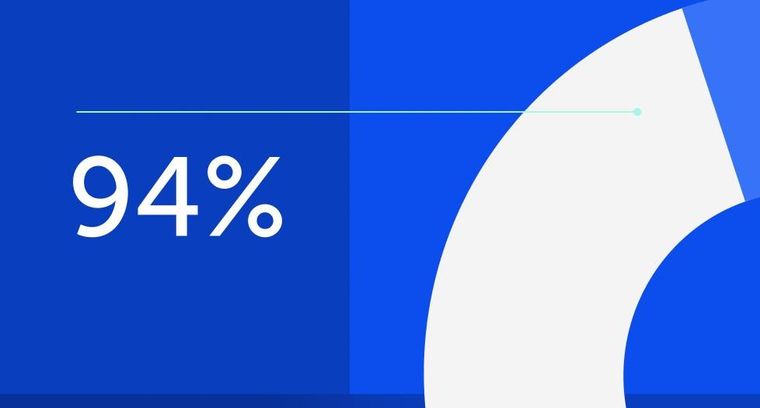
94% of researchers rate our articles as excellent or good
Learn more about the work of our research integrity team to safeguard the quality of each article we publish.
Find out more
REVIEW article
Front. Neurol., 03 August 2023
Sec. Neurotrauma
Volume 14 - 2023 | https://doi.org/10.3389/fneur.2023.1136367
This article is part of the Research TopicManifestations of mild-to-moderate traumatic brain injuryView all 9 articles
The human brain is an exceptionally complex organ that is comprised of billions of neurons. Therefore, when a traumatic event such as a concussion occurs, somatic, cognitive, behavioral, and sleep impairments are the common outcome. Each concussion is unique in the sense that the magnitude of biomechanical forces and the direction, rotation, and source of those forces are different for each concussive event. This helps to explain the unpredictable nature of post-concussion symptoms that can arise and resolve. The purpose of this narrative review is to connect the anatomical location, healthy function, and associated post-concussion symptoms of some major cerebral gray and white matter brain regions and the cerebellum. As a non-exhaustive description of post-concussion symptoms nor comprehensive inclusion of all brain regions, we have aimed to amalgamate the research performed for specific brain regions into a single article to clarify and enhance clinical and research concussion assessment. The current status of concussion diagnosis is highly subjective and primarily based on self-report of symptoms, so this review may be able to provide a connection between brain anatomy and the clinical presentation of concussions to enhance medical imaging assessments. By explaining anatomical relevance in terms of clinical concussion symptom presentation, an increased understanding of concussions may also be achieved to improve concussion recognition and diagnosis.
The field of concussion awareness, prevention, and mitigation is constantly growing. As a result, the medical knowledge of anatomical and physiological changes post-concussion is still evolving. To improve concussion diagnosis and personalized treatment, it is important to first understand brain structures and their respective functions. This review briefly defines concussion characteristics, but the main focus is on functional brain anatomy and the relationship of specific damaged brain regions to resulting post-concussion symptoms. The human brain is an exceptionally complex organ that comprises billions of neurons (1). Our brain consists of a large cerebrum, with left and right hemispheres made up of four lobes (frontal, temporal, parietal, and occipital), central sub-cortical structures (2), and the cerebellum (Latin for “little brain”) (3). The cortical surface, or gray matter, contains the neuronal cell bodies, dendrites, glial cells, axons, and synapses that produce neuronal signals and are found in the cortical, sub-cortical, and cerebellar areas as the gray layer (2). Conversely, white matter contains myelinated and unmyelinated neuronal axons, which are the physical connection between neuronal cell bodies that transmit the neuronal signals efficiently between gray matter regions (2). The cerebellum is an immensely folded brain region, segmented from the cerebrum, that is involved in all aspects of human function and cognition (3).
Concussions are complex injuries that can have various acute and chronic complications (4). A concussion is caused by a blow to the head, neck, or body that results in the brain becoming injured by resultant propagating forces (rapid de- or acceleration) and does not have to involve a loss of consciousness (4). Most adults who sustain a concussion recover within 10–14 days (~90%); however, many people have symptoms persisting longer than a month (4–6). Concussions are mainly caused by motor vehicle accidents, falls, assaults, and sports, with domestic events and non-professional sports being the leaders of this injury (5, 7). The forces applied to the brain during concussive events can produce serious shearing and tearing of tissues that trigger a cascade of neurometabolic changes (8). These structural, functional, and physiological brain alterations manifest uniquely in each individual, where these pathophysiological alterations are often undetectable on conventional clinical neuroimaging exams because these changes are on the molecular or microvascular scale. A head injury that results in structural injuries such as brain bleeding and swelling or a skull fracture would classify as a more severe traumatic brain injury and not a concussion. Thus, concussion-related brain damage or injury is most often present as shearing or tearing of white matter tracts (9) that result in the ionic dysregulation of sodium-potassium, impaired neurotransmission along axons (10), or more functional implications such as abnormal cerebral blood flow (11) and decreased blood-oxygen level-dependent (BOLD) signal, measured using functional magnetic resonance imaging (fMRI) scans (12), of focal brain regions (13) and across functional networks (14). With that information, this review will remain unspecific to the root injury pathophysiology (e.g., ionic dysregulation, decreased cerebral blood flow, or neurovascular uncoupling) when describing concussion symptoms to concussion-related brain damage. Furthermore, this review will focus on specific and focal brain regions as functional brain networks deserve a review of their own.
The brain has been the subject of extensive research, typically conducted as anatomical dissection, histology, and medical imaging, but also as cell/tissue culture and biochemical/genetic assays, which have allowed for an ever-improving understanding of normal and pathological brain function. This review focuses on 15 cerebral gray matter (Figure 1) and 10 cerebral white matter brain regions (Figure 2) that are physically large and have been shown in the literature to have important and specific functional relevance to concussions (21–25). The cerebellum and its subdivisions (Figure 3) were also examined to discuss its involvement in post-concussion symptoms, emphasize the influence it has over neurocognitive function, and encourage increased clinical and research attention to this important but often overlooked part of the human brain. This review is a non-exhaustive compilation of brain regions and concussion symptoms and is intended to be a reference point for researchers and clinicians. Neuroplasticity can alter regional brain function in specific individuals, especially post-injury, but the inclusion of all possible neuroplastic possibilities falls outside the scope of this review.
Figure 1. Gray matter brain regions (colored blue) relevant to concussion-related damage that is organized as (A–O): (A) amygdala, (B) anterior intra-parietal sulcus, (C) Broca's area, (D) hippocampus, (E) inferior parietal lobule, (F) insula, (G) lateral geniculate body, (H) mammillary body, (I) medial geniculate body, (J) premotor cortex, (K) primary motor cortex, (L) primary somatosensory cortex, (M) secondary somatosensory cortex, (N) superior parietal lobule, and (O) visual cortex. These brain regions are overlayed onto the MNI152 1 mm standard space T1-weighted brain from the (left to right) right sagittal, anterior frontal, and superior axial perspectives. These brain regions were from the Juelich Histological atlas (15–17).
Figure 2. White matter brain regions (colored blue) relevant to concussion-related damage that is organized as (A–J): (A) acoustic radiation, (B) callosal body, (C) cingulum, (D) corticospinal tract, (E) fornix, (F) inferior occipito-frontal fascicle, (G) optic radiation, (H) superior longitudinal fasciculus, (I) superior occipito-frontal fascicle, and (J) uncinate fascicle. These brain regions are overlayed onto the MNI152 1 mm standard space T1-weighted brain from the (left to right) right sagittal, anterior frontal, and superior axial perspectives. These brain regions were from the Juelich Histological atlas (15–17) and the JHU DTI-based white matter atlases (18–20).
Figure 3. Visualization of the cerebellum subdivisions overlayed onto the MNI152 1 mm standard space T1-weighted brain from the posterior, frontal plane perspective. There are 12 cerebellar regions in this figure, with lobules I–IV amalgamated, organized as follows: (A) I–IV, (B) V, (C) VI, (D) crus I, (E) crus II, (F) VII, (G) VIII, (H) IX, and (I) X. These brain regions were from the Probabilistic (FNIRT) cerebellar atlas (26).
Brain regions will be discussed in terms of their location within the human brain, their healthy functional involvement, and common alterations found post-concussion expressed as somatic, cognitive, emotional, or sleep-related symptoms (4, 27, 28) (Table 1). Somatic post-concussion symptoms can include headaches, nausea, vomiting, balance problems, visual problems, dizziness, light-headedness, fatigue, sensitivity to light, sensitivity to noise, numbness, tingling, bodily pain, and motor control problems. Cognitive post-concussion symptoms can include feeling “slow”, feeling “foggy”, difficulty concentrating, difficulty remembering, confusion, repetitive speech, and language problems. Emotional post-concussion symptoms can include irritability, sadness, feeling hopeless, nervousness, anxiousness, and feeling more emotional. Finally, sleep-related post-concussion symptoms can include trouble falling asleep, sleeping more or less than usual, and drowsiness. The variety of post-concussion symptoms indicates how a range of brain regions could be implicated during a single concussive injury, and why damage to specific brain regions may explain patient-specific symptoms.
Table 1. A summary table for the four primary categories of post-concussion symptoms—somatic, cognitive, emotional, and sleep—along with a list of the corresponding post-concussion symptoms within each category.
The amygdala is a symmetric deep brain structure that comprises a group of neurons located antero-medial to the hippocampus and sub-cortical to the temporal lobe (Figure 1A). It is almond-shaped and subdivided into the centro-medial, latero-basal, and superficial groups (29). The main role of the amygdala involves emotional and cognitive processing linked to the limbic system (30–32). Emotional responses related to pain, fear, incoming threats, reward-related activities, empathy, personal importance/significance, and facial expressions are all governed by the amygdala (33–36). Moreover, the amygdala has been noted to play roles in social attention, social responses, salience tagging, interpreting visual signals, tactile learning, explicit memory, and implicit learning (29, 37).
Damage to the amygdala can lead to deficits in emotional processing, emotional learning, and memory, which can be further manifested in autism spectrum disorder, psychopathy, and loss of the “cognitive control” system in adolescents (36, 38–42). Furthermore, sensitivity to fearful facial expressions, fear conditioning to social responses, alterations in vigilance, reduced self-motivation, and deficits in socio-emotional function can be caused by a damaged amygdala (29, 36, 43). This was corroborated in adolescents with persistent concussion symptoms who had elevated incidence of emotional/behavioral symptoms (e.g., depression, anxiety, and anhedonia) with decreased amygdala activity in response to an emotional face-processing task (44). With respect to sleep, a study using [18F]-fluorodeoxyglucose positron emission tomography (FDG PET) to measure the effect of combat-caused mild traumatic brain injuries on the relative cerebral metabolic rate of glucose (rCMRglc) found that head trauma was associated with lower rCMRglc while awake and during rapid-eye movement (REM) sleep in the amygdala, hippocampus, parahippocampal gyrus, thalamus, insula, uncus, culmen, visual association cortices, and midline medial frontal cortices (45). Based on the previously mentioned research on healthy and injured amygdala function, the common post-concussion symptoms related to the amygdala could result in somatic [e.g., headaches (46)], cognitive [e.g., feeling “slow” or “foggy”, difficulty concentrating, or difficulty remembering (29, 37)], emotional [e.g., irritability, sadness, nervousness, more emotional (33, 44)], or sleep symptoms [e.g., trouble falling to sleep, loss of sleep (45)] (Table 2).
Table 2. A summary of 15 gray matter brain regions and their associated functions and concussion-related symptoms.
The anterior intra-parietal sulcus lobule occupies the antero-lateral bank of the deep intraparietal sulcus that spans the surface of the parietal lobe (Figure 1B) (29). The anterior intra-parietal lobule can be further subdivided into three zones (hlP1, hlP2, and hlP3) based on cytoarchitecture (47, 48). Regions hlP1 and hlP2 are situated in the lateral wall of the anterior intra-parietal sulcus, while the hlP3 region lies more medial and has a distinctly different laminar pattern from the rest of the other superior parietal lobe gray matter, which ends posteriorly at the base of the intra-parietal sulcus (48). The anterior intra-parietal sulcus lobule communicates with the cingulum (33), superior longitudinal fasciculus (49), sensory and motor cortices (50, 51), insula (52), the temporal (50) and occipital lobes (53), and neighboring parietal structures such as the inferior and superior parietal lobules (48, 52).
The anterior intra-parietal sulcus lobule mainly contributes to visuomotor functions including finger manipulation (49, 51), tactility (53), eye movements (51, 54), vestibular and egocentric attention (55), auditory coordinate location (56), and hierarchical structure processing (50). The anterior intra-parietal sulcus lobule also plays a role in manipulating objects with responsiveness to size, shape, and surfaces of specific geometries (57, 58), temporal relations with regard to grasping (57), memorizing geometry (57), coordinated defensive movements (59, 60), and writing-related functions (61). Visual-dominant neurons, found only in the anterior intra-parietal sulcus lobule, activated differently with respect to ambient light levels (60, 62). A study on patients with moderate and severe traumatic brain injuries found the anterior intra-parietal sulcus to have decreased functional connectivity in relation to sensory processing and integration and attention networks (63). Damage to the anterior intra-parietal lobule has also been shown to manifest as reduced ability to manipulate objects (62, 64) such as ideomotor apraxia (64, 65), reduced grip (64, 66), reduced tactile sensitivity (58, 67), an inability to grasp objects (57, 62, 64), difficulty visualizing object rotation (68), spatial neglect (69–71), and autotopagnosia (67). Post-concussion symptoms associated with the anterior intra-parietal sulcus lobule are somatic [e.g., light sensitivity (58, 60, 62), noise sensitivity (55, 56), motor control problems (49, 57), visual problems (62, 72)] and cognitive symptoms [e.g., feeling “slow” (63)] (Table 2).
This area resides in the inferior and lateral aspect of the pars opercularis of the inferior frontal lobe, which is bordered by the Sylvian fissure and its ascending anterior ramus ventrally and the precentral sulcus dorsally in the dominant, and typically left, hemisphere (Figure 1C) (73). Broca's area is the language processing area and is fundamentally involved in the motor aspect of speech (73). Neurological signals generated from Broca's area help initiate the movement of musculature in the throat, mouth, and tongue to produce meaningful sounds and initiate complex speech (29). This area is the neural mechanism for language and plays a vital role in word decoding, language production, phonology, articulation, and ensuring proper grammar, and is associated with all language-related tasks (29, 42, 74, 75). Structurally, Broca's area can be further subdivided into two parts, Brodmann's Area (BA) 44 and BA45 (which is located in the pars triangularis of the frontal operculum of the inferior frontal lobe, which is bordered by the Sylvian fissure and its horizontal ramus ventrally and its ascending anterior ramus posteriorly), and the arcuate fasciculus pathway links Wernicke's area to Broca's area to produce the complete motor and sensory aspects of language and speech (64, 76). BA44 is more involved in language production, whereas BA45 is further involved in semantics and fluency, temporal or affective encoding (77).
Damage to Broca's area includes, but is not limited to, conduction aphasia, difficulty initiating speech, effortful speech production, difficulty forming sentences, impairment in speech melody, poor articulation, semantic and phonemic paraphasia, slurring, production of telegraphic sentences, abnormal grammatical forms, and omitting the ending of words (29, 73, 74, 76–78). The overall absence of auditory comprehension can also lead to a reduced ability to imitate other people's spoken words and difficulties with reading and writing (29, 78). One study on symptomatic adolescents at 1 month post-concussion found that Broca's Area had decreased activation during the working memory 1-back > 0-back contrast task, and symptomatic participants felt significantly more slowed down, mentally foggy, had difficulties remembering and concentrating, had a lower neurocognitive index, and complex attentional test scores (25). Post-concussion, injury of Broca's Area could manifest as cognitive symptoms [e.g., feeling “slow” or “foggy”, difficulty concentrating or remembering (25), or speech and language impairments (77, 78)] (Table 2).
The hippocampus is a symmetrically elongated brain structure that lies deep near the brain's hemispheric midline toward the splenium of the corpus callosum and follows it anteriorly but also has lateral extensions to the temporal lobe (Figure 1D). The hippocampus can be separated into head, body, and tail segments, with subdivisions of the hippocampal head that include the cornu ammonis, dentate gyrus, and the subiculum (29). The hippocampal entorhinal cortex facilitates learning, memory, emotion, and social behavior (43, 79), whereas the subiculum focuses on episodic memory functions (80). The hippocampi are a central structure that connects with and affects all brain functions (33, 79, 81, 82), with a closer influence over the premotor cortex (83), medial geniculate bodies (84), mammillary bodies (33, 79), and other deep brain structures in the diencephalon (29, 33, 85). The thalamus, hypothalamus, amygdala, mammillary body, and fornix also have key hippocampal connections to comprise the limbic system and enable memory facilitation (43, 84). Furthermore, important white matter structures such as the cingulum (33, 86), uncinate fasciculus (43, 81), and corticospinal tract (82) facilitate other functions of the hippocampus.
The main role of the hippocampi is to execute every aspect of memory (33, 42, 86), but other vital roles include learning new tasks (29), understanding verbal and spatial cues (29, 68, 87), motivation (43), egocentric and allocentric coding (88, 89), and executive function (33).
Damage to the hippocampus often manifests in a variety of memory impairments (81, 90) affecting verbal (29), spatial (29, 91), and episodic (29, 33, 79) memory functions (Table 2). Furthermore, hippocampal atrophy due to aging (92), concussions (93), and chronic stress (35) can produce cascading cell loss and/or gliosis (40) leading to myopathy, weakness, fatigue, bone decalcification, and further neural degeneration (94). Among other conditions, the sustained degradation of the hippocampus has been shown to cause amnesia (95), mild cognitive impairment (96, 97), Alzheimer's Disease (98), depression (99), and anxiety disorders (100). Since hippocampi are so crucial to learning, damage has also been shown to impair social conditioning and certain motor tasks (91). Concussions have been shown to cause cerebral blood flow and activity in the hippocampus which would be related to the presence of memory-related symptoms (13). Thus, in summary, common post-concussion symptoms related to focal hippocampus damage could be cognitive [e.g., feeling “foggy”, or difficulty remembering (13, 79)] or emotional symptoms [e.g., feeling more emotional, sadness, and nervousness (99, 100)]. Fortunately, in unilateral damage, the option of some compensation through the communication of the contralateral hippocampus, along with memory training, has been shown to initiate compensatory neuroplastic processes (33) to diminish impairments caused by pathology (80).
The inferior parietal lobule is a large symmetric lobule (i.e., grouping) on the inferior aspect of the parietal lobe below the inferior parietal sulcus (Figure 1E) (29). The inferior parietal lobule also harbors the upswing of the long, deep arcuate intra-parietal sulcus behind the lower postcentral gyrus which then slashes posteriorly across the convex surface of the parietal lobe (29). The inferior parietal lobule can be further separated into 3 subregions based on cytoarchitecture into the anterior, middle, and posterior subdivisions (101). The connectivity of the inferior parietal lobule with all major semantic areas of the brain lends itself to communicating with aspects of the temporal lobe (43, 102), occipital lobe (103), cerebellum (64), neighboring superior and anterior intraparietal lobules (33, 43, 75), sensory and motor cortices (43, 49, 102), and deep brain structures such as the insula (104), amygdala (34), medial geniculate bodies (105), and hippocampi (30). Additionally, white matter bundles such as the arcuate fasciculus (106), cingulum (33, 43), and superior longitudinal fasciculus (43, 107) form long U-shaped fibers (74) that connect Broca and Wernicke's area for the left inferior parietal lobule to aid in the language (43, 108).
Overall, the inferior parietal lobule interfaces with several areas of information convergence to facilitate a variety of sensorimotor and behavior-related actions (52, 109). Visuospatial navigation is primarily carried out by the inferior parietal lobule, which plays roles in visuomotor mechanisms (49, 110, 111), velocity/timing information (112), grasping (49), and complex tool use (49). Bilaterally, the inferior parietal lobule also plays roles in sound perception and auditory memory despite the primary auditory cortex being in Heschl's gyrus in the superior temporal lobe (103, 113), saccadic eye movements (114), egocentric decision-making (60), and emotional empathy (34). The left inferior parietal lobule is involved in speech and language processing (108) and reading and writing (75), while the right inferior parietal lobule is responsible for natural handwriting tempo (115).
Damage to the inferior parietal lobules generally causes reduced visuospatial and motor control abilities (52, 74, 86, 116), auditory agnosia (117), increased egocentric or allocentric behavior (55), and language deficits (108). Damage to the inferior parietal lobule is also related to speech pathology and shown to cause phonemic paraphasias (74), dysgraphia causing difficulties in reading and writing (52, 75, 118), disrupted phonological processing, and speech arrest (74). Specific to concussion, a study by Zuleger et al. found that the inferior parietal lobule was significantly altered post-concussion and significantly connected to the primary motor and sensory cortices and the inferior temporal gyrus; suggesting that sensorimotor, attention, cognition, and proprioception functions would be affected by concussion (119). In general, common post-concussion symptoms arising from inferior parietal lobule damage would include somatic [e.g., headaches, balance problems, dizziness, light or noise sensitivity (103, 113, 119) visual and motor problems (49, 119)] and cognitive symptoms [e.g., feeling “slow” or “foggy”, difficulty concentrating or remembering (103, 113, 119)] (Table 2).
The insula is a large triangular region that lies deep into the lateral cerebral fissure (i.e., Fissura Sylvi), covered by the lower parietal and frontal lobes and transitions to form the temporal lobes (Figure 1F) (29, 120). The central sulcus of the insula separates the small gyri (usually 3) from the long gyri (usually 2). The insula is cytoarchitectonically distinguishable from surrounding brain regions by lamination patterns and degrees of granularity (37, 120, 121). The insula can be subdivided into three main subregions known as the anterior granular, posterior granular, and intermediate dysgranular cortices (37, 120, 121). The anterior and posterior granular cortices are a central node within the limbic, frontal, and auditory pathways (37, 121); whereas the intermediate dysgranual cortex mainly facilitates vestibular and somatic sensations (122). Apart from its subdivisions, the insula's unique anatomical position allows for communication with many brain regions including the larger frontal, parietal, temporal, and occipital lobes (120, 123), and with more distinct structures such as the amygdala (43), hippocampus (43), thalamus (124), midbrain (125), medial geniculate nucleus (126), auditory cortex (126), somatosensory cortices (37), motor cortices (127), and Broca's area (128), and also has connections to the cerebellum (124). White matter bundles connected to the insula also consist of the cingulum (43, 124), corpus callosum (129), and arcuate fasciculus (76).
Given its multiple communication pathways, the insular system carries out a variety of somatic and motor functions and is involved with emotional behaviors (120). Sensorially, the insula interprets contralateral, and occasionally ipsilateral (130), tactility (37, 43) with regard to feelings of warmth (37, 131, 132) and vibration (133). Processing information for auditory (126), taste (37, 134), and pain (37, 135, 136) stimuli are also insular somatosensory functions. Because of its connections to the motor cortex, the insula is also involved in speech production (122, 125, 134, 137), gastric motility (37, 135, 138), repetitive motor movements (43, 139), cardiovascular function (37, 140), and also to other afferent vagal nerve fibers (141). Finally, the insula may allow the production of the appropriate emotional responses to stimuli (142) and connect feelings with decision-making (120, 143). Emotions such as empathy (60), body awareness (37, 86), decision-making tasks (37, 132), and disgust (37, 144) all have insular involvement.
Pathology associated with the insula is often characterized by spontaneous somatosensory sensations that cause discomfort and pain along with a series of other impairments (Table 2). Somatosensory discomfort can include warmth and thermal sensitivity, violent and painful electric current sensations in the face, mouth, and upper limbs, abdominal heaviness, and difficulty breathing (37, 134). As the insula forms part of a central pain pathway (145), patients with insular lesions may suffer from pseudothalamic syndrome (146, 147), painful paresthesias (37), nociceptive sensitivity, analgesia/hyperalgesia (146), and difficulties processing pain (37, 148). Insular pathologies can result in auditory impairments (131), including hearing loss (105), auditory agnosia (149), or auditory hallucinations (150), olfactory (29, 42) or gustatory impairments such as unpleasant and metallic tastes (37), alterations in gastro-intestinal movement/motility and tone (130), and deficits in discriminating size, texture, and shape of objects (151). Moreover, speech-related problems can manifest as conduction aphasia (76, 103), effortful speech, articulation impairments, semantic and phonemic paraphasias, telegraphic sentences, abnormal grammatical form, and dysphonic and dysarthric speech (37, 134). Other symptoms that have been noted due to insular damage include mental confusion (130), short-term memory deficits (76), and nausea (67). Isnard et al. also found that patients with insular lesions could suffer from hypersalivation, clonic jerks in the arm or face, anxiety, compulsive swallowing, and impaired consciousness (134). Untreated insular damage could lead to empathy or emotional deficits (86), disruptive behavior disorders in adolescents (86, 152), depression in adults (34, 153), anxiety (154), and a tendency toward substance abuse (155). A recent MRI study found significantly reduced insular functional connectivity and damaged white matter connections along with slower times on completing all cognitive tasks and lower test scores compared to controls (156). Another recent study on post-concussion headaches compared persistent concussion symptoms patients with migraine patients and identified numerous functional connectivity differences between brain regions, including the insula, cingulate, temporal pole, cuneus, secondary somatosensory cortex, ventro-medial prefrontal cortex, and others (46). In summary, insular damage caused by a concussion could result in somatic [e.g., headache (46, 157), nausea, vomiting (67, 130), noise sensitivity (105, 134), and motor problems (120, 134), or bodily pain (145, 148)], cognitive (e.g., feeling “slow” or “foggy” (156), or language problems (120, 134)], or emotional symptoms [e.g., irritability or feeling more emotional (86, 142, 153)] (Table 2).
The lateral geniculate bodies, also referred to as the lateral geniculate nuclei, are a pair of dense, symmetric neurons that lie directly lateral to the medial geniculate bodies (Figure 1G) (158). The lateral geniculate bodies play a significant role in relaying visual impulses from the retina by integrating pathways from the optic radiation, optic nerve, Meyer's loop, corpus callosum, brainstem, occipital cortex, and other visual-related nodes (31, 158). The lateral geniculate bodies are also considered to have a large thalamic component and thus are the first stage at which feedback signals affect visual processing (159, 160). The thalamic connection to the lateral geniculate bodies governs selective attention control related to visual inputs (158).
Pathology associated with the lateral geniculate bodies is characterized by an overall loss of visual experience, lack of visual awareness, and a reduced ability to understand visual inputs (Table 2) (158, 161). In addition, due to the link to selective attention, lateral geniculate body damage can also produce blindsight in particular areas and lead to difficulty concentrating on visual objects (158, 161, 162). A diffusion MRI case study on a 35-year-old mild traumatic brain injury female patient found auditory deficits and deafness in connection to white matter injury between the lateral geniculate body and occipital pole (163). Based on the neuroanatomical role of the lateral geniculate body and the diffusion case study, common post-concussion symptoms related to lateral geniculate body impairment could result in somatic symptoms (e.g., visual problems and sensitivity to noise and light).
The mammillary bodies are a pair of spherical structures within the inferior hypothalamus paramedian of the brain that lies directly adjacent to the rostral-anterior aspect of the brainstem (Figure 1H) (79, 164). The mammillary bodies are part of the limbic system and part of the Papez circuit, which facilitates memory and emotion (31, 79). The mammillary bodies are particularly involved in long-term memory function, word recognition, recall of episodic information, spatial processing, and the ability to understand olfactory inputs (33, 165, 166).
Damage to the mammillary bodies can lead to a variety of memory, olfactory, and spatial deficits, however, because it is considered a relay station in the Papez circuit, the bodies can undergo atrophy due to damage in its other connecting nodes such as the amygdala, fornix, hippocampus, and thalamus (31, 167) or due to chronic alcohol consumption (168). The functions of these structures have also been associated with dementia, epilepsy, schizophrenia, amnesia, and the loss of smell and/or the inability to process or understand the sense of smell (31, 165, 166). A recent article on chronic traumatic encephalopathy (CTE) found that mammillary bodies were frequently atrophied in CTE patient brains, and thus could apply to individual concussion cases (169). Post-concussion, damage to the mammillary bodies could result in somatic [e.g., headaches and loss of smell (33, 165)] and cognitive symptoms [e.g., difficulty remembering (33)] (Table 2).
The medial geniculate bodies, often referred to as the medial geniculate nuclei, are a pair of symmetric structures that lie directly adjacent to the brainstem and medial to the lateral geniculate nuclei (Figure 1I) (170, 171). The medial geniculate bodies can be subdivided into ventral (172), dorsal, and medial (173). Connections from the medial geniculate bodies to the inferior colliculus and the auditory cortex also form pathways to create a detailed association between speech and sound (173). The medial geniculate bodies are mainly responsible for relaying auditory impulses or sounds from the ear to the temporal lobe via acoustic radiation and the thalamus (173, 174).
Auditory frequencies processed in the medial geniculate bodies are organized such that complex and higher-order sounds are processed with the neuronally denser lemniscal pathway that integrates auditory and multisensory information, whereas secondary sounds, such as sharp responses to tones, are processed by less neuron-rich regions (171). The extralemniscal pathway then processes responses to basic tones (150, 174). The medial geniculate bodies have also been known to facilitate the efficient transmission of auditory linguistic signals in speech to preserve and perceive environmental sounds (175). Pathology associated with the medial geniculate body is generalized auditory agnosia and a reduced ability to understand auditory inputs (171, 176). Partially because of the close connection between the medial geniculate bodies and other subcortical structures such as the hippocampus and amygdala, a rodent model traumatic brain injury study found abnormally increased activity in the medial geniculate bodies in connection to auditory-induced post-traumatic stress and amygdala dysfunction (177). Based on the functions of the medial geniculate body and its connections to other subcortical structures, concussion-related damage could manifest as somatic symptoms [e.g., headaches and noise sensitivity (171, 177)] and possibly emotional symptoms [e.g., nervousness (177)] (Table 2).
The premotor cortex spans a substantial portion of the frontal lobe and lies directly anterior to the primary motor cortex (Figure 1J) (29). The premotor cortex can be subdivided into ventral and dorsal regions and interacts with the primary motor cortex, corticospinal tract, colliculi projections, acoustic radiation, auditory cortex, basal ganglia, cerebellum, and the limbic system to generate and plan motor movements (29, 124). Information from multimodal sensory inputs is sent to the premotor cortex where spatial coordinates are transformed into an appropriate visuomotor 3D representation of space for the primary motor cortex to convert abstract goals into planned motor actions (124, 178). The premotor cortex is therefore utilized in precise, fine-motor hand movements (43, 62, 178). The premotor cortex also combines tactile, visuospatial, proprioceptive, and cognitive information to carry out specialized tasks (43, 49, 52, 60, 64, 84, 178). Studies have further shown that the premotor cortex plays roles in particular social behaviors such as language and articulation processes (52, 60), writing tasks (75), music cognition (150), early phases of learning (64), imitation and empathy (60), understanding intentions and actions (106), vigilance (179), and motivation (180).
Pathology associated with the premotor cortex mainly manifests in reduced motor control which can cause difficulties in chewing and performing facial expressions (29), performing coordinated movements (64), learning a new skilled movement (64), and ideomotor apraxia (181). Premotor cortex damage can also affect precise hand movements, errors in limb position and trajectory, and praxis in hand and finger movements (64). Due to its involvement with speech, premotor pathology can also cause hearing impairments (150), complete speech arrest (74), articulatory disturbances (182), anarthria, or dysarthria (74, 122), and in severe cases can lead to Pick's disease (64). It is also important to note that significant crosstalk occurs between the bilateral premotor cortices and the ipsilateral primary motor cortex in which symptoms can sometimes be expressed (64). A study on acutely concussed adolescents found that the premotor cortex was one of several brain regions that had significantly reduced BOLD activity during working memory tasks (183). Meanwhile, a recent study on a different group of adolescents found that increased connectivity between the default mode network (DMN) and the lateral premotor cortex was correlated with motor impairments post-concussion (184). A study on white matter projections from the corpus callosum found that concussed female athletes had a lower white matter volume and fewer tracts projecting to the premotor cortex; related symptoms were not reported but would be related to motor movements and coordination (185). Specific to post-concussion headaches, a recent resting-state fMRI study found that the premotor cortex was consistently abnormal in those with mild, moderate, and severe post-concussion headaches (157). Post-concussion symptoms that could arise from premotor cortex injury would primarily be somatic [e.g., headaches (157), nausea, balance problems, dizziness, fatigue, and motor control problems (64, 184, 185)], but could also include cognitive symptoms [e.g., feeling “slow”, language problems (60, 74, 75), or difficulty remembering (64, 183)] (Table 2).
The primary motor cortex is located on the superior aspect of the frontal and parietal lobes, on either side of the central sulcus and anterior to the primary somatosensory cortex, and has some anatomical overlap with the premotor cortex (Figure 1K) (29). The primary motor cortex is closely connected to the premotor cortex, somatosensory cortex, thalamus, hippocampus, corpus callosum, and brainstem to effectively perform motor movements (29, 60, 186). Closely linked by proximity and functional communication to the somatosensory homunculus (187–189), the primary motor cortex is also organized somatotopically where specific zones are responsible for directing the action of specific groups of muscles, joints, and limbs (186). The organization of the primary motor cortex begins inferolaterally with the tongue, continuing superiorly in the order of lips, squinting, and fingers, with zones for the wrist, forearm, and elbow interspersed on the superolateral aspect of the primary motor cortex, with the lower limb and foot zone located on the superomedial aspect (29, 186).
Regarding function, the primary motor cortex is responsible for the execution of voluntary bodily movement. Once a specific motor task has been decided upon, a “blueprint” for the motor task is sent to the spinal cord or the cranial nerves for task execution (29, 186). Information from the primary motor cortex is transmitted through the brainstem's pyramidal desiccations to the contralateral corticospinal tract (i.e., motor plans for the right arm are generated by the left primary motor cortex) (29, 186). To ensure proper coordination, the primary motor cortex also incorporates important sensory feedback through touch, proprioception, autonomic functions, pain, temperature, strength of muscle contractions, and audiovisual inputs (124, 189). Apart from directly controlling movements, the primary motor cortex is also involved in writing tasks (75), executive control (190, 191), imitation (60), and early phases of learning (64).
Pathology associated with the primary motor cortex traditionally follows the loss of function in contralateral muscles, muscle weakness, and reduced motor skills and muscle selectivity (192, 193). Other pathological symptoms can include impairments to gait, balance, skilled movements, muscle paresis, muscle atrophy (29, 64), facial palsy, spasticity, and hearing loss (Table 2) (150, 194). Similar to the premotor cortex, the primary motor cortex also exhibits cross-talk between hemispheres, therefore, subtle abnormalities in ipsilateral limbs may also be present (64). However, if injuries persist, limb-kinetic apraxia can develop into corticobasal degeneration and further into Pick's disease (64, 195). Fortunately, the primary motor cortex is highly adaptive and has shown a high capacity for plasticity during injury recovery (196, 197). A 1H-MR spectroscopy study on acutely concussed young adults found that the primary motor cortex had significantly decreased glutamate (i.e., main excitatory neurotransmitter) and N-acetylaspartate that correlated with symptom severity (198). Another study, using transcranial magnetic stimulation, found that concussion severity and subsequent concussions had long-term effects resulting in subclinical motor cortex dysfunction (199). The primary motor cortex is involved in many functions, and as such these post-concussion symptoms could arise as somatic [e.g., headaches, nausea, vomiting, balance problems, fatigue, and movement impairments (29, 64, 199)] or cognitive symptoms [e.g., feeling “slow” (64, 190)] (Table 2).
The primary somatosensory cortex is a large brain region that is symmetric and directly posterior to the primary motor cortex and the central gyrus, with some anatomical overlap with the premotor cortex (Figure 1L) (29). The primary somatosensory cortex stretches from the longitudinal fissure to the Sylvian fissure (Lateral sulcus) approximately along the gyri immediately posterior to the central sulcus. The primary somatosensory cortex can be further subdivided into four cytoarchitectonic areas arranged from anterior to posterior termed Brodmann areas BA3a, BA3b, BA1, and BA2 that connect to other brain structures to process all sensory sensations of the human body (29, 200).
Mechanoreceptive somatosensory inputs from the primary sensory areas including the visual, auditory, vestibular, and other nervous systems send information through the spinal cord to the primary somatosensory cortex to contextualize sensory information to aid future motor-based decisions (29, 37). The primary somatosensory cortex is therefore processing the sensory information for proprioception (29, 55, 64, 180), vision (47, 180), motor control (201), regulating cortical excitability (67), involuntary movement activation (202), working memory (29), fast perceptual learning (203), and pain (204–206). Like the primary motor cortex, the primary somatosensory cortex is organized somatotopically in discrete zones, described by the somatosensory homunculus (187, 188). Furthermore, each subdivision has been demonstrated to have complete maps of the contralateral body surface (187). Neuroplasticity and cortical reorganization are present in the primary somatosensory cortex (207, 208), which indicates that cortical maps are in a constant state of fluctuation (203) and that neural representation is dependent on triggered stimuli (67).
Damage to the primary somatosensory cortex can cause an overall reduction in sensory input and interpretation, which can manifest as reduced tactile ability, poor grip, object manipulation, uncoordinated finger movements (29), impaired recognition of facial expressions (67, 209), and praxis errors involving orientation, limb coordination, and motor control (Table 2) (64, 201). Discomfort and pain are also commonly elicited as paresthesia, pins and needles, numbness, tingling, and warmth affecting the lips, cheek, face, tongue, upper limbs, and lower limbs (37, 134, 210). Additionally, deficits in pain processing occur where pain can be generated sporadically (94) or create phantom limb pain (67). A recent longitudinal fMRI study found that the functional connectivity of the primary somatosensory cortex significantly reduced at 1 month post-concussion but recovered after 5 months, and that improved somatosensory connectivity was correlated with symptom resolution (211). Thus, post-concussion symptoms would be expected in the presence of primary somatosensory injury as primarily somatic symptoms [e.g., headaches (46), nausea, vomiting, balance problems, light sensitivity, noise sensitivity, bodily pain, numbness, or visual problems (180, 201, 211)] (Table 2).
The secondary somatosensory cortex is another large brain region that is symmetric and lies directly posterior to the primary somatosensory cortex on the inferolateral aspect of the parietal lobe (Figure 1M) (29). Similar to the primary somatosensory cortex, the secondary somatosensory cortex can be further subdivided into four segments based on their cytoarchitecture and functional differences: Operculum (OP) 1 (lateral dorsal), OP2 (posterior ventral), OP3 (anterior ventral), and OP4 (anterior) (212, 213).
The secondary somatosensory cortex carries out similar sensory processing functions as the primary somatosensory cortex (29, 55, 64, 139). Also, like the primary somatosensory cortex, the secondary cortex is organized inferolaterally to superomedially in a somatotopic form in the order of face, hands, trunk, and legs (139, 213). The secondary somatosensory cortex sets itself apart in the ability to localize the origin of somatic sensations and communication with the parietal cortex (48) and limbic system (94). Moreover, the localization abilities are attributed to enlargements of representation maps (203, 208, 214) and less consistent somatotopic organization (139, 151, 215), where variability in activation improves discrimination abilities (203, 216).
Damage to the secondary somatosensory cortex often manifests in reduced psychophysical performance (203), reduced tactile sensitivity, memory problems (29), phantom limb pain (203, 217), and praxis errors (64). However, pathology is predominantly presented as diverse and unpleasant sensations of paresthesia, pins and needles, numbness, tingling, electrical current, warmth, electric discharge, and pain in the lips, cheek, face, tongue, upper and lower limbs, neck, and torso (29, 37, 62, 134). Fortunately, the secondary somatosensory cortex is known to have strong cortical reorganization abilities that drive plastic changes, along with similar contralateral somatotopy to reduce effects due to pathology (139, 151, 203). Similar to the primary somatosensory cortex, concussion-related damage to the secondary somatosensory cortex could result in somatic symptoms [e.g., headaches (46, 157), nausea, balance problems, dizziness, light sensitivity, noise sensitivity, numbness, visual problems, or bodily pain (134, 203)] (Table 2).
The superior parietal lobule is a large symmetric brain region on the superior aspect of the parietal lobe and is situated directly superior to the intra-parietal sulcus and incorporates a substantial amount of the cortical parietal lobe tissue posterior to the primary motor and somatosensory cortices (Figure 1N) (218). The superior parietal lobule can be further subdivided into five subregions (218). The main function of the superior parietal lobule is to integrate multimodal somatosensory and visual inputs to create specific motor movements and is thus highly connected to motor and sensory brain regions (29, 218). In addition to motor and sensory integration, the superior parietal lobule plays a role in egocentric tasks (219, 220), emotion-relevant behavior (86, 221), and auditory association (222, 223).
Damage to the superior parietal lobule mainly manifests in visuospatial navigation impairments (68, 111) causing a variety of praxis errors (64, 75), particularly in the dark (64), such as apraxic dysgraphia (52), autotopagnosia (64, 67), poor balance (48), and poor posture (64). Superior parietal lobule pathology has also been shown to reduce attention spans in youth (86) and is strongly correlated with pathology attributed to the inferior parietal lobe (52). A study on acutely concussed adolescents performing a navigational memory task had their fMRI BOLD signal change from baseline was negatively correlated with post-concussion symptom severity in the superior and inferior parietal lobes, premotor cortex, and parahippocampus (224). Another study on post-concussion adolescents also found verbal and visual memory impairments related to the superior and inferior parietal lobules (225). Post-concussion symptoms associated with superior parietal lobe injury could include somatic [e.g., headaches (46), balance problems, dizziness, light sensitivity, motor control problems, or visual problems (48, 64, 218)] or cognitive symptoms [e.g., feeling “slow”, difficulty remembering, and confusion (224, 225)] (Table 2).
The visual cortex is a large region that covers much of the occipital lobe (Figure 1O) (29). More specifically, the primary visual cortex is located at the most posterior point of the occipital lobe, which is medial and close to the longitudinal fissure (29). The secondary and association visual areas cover most of the remaining aspects of the occipital lobe, which is superolateral to the primary visual cortex (29). Based on the description provided by Purves et al. the visual cortex can be separated into eight different brain regions, where V1 is the primary visual cortex and V2 is the secondary visual cortex, while V3, V3a, V4, ventral posterior (VP), middle temporal (MT), and middle superior temporal (MST) comprise the remaining association visual areas (226). The calcarine sulcus runs transversely through the primary visual cortex, the secondary visual cortex wraps around the primary visual cortex, V3 and V3a are superior to the secondary visual cortex, and VP and V4 are inferior to it (201). The MT and MST regions are small and slightly separated from the other visual cortex regions, found on the inferior, lateral aspects of the occipital lobe (226).
In general, the visual cortex is responsible for receiving, processing, and interpreting visual information that travels from the retina, along the optic nerve, passing through the thalamus, and arriving at the primary visual cortex (29). This includes the processing of color, brightness, shape, and motion captured with the visual sensory system (226). Visual information is also processed on the contralateral side of the brain than the eye. The cortical visual regions of V3A, MT, and MST are involved in motion perceptions (226–228), whereas V4 is involved with color interpretation and processing (Table 2) (226, 227). A study on seven acutely concussed young adults found numerous oculomotor impairments related to the visual cortex during the first-week post-injury and after 30 days (229). Another study examining concussion patients exhibiting vestibular symptoms noted vestibular, visual, and sensory processing networks to be affected (230). Due to the visual implications of concussion-related injury to the visual cortex, concussion-related visual cortex damage would primarily present as somatic symptoms [e.g., headaches, nausea, vomiting, balance problems, dizziness, light sensitivity, and visual problems (226, 229, 230)] (Table 2).
Acoustic radiation is a white matter tract that originates at the medial geniculate nucleus of the thalamus and travels anterior and lateral toward the primary auditory cortex on the transverse temporal gyri of the temporal lobe (Figure 2A) (174, 231). It is essential to transmitting auditory information from the thalamus to the temporal cortex and is therefore essential to auditory and language comprehension (174, 232, 233).
Damage to the acoustic radiation could lead to a range of auditory impairments (Table 3). Studies have shown that damage to acoustic radiation is associated with hearing and language disorders, auditory processing deficits, and decreased speech comprehension (174). More serious damage could lead to cortical (central) deafness (117, 149), environmental sound agnosia, total auditory agnosia of all sounds (149), or verbal deafness (word agnosia) (175, 176). Additionally, an individual can experience auditory hallucinations (i.e., the experience of hearing music in the absence of any external stimuli) (150) or tinnitus (234). Language impairments may be more likely if an injury occurs to the left acoustic radiation as research has shown a more substantial acoustic radiation asymmetry and predicted that the more developed left acoustic radiation may be due to language processing being performed in the left hemisphere (232). One study found acoustic radiation damage in association with concussions (235), while another found increased acoustic radiation connectivity in association with years of soccer played (236); however, neither reported associations with those abnormalities in relation to concussion symptoms. Extrapolating from those concussion-related abnormalities and the normal function of the acoustic radiation, post-concussion symptoms could include somatic [e.g., noise sensitivity (149)] or cognitive symptoms [e.g., language problems (232)] (Table 3).
Table 3. A summary of 10 white matter brain regions and their associated functions and concussion-related symptoms.
The callosal body, also known as the corpus callosum, is a large commissural tract that connects the left and right hemispheres by way of more than 200 million nerve fibers (Figure 2B) (237, 238). The callosal body resides in the center of the brain and connects with and crosses many other white matter tracts (31, 238). The corpus callosum can be subdivided into anterior, middle, and posterior sections, respectively, named the genu, body, and splenium of the corpus callosum (239).
Due to its substantial inter-hemispheric connection, the callosal body is essential to most facets of cognitive function. Therefore, injury to this important white matter structure could cause a wide range of cognitive and neurological complications. These could include visual, motor, and visuospatial perception, information processing speed and ability, moral reasoning, tactile and somatosensory perception, behavior, higher cognitive functions, and learning bimanual tasks (31, 238, 240, 241). White matter injury to the callosal body following a concussion has been shown extensively in research (185, 242). Due to the vast connective importance of the corpus callosum, injury to it could result in the following post-concussion symptoms, and a review of concussion-related callosal body damage suggested that somatic (e.g., headaches, dizziness, motor control problems) and cognitive symptoms (e.g., feeling “slow” or “foggy”, difficulty concentrating, or remembering) would be present, with a more serious axonal present in women (243) (Table 3).
The cingulum, also referred to as the cingulum bundle, is a substantial white matter structure that nearly forms a complete circle within the medial cortex (Figure 2C) (33). From a sagittal perspective of either hemisphere, the cingulum encircles the corpus callosum with connections to the orbitofrontal regions before posteriorly traveling anterior to the body of the corpus callosum toward the occipital lobe, and then diving inferiorly and anteriorly toward the temporal pole (33, 244).
As a result of its structure, the cingulum is highly connected to various brain regions and has been linked to have important roles in executive control (81), attention (245), and episodic memory (Table 3) (33, 246–248). Additionally, the cingulum has also been linked to pain sensation processing (33, 94) and the development of psychosis or schizophrenic behavior (249), obsessive-compulsive (33, 250), anxiety (251), and depression disorders (252, 253). This is of interest specific to post-concussion assessment because anxiety and depression commonly occur following concussions (254, 255). A recent study found that concussed adolescents had significantly higher anxiety which was also associated with lower neurite density index in their bilateral cingulum and bilateral forceps minor, with older female adolescents with the cingulum differences (256). Another study found that adults with persistent concussion symptoms had significantly reduced fractional anisotropy of their cingulum which also significantly correlated with the number of symptoms and the self-paced saccades task outcome (257). Additionally, one study found that concussed adolescents who were experiencing lower sleep quality had lower white matter neurite density index across 18 of the 19 tracts examined, with significant findings present in the cingulum, optic radiation, and the superior longitudinal fasciculus (258). Finally, a study found a complex set of cingulum bundle abnormalities in concussed adolescents that was correlated with compromised memory and learning scores (259). Thus, post-concussion symptoms related to cingulum injury could be somatic [e.g., bodily pain (94)], cognitive [e.g., Feeling “slow” and difficulty remembering (259)], emotional [e.g., nervousness and anxiousness (256, 257)], or sleep symptoms [e.g., trouble falling asleep and sleeping more or less than usual (258)] (Table 3).
The corticospinal tracts are well-documented bilateral white matter structures that descend from the motor cortex, travel through the medullary pyramid in the brainstem, and then cross to continue descending contralaterally down the spinal cord to the dorsolateral funiculus (Figure 2D) (260). Thus, the left and right corticospinal tracts travel contralaterally within the spinal cord.
The corticospinal tracts are essential to motor control including spinal reflexes and motor neuron control (261). Thus, one of the primary deficits associated with impairment of this region is reduced voluntary motor control (262, 263). With a concussion, injury to the corticospinal tracts could affect motor control from the neck down. Injury to the corticospinal tracts, within the brain or spinal cord, could also lead to ipsilaterally impaired proprioception, paralysis, decreased muscle tone, spasticity, power production, and mass (264, 265). A study on white matter integrity in retired professional American-style football players found significantly increased axial diffusivity in the superior longitudinal fasciculus, corticospinal tract, and anterior thalamic radiations; however, the neuropsychological function was only compared to the superior longitudinal fasciculus (266). Although not directly compared, the retired athletes reported worse memory, executive function, language, sensory, behavior, constitutional, and headache scores (266). Post-concussion, injury of the corticospinal tract could manifest primarily as somatic symptoms[e.g., headaches, balance problems, and motor control problems (261, 262, 266)], but could also present as cognitive [e.g., feeling “foggy” and language problems (266)] or emotional symptoms [e.g., irritability (266)] (Table 3).
The fornix is a thin, arched white matter structure within the medial aspect of the cerebral hemispheres (Figure 2E) (79, 267). Due to the arched structure, the fornix can be separated into several sections including the alveus, subiculum, fimbria, crura, body, and columns (267). The fornix is a major hippocampal output tract and resultantly travels from the medial temporal lobe regions, where the alveus is formed medially to the inferior aspect of the temporal horn of the lateral ventricle (267). The alveus bundles together to form the fimbria, which curves posteriorly and superiorly, before forming the crura which curves anteriorly and superiorly (79, 267). The forneal crura travel underneath the splenium of the corpus callosum and project to connect to form the structure known as the dorsal hippocampal commissure (79, 267). The crura come together and form the forneal body, which arches superior to the thalamus and travels anteriorly before splitting, at the anterior commissure, into the left and right columns that descend into the anterior forebrain (79, 267).
As the primary white matter structure connected to the hippocampus, the fornix is closely related to memory and learning capabilities. Thus, damage to the fornix could involve decreased episodic memory function, learning capabilities, and attention impairment (Table 3) (79, 267). Several concussion and mild traumatic brain injury studies have found decreased fornix microstructural integrity and volume following an injury that was correlated with injury severity (268–270). Furthermore, atrophy of the fornix has been linked to several neurodegenerative diseases such as Alzheimer's Disease, Parkinson's Disease, Multiple Sclerosis, epilepsy, and schizophrenia (79). A study on adults with persistent concussion symptoms found the fornix to have significantly reduced fractional anisotropy compared to healthy controls and was significantly correlated with lower processing speed and reaction time (271). Another study on adult women∧ with persistent concussion symptoms found that reduced FA values were correlated with higher total Graded Symptom Scale Checklist scores (272). Finally, a study by de Souza et al. found that decreased fractional anisotropy and increased mean diffusivity were correlated with worse executive function and immediate, visual, and verbal memory performances (273). Based on the healthy function of the fornix and the results of those concussion studies, a concussion-related fornix injury could present as cognitive symptoms [e.g., feeling “slow” or “foggy”, difficulty concentrating, or remembering (271, 273)] (Table 3).
The inferior occipito-frontal fascicle is one of the long and highly connected white matter bundles in the human brain, however, its distinction from other white matter structures has been a point of controversy for decades (Figure 2F) (274, 275). Fortunately, the evolution of diffusion magnetic resonance imaging (dMRI) has recently allowed for highly detailed fiber tracking of the inferior occipito-frontal fascicle that can be corroborated with cadaveric brain dissections (123, 275, 276). The posterior aspect of the inferior occipito-frontal fascicle originates in the lateral, inferior portion of the occipital lobe and travels through the occipital lobe lateral to the ventricle horns (123, 276). The tract remains lateral through the temporal lobe before veering medially into the anterior portion of the insular short gyri and terminating anteriorly in the orbitofrontal cortex (123, 276). This white matter tract is also close in proximity and functional involvement to the inferior longitudinal fasciculus.
Based on its anterior-to-posterior anatomical structure, the inferior occipito-frontal fascicle is associated with many important functions that can involve anatomically distant regions (123). Furthermore, dMRI studies have found the inferior occipito-frontal fascicle to be specifically involved in various tasks. Due to its anterior connections within the frontal lobe, Brodmann's Area (BA) 10, the inferior occipito-frontal fascicle is associated with many complex cognitive functions such as social cognition, episodic memory, attention, and multitasking (123, 191). Obsessive-compulsive disorder, and its associated behavioral-cognitive flexibility, executive function, and decision-making deficits, has been linked to the inferior occipito-frontal fascicle's connection of the frontal lobe with the temporal and occipital lobes (123, 277, 278). Additionally, the fronto-temporal fiber section of the inferior occipito-frontal fascicle is associated with language and hearing, where the left region connects to Broca–Wernicke language centers (279) and is affected by auditory verbal hallucinations in individuals with schizophrenia (280). Finally, there has been some evidence of the inferior occipito-frontal fascicle being implicated with visual conceptualization and recognition (124). To the best of our knowledge, no study has directly explored post-concussion symptoms in specific relation to the inferior occipito-frontal fascicle. Therefore, potential post-concussion symptoms are extrapolated from the normal function of this white matter tract. Thus, injury to the inferior occipito-frontal fascicle could present as somatic (e.g., visual problems) or various cognitive symptoms [e.g., feeling “slow” or “foggy”, difficulty concentrating or remembering (123, 191), or language problems (279)] (Table 3).
Optic radiation is a vital white matter tract responsible for transmitting visual information from the eye to the visual cortex in the occipital lobe (Figure 2G) (281). Optic radiation is a hook-shaped white matter structure that originates at the lateral geniculate nucleus, a transfer point in the thalamus receiving visual information from the optic tracts and terminates in the primary visual cortices in the occipital lobe (281).
The visual information transmitted to the primary visual cortex via optic radiation is contralateral to the eyes. Thus, the main deficit associated with optic radiation damage is visual impairment (282). An injury to the optic radiation can lead to decreased visual field and light perception (283, 284) and reduced retinal function (285). A case study on two mild traumatic brain injury patients with complex visual field loss had significantly abnormal volume and diffusion characteristics (286). Another study found that adults with persistent symptoms after a mild traumatic brain injury experienced significantly reduced fractional anisotropy of the optic radiation that correlated with light sensitivity (287). Finally, a study on visuomotor function post-concussion found that significantly reduced optic radiation fractional anisotropy correlated with poorer visuomotor performance (288). Based on the normal role of the optic radiation and the previously mentioned concussion studies, a concussion could injury the optic radiation and present with somatic symptoms [e.g., balance problems, motor control problems (288), sensitivity to light, or visual problems (286, 287)] (Table 3).
The superior longitudinal fasciculus is another large white matter structure that due to its many branches and tracts has left researchers and clinicians with some ambiguity surrounding its exact anatomy (Figure 2H) (289). Generally, the superior longitudinal fasciculus connects most cortical regions of the parietal lobe to the frontal lobe, with some temporal connections as well (289). Due to the numerous names associated with the tracts and segments of the superior longitudinal fasciculus, one recent study (289) proposed a simplified naming convention separating the superior longitudinal fasciculus into four segments named dorsal, ventral, posterior, and arcuate fasciculus segments (289–292). The dorsal segment would be what has been previously referred to as the superior longitudinal II, the ventral segment to the arcuate fasciculus anterior and superior longitudinal fasciculus III, the posterior segment to the arcuate fasciculus posterior and temporoparietal segment of the superior longitudinal fasciculus, and the arcuate fasciculus to the arcuate fasciculus or the arcuate fasciculus long segment (289). The dorsal segment originates in the inferior parietal lobe and terminates in the superior and middle frontal gyri, while the ventral segment also originates in the inferior parietal lobe, slightly anterior and inferior to the dorsal segment, and terminates in the middle and inferior frontal gyri (289). The posterior segment originates in the superior, middle, and inferior temporal gyri and terminates within the inferior and superior parietal areas (289). Finally, the arcuate fasciculus segment originates across the superior, middle, and inferior temporal gyri before traveling posteriorly and arcing around the Sylvian fissure and insula to terminate anteriorly in the posterior aspects of the inferior and middle frontal gyri (289). To note also, the inferior longitudinal fasciculus follows a similar path through the brain as the superior longitudinal fasciculus and is often examined in the context of concussions.
Based on the four-segment naming convention proposed by Nakajima et al., each segment can be related to specific cognitive functions related to the cortical regions it connects (Table 3) (289). As proposed by Nakajima et al. and based on previous literature, the function of each superior longitudinal fasciculus segment can be classified as bilaterally or hemisphere-specific (289). The dorsal segment is involved in visuospatial attention in the right hemisphere and bilaterally in motor control, the ventral segment is involved in attention and social cognition in the right hemisphere, language, auditory comprehension, and articulation processing in the left hemisphere, and motor control bilaterally, the posterior segment is involved in auditory and visuospatial comprehension in the right hemisphere and auditory comprehension, reading and lexical access in the left hemisphere, and the arcuate fasciculus is involved in social cognition and visuospatial cognition in the right hemisphere and phonological language processing in the left hemisphere (289). A recent study on varying degrees of traumatic brain injuries found that the fractional anisotropy of superior longitudinal fasciculus was positively correlated with executive function, memory, and attention (293). Therefore, in summary, common post-concussion symptoms related to superior longitudinal fasciculus would include some somatic symptoms [e.g., motor control (289)], but primarily cognitive symptoms [e.g., feeling “slow” or “foggy”, difficulty concentrating or remembering, or language problems (289, 293)] (Table 3).
The superior occipito-frontal fascicle is a long association white matter tract that connects the frontal and occipital cortices (Figure 2I). The tract travels parallel to the corticospinal tracts and corpus callosum between the corticospinal tracts and the lateral ventricles, and inferiorly to the corpus callosum (294, 295). Anterior and posterior to the corpus callosum, the superior occipito-frontal fascicle projects superiorly (295).
Due to the location, length, and connection of the frontal and occipital cortices, the superior occipito-frontal fascicle is associated with several functions. A study of 90 awake glioma craniotomy patients found that the superior occipito-frontal fascicle had mapping points associated with specific characteristics for speech disorder (27.2%), motor disorder (24.7%), language disorder (16.1%), sensory disorder (15%), and several other functions with less distinction (295). The study also found that the superior occipito-frontal fascicle was positively associated with the visual field, visuospatial cognition, and spatial working memory (295). Another study found that young adults with Multiple Sclerosis had reduced processing speed and simple reaction time correlated with negative abnormalities in their superior occipito-frontal fascicle, corpus callosum, and corticospinal tracts (296). Thus, confirming that the superior occipital-frontal fascicle relays important information among the visual, motor, and executive functioning brain regions (296). Similar to the inferior occipito-frontal fascicle, there has been little to no research to this point on the direct effects of concussion on the superior occipito-frontal fascicle. Therefore, based on the healthy role of this brain structure and symptom presentation found in relation to abnormalities from other conditions, the damage could present as somatic [e.g., balance problems, visual problems, sensitivity to light, motor control problems (295)] or cognitive symptoms [e.g., feeling “slow” or “foggy”, difficulty concentrating, or language problems (296)] (Table 3).
The uncinate fascicle is an important white matter tract that connects the temporal cortex with the prefrontal cortex (Figure 2J). The structure originates in the temporal pole and travels posteriorly to the amygdala before the body of the uncinate fascicle curves superiorly through the external capsule medial to the insular cortex, and then has a unique hook shape to turn antero-medially toward the prefrontal cortex (297–299). The body of the uncinate fascicle then branches in three directions toward the lateral orbital gyri, frontopolar cortex, and subgenual cingulate cortex (297, 298).
Based on the anatomical location of this structure and its close connection to the prefrontal cortex and amygdala, it has been shown that the uncinate fascicle is involved in mood regulation, emotional expression, and depression (252, 297), and even problems interpreting facial expressions (300). The uncinate fascicle also passes close to the hippocampus and due to its presence in the temporal lobe has been associated with learning and memory (301, 302). Furthermore, it has also been linked to language due to its position within the parietal lobe (82, 301, 303). One study found that the uncinate fascicle had significantly decreased fractional anisotropy post-mild traumatic brain injury correlated with worse memory test performances (304). Other studies have found uncinate fascicle damage that correlated with attention, memory, cognitive reaction time, and learning in concussion or mild traumatic brain injury patients (305–307). Therefore, a concussion-related injury to the uncinate fascicle would likely be exhibited as cognitive symptoms [e.g., feeling “slow” or “foggy”, difficulty concentrating or remembering, and confusion (304–307)] (Table 3).
The cerebellum is a unique part of the brain that is located posteriorly within the skull, inferior to the occipital lobe. Despite the cerebellum being substantially smaller than the cerebrum, it has been shown to contain about four times as many cells as the entire cerebrum (308). Similar to the cerebrum, the cerebellum is separated into two hemispheres by the cerebellar vermis and consists of three lobes; the anterior, posterior, and flocculonodular lobes (26, 308, 309). Each lobe is also separated into lobules, which can be further separated into folia (308). The cerebellum has 12 lobules known as I, II, III, IV, V, VI, Crus I, Crus II, VIIb, VIII, IX, and X on each cerebellar hemisphere (Figure 3) (26, 309). These lobule regions are organized with distinctions between lobules from the superior to the inferior external surface (26, 309). There are also an additional set of cerebellar regions that are located along the proximal and medial aspect of the cerebellum between the two lobes, known as the cerebellar vermis (26, 309), but they will not be discussed in this review as they are smaller and have had little concussion-related research examining them to date.
From a cognitive and functional perspective, our understanding of the cerebellum has undergone a revolution. For around 200 years, the cerebellum was believed to be strictly involved with motor control (3). However, the advent of functional medical imaging techniques has allowed for the realization that the cerebellum is involved in motor control, language, attention, working memory, emotion, and social processing (3, 310, 311). Functional MRI studies have shown that the cerebellum and its lobules can be subdivided into two motor regions and three non-motor regions (267). Based on a summary article by Guell and Schmahmann, lobules I–VI make up the first motor region, lobule VI and crus I make up the first non-motor region, crus II and VIIb make up the second non-motor region, lobule VIII makes up the second motor region, and lobules XI and X make up the third non-motor region (310). Although the primary functions of the cerebellum, motor, attentional/executive, and default mode network activation, are expressed quite generally across the lobules, the less involved functions of emotional, vestibular, language, and social processing are exhibited in more specific cerebellar regions (Table 4) (310). Emotional processing has been found close to the cerebellar vermis and thus is more associated with medial aspects of the lobules VI, crus I, and crus II (310, 312). Vestibular activation has been found in the verbal aspects of lobules crus I, crus II, and VII, and lobules IX and X (313); however, this activation may overlap with visual, emotional, and other motor functions (310). Similar to the lateralization of the cerebrum, language activation is lateralized contralaterally to the cerebrum and found in the right cerebellar hemisphere (310, 314). Finally, social cognition overlaps greatly with the default mode network activation in the cerebellum, which can be generally seen in lobules crus I, crus II, XI, and X (310, 312).
Table 4. Cerebellar regions and their associated functions and concussion-related symptoms are based primarily on the summary provided by Guell and Schmahmann (310).
Concussions have been linked to cerebellar abnormalities. Functional connectivity of the cerebellum is significantly associated with the number of previous concussions some have had and concussion recovery time (315). A recent study on symptomatic and asymptomatic acutely concussed youth found that cerebellar inflammation was associated with acute symptom severity and that the cerebellum had significantly increased functional connectivity with the precuneus and inferior parietal lobule which was not present in asymptomatic concussed participants (316). Meanwhile, an older study by Jantzen et al. found that cerebellar abnormalities in concussed college football players were associated with worse movement sequencing, working memory, and motor control performance (317). However, due to the vast number of functions associated with the cerebellum, further research is required to determine the risk of injury to the cerebellum during a concussive event. However, common post-concussion symptoms could be somatic [e.g., balance problems, fatigue, bodily pain, motor control problems (186, 317)], cognitive [e.g., feeling “slow”, difficulty concentrating (310, 317)], or emotional in nature [e.g., irritability, feeling more emotional (310, 314)] (Table 4).
This review aimed to highlight the intimate connection between post-concussion symptoms in the event of concussion-related damage to specific brain regions. Complete incorporation of all brain structures and post-concussion symptoms was not feasible, especially considering spatial resolution limitations of medical imaging techniques for very small brain regions, and thus this review is understandably not exhaustive at that scale. Further research is also required to connect common post-concussion symptoms more concretely to specific brain regions.
The diagnosis of a concussion is primarily based on emergency physician examination, patients reporting their symptoms, and potentially basic medical imaging, but could include further testing if more serious brain damage is suspected (5). Despite efforts to improve concussion recognition and management protocols (4, 318), diagnosis can still be highly variable due to the subjectivity of patient self-reporting, varying concussion assessment guidelines, and clinician interpretation (319). Some of the tests used to diagnose concussions can be self-administered, while some must be administered by a trained clinician (320, 321). Commonly used concussion diagnosis tests include Immediate Post-Concussion Assessment and Cognition Tool (ImPACT) (322), Sport-related Concussion Assessment Tool-−5th Edition (SCAT5) (323), CogSport (324), the Post-Concussion Symptom Scale (28), and the Rivermead Post-Concussion Symptoms Questionnaire (325), with others described in detailed review articles (326).
Although symptom-based diagnosis and recovery tracking remains the clinical gold standard, structural computed tomography (CT) and magnetic resonance imaging (MRI) scans are used to rule out skull fractures and bleeding in more serious concussion and traumatic brain injury cases (327). However, advances in MRI have provided powerful insights into brain function following a concussion using safe, non-invasive, objective, and reproducible methods. Routine clinical 3-dimensional T1-weighted and T2-weighted MRI scan protocols usually fail to identify any concussion-related damage (327). Susceptibility-weighted imaging (SWI) might depict sheering and microbleed injuries.
The MRI scan techniques used in research with promising clinical potential include diffusion MRI (dMRI), functional MRI (fMRI), MR spectroscopy (MRS), and arterial spin labeling (ASL). Those techniques have all been shown to identify microstructural, functional, metabolic, or tissue perfusion alterations, respectively, in acute and chronic concussion patients (27, 327). Instead of MRI being limited to ruling out serious brain bleeding and skull fractures, symptom-based testing can be supplemented by highly sensitive MRI techniques. These more advanced MRI techniques can provide extensive information but are not yet implemented for clinical concussion diagnoses. Incorporation of these techniques can inform clinicians of a concussion patient's current brain health, track recovery, and objectify post-concussion symptoms (i.e., nausea) with data acquired from highly reproducible MRI scans and post-scan analyses.
The heterogeneity of concussions has challenged clinicians and patients alike. However, connecting the functional and anatomical brain characteristics may highlight opportunities for personalized treatments and be useful in determining an individual concussion patient's recovery prognosis. Unfortunately, identifying that a concussion patient is suffering from light sensitivity and has visual cortex and optic radiation abnormalities, for example, currently does not guarantee their complete recovery. However, the summed knowledge we present here aims to allow clinicians and researchers alike to focus their efforts on developing injury and patient-specific treatment options.
ED, NS, DK, and MN were involved in the conceptualization of this work. ED and NS were responsible for the investigation and collection of information, as well as the writing of the original draft. ED, NS, CD, DK, SU, and MN were involved in the editing and review of the manuscript. ED was responsible for project administration. MN was responsible for supervision. ED and MN were responsible for the visualization of the published article. All authors contributed to the article and approved the submitted version.
The content of this article was partially included as the review chapter in the Ph.D. thesis of ED (328).
MN, DK, and NS have ownership in a data analytics company called TBIfinder (https://tbifinder.com) that specializes in personalized concussion assessment. ED is a research intern with TBIFinder Inc.
The remaining authors declare that the research was conducted in the absence of any commercial or financial relationships that could be construed as a potential conflict of interest.
All claims expressed in this article are solely those of the authors and do not necessarily represent those of their affiliated organizations, or those of the publisher, the editors and the reviewers. Any product that may be evaluated in this article, or claim that may be made by its manufacturer, is not guaranteed or endorsed by the publisher.
1. von Bartheld CS, Bahney J, Herculano-Houze S. The search for true numbers of neurons and glial cells in the human brain: a review of 150 years of cell counting. J Comp Neurol. (2016) 524:3865–95. doi: 10.1002/cne.24040
2. Forstmann BU, Wagenmakers E-J. (eds). An Introduction to Model-Based Cognitive Neuroscience. New York, NY: Springer New York (2015).
3. Schmahmann JD, Guell X, Stoodley CJ, Halko MA. The theory and neuroscience of cerebellar cognition. Annu Rev Neurosci. (2019) 42:337–64. doi: 10.1146/annurev-neuro-070918-050258
4. McCrory P, Meeuwisse W, Dvorak J, Aubry M, Bailes J, Broglio S, et al. Consensus statement on concussion in sport—the 5th international conference on concussion in sport held in Berlin, October 2016. Br J Sports Med. (2017) 51:838–47. doi: 10.1136/bjsports-2017-097699
5. Hon KL, Leung AK, Torres AR. Concussion: a global perspective. Sem Ped Neurol. (2019) 30:117–27. doi: 10.1016/j.spen.2019.03.017
6. Zuckerman SL, Lee YM, Odom MJ, Solomon GS, Forbes JA, Sills AK. Recovery from sports-related concussion: days to return to neurocognitive baseline in adolescents versus young adults. Surg Neurol Int. (2012) 3:130. doi: 10.4103/2152-7806.102945
7. Cassidy JD, Carroll L, Peloso P, Borg J, von Holst H, Holm L, et al. Incidence, risk factors and prevention of mild traumatic brain injury: results of the who collaborating centre task force on mild traumatic brain injury. J Rehabil Med. (2004) 36:28–60. doi: 10.1080/16501960410023732
8. Giza CC, Hovda DA. The new neurometabolic cascade of concussion. Neurosurg. (2014) 75:S24–33. doi: 10.1227/NEU.0000000000000505
9. Bayly PV, Cohen TS, Leister EP, Ajo D, Leuthardt EC, Genin GM. Deformation of the human brain induced by mild acceleration. J Neurotrauma. (2005) 22:845–56. doi: 10.1089/neu.2005.22.845
10. Shrey DW, Griesbach GS, Giza CC. The pathophysiology of concussions in youth. Phys Med Rehabil Clin N Am. (2011) 22:577–602. doi: 10.1016/j.pmr.2011.08.002
11. Wang Y, West JD, Bailey JN, Westfall DR, Xiao H, Arnold TW, et al. Decreased cerebral blood flow in chronic pediatric mild TBI: An MRI perfusion study. Dev Neuropsychol. (2015) 40:40–4. doi: 10.1080/87565641.2014.979927
12. Ogawa S, Lee TM, Nayak AS, Glynn P. Oxygenation-sensitive contrast in magnetic resonance image of rodent brain at high magnetic fields. Mag Res Med. (1990) 14:68–78. doi: 10.1002/mrm.1910140108
13. Churchill NW, Hutchison MG, Richards D, Leung G, Graham SJ, Schweizer TA. The first week after concussion: blood flow, brain function and white matter microstructure. NeuroImage Clin. (2017) 14:480–9. doi: 10.1016/j.nicl.2017.02.015
14. Cassoudesalle H, Petit A, Chanraud S, Petit H, Badaut J, Sibon I, et al. Changes in resting-state functional brain connectivity associated with head impacts over one men's semi-professional soccer season. J Neurosci Res. (2020) 99:446–454. doi: 10.1002/JNR.24742/v3/response1
15. Eickhoff SB, Stephan KE, Mohlberg H, Grefkes C, Fink GR, Amunts K, et al. A new SPM toolbox for combining probabilistic cytoarchitectonic maps and functional imaging data. Neuroimage. (2005) 25:1325–35. doi: 10.1016/j.neuroimage.2004.12.034
16. Eickhoff SB, Heim S, Zilles K, Amunts K. Testing anatomically specified hypotheses in functional imaging using cytoarchitectonic maps. Neuroimage. (2006) 32:570–82. doi: 10.1016/j.neuroimage.2006.04.204
17. Eickhoff SB, Paus T, Caspers S, Grosbras M-H, Evans AC, Zilles K, et al. Assignment of functional activations to probabilistic cytoarchitectonic areas revisited. NeuroImage. (2007) 36:511–21. doi: 10.1016/j.neuroimage.2007.03.060
18. Hua K, Zhang J, Wakana S, Jiang H, Li X, Reich DS, et al. Tract probability maps in stereotaxic spaces: analyses of white matter anatomy and tract-specific quantification. Neuroimage. (2008) 39:336–47. doi: 10.1016/j.neuroimage.2007.07.053
19. Mori S, Wakana S, Nagae-Poetscher LM, van Zijl PCM. MRI Atlas of Human White Matter. 1st ed. Amsterdam: Elsevier (2005).
20. Wakana S, Caprihan A, Panzenboeck MM, Fallon JH, Perry M, Gollub RL, et al. Reproducibility of quantitative tractography methods applied to cerebral white matter. Neuroimage. (2007) 36:630–44. doi: 10.1016/j.neuroimage.2007.02.049
21. Brett BL, Bobholz SA, España LY, Huber DL, Mayer AR, Harezlak J, et al. Cumulative effects of prior concussion and primary sport participation on brain morphometry in collegiate athletes: a study from the NCAA–DoD CARE Consortium. Front Neurol. (2020) 11:673. doi: 10.3389/fneur.2020.00673
22. Danielli E, Simard N, Sharma B, Doughty M, Noseworthy MD. Functional, but minimal microstructural brain changes present in aging Canadian football league players years after retirement. Brain Disord. (2022) 6:100036. doi: 10.1016/j.dscb.2022.100036
23. Lancaster MA, Meier TB, Olson DV, McCrea MA, Nelson LD, Muftuler LT. Chronic differences in white matter integrity following sport-related concussion as measured by diffusion MRI: 6-Month follow-up. Hum Brain Mapp. (2018) 39:4276–89. doi: 10.1002/hbm.24245
24. Stillo D, Danielli E, Ho RA, DeMatteo C, Hall GB, Bock NA, et al. Localization and identification of brain microstructural abnormalities in paediatric concussion. Front Hum Neurosci. (2021) 15:657374. doi: 10.3389/fnhum.2021.657374
25. Khetani A, Rohr CS, Sojoudi A, Bray S, Barlow KM. Alteration in cerebral activation during a working memory task after pediatric mild traumatic brain injury: a prospective controlled cohort study. J Neurotrauma. (2019) 36:3274–83. doi: 10.1089/neu.2018.6117
26. Diedrichsen J, Balsters JH, Flavell J, Cussans E, Ramnani N. A probabilistic MR atlas of the human cerebellum. Neuroimage. (2009) 46:39–46. doi: 10.1016/j.neuroimage.2009.01.045
27. Daneshvar DH, Riley DO, Nowinski CJ, McKee AC, Stern RA, Cantu RC. Long-term consequences: effects on normal development profile after concussion. Phys Med Rehabil Clin N America. (2011) 22:683–700. doi: 10.1016/j.pmr.2011.08.009
28. Kontos AP, Elbin R, Schatz P, Covassin T, Henry L, Pardini J, et al. A revised factor structure for the Post-Concussion Symptom Scale: baseline and postconcussion factors. Am J Sports Med. (2012) 40:2375–84. doi: 10.1177/0363546512455400
29. Tortora GJ, Derrickson BH. Tortora's Principles of Anatomy & Physiology. New Jersey, NJ: Wiley (2017).
30. Heilbronner SR, Haber SN. Frontal cortical and subcortical projections provide a basis for segmenting the cingulum bundle: implications for neuroimaging and psychiatric disorders. J Neurosci. (2014) 34:10041–54. doi: 10.1523/JNEUROSCI.5459-13.2014
31. Kollias S. Parcelation of the white matter using DTI: insights into the functional connectivity of the brain. J Neuroradiol. (2009) 22:74–84. doi: 10.1177/19714009090220S114
32. Steele JD, Lawrie SM. Segregation of cognitive and emotional function in the prefrontal cortex: a stereotactic meta-analysis. Neuroimage. (2004) 21:868–75. doi: 10.1016/j.neuroimage.2003.09.066
33. Bubb EJ, Metzler-Baddeley C, Aggleton JP. The cingulum bundle: anatomy, function, and dysfunction. Neurosci Biobehav Rev. (2018) 92:104–27. doi: 10.1016/j.neubiorev.2018.05.008
34. Comes-Fayos J, Romero-Martínez Á, Moya-Albiol L. Role of major long fiber tracts association in empathy. Rev Neurol. (2018) 67:263–72. doi: 10.33588/rn.6707.2017468
35. Olson IR. Heide RJVD, Alm KH, Vyas G. Development of the uncinate fasciculus: implications for theory and developmental disorders. Dev Cog Neurosci. (2015) 14:50–61. doi: 10.1016/j.dcn.2015.06.003
36. Waller R, Dotterer HL, Murray L, Maxwell AM, Hyde LW. White-matter tract abnormalities and antisocial behavior: a systematic review of diffusion tensor imaging studies across development. NeuroImage Clin. (2017) 14:201–15. doi: 10.1016/j.nicl.2017.01.014
37. Stephani C, Fernandez-Baca Vaca G, Maciunas R, Koubeissi M, Lüders HO. Functional neuroanatomy of the insular lobe. Brain Struct Funct. (2011) 216:137–49. doi: 10.1007/s00429-010-0296-3
38. Dalton KM, Nacewicz BM, Johnstone T, Schaefer HS, Gernsbacher MA, Goldsmith HH, et al. Gaze fixation and the neural circuitry of face processing in autism. Nat Neurosci. (2005) 8:519–26. doi: 10.1038/nn1421
39. Kleinhans NM, Johnson LC, Richards T, Mahurin R, Greenson J, Dawson G, et al. Reduced neural habituation in the amygdala and social impairments in autism spectrum disorders. Am J Psychiatry. (2009) 166:467–75. doi: 10.1176/appi.ajp.2008.07101681
40. Nacewicz BM, Dalton KM, Johnstone T, Long MT, McAuliff EM, Oakes TR, et al. Amygdala volume and nonverbal social impairment in adolescent and adult males with autism. Arch Gen Psychiatry. (2006) 63:1417–28. doi: 10.1001/archpsyc.63.12.1417
41. Steinberg L. A dual systems model of adolescent risk-taking. Dev Psychobiol. (2010) 52:216–24. doi: 10.1002/dev.20445
42. Von Der Heide RJ, Skipper LM, Klobusicky E, Olson IR. Dissecting the uncinate fasciculus: disorders, controversies and a hypothesis. Brain. (2013) 136:1692–707. doi: 10.1093/brain/awt094
44. Bohorquez-Montoya L, España LY, Nader AM, Furger RE, Mayer AR, Meier TB. Amygdala response to emotional faces in adolescents with persistent post-concussion symptoms. NeuroImage Clin. (2020) 26:102217. doi: 10.1016/j.nicl.2020.102217
45. Stocker RPJ, Cieply MA, Paul B, Khan H, Henry L, Kontos AP, et al. Combat-related blast exposure and traumatic brain injury influence brain glucose metabolism during REM sleep in military veterans. Neuroimage. (2014) 99:207–14. doi: 10.1016/j.neuroimage.2014.05.067
46. Dumkrieger G, Chong CD, Ross K, Berisha V, Schwedt TJ. Static and dynamic functional connectivity differences between migraine and persistent post-traumatic headache: A resting-state magnetic resonance imaging study. Cephalalgia. (2019) 39:1366–81. doi: 10.1177/0333102419847728
47. Colby CL, Gattass R, Olson CR, Gross CG. Topographical organization of cortical afferents to extrastriate visual area PO in the macaque: a dual tracer study. J Comp Neurol. (1988) 269:392–413. doi: 10.1002/cne.902690307
48. Scheperjans F, Hermann K, Eickhoff SB, Amunts K, Schleicher A, Zilles K. Observer-independent cytoarchitectonic mapping of the human superior parietal cortex. Cereb Cortex. (2008) 18:846–67. doi: 10.1093/cercor/bhm116
49. Ramayya AG, Glasser MF, Rilling JK. A DTI investigation of neural substrates supporting tool use. Cereb Cortex. (2010) 20:507–16. doi: 10.1093/cercor/bhp141
50. Anwander A, Tittgemeyer M, von Cramon D, Friederici A, Knosche T. Connectivity-based parcellation of Broca's Area. Cerebral Cortex. (2007) 17:816–25. doi: 10.1093/cercor/bhk034
51. Graziano MSA, Cooke DF. Parieto-frontal interactions, personal space, and defensive behavior. Neuropsychologia. (2006) 44:845–59. doi: 10.1016/j.neuropsychologia.2005.09.009
52. Burks JD, Boettcher LB, Conner AK, Glenn CA, Bonney PA, Baker CM, et al. White matter connections of the inferior parietal lobule: a study of surgical anatomy. Brain Behav. (2017) 7:e00640. doi: 10.1002/brb3.640
53. Avillac M, Denéve S, Olivier E, Pouget A. Duhamel J-R. Reference frames for representing visual and tactile locations in parietal cortex. Nat Neurosci. (2005) 8:941–9. doi: 10.1038/nn1480
54. Prado J, Clavagnier S, Otzenberger H, Scheiber C, Kennedy H, Perenin, M.-T. Two cortical systems for reaching in central and peripheral vision. Neuron. (2005) 48:849–58. doi: 10.1016/j.neuron.2005.10.010
55. Chen X, DeAngelis GC, Angelaki DE. Flexible egocentric and allocentric representations of heading signals in parietal cortex. Proc Natl Acad Sci USA. (2018) 115:E3305-12. doi: 10.1073/pnas.1715625115
56. Bremmer F, Schlack A, Shah NJ, Zafiris O, Kubischik M, Hoffmann K-P, et al. Polymodal motion processing in posterior parietal and premotor cortex: a human fMRI study strongly implies equivalencies between humans and monkeys. Neuron. (2001) 29:287–96. doi: 10.1016/S0896-6273(01)00198-2
57. Murata A, Gallese V, Luppino G, Kaseda M, Sakata H. Selectivity for the shape, size, and orientation of objects for grasping in neurons of monkey parietal area AIP. J Neurophysiol. (2000) 83:2580–601. doi: 10.1152/jn.2000.83.5.2580
58. Shikata E, Hamzei F, Glauche V, Koch M, Weiller C, Binkofski F, et al. Functional properties and interaction of the anterior and posterior intraparietal areas in humans. Eur J Neurosci. (2003) 17:1105–10. doi: 10.1046/j.1460-9568.2003.02540.x
59. Aziz-Zadeh L, Wilson SM, Rizzolatti G, Iacoboni M. Congruent embodied representations for visually presented actions and linguistic phrases describing actions. Curr Biol. (2006) 16:1818–23. doi: 10.1016/j.cub.2006.07.060
60. Iacoboni M, Dapretto M. The mirror neuron system and the consequences of its dysfunction. Nat Rev Neurosci. (2006) 7:942–51. doi: 10.1038/nrn2024
61. Sugihara G, Kaminaga T, Sugishita M. Interindividual uniformity and variety of the “Writing center”: a functional MRI study. Neuroimage. (2006) 32:1837–49. doi: 10.1016/j.neuroimage.2006.05.035
62. Grefkes C, Fink GR. REVIEW the functional organization of the intraparietal sulcus in humans and monkeys. J Anat. (2005) 207:3–17. doi: 10.1111/j.1469-7580.2005.00426.x
63. Sours C, Raghavan P, Medina AE, Roys S, Jiang L, Zhuo J, et al. Structural and functional integrity of the intraparietal sulcus in moderate and severe traumatic brain injury. J Neurotrauma. (2017) 34:1473–81. doi: 10.1089/neu.2016.4570
64. Leiguarda RC, Marsden CD. Limb apraxias: higher-order disorders of sensorimotor integration. Brain. (2000) 123:860–79. doi: 10.1093/brain/123.5.860
65. Binkofski F, Dohle C, Posse S, Stephan KM, Hefter H, Seitz RJ, et al. Human anterior intraparietal area subserves prehension: a combined lesion and functional MRI activation study. Neurology. (1998) 50:1253–9. doi: 10.1212/WNL.50.5.1253
66. Glover S. Separate visual representations in the planning and control of action. Behav Brain Sci. (2004) 27:3–24. doi: 10.1017/S0140525X04000020
67. Longo MR, Azañón E, Haggard P. More than skin deep: body representation beyond primary somatosensory cortex. Neuropsychologia. (2010) 48:655–68. doi: 10.1016/j.neuropsychologia.2009.08.022
68. Wolbers T, Wiener JM, Mallot HA, Buchel C. Differential recruitment of the hippocampus, medial prefrontal cortex, and the human motion complex during path integration in humans. J Neuroscience. (2007) 27:9408–16. doi: 10.1523/JNEUROSCI.2146-07.2007
69. Kravitz DJ, Saleem KS, Baker CI, Mishkin M. A new neural framework for visuospatial processing. Nat Rev Neurosci. (2011) 12:217–30. doi: 10.1038/nrn3008
70. de Schotten MT, Urbanski M, Duffau H, Volle E, Lévy R, Dubois B, et al. Direct evidence for a parietal-frontal pathway subserving spatial awareness in humans. Science. (2005) 309:2226–8. doi: 10.1126/science.1116251
71. Shinoura N, Suzuki Y, Yamada R, Tabei Y, Saito K, Yagi K. Damage to the right superior longitudinal fasciculus in the inferior parietal lobe plays a role in spatial neglect. Neuropsychologia. (2009) 47:2600–3. doi: 10.1016/j.neuropsychologia.2009.05.010
72. Perenin M-T. Vighetto A. Optic ataxia: a specific disruption in visuo-motor mechanisms: (I) different aspects of the deficity in reaching for objects. Brain. (1988) 111:643–74. doi: 10.1093/brain/111.3.643
73. Broca MP. Remarques sur le siége de la faculté du langage articulé, suivies d'une observation d'aphémie (perte de la parole). Bull Mem Soc Anat Paris. (1861) 6:330–57.
74. Maldonado IL, Moritz-Gasser S, Duffau H. Does the left superior longitudinal fasciculus subserve language semantics? A brain electrostimulation study. Brain Struct Funct. (2011) 216:263. doi: 10.1007/s00429-011-0309-x
75. Motomura K, Fujii M, Maesawa S, Kuramitsu S, Natsume A, Wakabayashi T. Association of dorsal inferior frontooccipital fasciculus fibers in the deep parietal lobe with both reading and writing processes: a brain mapping study - case report. J Neurosurg. (2014) 121:142–8. doi: 10.3171/2014.2.JNS131234
76. Bernal B, Ardila A. The role of the arcuate fasciculus in conduction aphasia. Brain. (2009) 132:2309–16. doi: 10.1093/brain/awp206
77. Friederici AD. Broca's area and the ventral premotor cortex in language: Functional differentiation and specificity. Cortex. (2006) 42:472–5. doi: 10.1016/S0010-9452(08)70380-0
78. Alexander MP, Naeser MA, Palumbo C. Broca's area aphasias: aphasia after lesions including the frontal operculum. Neurology. (1990) 40:353–353. doi: 10.1212/WNL.40.2.353
79. Douet V, Chang L. Fornix as an imaging marker for episodic memory deficits in healthy aging and in various neurological disorders. Front Aging Neurosci. (2015) 6:343. doi: 10.3389/fnagi.2014.00343
80. Vann SD, Aggleton JP. The mammillary bodies: two memory systems in one? Nat Rev Neurosci. (2004) 5:35–44. doi: 10.1038/nrn1299
81. Metzler-Baddeley C, Hunt S, Jones DK, Leemans A, Aggleton JP, O'Sullivan MJ. Temporal association tracts and the breakdown of episodic memory in mild cognitive impairment. Neurology. (2012) 79:2233–40. doi: 10.1212/WNL.0b013e31827689e8
82. Saur D, Kreher BW, Schnell S, Kümmerer D, Kellmeyer P, Vry M-S, et al. Ventral and dorsal pathways for language. Proc Natl Acad Sci USA. (2008) 105:18035–40. doi: 10.1073/pnas.0805234105
83. Weissman-Fogel I, Moayedi M, Taylor KS, Pope G, Davis KD. Cognitive and default-mode resting state networks: do male and female brains “rest” differently? Hum Brain Mapp. (2010) 31:1713–26. doi: 10.1002/hbm.20968
84. Winer JA, Lee CC. The distributed auditory cortex. Hear Res. (2007) 229:3–13. doi: 10.1016/j.heares.2007.01.017
85. Adolphs R. What does the amygdala contribute to social cognition? Annals NY Acad Sci. (2010) 1191:42–61. doi: 10.1111/j.1749-6632.2010.05445.x
86. Hyde LW, Shaw DS, Hariri AR. Understanding youth antisocial behavior using neuroscience through a developmental psychopathology lens: review, integration, and directions for research. Devel Rev. (2013) 33:168–223. doi: 10.1016/j.dr.2013.06.001
87. Spiers HJ, Maguire EA. A navigational guidance system in the human brain. Hippocampus. (2007) 17:618–26. doi: 10.1002/hipo.20298
88. Elliott R, Dolan RJ, Frith CD. Dissociable functions in the medial and lateral orbitofrontal cortex: evidence from human neuroimaging studies. Cerebral Cortex. (2000) 10:308–17. doi: 10.1093/cercor/10.3.308
89. Wilber AA, Clark BJ, Forster TC, Tatsuno M, McNaughton BL. Interaction of egocentric and world-centered reference frames in the rat posterior parietal cortex. J Neurosci. (2014) 34:5431–46. doi: 10.1523/JNEUROSCI.0511-14.2014
90. Rudebeck SR, Scholz J, Millington R, Rohenkohl G, Johansen-Berg H, Lee ACH. Fornix microstructure correlates with recollection but not familiarity memory. J Neuroscience. (2009) 29:14987–92. doi: 10.1523/JNEUROSCI.4707-09.2009
91. Petrides M. Deficits on conditional associative-learning tasks after frontal- and temporal-lobe lesions in man. Neuropsychologia. (1985) 23:601–14. doi: 10.1016/0028-3932(85)90062-4
92. Fjell AM, Sørensen Ø, Amlien IK, Bartrés-Faz D, Bros DM, Buchmann N, et al. Self-reported sleep relates to hippocampal atrophy across the adult lifespan: results from the Lifebrain consortium. Sleep. (2020) 43:zsz280. doi: 10.1093/sleep/zsz280
93. June D, Williams OA, Huang C-W, An Y, Landman BA, Davatzikos C, et al. Lasting consequences of concussion on the aging brain: findings from the Baltimore Longitudinal Study of Aging. NeuroImage. (2020) 221:117182. doi: 10.1016/j.neuroimage.2020.117182
94. Melzack R. Evolution of the Neuromatrix theory of pain. The Prithvi Raj Lecture: presented at the Third World Congress of World Institute of Pain, Barcelona 2004. Pain Pract. (2005) 5:85–94. doi: 10.1111/j.1533-2500.2005.05203.x
95. Gilboa A, Winocur G, Rosenbaum RS, Poreh A, Gao F, Black SE, et al. Hippocampal contributions to recollection in retrograde and anterograde amnesia. Hippocampus. (2006) 16:966–80. doi: 10.1002/hipo.20226
96. Fletcher E, Raman M, Huebner P, Liu A, Mungas D, Carmichael O, et al. Loss of fornix white matter volume as a predictor of cognitive impairment in cognitively normal elderly individuals. JAMA Neurol. (2013) 70:1389–95. doi: 10.1001/jamaneurol.2013.3263
97. Goukasian N, Porat S, Blanken A, Avila D, Zlatev D, Hurtz S, et al. Cognitive correlates of hippocampal atrophy and ventricular enlargement in adults with or without mild cognitive impairment. Dement Geriatr Cogn Disord Extra. (2019) 9:281–93. doi: 10.1159/000490044
98. Uysal G, Ozturk M. Hippocampal atrophy based Alzheimer's disease diagnosis via machine learning methods. J Neurosci Meth. (2020) 337:108669. doi: 10.1016/j.jneumeth.2020.108669
99. Wu K-Y, Lin K-J, Chen C-H, Chen C-S, Liu C-Y, Huang S-Y, et al. Diversity of neurodegenerative pathophysiology in non- demented patients with major depressive disorder: evidence of cerebral amyloidosis and hippocampal atrophy. Brain Behav. (2018) 8:e01016. doi: 10.1002/brb3.1016
100. Ahmed-Leitao F, Rosenstein D, Marx M, Young S, Korte K, Seedat S. Posttraumatic stress disorder, social anxiety disorder and childhood trauma: differences in hippocampal subfield volume. Psychiat Res Neuroimag. (2019) 284:45–52. doi: 10.1016/j.pscychresns.2018.12.015
101. Ruschel M, Knösche TR, Friederici AD, Turner R, Geyer S, Anwander A. Connectivity architecture and subdivision of the human inferior parietal cortex revealed by diffusion MRI. Cereb Cortex. (2014) 24:2436–48. doi: 10.1093/cercor/bht098
102. Meteyard L, Cuadrado SR, Bahrami B, Vigliocco G. Coming of age: a review of embodiment and the neuroscience of semantics. Cortex. (2012) 48:788–804. doi: 10.1016/j.cortex.2010.11.002
103. Fadiga L, Craighero L, D'Ausilio A. Broca's Area in language, action, and music. Ann NY Acad Sci. (2009) 1169:448–58. doi: 10.1111/j.1749-6632.2009.04582.x
104. Faillenot I. Visual pathways for object-oriented action and object recognition: functional anatomy with PET. Cereb Cortex. (1997) 7:77–85. doi: 10.1093/cercor/7.1.77
105. Tanaka Y, Kamo T, Yoshida M, Yamadori A. “So-called' - cortical deafness: clinical, neurophysiological and readiological observations. Brain. (1991) 114:2385–401. doi: 10.1093/brain/114.6.2385
106. Herbet G, Lafargue G, Bonnetblanc F, Moritz-Gasser S, Menjot de Champfleur N, Duffau H. Inferring a dual-stream model of mentalizing from associative white matter fibres disconnection. Brain. (2014) 137:944–59. doi: 10.1093/brain/awt370
107. Martino J, De Witt Hamer PC, Berger MS, Lawton MT, Arnold CM, de Lucas EM, et al. Analysis of the subcomponents and cortical terminations of the perisylvian superior longitudinal fasciculus: a fiber dissection and DTI tractography study. Brain Struct Funct. (2013) 218:105–21. doi: 10.1007/s00429-012-0386-5
108. Coslett HB, Schwartz MF. The parietal lobe and language. Handb Clin Neurol. (2018) 151:365–75. doi: 10.1016/B978-0-444-63622-5.00018-8
109. Binder JR, Desai RH, Graves WW, Conant LL. Where is the semantic system? A critical review and meta-analysis of 120 functional neuroimaging studies. Cereb Cortex. (2009) 19:2767–96. doi: 10.1093/cercor/bhp055
110. Solomon M, Lo YH. Visual-spatial processing: the parietal lobe in engaging a 3D world. In:Albert MV, Lin L, Spector MJ, Dunn LS., Bridging Human Intelligence and Artificial Intelligence, ed. by Educational Communications and Technology: Issues and Innovations. Cham: Springer International Publishing (2022).
111. Vallar G, Bello L, Bricolo E, Castellano A, Casarotti A, Falini A, et al. Cerebral correlates of visuospatial neglect: a direct cerebral stimulation study. Hum Brain Mapp. (2014) 35:1334–50. doi: 10.1002/hbm.22257
112. Harrington DL, Haaland KY, Knight RT. Cortical networks underlying mechanisms of time perception. J Neurosci. (1998) 18:1085–95. doi: 10.1523/JNEUROSCI.18-03-01085.1998
113. Alain C, He Y, Grady C. The contribution of the inferior parietal lobe to auditory spatial working memory. J Cog Neurosci. (2008) 20:285–95. doi: 10.1162/jocn.2008.20014
114. Talanow T, Kasparbauer A-M, Lippold JV, Weber B, Ettinger U. Neural correlates of proactive and reactive inhibition of saccadic eye movements. Brain Imag Behav. (2020) 14:72–88. doi: 10.1007/s11682-018-9972-3
115. Bonzano L, Bisio A, Pedullá L, Brichetto G, Bove M. Right inferior parietal lobule activity is associated with handwriting spontaneous tempo. Front Neurosci. (2021) 15:656856. doi: 10.3389/fnins.2021.656856
116. Buxbaum LJ. Ideomotor apraxia: a call to action. Neurocase. (2001) 7:445–58. doi: 10.1093/neucas/7.6.445
117. Griffiths TD. Central auditory pathologies. Br Med Bull. (2002) 63:107–20. doi: 10.1093/bmb/63.1.107
118. Dejerine J. Sémiologie des affections du systéme nerveux. Masson et Cie. (1914). doi: 10.1097/00005053-191511000-00029
119. Zuleger TM, Slutsky-Ganesh AB, Anand M, Kim H, Warren SM, Grooms DR, et al. The effects of sports-related concussion history on female adolescent brain activity and connectivity for bilateral lower extremity knee motor control. Psychophysiology. (2023) e14314. doi: 10.1111/psyp.14314
120. Centanni SW, Janes AC, Haggerty DL, Atwood B, Hopf FW. Better living through understanding the insula: why subregions can make all the difference. Neuropharmacol. (2021) 198:108765. doi: 10.1016/j.neuropharm.2021.108765
121. Taylor KS, Seminowicz DA, Davis KD. Two systems of resting state connectivity between the insula and cingulate cortex. Hum Brain Mapp. (2009) 30:2731–45. doi: 10.1002/hbm.20705
122. Duffau H, Capelle L, Denvil D, Gatignol P, Sichez N, Lopes M, et al. The role of dominant premotor cortex in language: a study using intraoperative functional mapping in awake patients. Neuroimage. (2003) 20:1903–14. doi: 10.1016/S1053-8119(03)00203-9
123. Wu Y, Sun D, Wang Y, Wang Y. Subcomponents and connectivity of the inferior fronto-occipital fasciculus revealed by diffusion spectrum imaging fiber tracking. Front Neuroanat. (2016) 10:88. doi: 10.3389/fnana.2016.00088
124. Sarubbo S, De Benedictis A, Maldonado IL, Basso G, Duffau H. Frontal terminations for the inferior fronto-occipital fascicle: anatomical dissection, DTI study and functional considerations on a multi-component bundle. Brain Struct Funct. (2013) 218:21–37. doi: 10.1007/s00429-011-0372-3
125. Duffau H, Bauchet L, Lehéricy S, Capelle L. Functional compensation of the left dominant insula for language. Neuroreport. (2001) 12:2159–63. doi: 10.1097/00001756-200107200-00023
126. Heath CJ, Jones EG. The Anatomical Organization of the Suprasylvian Gyrus of the Cat. New York, NY: Springer Science & Business Media (2013).
127. Uddin LQ, Supekar K, Amin H, Rykhlevskaia E, Nguyen DA, Greicius MD, et al. Dissociable connectivity within human angular gyrus and intraparietal sulcus: evidence from functional and structural connectivity. Cereb Cortex. (2010) 20:2636–46. doi: 10.1093/cercor/bhq011
128. Cantalupo C, Hopkins WD. Asymmetric Broca's area in great apes. Nature. (2001) 414:505–505. doi: 10.1038/35107134
129. Winer JA, Larue DT, Diehl JJ, Hefti BJ. Auditory cortical projections to the cat inferior colliculus. J Comp Neurol. (1998) 400:147–74. doi: 10.1002/(SICI)1096-9861(19981019)400:2<147::AID-CNE1>3.0.CO;2-9
130. Penfield W, Faulk ME. The insula: further observations on its function. Brain. (1955) 78:445–70. doi: 10.1093/brain/78.4.445
131. Brooks JCW, Zambreanu L, Godinez A, Craig AD, Tracey I. Somatotopic organisation of the human insula to painful heat studied with high resolution functional imaging. Neuroimage. (2005) 27:201–9. doi: 10.1016/j.neuroimage.2005.03.041
132. Craig A. How do you feel — now? The anterior insula and human awareness. Nat Rev Neurosci. (2009) 10:59–70. doi: 10.1038/nrn2555
133. Francis S, Kelly E, Bowtell R, Dunseath W, Folger S, McGlone F. fMRI of the responses to vibratory stimulation of digit tips. Neuroimage. (2000) 11:188–202. doi: 10.1006/nimg.2000.0541
134. Isnard J, Guénot M, Sindou M, Mauguiére F. Clinical manifestations of insular lobe seizures: a stereo-electroencephalographic study. Epilepsia. (2004) 45:1079–90. doi: 10.1111/j.0013-9580.2004.68903.x
135. Brenner L, Zerlin L, Tan LL. Functional disruption of cortical cingulate activity attenuates visceral hypersensitivity and anxiety induced by acute experimental colitis. Sci Rep. (2021) 11:2103. doi: 10.1038/s41598-021-81256-x
136. Makovac E, Dipasquale O, Jackson JB, Medina S, O'Daly O, O'Muircheartaigh J, et al. Sustained perturbation in functional connectivity induced by cold pain. Eur J Pain. (2020) 24:1850–61. doi: 10.1002/ejp.1633
137. Duffau H, Capelle L, Lopes M, Faillot T, Sichez J-P, Fohanno D. The insular lobe: physiopathological and surgical considerations. Neurosurg. (2000) 47:801–11. doi: 10.1097/00006123-200010000-00001
138. Wang G-J, Tomasi D, Backus W, Wang R, Telang F, Geliebter A, et al. Gastric distention activates satiety circuitry in the human brain. NeuroImage. (2008) 39:1824–31. doi: 10.1016/j.neuroimage.2007.11.008
139. Ruben J, Schwiemann J, Deuchert M, Meyer R, Krause T, Curio G, et al. Somatotopic organization of human secondary somatosensory cortex. Cereb Cortex. (2001) 11:463–73. doi: 10.1093/cercor/11.5.463
140. Abboud H, Berroir S, Labreuche J, Orjuela K, Amarenco P. Insular involvement in brain infarction increases risk for cardiac arrhythmia and death. Annals Neurol. (2006) 59:691–9. doi: 10.1002/ana.20806
141. Pollatos O, Schandry R, Auer DP, Kaufmann C. Brain structures mediating cardiovascular arousal and interoceptive awareness. Brain Res. (2007) 1141:178–87. doi: 10.1016/j.brainres.2007.01.026
142. Caruana F, Jezzini A, Sbriscia-Fioretti B, Rizzolatti G, Gallese V. Emotional and social behaviors elicited by electrical stimulation of the insula in the macaque monkey. Curr Biol. (2011) 21:195–9. doi: 10.1016/j.cub.2010.12.042
143. Damasio A, Damasio H, Tranel D. Persistence of feelings and sentience after bilateral damage of the insula. Cerebral Cortex. (2013) 23:833–46. doi: 10.1093/cercor/bhs077
144. Dolensek N, Gehrlach DA, Klein AS, Gogolla N. Facial expressions of emotion states and their neuronal correlates in mice. Science. (2020) 368:89–94. doi: 10.1126/science.aaz9468
145. Burton H, Videen TO, Raichle ME. Tactile-vibration-activated foci in insular and parietal-opercular cortex studied with Positron Emission Tomography: mapping the second somatosensory area in humans. Somatosens Motor Res. (1993) 10:297–308. doi: 10.3109/08990229309028839
146. Burton H, Jones EG. The posterior thalamic region and its cortical projection in new world and old world monkeys. J Compar Neurol. (1976) 168:249–301. doi: 10.1002/cne.901680204
147. Critchley HD, Wiens S, Rotshtein P, Öhman A, Dolan RJ. Neural systems supporting interoceptive awareness. Nat Neurosci. (2004) 7:189–95. doi: 10.1038/nn1176
148. Birklein F, Rolke R, Müller-Forell W. Isolated insular infarction eliminates contralateral cold, cold pain, and pinprick perception. Neurology. (2005) 65:1381–1381. doi: 10.1212/01.wnl.0000181351.82772.b3
149. Taniwaki T, Tagawa K, Sato F, Iino K. Auditory agnosia restricted to environmental sounds following cortical deafness and generalized auditory agnosia. Clin Neurol Neurosurg. (2000) 102:156–62. doi: 10.1016/S0303-8467(00)00090-1
150. Woo PY, Leung LN, Cheng ST, Chan K-Y. Monoaural musical hallucinations caused by a thalamocortical auditory radiation infarct: a case report. J Med Case Rep. (2014) 8:400. doi: 10.1186/1752-1947-8-400
151. Disbrow E, Roberts T, Krubitzer L. Somatotopic organization of cortical fields in the lateral sulcus of Homo sapiens: evidence for SII and PV. J Comp Neurol. (2000) 418:1–21. doi: 10.1002/(SICI)1096-9861(20000228)418:1<1::AID-CNE1>3.0.CO;2-P
152. Fahim C, He Y, Yoon U, Chen J, Evans A, Pérusse D. Neuroanatomy of childhood disruptive behavior disorders. Aggress Behav. (2011) 37:326–37. doi: 10.1002/ab.20396
153. Drevets WC. Neuroimaging studies of mood disorders. Biol Psychiatry. (2000) 48:813–29. doi: 10.1016/S0006-3223(00)01020-9
154. Baur V, Hänggi J, Langer N, Jäncke L. Resting-state functional and structural connectivity within an insula–amygdala route specifically index state and trait anxiety. Biol Psychiatry. (2013) 73:85–92. doi: 10.1016/j.biopsych.2012.06.003
155. Fedota JR, Ding X, Matous AL, Salmeron BJ, McKenna MR, Gu H, et al. Nicotine abstinence influences the calculation of salience in discrete insular circuits. Biol Psychiatry Cogn Neurosci Neuroimag. (2018) 3:150–9. doi: 10.1016/j.bpsc.2017.09.010
156. Churchill NW, Hutchison MG, Graham SJ, Schweizer TA. Insular connectivity is associated with self-appraisal of cognitive function after a concussion. Front Neurol. (2021) 12:653442. doi: 10.3389/fneur.2021.653442
157. Flowers M, Leung A, Schiehser DM, Metzger-Smith V, Delano-Wood L, Sorg S, et al. Severities in persistent mild traumatic brain injury related headache is associated with changes in supraspinal pain modulatory functions. Mol Pain. (2021) 17:174480692110378. doi: 10.1177/17448069211037881
158. Liu X, Ma J, Wang N. Lateral geniculate body. In:Wang N, Liu X, Fan N, , editors. Optic Disorders and Visual Field. Vol. 2. Singapore: Springer (2019). p. 27–9.
160. O'Connor DH, Fukui MM, Pinsk MA, Kastner S. Attention modulates responses in the human lateral geniculate nucleus. Nat Neurosci. (2002) 5:1203–9. doi: 10.1038/nn957
161. Schmid MC, Mrowka SW, Turchi J, Saunders RC, Wilke M, Peters AJ, et al. Blindsight depends on the lateral geniculate nucleus. Nature. (2010) 466:373–7. doi: 10.1038/nature09179
162. Cowey A. Erratum to: the blindsight saga. Exp Brain Res. (2010) 202:527–527. doi: 10.1007/s00221-010-2222-6
163. Jang SH, Bae CH, Seo JP. Injury of auditory radiation and sensorineural hearing loss from mild traumatic brain injury. Brain Injury. (2019) 33:249–52. doi: 10.1080/02699052.2018.1539243
164. Tagliamonte M, Sestieri C, Romani GL, Gallucci M, Caulo M, MRI. anatomical variants of mammillary bodies. Brain Struct Funct. (2015) 220:85–90. doi: 10.1007/s00429-013-0639-y
165. Tsivilis D, Vann SD, Denby C, Roberts N, Mayes AR, Montaldi D, et al. A disproportionate role for the fornix and mammillary bodies in recall versus recognition memory. Nat Neurosci. (2008) 11:834–42. doi: 10.1038/nn.2149
166. Vann SD, Nelson AJ. The mammillary bodies and memory: more than a hippocampal relay. Prog Brain Res. (2015) 219:163–85. doi: 10.1016/bs.pbr.2015.03.006
167. Meys K, de Vries L, Groenendaal F, Vann S, Lequin M. The mammillary bodies: a review of causes of injury in infants and children. Am J Neuroradiol. (2022) 43:802–12. doi: 10.3174/ajnr.A7463
168. Zahr NM, Kaufman KL, Harper CG. Clinical and pathological features of alcohol-related brain damage. Nat Rev Neurol. (2011) 7:284–94. doi: 10.1038/nrneurol.2011.42
169. McKee AC, Stein TD, Huber BR, Crary JF, Bieniek K, Dickson D, et al. Chronic traumatic encephalopathy (CTE): criteria for neuropathological diagnosis and relationship to repetitive head impacts. Acta Neuropathol. (2023) 145:371–94. doi: 10.1007/s00401-023-02540-w
170. Winer JA. The human medial geniculate body. Hear Res. (1984) 15:225–47. doi: 10.1016/0378-5955(84)90031-5
171. Winer JA, Miller LM, Lee CC, Schreiner CE. Auditory thalam-ocortical transformation: structure and function. Trends Neurosci. (2005) 28:255–63. doi: 10.1016/j.tins.2005.03.009
172. Sherman SM, Guillery RW. On the actions that one nerve cell can have on another: distinguishing “drivers” from “modulators”. Proc Nat Acad Sci USA. (1998) 95:7121–6. doi: 10.1073/pnas.95.12.7121
173. Vasquez-Lopez SA, Weissenberger Y, Lohse M, Keating P, King AJ, Dahmen JC. Thalamic input to auditory cortex is locally heterogeneous but globally tonotopic. Elife. (2017) 6:e25141. doi: 10.7554/eLife.25141
174. Maffei C, Sarubbo S, Jovicich J. A missing connection: a review of the macrostructural anatomy and tractography of the acoustic radiation. Front Neuroanat. (2019) 13:27. doi: 10.3389/fnana.2019.00027
175. Shivashankar N, Shashikala HR, Nagaraja D, Jayakumar PN, Ratnavalli E. Pure word deafness in two patients with subcortical lesions. Clin Neurol Neurosurg. (2001) 103:201–5. doi: 10.1016/S0303-8467(01)00136-6
176. Maffei C, Capasso R, Cazzolli G, Colosimo C, Dell'Acqua F, Piludu F, et al. Pure word deafness following left temporal damage: behavioral and neuroanatomical evidence from a new case. Cortex. (2017) 97:240–54. doi: 10.1016/j.cortex.2017.10.006
177. Hoffman AN, Lam J, Hovda DA, Giza CC, Fanselow MS. Sensory sensitivity as a link between concussive traumatic brain injury and PTSD. Sci Rep. (2019) 9:13841. doi: 10.1038/s41598-019-50312-y
178. O'Shea J, Johansen-Berg H, Trief D, Göbel S, Rushworth MFS. Functionally specific reorganization in human premotor cortex. Neuron. (2007) 54:479–90. doi: 10.1016/j.neuron.2007.04.021
179. De Borst AW, de Gelder B. Threat detection in nearby space mobilizes human ventral premotor cortex, intraparietal sulcus, and amygdala. Brain Sci. (2022) 12:391. doi: 10.3390/brainsci12030391
180. Rizzolatti G, Luppino G, Matelli M. The organization of the cortical motor system: new concepts. Electroencephalo Clin Neurophysiol. (1998) 106:283–96. doi: 10.1016/S0013-4694(98)00022-4
181. Rothi LJG, Heilman KM (editprs). Apraxia: The Neuropsychology of Action. London: Psychology Press (2014).
182. Duffau H, Gatignol P, Denvil D, Lopes M, Capelle L. The articulatory loop: study of the subcortical connectivity by electrostimulation. Neuroreport. (2003) 14:2005–8. doi: 10.1097/00001756-200310270-00026
183. Keightley ML, Singh Saluja R, Chen JK, Gagnon I, Leonard G, Petrides M, et al. A functional magnetic resonance imaging study of working memory in youth after sports-related concussion: is it still working? J Neurotrauma. (2014) 31:437–51. doi: 10.1089/neu.2013.3052
184. Crasta JE, Nebel MB, Svingos A, Tucker RN, Chen HW, Busch T, et al. Rethinking recovery in adolescent concussions: Network-level functional connectivity alterations associated with motor deficits. Hum Brain Mapp. (2023) 1–12. doi: 10.1002/hbm.26280
185. Chamard E, Lefebvre G, Lassonde M, Theoret H. long-term abnormalities in the corpus callosum of female concussed athletes. J Neurotrauma. (2016) 33:1220–6. doi: 10.1089/neu.2015.3948
186. Meier JD, Aflalo TN, Kastner S, Graziano MSA. Complex organization of human primary motor cortex: a high-resolution fMRI study. J Neurophysiol. (2008) 100:1800–12. doi: 10.1152/jn.90531.2008
187. Muret D, Root V, Kieliba P, Clode D, Makin TR. Beyond body maps: information content of specific body parts is distributed across the somatosensory homunculus. Cell Rep. (2022) 38:110523. doi: 10.1016/j.celrep.2022.110523
188. Penfield W, Boldrey E. Somatic motor and sensory representation in the cerebral cortex of man as studied by electrical stimulation. Brain. (1937) 60:389–443. doi: 10.1093/brain/60.4.389
189. Thompson A, Murphy D, Dell'Acqua F, Ecker C, McAlonan G, Howells H, et al. Impaired communication between the motor and somatosensory homunculus is associated with poor manual dexterity in autism spectrum disorder. Biol Psychiatry. (2017) 81:211–9. doi: 10.1016/j.biopsych.2016.06.020
190. Kana RK, Libero LE, Hu CP, Deshpande HD, Colburn JS. Functional brain networks and white matter underlying theory-of-mind in autism. Soc Cog Affect Neurosci. (2014) 9:98–105. doi: 10.1093/scan/nss106
191. Moayedi M, Salomons TV, Dunlop KAM, Downar J, Davis KD. Connectivity-based parcellation of the human frontal polar cortex. Brain Struct Funct. (2015) 220:2603–16. doi: 10.1007/s00429-014-0809-6
192. Buetefisch CM, Revill KP, Haut MW, Kowalski GM, Wischnewski M, Pifer M, et al. Abnormally reduced primary motor cortex output is related to impaired hand function in chronic stroke. J Neurophysiol. (2018) 120:1680–94. doi: 10.1152/jn.00715.2017
193. Lang CE, Schieber MH. Reduced muscle selectivity during individuated finger movements in humans after damage to the motor cortex or corticospinal tract. J Neurophysiol. (2004) 91:1722–33. doi: 10.1152/jn.00805.2003
194. Kumar S, Sedley W, Barnes GR, Teki S, Friston KJ, Griffiths TD, et al. brain basis for musical hallucinations. Cortex. (2014) 52:86–97. doi: 10.1016/j.cortex.2013.12.002
195. Fukui T, Sugita K, Kawamura M, Shiota J, Nakano I. Primary progressive apraxia in Pick's disease: a clinicopathologic study. Neurology. (1996) 47:467–73. doi: 10.1212/WNL.47.2.467
196. Stoeckel MC, Binkofski F. The role of ipsilateral primary motor cortex in movement control and recovery from brain damage. Exp Neurol. (2010) 221:13–7. doi: 10.1016/j.expneurol.2009.10.021
197. Tamura K, Osada T, Ogawa A, Tanaka M, Suda A, Shimo Y, et al. MRI-based visualization of rTMS-induced cortical plasticity in the primary motor cortex. PLoS ONE. (2019) 14:e0224175. doi: 10.1371/journal.pone.0224175
198. Henry LC, Tremblay S, Boulanger Y, Ellemberg D, Lassonde M. Neurometabolic changes in the acute phase after sports concussions correlate with symptom severity. J Neurotrauma. (2010) 27:65–76. doi: 10.1089/neu.2009.0962
199. De Beaumont L, Lassonde M, Leclerc S, Théoret H. Long-term and cumulative effects of sports concussion on motor cortex inhibition. Neurosurg. (2007) 61:329–37. doi: 10.1227/01.NEU.0000280000.03578.B6
200. Brodmann K. Vergleichende Lokalisationslehre der Grosshirnrinde in ihren Prinzipien dargestellt auf Grund des Zellenbaues de Leipzig: Barth. Leipzig, DE: Barth (1909).
201. Borich MR, Brodie SM, Gray WA, Ionta S, Boyd LA. Understanding the role of the primary somatosensory cortex: opportunities for rehabilitation. Neuropsychologia. (2015) 79:246–55. doi: 10.1016/j.neuropsychologia.2015.07.007
202. Sakata H, Taira M, Murata A, Mine S. Neural mechanisms of visual guidance of hand action in the parietal cortex of the monkey. Cerebral Cortex. (1995) 5:429–38. doi: 10.1093/cercor/5.5.429
203. Pleger B, Foerster A-F, Ragert P, Dinse HR, Schwenkreis P, Malin J-P, et al. Functional imaging of perceptual learning in human primary and secondary somatosensory cortex. Neuron. (2003) 40:643–53. doi: 10.1016/S0896-6273(03)00677-9
204. Bushnell MC, Duncan GH, Hofbauer RK, Ha B, Chen J-I, Carrier B. Pain perception: is there a role for primary somatosensory cortex? Proc Natl Acad Sci USA. (1999) 96:7705–9. doi: 10.1073/pnas.96.14.7705
205. Casey KL, Minoshima S, Berger KL, Koeppe RA, Morrow TJ, Frey KA. Positron emission tomographic analysis of cerebral structures activated specifically by repetitive noxious heat stimuli. J Neurophysiol. (1994) 71:802–7. doi: 10.1152/jn.1994.71.2.802
206. Flor H, Braun C, Elbert T, Birbaumer N. Extensive reorganization of primary somatosensory cortex in chronic back pain patients. Neurosci Lett. (1997) 224:5–8. doi: 10.1016/S0304-3940(97)13441-3
207. Dinse HR, Merzenich MM. Adaptation of Inputs in the somatosensory. In:Fahle M, Poggio T, , editors. Perceptual Learning. Cambridge, MA: MIT Press (2002). p. 19–42.
208. Pleger B, Dinse HR, Ragert P, Schwenkreis P, Malin JP, Tegenthoff M. Shifts in cortical representations predict human discrimination improvement. Proc Nat Acad Sci USA. (2001) 98:12255–60. doi: 10.1073/pnas.191176298
209. Pitcher D, Garrido L, Walsh V, Duchaine BC. Transcranial Magnetic Stimulation disrupts the perception and embodiment of facial expressions. J Neurosci. (2008) 28:8929–33. doi: 10.1523/JNEUROSCI.1450-08.2008
210. Singh A, Patel D, Li A, Hu L, Zhang Q, Liu Y, et al. Mapping cortical integration of sensory and affective pain pathways. Curr Biol. (2020) 30:1703–15.e5. doi: 10.1016/j.cub.2020.02.091
211. Chong CD, Wang L, Wang K, Traub S, Li J. Homotopic region connectivity during concussion recovery: a longitudinal fMRI study. PLoS ONE. (2019) 14:e0221892. doi: 10.1371/journal.pone.0221892
212. Eickhoff SB, Schleicher A, Zilles K, Amunts K. The human parietal operculum. I Cytoarchitectonic mapping of subdivisions. Cereb Cortex. (2006) 16:254–67. doi: 10.1093/cercor/bhi105
213. Eickhoff SB, Grefkes C, Zilles K, Fink GR. The somatotopic organization of cytoarchitectonic areas on the human parietal operculum. Cereb Cortex. (2007) 17:1800–11. doi: 10.1093/cercor/bhl090
214. Dinse HR, Ragert P, Pleger B, Schwenkreis P, Tegenthoff M. Pharmacological modulation of perceptual learning and associated cortical reorganization. Science. (2003) 301:91–4. doi: 10.1126/science.1085423
215. Del Gratta C, Della Penna S, Ferretti A, Franciotti R, Pizzella V, Tartaro A, et al. Topographic organization of the human primary and secondary somatosensory cortices: comparison of fMRI and MEG findings. Neuroimage. (2002) 17:1373–83. doi: 10.1006/nimg.2002.1253
216. Romo R, Hernández A, Zainos A, Lemus L, Brody CD. Neuronal correlates of decision-making in secondary somatosensory cortex. Nat Neurosci. (2002) 5:1217–25. doi: 10.1038/nn950
217. Guo X, Lyu Y, Wang Z, Li Y, Xiang J, Pan C, et al. Correlates of residual limb pain: from residual limb length and usage to metabolites and activity in secondary somatosensory cortex. IEEE Transact Neural Sys Rehabil Eng. (2019) 27:96–104. doi: 10.1109/TNSRE.2018.2885146
218. Wang J, Yang Y, Fan L, Xu J, Li C, Liu Y, et al. Convergent functional architecture of the superior parietal lobule unraveled with multimodal neuroimaging approaches. Hum Brain Mapp. (2015) 36:238–57. doi: 10.1002/hbm.22626
219. Lester BD, Dassonville P. The role of the right superior parietal lobule in processing visual context for the establishment of the egocentric reference frame. J Cog Neurosci. (2014) 26:2201–9. doi: 10.1162/jocn_a_00636
220. Rosenbaum RS, Ziegler M, Winocur G, Grady CL, Moscovitch M. “I have often walked down this street before”: fMRI studies on the hippocampus and other structures during mental navigation of an old environment. Hippocampus. (2004) 14:826–35. doi: 10.1002/hipo.10218
221. Spunt RP, Lieberman MD. An integrative model of the neural systems supporting the comprehension of observed emotional behavior. Neuroimage. (2012) 59:3050–9. doi: 10.1016/j.neuroimage.2011.10.005
222. Molholm S, Sehatpour P, Mehta AD, Shpaner M, Gomez-Ramirez M, Ortigue S, et al. Audio-visual multisensory integration in superior parietal lobule revealed by human intracranial recordings. J Neurophysiol. (2006) 96:721–9. doi: 10.1152/jn.00285.2006
223. Mørch-Johnsen L, Nerland S, Jørgensen KN, Osnes K, Hartberg CB, Andreassen OA, et al. Cortical thickness abnormalities in bipolar disorder patients with a lifetime history of auditory hallucinations. Bipolar Disord. (2018) 20:647–57. doi: 10.1111/bdi.12627
224. Saluja RS, Chen JK, Gagnon IJ, Keightley M, Ptito A. Navigational memory functional magnetic resonance imaging: a test for concussion in children. J Neurotrauma. (2015) 32:712–22. doi: 10.1089/neu.2014.3470
225. Murdaugh DL, King TZ, Sun B, Jones RA, Ono KE, Reisner A, et al. Longitudinal changes in resting state connectivity and white matter integrity in adolescents with sports-related concussion – erratum. J Int Neuropsychol Soc. (2018) 24:890–890. doi: 10.1017/S1355617718000875
226. Purves D, Cabeza R, Huettel SA, LaBar KS, Platt ML, Woldorff MG. Principles of Cognitive Neuroscience. 2nd ed. Sunderland, MA: Sinauer Associates (2013).
227. Ozdemir A, Black PM. Mapping of human visual cortex. Neurosurg Quar. (2005) 15:65–71. doi: 10.1097/01.wnq.0000155121.49959.2c
228. Tootell RBH, Mendola JD, Hadjikhani NK, Ledden PJ, Liu AK, Reppas JB, et al. Functional analysis of V3A and related areas in human visual cortex. J Neurosci. (1997) 17:7060–78. doi: 10.1523/JNEUROSCI.17-18-07060.1997
229. Johnson B, Hallett M, Slobounov S. Follow-up evaluation of oculomotor performance with fMRI in the subacute phase of concussion. Neurol. (2015) 85:1163–6. doi: 10.1212/WNL.0000000000001968
230. Trofimova A, Smith JL, Ahluwalia V, Hurtado J, Gore RK, Allen J.W Alterations in resting-state functional brain connectivity and correlations with vestibular/ocular-motor screening measures in postconcussion vestibular dysfunction. J Neuroimag. (2021) 31:277–86. doi: 10.1111/jon.12834
231. Maffei C, Sarubbo S, Jovicich J. Diffusion-based tractography atlas of the human acoustic radiation. Sci Rep. (2019) 9:4046. doi: 10.1038/s41598-019-40666-8
232. Berman J, Lanza M, Blaskey L, Edgar J, Roberts T. High angular resolution diffusion imaging probabilistic tractography of the auditory radiation. Am J Neuroradiol. (2013) 34:1573–8. doi: 10.3174/ajnr.A3471
233. Rademacher J, Bürgel U, Zilles K. Stereotaxic localization, intersubject variability, and interhemispheric differences of the human auditory thalamocortical system. Neuroimage. (2002) 17:142–60. doi: 10.1006/nimg.2002.1178
234. Koops EA, Haykal S, van Dijk P. Macrostructural changes of the acoustic radiation in humans with hearing loss and Tinnitus revealed with fixel-based analysis. J Neurosci. (2021) 41:3958–65. doi: 10.1523/JNEUROSCI.2996-20.2021
235. Cubon VA, Putukian M, Boyer C, Dettwiler A. A diffusion tensor imaging study on the white matter skeleton in individuals with sports-related concussion. J Neurotrauma. (2011) 28:189–201. doi: 10.1089/neu.2010.1430
236. Yao ZF, Sligte IG, Moreau D, Hsieh S, Yang CT, Ridderinkhof KR, et al. The brains of elite soccer players are subject to experience-dependent alterations in white matter connectivity. Cortex. (2020) 132:79–91. doi: 10.1016/j.cortex.2020.07.016
237. Aboitiz FD, Scheibel ABS, Fisher RS, Zaidel E. Fiber composition of the human corpus callosum. Brain Res. (1992) 598:143–53. doi: 10.1016/0006-8993(92)90178-C
238. Luders E, Thompson PM, Toga AW. The development of the corpus callosum in the healthy human brain. J Neurosci. (2010) 30:10985–90. doi: 10.1523/JNEUROSCI.5122-09.2010
239. Pietrasik W, Cribben I, Olsen F, Huang Y, Malykhin NV. Diffusion tensor imaging of the corpus callosum in healthy aging: investigating higher order polynomial regression modelling. Neuroimage. (2020) 213:116675. doi: 10.1016/j.neuroimage.2020.116675
241. van der Knaap LJ, van der Ham IJM. How does the corpus callosum mediate interhemispheric transfer? A review. Behav Brain Res. (2011) 223:211–21. doi: 10.1016/j.bbr.2011.04.018
242. McAllister TW, Ford JC Ji S, Beckwith JG, Flashman LA, Paulsen K, et al. Maximum principal strain and strain rate associated with concussion diagnosis correlates with changes in corpus callosum white matter indices. Ann Biomed Eng. (2012) 40:127–40. doi: 10.1007/s10439-011-0402-6
243. Solomito MJ, Reuman H, Wang DH. Sex differences in concussion: a review of brain anatomy, function, and biomechanical response to impact. Brain Injury. (2019) 33:105–10. doi: 10.1080/02699052.2018.1542507
244. Shah A, Jhawar SS, Goel A. Analysis of the anatomy of the Papez circuit and adjoining limbic system by fiber dissection techniques. J Clin Neurosci. (2012) 19:289–98. doi: 10.1016/j.jocn.2011.04.039
245. Chiang H-L, Chen Y-J, Shang C-Y, Tseng W-YI, Gau SS-F. Different neural substrates for executive functions in youths with ADHD: a diffusion spectrum imaging tractography study. Psychol Med. (2016) 46:1225–38. doi: 10.1017/S0033291715002767
246. Koenig KA, Sakaie KE, Lowe MJ, Lin J, Stone L, Bermel RA, et al. The relationship between cognitive function and high-resolution diffusion tensor MRI of the cingulum bundle in multiple sclerosis. Mult Scler. (2015) 21:1794–801. doi: 10.1177/1352458515576983
247. Metzler-Baddeley C, Jones DK, Belaroussi B, Aggleton JP, O'Sullivan MJ. Frontotemporal connections in episodic memory and aging: a diffusion MRI tractography study. J Neurosci. (2011) 31:13236–45. doi: 10.1523/JNEUROSCI.2317-11.2011
248. Ray NJ, Metzler-Baddeley C, Khondoker MR, Grothe MJ, Teipel S, Wright P, et al. Cholinergic basal forebrain structure influences the reconfiguration of white matter connections to support residual memory in mild cognitive impairment. J Neurosci. (2015) 35:739–47. doi: 10.1523/JNEUROSCI.3617-14.2015
249. Pan Y, Dempster K, Jeon P, Théberge J, Khan AR, Palaniyappan L. Acute conceptual disorganization in untreated first-episode psychosis: a combined magnetic resonance spectroscopy and diffusion imaging study of the cingulum. J Psychiatry Neurosci. (2021) 46:E337–46. doi: 10.1503/jpn.200167
250. Ballantine HT, Bouckoms AJ, Thomas EK, Giriunas IE. Treatment of psychiatric illness by stereotactic cingulotomy. Biol Psychiatry. (1987) 22, 807–19. doi: 10.1016/0006-3223(87)90080-1
251. Sindermann L, Redlich R, Opel N, Böhnlein J, Dannlowski U, Leehr EJ. Systematic transdiagnostic review of magnetic-resonance imaging results: depression, anxiety disorders and their co-occurrence. J Psychiatric Res. (2021) 142:226–39. doi: 10.1016/j.jpsychires.2021.07.022
252. Bhatia KD, Henderson LA, Hsu E, Yim M. Reduced integrity of the uncinate fasciculus and cingulum in depression: a stem-by-stem analysis. J Affect Disord. (2018) 235:220–8. doi: 10.1016/j.jad.2018.04.055
253. Chahal R, Ho TC, Miller JG, Borchers LR, Gotlib IH. Sex- specific vulnerability to depressive symptoms across adolescence and during the COVID-19 pandemic: the role of the cingulum bundle. JCPP Adv. (2022) 2:e12061. doi: 10.1002/jcv2.12061
254. Kontos AP, Covassin T, Elbin RJ, Parker T. Depression and neurocognitive performance after concussion among male and female high school and collegiate athletes. Arch Phys Med Rehabil. (2012) 93:1751–6. doi: 10.1016/j.apmr.2012.03.032
255. Rapoport MJ, Mccullagh S, Streiner D, Feinstein A. The clinical significance of major depression following mild traumatic brain injury. Psychosomat. (2003) 44:31–7. doi: 10.1176/appi.psy.44.1.31
256. Lima Santos JP, Kontos AP, Holland CL, Suss SJ, Stiffler RS, Bitzer HB, et al. The role of puberty and sex on brain structure in adolescents with anxiety following concussion. Biol Psych Cog Neurosci Neuroimag. (2022). doi: 10.1016/j.bpsc.2022.09.013 [Epubh ahead of print].
257. Taghdiri F, Chung J, Irwin S, Multani N, Tarazi A, Ebraheem A, et al. Decreased number of self-paced saccades in post-concussion syndrome associated with higher symptom burden and reduced white matter integrity. J Neurotrauma. (2018) 35:719–29. doi: 10.1089/neu.2017.5274
258. Lima Santos JP, Kontos AP, Holland CL, Stiffler RS, Bitzer HB, Caviston K, et al. The role of sleep quality on white matter integrity and concussion symptom severity in adolescents. NeuroImage Clin. (2022) 35:103130. doi: 10.1016/j.nicl.2022.103130
259. Wu TC, Wilde EA, Bigler ED, Yallampalli R, McCauley SR, Troyanskaya M, et al. Evaluating the relationship between memory functioning and cingulum bundles in acute mild traumatic brain injury using diffusion tensor imaging. J Neurotrauma. (2010) 27:303–7. doi: 10.1089/neu.2009.1110
260. Schieber MH. Chapter 2 Comparative anatomy and physiology of the corticospinal system. Handb Clin Neurol. (2007) 82:15–37. doi: 10.1016/S0072-9752(07)80005-4
261. Welniarz Q, Dusart I, Roze E. The corticospinal tract: evolution, development, and human disorders. Dev Neurobiol. (2017) 77:810–29. doi: 10.1002/dneu.22455
262. Carper RA, Solders S, Treiber JM, Fishman I, Müller R-A. Corticospinal tract anatomy and functional connectivity of primary motor cortex in autism. J Am Acad Child Adol Psychiatry. (2015) 54:859–67. doi: 10.1016/j.jaac.2015.07.007
263. Jang SH. The corticospinal tract from the viewpoint of brain rehabilitation. J Rehabil Med. (2014) 46:193–9. doi: 10.2340/16501977-1782
265. Van Wittenberghe IC, Peterson DC. Corticospinal Tract Lesion. Treasure Island, FL: StatPearls Publishing (2019). 9 p.
266. Multani N, Goswami R, Khodadadi M, Ebraheem A, Davis KD, Tator CH, et al. The association between white-matter tract abnormalities, and neuropsychiatric and cognitive symptoms in retired professional football players with multiple concussions. J Neurol. (2016) 263:1332–41. doi: 10.1007/s00415-016-8141-0
267. Senova S, Fomenko A, Gondard E, Lozano AM. Anatomy and function of the fornix in the context of its potential as a therapeutic target. J Neurol Neurosurg Psychiatry. (2020) 91:547–59. doi: 10.1136/jnnp-2019-322375
268. Jang SH, Kim SH, Do Lee H. Relation between memory impairment and the fornix injury in patients with mild Traumatic Brain Injury: a diffusion tensor tractography study. Am J Phys Med Rehabil. (2018) 97:892–6. doi: 10.1097/PHM.0000000000000996
269. Kinnunen KM, Greenwood R, Powell JH, Leech R, Hawkins PC, Bonnelle V, et al. White matter damage and cognitive impairment after traumatic brain injury. Brain. (2011) 134:449–63. doi: 10.1093/brain/awq347
270. Tomaiuolo F. Gross morphology and morphometric sequelae in the hippocampus, fornix, and corpus callosum of patients with severe non-missile traumatic brain injury without macroscopically detectable lesions: a T1 weighted MRI study. J Neurol Neurosurg Psychiatry. (2004) 75:1314–22. doi: 10.1136/jnnp.2003.017046
271. Yeh PH, Wang B, Oakes TR, French LM, Pan H, Graner J, et al. Postconcussional disorder and PTSD symptoms of military-related traumatic brain injury associated with compromised neurocircuitry. Hum Brain Map. (2014) 35:2652–73. doi: 10.1002/hbm.22358
272. Wong JKY, Churchill NW, Graham SJ, Baker AJ, Schweizer TA. Altered connectivity of default mode and executive control networks among female patients with persistent post-concussion symptoms. Brain Injury. (2023) 37:147–58. doi: 10.1080/02699052.2022.2163290
273. de Souza NL, Buckman JF, Dennis EL, Parrott JS, Velez C, Wilde EA, et al. Association between white matter organization and cognitive performance in athletes with a history of sport-related concussion. J Clin Exp Neuropsychol. (2021) 43:704–15. doi: 10.1080/13803395.2021.1991893
274. de Benedictis A, Efisio Marras C, Petit L, Sarubbo S. The inferior fronto-occipital fascicle: a century of controversies from anatomy theaters to operative neurosurgery. J Neurosurg Sci. (2021) 6:605–15. doi: 10.23736/S0390-5616.21.05360-1
275. Makris N, Papadimitriou GM, Sorg S, Kennedy DN, Caviness VS, Pandya DN. The occipitofrontal fascicle in humans: a quantitative, in vivo, DT-MRI study. Neuroimage. (2007) 37:1100–11. doi: 10.1016/j.neuroimage.2007.05.042
276. Palejwala AH, O'Connor KP, Pelargos P, Briggs RG, Milton CK, Conner AK, et al. Anatomy and white matter connections of the lateral occipital cortex. Surg Radiol Anat. (2020) 42:315–28. doi: 10.1007/s00276-019-02371-z
277. Garibotto V, Scifo P, Gorini A, Alonso CR, Brambati S, Bellodi L, et al. Disorganization of anatomical connectivity in obsessive compulsive disorder: a multi-parameter diffusion tensor imaging study in a subpopulation of patients. Neurobiol Dis. (2010) 37:468–76. doi: 10.1016/j.nbd.2009.11.003
278. Lawrence NS, Jollant F, O'Daly O, Zelaya F, Phillips ML. Distinct roles of prefrontal cortical subregions in the Iowa gambling task. Cerebral Cortex. (2009) 19:1134–43. doi: 10.1093/cercor/bhn154
279. Duffau H, Moritz-Gasser S, Mandonnet E. A re-examination of neural basis of language processing: proposal of a dynamic hodotopical model from data provided by brain stimulation mapping during picture naming. Brain Lang. (2014) 131:1–10. doi: 10.1016/j.bandl.2013.05.011
280. Oestreich LKL, McCarthy-Jones S, Whitford TJ. Australian Schizophrenia Research Bank. Decreased integrity of the fronto-temporal fibers of the left inferior occipito-frontal fasciculus associated with auditory verbal hallucinations in schizophrenia. Brain Imag Behav. (2016) 10:445–54. doi: 10.1007/s11682-015-9421-5
281. Dayan M, Munoz M, Jentschke S, Chadwick MJ, Cooper JM, Riney K, et al. Optic radiation structure and anatomy in the normally developing brain determined using diffusion MRI and tractography. Brain Struct Funct. (2015) 220:291–306. doi: 10.1007/s00429-013-0655-y
282. Jang SH, Chang CH, Jung YJ, Kim SH, Seo JP. Optic radiation injury in patients with aneurismal subarachnoid hemorrhage: a preliminary diffusion tensor imaging report. Neural Regen Res. (2018) 13:563–6. doi: 10.4103/1673-5374.228763
283. Brahm KD, Wilgenburg HM, Kirby J, Ingalla S, Chang C-Y, Goodrich GL. Visual impairment and dysfunction in combat-injured service members with traumatic brain injury. Optom Vision Sci. (2009) 86:817–25. doi: 10.1097/OPX.0b013e3181adff2d
284. Suchoff IB, Kapoor N, Ciuffreda KJ, Rutner D, Han E, Craig S. The frequency of occurrence, types, and characteristics of visual field defects in acquired brain injury: a retrospective analysis. Opt J Am Opt A. (2008) 79:259–65. doi: 10.1016/j.optm.2007.10.012
285. Lennartsson F, Nilsson M, Flodmark O, Jacobson L, Larsson J. Injuries to the immature optic radiation show correlated thinning of the macular ganglion cell layer. Front Neurol. (2018) 9:321. doi: 10.3389/fneur.2018.00321
286. Jang SH, Seo JP. Damage to the optic radiation in patients with mild traumatic brain injury. J NeuroOphthal. (2015) 35:270. doi: 10.1097/WNO.0000000000000249
287. Astafiev SV, Zinn KL, Shulman GL, Corbetta M. Exploring the physiological correlates of chronic mild traumatic brain injury symptoms. NeuroImage Clin. (2016) 11:10–9. doi: 10.1016/j.nicl.2016.01.004
288. Caeyenberghs K, Leemans A, Geurts M, Taymans T, Linden CV, Smits-Engelsman BCM, et al. Brain-behavior relationships in young traumatic brain injury patients: Fractional anisotropy measures are highly correlated with dynamic visuomotor tracking performance. Neuropsychologia. (2010) 48:1472–82. doi: 10.1016/j.neuropsychologia.2010.01.017
289. Nakajima R, Kinoshita M, Shinohara H, Nakada M. The superior longitudinal fasciculus: reconsidering the fronto-parietal neural network based on anatomy and function. Brain Imag Behav. (2020) 14:2817–30. doi: 10.1007/s11682-019-00187-4
290. Panesar SS, Belo JTA, Yeh F-C, Fernandez-Miranda JC. Structure, asymmetry, and connectivity of the human temporo-parietal aslant and vertical occipital fasciculi. Brain Struct Funct. (2019) 224:907–23. doi: 10.1007/s00429-018-1812-0
291. de Schotten MT, Dell'Acqua F, Valabregue R, Catani M. Monkey to human comparative anatomy of the frontal lobe association tracts. Cortex. (2012) 48:82–96. doi: 10.1016/j.cortex.2011.10.001
292. Wang X, Pathak S, Stefaneanu L, Yeh F-C, Li S, Fernandez-Miranda JC. Subcomponents and connectivity of the superior longitudinal fasciculus in the human brain. Brain Struct Funct. (2016) 221:2075–92. doi: 10.1007/s00429-015-1028-5
293. Zhang J, Tian L, Zhang L, Cheng R, Wei R, He F, et al. Relationship between white matter integrity and post-traumatic cognitive deficits: a systematic review and meta-analysis. J Neurol Neurosurg Psychiatry. (2019) 90:98–107. doi: 10.1136/jnnp-2017-317691
294. Bürgel U, Amunts K, Hoemke L, Mohlberg H, Gilsbach JM, Zilles K. White matter fiber tracts of the human brain: three-dimensional mapping at microscopic resolution, topography and intersubject variability. Neuroimage. (2006) 29:1092–105. doi: 10.1016/j.neuroimage.2005.08.040
295. Liu X, Kinoshita M, Shinohara H, Hori O, Ozaki N, Nakada M. Does the superior fronto-occipital fascicle exist in the human brain? Fiber dissection and brain functional mapping in 90 patients with gliomas. Neuroimage Clin. (2020) 25:102192. doi: 10.1016/j.nicl.2020.102192
296. Govindarajan ST, Liu Y, Parra Corral MA, Bangiyev L, Krupp L, Charvet L, et al. White matter correlates of slowed information processing speed in unimpaired multiple sclerosis patients with young age onset. Brain Imag Behav. (2021) 15:1460–8. doi: 10.1007/s11682-020-00345-z
297. Bhatia K, Henderson L, Yim M, Hsu E, Dhaliwal R. Diffusion Tensor Imaging investigation of uncinate fasciculus anatomy in healthy controls: description of a subgenual stem. Neuropsychobiol. (2017) 75:132–40. doi: 10.1159/000485111
298. Hau J, Sarubbo S, Houde JC, Corsini F, Girard G, Deledalle C, et al. Revisiting the human uncinate fasciculus, its subcomponents and asymmetries with stem-based tractography and microdissection validation. Brain Struct Funct. (2017) 222:1645–62. doi: 10.1007/s00429-016-1298-6
299. Kier EL, Staib LH, Davis LM, Bronen RA. MR imaging of the temporal stem: anatomic dissection tractography of the uncinate fasciculus, inferior occipitofrontal fasciculus, and Meyer's Loop of the optic radiation. Am J Neuroradiol. (2004) 25:677–91.
300. Tottenham N, Hare T, Casey B. Behavioral assessment of emotion discrimination, emotion regulation, and cognitive control in childhood, adolescence, and adulthood. Front Psychol. (2011) 2:39. doi: 10.3389/fpsyg.2011.00039
301. Binney RJ, Parker GJ, Ralph MAL. Convergent connectivity and graded specialization in the rostral human temporal lobe as revealed by Diffusion-Weighted Imaging probabilistic tractography. J Cog Neurosci. (2012) 24:1998–2014. doi: 10.1162/jocn_a_00263
302. Thomas C, Avram A, Pierpaoli C, Baker C. Diffusion MRI properties of the human uncinate fasciculus correlate with the ability to learn visual associations. Cortex. (2015) 72:65–78. doi: 10.1016/j.cortex.2015.01.023
303. Papagno C, Miracapillo C, Casarotti A, Romero Lauro LJ, Castellano A, Falini A, et al. What is the role of the uncinate fasciculus? Surgical removal and proper name retrieval. Brain. (2011) 134:405–14. doi: 10.1093/brain/awq283
304. McCauley SR, Wilde EA, Bigler ED, Chu Z, Yallampalli R, Oni MB, et al. Diffusion tensor imaging of incentive effects in prospective memory after pediatric traumatic brain injury. J Neurotrauma. (2011) 28:503–16. doi: 10.1089/neu.2010.1555
305. Geary EK, Kraus MF, Pliskin NH, Little DM. Verbal learning differences in chronic mild traumatic brain injury. J Int Neuropsychol Soc. (2010) 16:506–16. doi: 10.1017/S135561771000010X
306. Niogi SN, Mukherjee P, Ghajar J, Johnson CE, Kolster R, Lee H, et al. Structural dissociation of attentional control and memory in adults with and without mild traumatic brain injury. Brain. (2008) 131:3209–21. doi: 10.1093/brain/awn247
307. Niogi SN, Mukherjee P, Ghajar J, Johnson C, Kolster RA, Sarkar R, et al. Extent of microstructural white matter injury in postconcussive syndrome correlates with impaired cognitive reaction time: a 3T diffusion tensor imaging study of mild traumatic brain injury Am. J Neuroradiol. (2008) 29:967–73. doi: 10.3174/ajnr.A0970
308. Andersen BB, Korbo L, Pakkenberg B. A quantitative study of the human cerebellum with unbiased stereological techniques. J Comp Neurol. (1992) 326:549–60. doi: 10.1002/cne.903260405
309. Ashida R, Cerminara NL, Brooks J, Apps R. Principles of organization of the human cerebellum: macro- and microanatomy. Handb Clin Neurol. (2018) 154, 45–58. doi: 10.1016/B978-0-444-63956-1.00003-5
310. Guell X, Schmahmann J. Cerebellar functional anatomy: a didactic summary based on human fMRI evidence. Cerebellum. (2020) 19:1–5. doi: 10.1007/s12311-019-01083-9
311. Marek S, Siegel JS, Gordon EM, Raut RV, Gratton C, Newbold DJ, et al. Spatial and temporal organization of the individual human cerebellum. Neuron. (2018) 100:977–93.e7. doi: 10.1016/j.neuron.2018.10.010
312. Guell X, Gabrieli JDE, Schmahmann JD. Triple representation of language, working memory, social and emotion processing in the cerebellum: convergent evidence from task and seed-based resting-state fMRI analyses in a single large cohort. Neuroimage. (2018) 172:437–49. doi: 10.1016/j.neuroimage.2018.01.082
313. Manto M, Mariën P. Schmahmann's syndrome - identification of the third cornerstone of clinical ataxiology. Cerebellum Ataxias. (2015) 2:2. doi: 10.1186/s40673-015-0023-1
314. Marien P, Engelborghs S, Fabbro F, de Deyn PP. The lateralized linguistic cerebellum: a review and a new hypothesis. Brain Lang. (2001) 79:580–600. doi: 10.1006/brln.2001.2569
315. Churchill N, Hutchison MG, Leung G, Graham S, Schweizer TA. Changes in functional connectivity of the brain associated with a history of sport concussion: a preliminary investigation. Brain Inj. (2017) 31:39–48. doi: 10.1080/02699052.2016.1221135
316. Pinky NN, Debert CT, Dukelow SP, Benson BW, Harris AD, Yeates KO, et al. Multimodal magnetic resonance imaging of youth sport-related concussion reveals acute changes in the cerebellum, basal ganglia, and corpus callosum that resolve with recovery. Front Hum Neurosci. (2022) 16:976013. doi: 10.3389/fnhum.2022.976013
317. Jantzen KJ, Anderson B, Steinberg FL, Kelso JAS. A prospective functional mr imaging study of mild traumatic brain injury in college football players. Am J Neuroradiol. (2004) 25:738–45.
318. DeMatteo C, McCauley D, Stazyk K, Harper J, Adamich J, Randall S, et al. Post-concussion return to play and return to school guidelines for children and youth: a scoping methodology. Disabil Rehabil. (2015) 37:1107–12. doi: 10.3109/09638288.2014.952452
319. Stoller J, Snow CL, Law M, Frémont P. Do family physicians, emergency department physicians, and pediatricians give consistent sport-related concussion management advice? Can Fam Physician. (2014) 60:548–52.
320. Broglio SP, Katz BP, Zhao S, McCrea M, McAllister T, Hoy AR, et al. Test-retest reliability and interpretation of common concussion assessment tools: findings from the NCAA-DoD CARE Consortium. Sports Med. (2018) 48:1255–68. doi: 10.1007/s40279-017-0813-0
321. Dziemianowicz MS, Kirschen MP, Pukenas BA, Laudano E, Balcer LJ, Galetta SL. Sports-related concussion testing. Curr Neurol Neurosci Rep. (2012) 12:547–59. doi: 10.1007/s11910-012-0299-y
322. Lovell MR. The ImPACT neuropsychological test battery. In:Echemendia RJ, , editor. Sports Neuropsychology: Assessment and Management of Traumatic Brain Injury. New York, NY: Guilford Press (2006). p. 193–215.
323. Echemendia RJ, Meeuwisse W, McCrory P, Davis GA, Putukian M, Leddy J, et al. The Sport Concussion Assessment Tool 5th Edition (SCAT5): background and rationale. Br J Sport Med. (2017) 51:848–51. doi: 10.1136/bjsports-2017-097506
324. Collie A, Maruff P, Darby DG, McStephen M. The effects of practice on the cognitive test performance of neurologically normal individuals assessed at brief test–retest intervals. J Int Neuropsychol Soc. (2003) 9:419–28. doi: 10.1017/S1355617703930074
325. Potter S, Leigh E, Wade D, Fleminger S. The Rivermead post concussion symptoms questionnaire. J Neurol. (2006) 253:1603–14. doi: 10.1007/s00415-006-0275-z
326. Kaufman MW, Su CA, Trivedi NN, Lee MK, Nelson GB, Cupp SA, et al. The current status of concussion assessment scales: a critical analysis review. JBJS Rev. (2021) 9:e20.00108. doi: 10.2106/JBJS.RVW.20.00108
327. Chamard E, Lichtenstein JD. A systematic review of neuroimaging findings in children and adolescents with sports-related concussion. Brain Injury. (2018) 32:816–31. doi: 10.1080/02699052.2018.1463106
Keywords: post-concussion symptoms, brain anatomy, concussion, gray matter, white matter, cerebellum, superior longitudinal fasciculus, insula
Citation: Danielli E, Simard N, DeMatteo CA, Kumbhare D, Ulmer S and Noseworthy MD (2023) A review of brain regions and associated post-concussion symptoms. Front. Neurol. 14:1136367. doi: 10.3389/fneur.2023.1136367
Received: 03 January 2023; Accepted: 12 July 2023;
Published: 03 August 2023.
Edited by:
Steven H. Rauchman, University Neurosciences Institute, United StatesReviewed by:
Filomeno Cortese, University of Calgary, CanadaCopyright © 2023 Danielli, Simard, DeMatteo, Kumbhare, Ulmer and Noseworthy. This is an open-access article distributed under the terms of the Creative Commons Attribution License (CC BY). The use, distribution or reproduction in other forums is permitted, provided the original author(s) and the copyright owner(s) are credited and that the original publication in this journal is cited, in accordance with accepted academic practice. No use, distribution or reproduction is permitted which does not comply with these terms.
*Correspondence: Michael D. Noseworthy, bm9zZXdvckBtY21hc3Rlci5jYQ==
Disclaimer: All claims expressed in this article are solely those of the authors and do not necessarily represent those of their affiliated organizations, or those of the publisher, the editors and the reviewers. Any product that may be evaluated in this article or claim that may be made by its manufacturer is not guaranteed or endorsed by the publisher.
Research integrity at Frontiers
Learn more about the work of our research integrity team to safeguard the quality of each article we publish.