectroscopy, PbtO2 = brain tissue oxygen tension, cerebral autoregulation, neurovascular coupling, pressure reactivity index
Citation: Vitt JR, Loper NE and Mainali S (2023) Multimodal and autoregulation monitoring in the neurointensive care unit. Front. Neurol. 14:1155986. doi: 10.3389/fneur.2023.1155986
Edited by:
Rohan Sharma, Mayo Clinic Florida, United StatesReviewed by:
Amjad Elmashala, University of Florida, United StatesMariam Tsikvadze, Mayo Clinic Florida, United States
Vishank A. Shah, Johns Hopkins University, United States
Copyright © 2023 Vitt, Loper and Mainali. This is an open-access article distributed under the terms of the Creative Commons Attribution License (CC BY). The use, distribution or reproduction in other forums is permitted, provided the original author(s) and the copyright owner(s) are credited and that the original publication in this journal is cited, in accordance with accepted academic practice. No use, distribution or reproduction is permitted which does not comply with these terms.
*Correspondence: Shraddha Mainali, c2hyYWRkaGEubWFpbmFsaUB2Y3VoZWFsdGgub3Jn