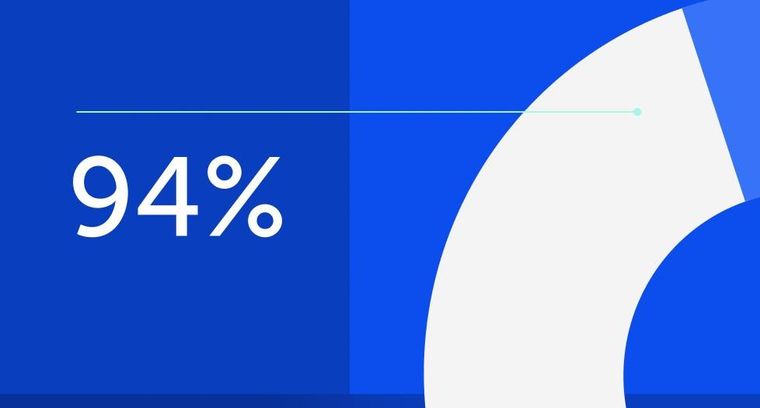
94% of researchers rate our articles as excellent or good
Learn more about the work of our research integrity team to safeguard the quality of each article we publish.
Find out more
REVIEW article
Front. Neurol., 20 April 2023
Sec. Dementia and Neurodegenerative Diseases
Volume 14 - 2023 | https://doi.org/10.3389/fneur.2023.1133546
There are trillions of different microorganisms in the human digestive system. These gut microbes are involved in the digestion of food and its conversion into the nutrients required by the body. In addition, the gut microbiota communicates with other parts of the body to maintain overall health. The connection between the gut microbiota and the brain is known as the gut–brain axis (GBA), and involves connections via the central nervous system (CNS), the enteric nervous system (ENS), and endocrine and immune pathways. The gut microbiota regulates the central nervous system bottom-up through the GBA, which has prompted researchers to pay considerable attention to the potential pathways by which the gut microbiota might play a role in the prevention and treatment of amyotrophic lateral sclerosis (ALS). Studies with animal models of ALS have shown that dysregulation of the gut ecology leads to dysregulation of brain–gut signaling. This, in turn, induces changes in the intestinal barrier, endotoxemia, and systemic inflammation, which contribute to the development of ALS. Through the use of antibiotics, probiotic supplementation, phage therapy, and other methods of inducing changes in the intestinal microbiota that can inhibit inflammation and delay neuronal degeneration, the clinical symptoms of ALS can be alleviated, and the progression of the disease can be delayed. Therefore, the gut microbiota may be a key target for effective management and treatment of ALS.
Amyotrophic lateral sclerosis (ALS) is a neurological disorder of unknown etiology involving the motor neurons, usually accompanied by degeneration of the associated neurons. It is the most common neurodegenerative disease of middle age, with a lifetime risk of occurrence of one in 300 (1) and a peak age of onset of ~60 years (2). ALS leads to progressive paralysis with reduced speech, swallowing, and motor function, eventually resulting in respiratory insufficiency and death. The median survival time of ALS patients is ~2–4 years, but this varies widely, with some individuals surviving for more than 50 years. It has been estimated that ALS causes ~30,000 deaths worldwide each year (3). Factors influencing survival heterogeneity may be environmental or genetic, and some may be random. The etiology of ALS is still unclear, but the more widely accepted factors include genetics, oxidative stress, excitotoxicity, neurotrophic factor disorders, autoimmune mechanisms, viral infections, and environmental factors (4). Approximately 10% of ALS cases are family aggregates (fALS), which can be transmitted within families, and the rest are considered sporadic episodes (sALS) (5) with no clear family history. The incidence of ALS varies slightly by gender: the risk of developing the disease is slightly higher among men (3.0 cases per 100,000 person-years) compared to women (2.4 cases per 100,000 person-years). However, for fALS, the risk is similar for both men and women. The majority of patients (~70%) present with limb-initiating ALS, ~25% present with bulbar onset, and the remainder (5%) present with respiratory involvement or initial trunk onset (6). There is no specific treatment available for ALS, although the more popular novel treatments include gene therapy (7) and stem cell therapy (8). However, two recognized therapeutic agents have been classified as disease-modifying drugs, with riluzole being the most widely used (6). Despite its ability to slow the progression of ALS, its effectiveness is limited. Another drug, edaravone, has also been approved by the FDA for oral use (9) and possesses potent antioxidant properties that mitigate the effects of oxidative stress by eliminating oxidized lipids and hydroxyl radicals, which may be a key factor in the pathogenesis and development of ALS. However, clinical studies have also suggested that edaravone may be ineffective in treating ALS (10). Other drugs include phenylbutyrate-taurursodiol (11) (sodium phenylbutyrate and urocholine). Despite these therapeutic options, there is currently a lack of effective treatments for ALS; therefore, it is crucial to seek a novel approach to treatment.
The gastrointestinal tract contains hundreds of millions of microorganisms, collectively referred to as the gut microbiota, which are formed according to host genetics and environmental exposure. The gut microbiota includes bacteria, fungi, pathogens, commensals, viruses, protozoa, and parasites (12), with both disease-causing and disease-preventing organisms among them. In a healthy human gut, these microorganisms play a crucial role in maintaining overall health and in the development of diseases. Moreover, research has identified links between diseases whose etiology is not yet clear and the gut bacterial map (13, 14). The gut microbiota plays a role in human metabolism (including food and drug metabolism); gut homeostasis; maintenance of the integrity of the gut barrier; immune development, immunomodulation, and prevention of pathogens; and brain processes and behavior (15, 16). When the gut microbiota undergoes pathological changes, its metabolites also change, leading to corresponding changes in normal human physiological functions through immune and circulatory mechanisms, thereby affecting the development of disease (17). Conversely, disease in turn also affects the gut, leading to changes in the gut microbiota. The intestinal microbiota has been found to be a unique ecosystem and has been referred to as the “second genome” of humans (13). With the widespread use of next-generation sequencing (NGS) (18), especially 16SrRNA and shotgun sequencing, the relationship between the gut microbiota and various systemic diseases has been gradually revealed. Lack of a “healthy” microbiota is associated with many forms of disease (19–24), such as metabolic diseases (obesity, type 2 diabetes), gastrointestinal cancer, inflammatory bowel disease, and renal disease, as well as neurodegenerative diseases (NDs) associated with aging, in which the activity and composition of the gut microbiota change during the life cycle. Fang et al. (25) found that altering the gut microbiota in animal models alters immune function, metabolic activity, and ultimately lifespan by mechanisms associated with the aging process, and that alterations to the gut microbiota may positively or negatively affect the neuropathology and clinical manifestations of disease. A growing number of studies have reported changes in the microbiota among patients with Parkinson's disease (PD), Alzheimer's disease (AD), ALS, and Huntington's disease (HD) (26–28), and although the exact mechanisms underlying these phenomena remain unclear, there is a growing body of evidence to support the concept of a “gut microbiota–brain axis” (29). Overall, these findings have generated interest among researchers in further investigation of the microbiota-gut-neural system.
ALS is a fatal neurodegenerative disease of the nervous system. Numerous studies have revealed an inextricable relationship between translocation or altered abundance of gut microbes and risk factors for the onset and progression of ALS, as well as its treatment and prognosis (30–32). Studies have found that, in ALS patients, gastrointestinal symptoms precede neurological symptoms in all cases (33); these include gastroesophageal reflux, chronic constipation, intermittent diarrhea, bloating, and abdominal pain. The gut–brain axis (GBA) consists of the CNS, the autonomic nervous system (ANS), the endocrine system (ENS), the hypothalamic-pituitary-adrenal (HPA) axis, and the immune system (34). The GBA is a bidirectional communication pathway between the gut and the CNS, involving multiple feedback loops and the interaction of different channels (34). The gut microbiota is closely linked to the CNS through the GBA (34). Existing evidence suggests that bottom-up regulation of the CNS by the gut microbiota occurs primarily through neuroimmune and neuroendocrine mechanisms, mediated by microbial-derived molecules such as short-chain fatty acids (SCFAs), secondary bile acids (2BAs), and tryptophan metabolites, which propagate signals primarily through interactions with enteroendocrine cells (EECs), enterochromatic cells (ECCs), and the mucosal immune system, with some molecules crossing the intestinal barrier, entering the systemic circulation, and possibly crossing the blood–brain barrier (BBB) (35). The ANS can transmit afferent and efferent nerve signals between the gut and the brain (35). The HPA axis is an important component of the neuroendocrine system (36). On the one hand, in pathological states in which gut bacteria are displaced and their abundance is altered, the intestinal microbiota produces pro-inflammatory cytokines such as lipopolysaccharides (e.g., endotoxin LPS) during the processes of composition and catabolism, and these affect the synthesis of γ-aminobutyric acid (GABA), serotonin, and glutamate, ultimately leading to the development of neurological inflammation (37). On the other hand, considering the role of the GBA, the emergence of an unhealthy gut microbiota can alter intestinal permeability and cause a series of problems. First, changes in intestinal permeability can easily cause damage to the intestinal barrier, resulting in increased permeability of the intestinal epithelium and, to a certain extent, decreased expression of intestinal junction proteins. Second, when the intestinal barrier is destroyed, inflammatory factors (such as microglia, inflammasomes, complement proteins, and cytokines) (38, 39), unhealthy intestinal microflora, and toxic substances become able to enter the circulatory system, activating the intestinal neuroimmune system, thereby destroying neuronal cells and affecting the onset and development of ALS (35, 36).
Bidirectional communication in the GBA occurs through multiple complex pathways. Although the precise pathways by which gut microbes communicate with the CNS through the GBA remain to be determined, both direct pathways (including the ENS and the vagus nerve) and indirect pathways (involving neurotransmitters, SCFAs, and cytokines) are known to be involved. The sympathetic and parasympathetic nervous systems and the vagus nerve, in turn, affect the CNS through the production of bacterial metabolites (40). The specific process is shown in Figure 1.
Figure 1. The GBA is a bidirectional communication pathway between the gut and the CNS involving multiple feedback loops. Changes in the gut microbiota lead to alterations in the intestinal epithelial barrier, and the gut microbiota produces a variety of neuroactive metabolites, including SCFAs, neurotransmitters, and endotoxins. These factors affect autophagy, activate an immune response, and lead to dysregulation of the GBA via the ascending pathway from the gut to the brain, triggering neuroinflammation and neurodegenerative diseases of the central nervous system, such as ALS, AD, and PD. A potential avenue for treatment of ALS may involve manipulation of the gut microbiota and its derivatives, including via intestinal mucosal resurfacing, dietary intervention, probiotic supplementation, antibiotics, and post-antibiotic therapy, among other potential methods.
The HPA axis, or neuroendocrine system, is an important component of the GBA (36); its role involves the transmission of information mainly through the vagus nerve. The neuroendocrine system, which is involved in controlling stress responses, consists primarily of the vagus nerve and sensory neurons that transmit information from the gut to the brain stem, which, in turn, includes the hypothalamus and the limbic system. Under stress stimulation, the limbic system in turn affects the autonomic activities of the intestine (41). In the GBA, responses to stressful stimuli (including digestion, immune system function, mood, and energy balance) are mediated through afferent and efferent nerves that in turn activate the HPA axis (41). The hypothalamus releases an adrenocorticotropin-releasing hormone (CRH), which reaches the pituitary gland through the bloodstream and stimulates the synthesis of adrenocorticotropic hormone (ACTH). ACTH stimulates the synthesis of glucocorticoids (stress hormones), such as cortisol or corticosterone, in the adrenal glands, resulting in a systemic response that leads to leakage from the tight junctions of the intestine, thus increasing the permeability of the intestinal barrier (41–43). Moreover, excessive release of cytokines (IL-1β, IL-6, and TNF-α) and small bioactive molecules, as well as gut microbial derivatives such as lipopolysaccharide (LPS) and peptidoglycan (44), means that these can cross the BBB into the brain and activate the HPA axis when there are changes in the diversity and abundance of the gut microbiota (42). These findings suggest that communication between the gut microbiota, the neuroendocrine system, and the brain occurs through multiple direct and indirect pathways; further exploration of the mechanisms involved is warranted.
The GBA is the main communication pathway between the ENS and the CNS (45). The ENS is responsible for coordinating intestinal functions such as intestinal motility, fluid secretion, and metabolite responses to gut microbiota production. Evidence currently suggests that specific bacterial strains may play a key role in the development and function of the ENS (43). The gut microbiota interacts with the ENS via the vagus nerve (46) and plays an important role in regulating the gastrointestinal tract, influencing the development and function of the ENS and enteroglial cells (EGCs). The gut microbiota also communicates with the CNS through direct pathways [via regulation of gut neuron survival and promotion of neurogenesis (47)] and through indirect pathways involving neurotransmitters (e.g., serotonin), bacterial metabolites (e.g., SCFAs), and cytokines (48, 49). In response to signals from the gut, the CNS sends regulatory messages to the intestinal microenvironment via ENS units of neuron–glial–epithelial cells, regulating the contraction of intestinal smooth muscle and the activity of glands and blood vessels (50). Gut microbes are key regulators of GBA function and modulation of neuronal activity via stimulation of the intestinal ENS (50).
The brain and the gastrointestinal tract are key sensory organs responsible for detecting, transmitting, integrating, and responding to signals from the in vivo and in vitro environment (51, 52). SCFAs are important metabolites of the gut microbiota, produced by the anaerobic fermentation of indigestible polysaccharides (e.g., dietary fiber and resistant starch) in the colon, and consist mainly of acetate (40%−60%), propionate (20%−25%), and butyrate (15%−20%) (53). The gut microbiota of the Bacillus produces acetate and propionate, and individuals of this thick-walled phylum produce large amounts of butyrate. SCFAs are mostly in ionized form and require transporter proteins for absorption. In the small intestine, transport of SCFAs is effected by monocarboxylate transporter protein (MCT)1 (SLC16A1), sodium-coupled MCT (SMCT)2 (SLC5A12), and SLC16A7; and in the colon, this function is fulfilled by MCT1 (SLC16A1), SMCT2 (SLC5A12), SMCT1 (SL5CA8), and SLC26A3. Therefore, the concentration of SCFAs depends on absorption and local physiological concentration (7). It has been shown (54) that the gut microbiota can regulate genes through metabolism, differentiation, and proliferation, and that butyrate regulates the expression of 5%−20% of human genes. SCFAs can help maintain the integrity of the intestinal epithelial barrier and the innate tolerance of intestinal immunity by regulating epithelial cell growth and differentiation and by affecting protein expression in tight junctions and mucosal permeability (55). They can also cross the BBB by upregulating the expression of tight junction proteins in the BBB with the assistance of endothelial cell monocarboxylate transport proteins (56). Through SCFAs, the gut microbiota actively communicates with host cells to regulate neurotrophic factors, neurotransmitters, and levels of neuroinflammation by affecting glial cell morphology and function, mitochondrial function, immune activation, lipid metabolism, and gene expression, thereby influencing CNS functions. In addition, various effects on host metabolism and the immune system have been observed (54, 57). For example, SCFAs (mainly butyrate) modulate the systemic inflammatory response by inducing T regulatory cell (Treg) differentiation and interleukin secretion. A significant elevation in the number of T cells was observed when germ-free (GF) mice were treated with SCFAs (58). Xiao et al. (59) found that modulation of the gut microbiota and supplementation with SCFAs promoted gastrointestinal transit, and modulated intestinal barrier function in a rat model of bilateral carotid artery obstruction, as well as improving symptoms of cognitive decline and depressive-like behavior, revealing the neuroprotective potential of gastrointestinal microbes. As this evidence shows, gut microbial metabolites (SCFAs) can exert a wide range of beneficial effects on the host and have been implicated in the prevention and treatment of many diseases. SCFAs affect GBA signaling and may directly or indirectly influence the pathophysiology of NDs (29).
Johnson and Foster (60) found that the different microbes of the gut microbiota secrete different neurotransmitters: e.g., Bacillus species secrete mainly acetylcholine, dopamine, and norepinephrine, while Escherichia secrete serotonin. The intestinal microbiota and its metabolites (e.g., SCFAs) can also produce serotonin, norepinephrine, and GABA through stimulation of intestinal chromogranin cells (52), and these neurotransmitters can reach the brain and directly affect central nervous system function through the intestinal epithelium or the BBB (24). Furthermore, we found that the overall proportions of various neurotransmitters are higher in the gut than in the brain (39), and Bellon et al. (61) found that enterochromaffin cells synthesize more than 90% of serotonin synthesized in vivo, this being a key neurotransmitter involved in regulation of mood, feeding, sleep, and pain processing (62). El Oussini et al. (63) found that, in mice with ALS-linked SOD1 mutations, the conditional allele of SOD1 (G37R) caused degeneration of serotonergic neurons, leading to the inactivation of 5-HT2B/C receptors, with clinical manifestations such as increased muscle tone and spasticity disorders. Additionally, in a mouse model, elective deletion of mutant SOD1 expression was found to maintain the serotonin innervation of spinal motor neurons to a large extent, alleviating the manifestations of increased muscle tone.
The gut microbiota releases byproducts from the cell wall, such as endotoxins like LPS, which can act locally and may be present in the gut, gingiva, and skin, as well as other tissues, causing dysregulation of GBA signaling (64), or can cross the intestinal barrier to enter the circulatory system; this is called metabolic endotoxemia (65). LPS is the organism's innate immune-sensing “alarm molecule” that elicits a host immune response (66). Alhasson (67) observed simultaneous intestinal inflammation and neuroinflammation in a mouse model of Gulf War disease, suggesting that alterations in the microbiota may lead to leaky gut and endotoxemia, exacerbating neuroinflammation and activation of Toll-like receptors (specifically, TLR4) in the small intestine and brain. TLR4 (68) is an innate mammalian receptor central to the immune system and plays a key role in LPS-mediated inflammatory responses by recognizing LPS, activating the TLR4 signaling pathway, releasing pro-inflammatory factors, and inducing inflammatory responses. Toll-like receptors TLR2 and TLR4 have recently been found to be expressed at higher levels in both the blood and the brain in patients with Parkinson's disease (69). Elevated blood LPS levels have been found in ALS patients (51), which may be attributable to gut inflammation and microbiota changes (64). Although the question of whether neuroinflammation is a cause or a consequence of ALS remains to be investigated, what can be stated is that widespread and prolonged neuroinflammation exacerbates the degeneration of motor neurons (70). TDP-43 is an ALS-specific mutated gene, and the addition of LPS to microglia or astrocytes in culture can cause improper localization and aggregation of TDP-43. The addition of peripheral LPS in TDP-43 (A315T) transgenic mice can lead to TDP-43 aggregation in vivo (71). This suggests that increased endotoxin levels, combined with TDP-43 aggregation, exacerbate neurodegeneration and are relevant to the pathogenesis of ALS.
The gut microbiota and its metabolites, such as SCFAs, contribute to protection of the integrity of the intestinal epithelial barrier. Studies have shown (24) that pathological changes in the composition of the intestinal microbiota can lead to disruption of the intestinal epithelial barrier and increased mucosal permeability. As well as inducing intestinal motor dysfunction, this disruption of the integrity of the barrier can also lead to dysregulation of the GBA via the ascending tracts of the gut–brain pathway, triggering neuroinflammatory and neurodegenerative conditions of the central nervous system, such as ALS, AD, multiple sclerosis (MS), and PD. A study by Wu et al. (48) found that an ALS-specific SOD1G93A mouse model exhibited dysregulation of the intestinal ecology (characterized by a reduction in the abundance of Vibrio fibrate butyric acidophilus, Escherichia coli, and thick-walled phylum) early in the disease progression, before the onset of neuromuscular symptoms. These changes were associated with reduced expression of tight junction (TJ) and adherent junction (AJ) proteins, as well as impairment of the intestinal barrier, leading to increased intestinal permeability. A permeability analysis of mouse sera revealed a two-fold increase in the fluorescence intensity readings of immunofluorescence-labeled FITC-dextrin in SOD1G93A mice compared to wild-type mice, further indicating altered intestinal integrity and impairment of the intestinal barrier in SOD1G93A mice. In addition, the number of abnormal Paneth cells and the level of pro-inflammatory IL-17 cytokines were increased in the intestinal tissues and blood of ALS mice. Zhang et al. (72) found, using the 16sRDNA technique, that butyrate-producing bacteria (Butyrivibrio Fibrisolvens) were reduced in SOD1G93A mice, but after butyrate treatment, the mice exhibited restoration of homeostasis of the intestinal flora, restoration of intestinal epithelial barrier integrity, and improvement in clinical signs. It can be hypothesized that damage to the intestinal barrier is correlated with the development of ALS.
Interaction between the host genome and the gut microbiota (53) affects the normal signaling of the GBA, and gene variants can regulate the gene-editing system of the natural gut flora and influence the enterobacterial composition. Significant progress has been made in identification of the genetic abnormalities associated with ALS, with at least 20 genes having been confirmed to be associated with the condition. The most common genes identified in clinical practice include SOD1, C9ORF72, TARDBP, FUS, and OPTNTD-P43 (55–57, 73, 74). Genetic variation activates phages that originally coexisted naturally with intestinal microbial hosts. Phages can cause immune disorders by recognizing foreign genetic elements and destroying their DNA fragments (75). Chronic neuroinflammation caused by immune disorders triggers the accumulation of misfolded proteins in nervous system cells and peripheral nerves (76), further leading to neuronal death (77). Aggregation of the now well-established SOD1 mutant protein is a hallmark of ALS pathology (78), and SOD1G93A mice (containing the human Cu/Zn superoxide dismutase gene mutation SOD1G93A) can serve as a model for the neuronal and muscle damage that occurs in human ALS. Intestinal biolysis and increased intestinal permeability have been observed in the SOD1G93A ALS mouse model (79). Zhang et al. (80) found that SOD1G93A mice exhibited changes in the intestinal microbiota at 1 month and structural defects in the intestinal nervous system starting from 2 months. The accumulation of SOD1G93A in the spinal cord and neurons serves as a molecular marker of the progression of ALS in SOD1G93A mice, beginning at 2 months of age; the aggregation of human SOD1G93A protein is observed in the white and gray matter of the lumbar spine, accompanied by increased levels of glial fibrillary acidic protein (GFAP) and neuromuscular symptoms such as decreased muscle strength, which are indicative of neuromuscular degeneration. Additionally, disruption of GFAP in the ENS suggests that the gut microbiota affects gut neuromuscular structure as well as function, and that imbalance in the gut microbiota may act as a potential trigger for degenerative diseases (81). Further research is needed to fully understand this relationship. The association between gut flora and ALS was revealed in a 2022 genome-wide association study (GWAS) of ALS (82), which analyzed data from 20,806 patients and 59,804 control individuals. Enterobacteriaceae and unclassified Acidaminococcaceae were found to be associated with a higher risk of ALS. Additionally, γ-glutamyl amino acids may be negatively associated with the risk of developing ALS, while γ-glutamyl phenylalanine is a specific risk factor for the disease. Both of its metabolites, 1-arachidonoyl-GPI and 3-methyl-2-oxobutyrate, have been found to increase the risk of developing ALS, whereas increased levels of 4-acetylaminobutyric acid may reduce the risk of ALS. Nezami and Srinivasan (83) found that transgenic mice with human ALS mutations (Prp-TDP43 A315T58) exhibited defects in the intestinal neurons, which may be associated with genetic mutations that result in abnormalities of the microbiota and the mucosal barrier. Another mouse model study (30) indicated that ALS-prone Sod1 transgenic (Sod1-Tg) mice exhibit significant mitigation of the decrease in intestinal motility as a result of improvement of the intestinal flora: specifically, Akkermansia muciniphila (AM) was found to alleviate symptoms of ALS, whereas Ruminococcus torques and Parabacteroides distasonis were found to exacerbate them. Studies of genetically mutated C9ORF72 mice have shown that mutations in C9ORF72 cause infiltration of neutrophils and other immune cells and activation of microglia in the spinal cord (79). A proinflammatory feeding environment leads to a decrease in the alpha diversity of the gut microbiota. When broad-spectrum antibiotics are used to reduce the burden of intestinal microbes, this phenotype is improved, and the lifespan of such mice is prolonged (74).
Notably, many severe neurodegenerative diseases, such as ALS, AD, and PD, are difficult to treat with conventional drugs. Neurodegenerative alterations are caused by a complex combination of genetic, environmental, and transgenic interactions, which are more closely linked than previously believed (84). There is a need for further elucidation of the effects of gene editing on the CNS, bottom-up through the GBA, via regulation of the gut microbiota.
The gut microbiota has been shown to play a fundamental role in deeply connecting the gut to the brain. Clinical and experimental evidence (48) suggests the presence of biological barriers to the gut microbiota and microbial metabolites in ALS. Of particular interest among the proteostasis pathways that have been characterized to date is the process known as autophagy (85). This self-consumptive pathway maintains cellular and organismal integrity in to the context of various developmental events or stressors by breaking down cellular components and organelles for reuse or redistribution. Chua et al. (85) have shown that autophagy and proteostasis are not only observed in experimental models of fALS, where specific genetic mutations can impair autophagic effectors or generate proteotoxic stress, but may also play a key role in the pathogenesis of sALS. Metabolites of the intestinal microbiota regulate intestinal inflammation through the autophagic pathway. In addition, autophagy in turn breaks down invading pathogens, regulates pathogens, triggers the release of pro-inflammatory cytokines, and participates in antigen presentation and lymphocyte development (25). Dysregulated autophagy mediated by dysbiosis affects the integrity of the intestinal barrier and increases intestinal permeability, thereby translocating intestinal microbes, their metabolites, and microbial-associated molecular patterns to mesenteric lymphoid tissue. The resulting neuroinflammatory response causes the development and progression of neurological disorders. Dysregulated autophagy leads to impaired clearance of polyubiquitinated protein aggregates in neurons and impairs endoplasmic reticulum (ER) homeostasis during stress, in aging, and in neurodegenerative diseases such as ALS. These findings suggest that the failure of the autophagic system to clear misfolded proteins is a major trigger of neuroinflammation and is closely associated with the development of ALS (86), in which inflammation propagates from somatic circulation to the brain via the cerebro-intestinal axis; they also suggest that protein misfolding and its aggregation, axonal damage, and neuronal demyelination are among the main mechanisms leading to neurodegenerative lesions. Wu et al. (48) found that the intestines of SOD1G93A mice (with impaired tight junction structures) exhibited increased permeability, significantly reduced expression levels of tight junction protein ZO-1 and adhesion junction protein E-cadherin, significantly reduced normal Paneth cells, and increased levels of inflammatory cytokine IL-17. Paneth cells are epithelial cells in the small intestine that regulate intestinal autophagic activity and host–bacteria interactions (38). Paneth cells contain antimicrobial peptides that play an important role (87) in the host's innate immune response and in shaping the gut microbiome. Dysregulation of autophagy appears to lead to the elimination of misfolded proteins, such as superoxide dismutase 1, in G93A mice, and this dismutant form accumulates in the motor neurons involved in the pathogenesis of ALS (88). At the same time, the reduced ability to promote intestinal dysfunction affects the functioning of the brain–gut axis and plays an important role in the pathophysiological function of the intestine, affecting the development of ALS (86). It is necessary to analyze intestinal autophagy in ALS patients to further explore its relevance to the pathogenesis of ALS, which will help us to develop new targets for ALS treatment strategies.
The gut microbiota and the gut immune system interact through the GBA to help maintain immune tolerance and shape the immune response during inflammation (89). The maturation and function of CNS-resident macrophages and microglia are controlled by the gut microbiota (54). Alterations to the gut bacteria indirectly affect the ratio of macrophages to microglia, with M1 (pro-inflammatory) microglia producing ROS and pro-inflammatory cytokines such as NO, IL-1β, IL-6, TNF-α, and IFN-γ, and M2 (anti-inflammatory)-like microglial cytoplasm being associated with production of anti-inflammatory cytokines such as IL-4, IL-10, and IL-13 (28, 90). Rodrigues et al. (90) found that mSOD1 microglia may contribute to the progression of ALS and that SOD1G93A increased the expression of the pro-inflammatory pattern recognition receptor RAGE, as well as releasing HMGB1- and SOD1-rich exosomes that promote pro-inflammatory responses. In addition, activation of TLR4 due to changes in gut microbiota composition and gut permeability further leads to the release of pro-inflammatory cytokines, endotoxins (LSP) (67), etc., from astrocytes and from the microglia themselves. LPS may alter gut homeostasis, inflammation, and permeability (91, 92) while activating various immune cells, including macrophages, neutrophils, and dendritic cells. Once activated (93), these cells produce a variety of pro-inflammatory cytokines, such as IL-2, IL-1β, IL-6, and TNF-α, which then cross the BBB into the brain via diffusion and cytokine transporters. These pro-inflammatory cytokines act on neuronal and glial cell-expressed receptors such as IL-1β, IL-6, and TNF-α, leading to neuroinflammation and neuronal death (94). Astrocytes (95), the most abundant glial cells in the nervous system, are important immune cells that play a key role in maintaining the BBB and regulating immune cell traffic and pro-inflammatory responses; they are widely found to be present in the postmortem brain tissue, cerebral white matter, and spinal cord of sALS and fALS patients. Gomes et al. (96) found that cortical astrocytes isolated from SOD1G93A mouse model in the early stages of the disease exhibited neuroprotective features, with reduced cell proliferation, low expression of NF-κB and micro(miR)-146a, and weakened pro-inflammatory pathways, indicating impaired immune function in ALS patients. Changes in the gut microbiota also occur in ALS: in a controlled observational study of 37 ALS patients and 29 healthy BMI- and age-matched family members, Blacher et al. (30) found significant differences in gut flora and overall bacterial gene content between the ALS patients and the healthy controls. In most patients, stool analysis revealed the presence of inflammatory biomarkers, including fecal secretion of IgA, calcitonin, and eosinophil X. The presence of circulating endotoxins and activation of the immune system suggest disruption of the intestinal barrier and local inflammation in ALS. In a study of six ALS patients by Fang et al. (25), the fecal microbiota of ALS patients was noted to exhibit reduced Firmicutes/Bacteroidetes in proportion to an increase in the abundance of Dorea and a concomitant decrease in the abundance of Oscillibacter, Anaerostipes, and Lachnospiraceae. It has been found that most patients exhibit a decrease in microbiota diversity, including a decrease in the Firmicutes/Bacteroidetes (F/B) ratio, which is an indicator of dysbiosis. Changes in the F/B ratio have been found to be associated with various disorders, such as irritable bowel syndrome and inflammatory bowel disease (97), as well as with neurodegenerative diseases, such as PD, AD, and ALS (98). Several studies have shown that neuroinflammation is associated with the progression of ALS and that the microbiota, as a modifier of neurodegenerative disease risk (25), is closely associated with the inflammatory immune response. Given its role as the beginning of the GBA, further investigation of the corresponding immune mechanisms is warranted.
It has been shown that altering the gut microbiota has clinical efficacy in the treatment of neurodegenerative diseases (99); gut-centered therapies include dietary interventions, next-generation probiotics, and fecal microbial transplants.
In the early 20th century, Nobel laureate Elie Metchnikoff proposed the concept of probiotics as “life” (3). The modern definition of probiotics is now widely recognized as “live microorganisms that when administered in adequate amounts confer a beneficial health effect on the host.” Probiotics are considered to be dietary factors that can influence the human intestinal microbiota, which can affect the composition and structure of the intestinal flora; which, in turn, plays a role in protecting the intestinal epithelial barrier, inhibiting pathogen production, and regulating the immune system (3). Studies have shown that the composition and products of the intestinal flora have a strong influence on immune response (16), and that the central nervous system is also influenced by the immune system (100). SCFAs, as important metabolites of the intestinal flora, can modulate the immune response (38) and act as important communication signals on the brain–gut axis (39). Through use of probiotics, the number of SCFA-producing microorganisms in the intestine can be increased (3). Abraham et al. (10) found that transgenic AD mice treated with probiotics exhibited better cognitive performance and a reduced number of Aβ plaques in the hippocampus compared to untreated AD mice. Akbari et al. (101) found that supplementation of Lactobacillus acidophilus, Lactobacillus casei, Bifidobacterium bifidum, and Lactobacillus fermentum improved cognitive function in patients with AD through facilitation of the normal functioning of the GBA pathway. Similarly, in a prospective longitudinal study of patients with ALS, Di Gioia et al. (102) found that intestinal flora was restored by probiotic supplementation compared to normal controls.
Zhang et al. (72) found that treatment of ALS mice with oxalate extended their life span by an average of 38 days. This demonstrates the effectiveness of oxalate, a natural bacterial product, in restoring microbial and intestinal homeostasis. Additionally, treatment of ALS mice with butyrate resulted in a significant decrease in the percentage of abnormal Paneth cells in the intestine. This treatment also restored the levels of lysozyme 1 and antimicrobial peptide defensin 5α in the intestine, as well as improving and slowing pathological progression of the intestine. This indicates the important role of butyrate in maintaining the physiological integrity of the intestine. Yang et al. (103) found that treatment with probiotic double immuno-galactooligosaccharide (Bimuno® galactooligosaccharide; B-GOS) alleviated neuroinflammation and cognitive function in rats and greatly inhibited microglia activation, as well as expression of iNOS, CD68, CD32, SOCS3, and IL-6 expression. The use of symbiotic A. muciniphila supplementation alleviated ALS symptoms, while Ruminococcus torque and P. distasonis exacerbated these symptoms in Tg mice (74). Probiotic-4 (which contains Lactobacillus, Bifidobacterium, Typhimurium, and Eosinophilus) has been shown to attenuate aging-related disruptions in the integrity of the blood–brain barrier and the intestinal barrier; in plasma and brain LPS levels; and in IL-6, TNF-α, TLR4, and NF-κB translocation in the brains of aged mice (104). New research is now emerging on the use of synthetic bacterial therapies (105), in which engineered probiotics can be specifically designed to transform toxic metabolites in the gut into non-toxic forms. In addition to the potential to restore microbial homeostasis, probiotics can be considered as a vehicle for delivery of neuroactive compounds, which function as vital links in the brain–gut axis. Thus, probiotics should be considered a possible therapeutic/preventive strategy for neurological disorders.
Włodarek et al. (106) found that a ketogenic diet, which is high in fat, moderate in protein, and low in carbohydrates, has the potential to reduce neuronal degeneration, and that this specific type of nutritional intervention may provide neuroprotection. Goncharova et al. (107), found that supplementation of pharmacological treatment with vitamins and changing the diet to a ketogenic diet could reduce the rate of motor neuron degeneration in ALS patients. However, it is important to note that the approach to dietary selection must be individualized. Modifying the gut microbiota through the diet and altering the signaling pathway of the GBA from the bottom up can slow down the rate of disease progression. Chico et al. (108) supplemented the diets of ALS patients with curcumin, a non-flavonoid polyphenol, and observed no clear progression of the disease after 6 months of follow-up. Fitzgerald et al. (109) concluded that total carotenoid intake was associated the risk of ALS. Polyphenols are a class of compounds composed of a variety of molecules; several studies in animal models of ALS have shown that these have neuroprotective effects (110). An effective anti-ALS food or compound typically appears to have at least one of either anti-inflammatory or antioxidant properties, as oxidative stress and inflammation play an important role in neuronal degeneration (111). Changing the gut microbiome through the diet and changing the signaling pathways of the GBA from the bottom up can slow the rate of disease progression.
Fecal microbiota transplantation (FMT) (112) refers to the transplantation of functional flora from the feces of healthy humans into the gastrointestinal tract of patients in order to establish new intestinal flora, with the goal of treating either intestinal or extraintestinal diseases. Zhang et al. (113) transplanted intestinal flora from patients with major depression into the intestine of GF mice and found that the mice developed depression-like behavior. Lu et al. (114) reported the case of a woman with ALS who was found at 12-month follow-up to have benefited from a washed microbiome transplant (WMT), an improved form of FMT, via a trans endoscopic bowel tube. The patient later suffered an unexpected scalp trauma, which was treated with prescribed antibiotics, leading to worsening of her ALS. The subsequent use of WMT rapidly improved this situation, successfully halting the progression of the disease. Based on current findings (115), FMT has the potential to play a role in treatment of neurological disorders such as ALS. The relatively low cost and low risk of FMT necessitates the study of mouse models of ALS for further exploration of this developing field.
The gut microbiota plays a key role in the neuropathogenesis of CNS-related diseases by directly or indirectly affecting the function of the GBA. Improving intestinal ecology through methods such as probiotic supplementation, dietary intervention, and FMT can help restore the intestinal ecology in ASL patients and improve the normal physiological functioning of the GBA. This may represent a new treatment strategy for central nervous system-related diseases such as ALS.
Antibiotics have recently found new uses in the treatment of NDs (116). In particular, beta-lactams (cephalosporin antibiotics) have been shown to be effective in the treatment and alleviation of ND symptoms and in the treatment of experimentally induced neurological disorders such as PD, AD, ALS, traumatic brain injury (TBI), epilepsy, cerebral ischemia, and neuropathy (84, 117, 118). CFT has been reported to have neuroprotective and anti-inflammatory effects, to protect neurons from oxidative stress and ionizing radiation, and to act as a free radical scavenger (84). Correlations between ALS and environmental effects have been found to suggest that SOD-1-Tg mice tend to exhibit virus-dependent biolysis and abnormal metabolite patterns during the prodromal phase when treated with broad-spectrum antibiotics (119). The results of this study suggest a direct correlation between viruses exposed to antibiotic treatment in SOD-1-Tg mice and certain bacterial species, particularly mucinophilic granulocytes that alleviate the symptoms of ALS and (Erythrocyte torque and parabacteria), which exacerbate the symptoms of ALS. Mice treated with the mucophilic granulocyte Akkermansia have also been found to show accumulation of nicotinamide in the central nervous system. Nicotinamide has recently been investigated to determine its relevance in improving ALS symptoms and thus motor function (120).
Bacterial metabolites, also known as post-antibiotics (121) or postbiotics, were treated with the bacterial product butyrate, an SCFA, in a study with SOD1G93A mice (50). Butyrate treatment improves intestinal integrity and microbial homeostasis, acting along the brain–gut axis, and was found to have beneficial effects in SOD1G93A mice in terms of extending the lifespan and delaying the progression of ALS.
Targeted therapies have gained popularity in recent years, and novel treatment options have become available, including phage therapy, carbon nanoparticles, and intestinal mucosal resurfacing. Further research on these approaches may hold promise for application in the treatment of ALS.
Phages (121) are viruses that specifically infect and kill particular intestinal bacterial pathogens. Unlike antibiotics, phages do not induce drug resistance (122). Ghadge et al. (123) used phage-specific raised single-chain variable fragment antibodies (scFvs) against SOD1G93A in mice and found that these antibodies reduced motor neuron loss, microgliosis, astrocytosis, and SOD1 burden and aggregation. Improvements were observed in the aggregation and in vitro toxicity of mutant SOD1. Federici et al. (124) demonstrated that oral compound phage-targeted therapy can inhibit human intestinal microbiota symbiosis, improve intestinal inflammation, and effectively avoid antibiotic resistance. It can be speculated that the use of “phage cocktail” therapy or oral phage-targeted drugs to enhance SOD1 antibodies or antibody mimetics may be an effective treatment option for fALS (75, 76).
Carbon nanoparticles (125) have been shown to have a high adsorption capacity for bacterial toxins such as LPS. They are currently used in patients with advanced cirrhosis to improve their intestinal microbiota and are a new strategy for counteracting the biodivision and translocation of bacterial-derived products.
Intestinal mucosal resurfacing (124) is a technique that targets the duodenal surface in order to improve the intestinal barrier. This technique is currently used in patients with type 2 diabetes to improve the proliferation of enteroendocrine cells and endocrine cells, ultimately improving blood glucose levels.
ALS is a multifactorial syndrome as opposed to a single disease (115). Clinical treatment of various targets via the intestinal microbiota and its metabolites, including treatments aiming to improve the intestinal mucosal barrier and alter intestinal permeability, has the potential to treat ALS. Intestinal microbiota-mediated phage therapy can reduce both intestinal inflammation and neuroinflammation, decrease the accumulation of abnormal protein in motor neurons, reduce apoptosis, promote autophagy, and inhibit neuroinflammation. Therefore, intestinal mucosal resurfacing may be a promising therapy for ALS via the mechanisms of improving the gut microbiota and affecting GBA communication. Specific schemes are summarized in Table 1.
ALS is a progressive and ultimately fatal disease that affects the motor neurons. The gut microbiota plays a key role in the pathogenesis of ALS by directly or indirectly altering GBA function. The review presented in this article suggests the conclusion that neuroinflammation is induced by an increased burden of pathogenic microbes and metabolites, compromising the intestinal barrier and activating immune regulation. These resulting induction of immune dysfunction, deposition and propagation of misfolded proteins, and autophagic responses marked by chronic inflammation impair normal neurons and contribute to the development of ALS. We can treat ALS via the gut microbiota and related derivatives; such treatment approaches include improving the intestinal microecology and repairing the intestinal barrier through probiotic supplementation, dietary intervention, or fecal transplantation. The use of antibiotics, post-antibiotic therapy, and targeted therapeutic approaches such as phage therapy can improve the gut microbiota and impact the nervous system via the brain–gut axis, opening up a new therapeutic avenue. Given the wide variety of derivatives of the gut microbiota machinery and the complex interactions of these derivatives with the GBA, a promising strategy is to combine multiple approaches from the domains of metabolomics, metagenomics, transcriptomics, and proteomics in order to identify the bacterial genes involved in regulation and to validate their potential efficacy as treatments for GBA-related diseases. Thus, with mounting evidence demonstrating the feasibility of treating ALS through mechanisms such as modulation of the gut microbiota, it is becoming increasingly plausible that modulation of the GBA via the gut microbiota represents a new era in the treatment of ALS.
CZ devised the study. WW and XL were involved in the conception of the study and critically revised the manuscript. DH and LZ were involved in writing the article. All authors agreed to be accountable for the content of this article.
This study was supported by the Zhejiang Provincial Basic Public Welfare Research Project (GD21H270008).
The authors declare that the research was conducted in the absence of any commercial or financial relationships that could be construed as a potential conflict of interest.
All claims expressed in this article are solely those of the authors and do not necessarily represent those of their affiliated organizations, or those of the publisher, the editors and the reviewers. Any product that may be evaluated in this article, or claim that may be made by its manufacturer, is not guaranteed or endorsed by the publisher.
1. Longinetti E, Fang F. Epidemiology of amyotrophic lateral sclerosis: an update of recent literature. Curr Opin Neurol. (2019) 32:771–6. doi: 10.1097/WCO.0000000000000730
2. Shatunov A, Al-Chalabi A. The genetic architecture of ALS. Neurobiol Dis. (2021) 147:105156. doi: 10.1016/j.nbd.2020.105156
3. Petrov D, Mansfield C, Moussy A, Hermine O. ALS clinical trials review: 20 years of failure. Are we any closer to registering a new treatment? Front Aging Neurosci. (2017) 9:68. doi: 10.3389/fnagi.2017.00068
4. Dhasmana S, Dhasmana A, Narula AS, Jaggi M, Yallapu MM, Chauhan SC, et al. The panoramic view of amyotrophic lateral sclerosis: a fatal intricate neurological disorder. Life Sci. (2022) 288:120156. doi: 10.1016/j.lfs.2021.120156
5. Andrew AS, Caller TA, Tandan R, Duell EJ, Henegan PL, Field NC, et al. Environmental and occupational exposures and amyotrophic lateral sclerosis in New England. Neurodegener Dis. (2017) 17:110–6. doi: 10.1159/000453359
6. Nowicka N, Juranek J, Juranek JK, Wojtkiewicz J. Risk factors and emerging therapies in amyotrophic lateral sclerosis. Int J Mol Sci. (2019) 20:2616. doi: 10.3390/ijms20112616
7. Meijboom KE, Brown RH. Approaches to gene modulation therapy for ALS. Neurotherapeutics. (2022) 19:1159–79. doi: 10.1007/s13311-022-01285-w
8. Mazzini L, Gelati M, Profico DC, Sorarù G, Ferrari D, Copetti M, et al. Results from phase I clinical trial with intraspinal injection of neural stem cells in amyotrophic lateral sclerosis: a long-term outcome. Stem Cells Transl Med. (2019) 8:887–97. doi: 10.1002/sctm.18-0154
9. Khamaysa M, Pradat PF. Status of ALS treatment, insights into therapeutic challenges and dilemmas. J Pers Med. (2022) 12:1601. doi: 10.3390/jpm12101601
10. Abraham A, Nefussy B, Fainmesser Y, Ebrahimi Y, Karni A, Drory VE, et al. Early post-marketing experience with edaravone in an unselected group of patients with ALS. Amyotroph Lateral Scler Frontotemporal Degener. (2019) 20:260–3. doi: 10.1080/21678421.2019.1572191
11. Paganoni S, Macklin EA, Hendrix S, Berry JD, Elliott MA, Maiser S, et al. Trial of sodium phenylbutyrate-taurursodiol for amyotrophic lateral sclerosis. N Engl J Med. (2020) 383:919–30. doi: 10.1056/NEJMoa1916945
12. Rooks MG, Garrett WS. Gut microbiota, metabolites and host immunity. Nat Rev Immunol. (2016) 16:341–52. doi: 10.1038/nri.2016.42
13. Agus A, Planchais J, Sokol H. Gut microbiota regulation of tryptophan metabolism in health and disease. Cell Host Microbe. (2018) 23:716–24. doi: 10.1016/j.chom.2018.05.003
14. Mitrea L, Nemeş SA, Szabo K, Teleky BE, Vodnar DC. Guts imbalance imbalances the brain: a review of gut microbiota association with neurological and psychiatric disorders. Front Med. (2022) 9:813204. doi: 10.3389/fmed.2022.813204
15. Barrio C, Arias-Sánchez S, Martín-Monzón I. The gut microbiota-brain axis, psychobiotics and its influence on brain and behaviour: a systematic review. Psychoneuroendocrinology. (2022) 137:105640. doi: 10.1016/j.psyneuen.2021.105640
16. Zhou B, Yuan Y, Zhang S, Guo C, Li X, Li G, et al. Intestinal flora and disease mutually shape the regional immune system in the intestinal tract. Front Immunol. (2020) 11:575. doi: 10.3389/fimmu.2020.00575
17. Gomaa EZ. Human gut microbiota/microbiome in health and diseases: a review. Antonie Van Leeuwenhoek. (2020) 113:2019–40. doi: 10.1007/s10482-020-01474-7
18. Wang Y, Zhou Y, Xiao X, Zheng J, Zhou H. Metaproteomics: a strategy to study the taxonomy and functionality of the gut microbiota. J Proteomics. (2020) 219:103737. doi: 10.1016/j.jprot.2020.103737
19. Aron-Wisnewsky J, Warmbrunn MV, Nieuwdorp M, Clément K. Metabolism and metabolic disorders and the microbiome: the intestinal microbiota associated with obesity, lipid metabolism, and metabolic health-pathophysiology and therapeutic strategies. Gastroenterology. (2021) 160:573–99. doi: 10.1053/j.gastro.2020.10.057
20. Lee M, Chang EB. Inflammatory bowel diseases (IBD) and the microbiome-searching the crime scene for clues. Gastroenterology. (2021) 160:524–37. doi: 10.1053/j.gastro.2020.09.056
21. Tong Y, Gao H, Qi Q, Liu X, Li J, Gao J, et al. High fat diet, gut microbiome and gastrointestinal cancer. Theranostics. (2021) 11:5889–910. doi: 10.7150/thno.56157
22. Miller AW, Penniston KL, Fitzpatrick K, Agudelo J, Tasian G, Lange D, et al. Mechanisms of the intestinal and urinary microbiome in kidney stone disease. Nat Rev Urol. (2022) 19:695–707. doi: 10.1038/s41585-022-00647-5
23. Song M, Chan AT, Sun J. Influence of the gut microbiome, diet, and environment on risk of colorectal cancer. Gastroenterology. (2020) 158:322–40. doi: 10.1053/j.gastro.2019.06.048
24. Pellegrini C, Antonioli L, Colucci R, Blandizzi C, Fornai M. Interplay among gut microbiota, intestinal mucosal barrier and enteric neuro-immune system: a common path to neurodegenerative diseases? Acta Neuropathol. (2018) 136:345–61. doi: 10.1007/s00401-018-1856-5
25. Fang P, Kazmi SA, Jameson KG, Hsiao EY. The microbiome as a modifier of neurodegenerative disease risk. Cell Host Microbe. (2020) 28:201–22. doi: 10.1016/j.chom.2020.06.008
26. Mou Y, Du Y, Zhou L, Yue J, Hu X, Liu Y, et al. Gut microbiota interact with the brain through systemic chronic inflammation: implications on neuroinflammation, neurodegeneration, and aging. Front Immunol. (2022) 13:796288. doi: 10.3389/fimmu.2022.796288
27. Sun MF, Shen YQ. Dysbiosis of gut microbiota and microbial metabolites in Parkinson's disease. Ageing Res Rev. (2018) 45:53–61. doi: 10.1016/j.arr.2018.04.004
28. Wang Q, Luo Y, Ray Chaudhuri K, Reynolds R, Tan EK, Pettersson S, et al. The role of gut dysbiosis in Parkinson's disease: mechanistic insights and therapeutic options. Brain. (2021) 144:2571–93. doi: 10.1093/brain/awab156
29. Chidambaram SB, Essa MM, Rathipriya AG, Bishir M, Ray B, Mahalakshmi AM, et al. Gut dysbiosis, defective autophagy and altered immune responses in neurodegenerative diseases: tales of a vicious cycle. Pharmacol Therap. (2022) 231:107988. doi: 10.1016/j.pharmthera.2021.107988
30. Blacher E, Bashiardes S, Shapiro H, Rothschild D, Mor U, Dori-Bachash M, et al. Potential roles of gut microbiome and metabolites in modulating ALS in mice. Nature. (2019) 572:474–80. doi: 10.1038/s41586-019-1443-5
31. Boddy SL, Giovannelli I, Sassani M, Cooper-Knock J, Snyder MP, Segal E, et al. The gut microbiome: a key player in the complexity of amyotrophic lateral sclerosis (ALS). BMC Med. (2021) 19:13. doi: 10.1186/s12916-020-01885-3
32. McCombe PA, Henderson RD, Lee A, Lee JD, Woodruff TM, Restuadi R, et al. Gut microbiota in ALS: possible role in pathogenesis? Expert Rev Neurother. (2019) 19:785–805. doi: 10.1080/14737175.2019.1623026
33. Rowin J, Xia Y, Jung B, Sun J. Gut inflammation and dysbiosis in human motor neuron disease. Physiol Rep. (2017) 5. doi: 10.14814/phy2.13443
34. Mayer EA, Nance K, Chen S. The gut-brain axis. Annu Rev Med. (2022) 73:439–53. doi: 10.1146/annurev-med-042320-014032
35. Martin CR, Osadchiy V, Kalani A, Mayer EA. The brain-gut-microbiome axis. Cell Mol Gastroenterol Hepatol. (2018) 6:133–48. doi: 10.1016/j.jcmgh.2018.04.003
36. Osadchiy V, Martin CR, Mayer EA. The gut-brain axis and the microbiome: mechanisms and clinical implications. Clin Gastroenterol Hepatol. (2019) 17:322–32. doi: 10.1016/j.cgh.2018.10.002
37. Trzeciak P, Herbet M. Role of the intestinal microbiome, intestinal barrier and psychobiotics in depression. Nutrients. (2021) 13:927. doi: 10.3390/nu13030927
38. Poole S, Singhrao SK, Chukkapalli S, Rivera M, Velsko I, Kesavalu L, et al. Active invasion of Porphyromonas gingivalis and infection-induced complement activation in ApoE-/- mice brains. J Alzheimers Dis. (2015) 43:67–80. doi: 10.3233/JAD-140315
39. Brouwers N, Van Cauwenberghe C, Engelborghs S, Lambert JC, Bettens K, Le Bastard N, et al. Alzheimer risk associated with a copy number variation in the complement receptor 1 increasing C3b/C4b binding sites. Mol Psychiatry. (2012) 17:223–33. doi: 10.1038/mp.2011.24
40. Labanski A, Langhorst J, Engler H, Elsenbruch S. Stress and the brain-gut axis in functional and chronic-inflammatory gastrointestinal diseases: a transdisciplinary challenge. Psychoneuroendocrinology. (2020) 111:104501. doi: 10.1016/j.psyneuen.2019.104501
41. Farzi A, Fröhlich EE, Holzer P. Gut microbiota and the neuroendocrine system. Neurotherapeutics. (2018) 15:5–22. doi: 10.1007/s13311-017-0600-5
42. Jang HM, Lee KE, Lee HJ, Kim DH. Immobilization stress-induced Escherichia coli causes anxiety by inducing NF-κB activation through gut microbiota disturbance. Sci Rep. (2018) 8:13897. doi: 10.1038/s41598-018-31764-0
43. Chudzik A, Orzyłowska A, Rola R, Stanisz GJ. Probiotics, prebiotics and postbiotics on mitigation of depression symptoms: modulation of the brain-gut-microbiome axis. Biomolecules. (2021) 11:1000. doi: 10.3390/biom11071000
44. Juruena MF, Bocharova M, Agustini B, Young AH. Atypical depression and non-atypical depression: is HPA axis function a biomarker? A systematic review. J Affect Disord. (2018) 233:45–67. doi: 10.1016/j.jad.2017.09.052
45. Giuffrè M, Moretti R, Campisciano G, da Silveira ABM, Monda VM, Comar M, et al. You talking to me? Says the enteric nervous system (ENS) to the microbe how intestinal microbes interact with the ENS. J Clin Med. (2020) 9:3705. doi: 10.3390/jcm9113705
46. Ahlawat S, Asha, Sharma KK. Gut-organ axis: a microbial outreach and networking. Lett Appl Microbiol. (2021) 72:636–68. doi: 10.1111/lam.13333
47. Vicentini FA, Keenan CM, Wallace LE, Woods C, Cavin JB, Flockton AR, et al. Intestinal microbiota shapes gut physiology and regulates enteric neurons and glia. Microbiome. (2021) 9:210. doi: 10.1186/s40168-021-01165-z
48. Wu S, Yi J, Zhang YG, Zhou J, Sun J. Leaky intestine and impaired microbiome in an amyotrophic lateral sclerosis mouse model. Physiol Rep. (2015) 3:e12356. doi: 10.14814/phy2.12356
49. Dicks LMT. Gut bacteria and neurotransmitters. Microorganisms. (2022) 100:1838. doi: 10.3390/microorganisms10091838
50. Zhao L, Xiong Q, Stary CM, Mahgoub OK, Ye Y, Gu L, et al. Bidirectional gut-brain-microbiota axis as a potential link between inflammatory bowel disease and ischemic stroke. J Neuroinflammation. (2018) 15:339. doi: 10.1186/s12974-018-1382-3
51. Zhang R, Miller RG, Gascon R, Champion S, Katz J, Lancero M, et al. Circulating endotoxin and systemic immune activation in sporadic amyotrophic lateral sclerosis (sALS). J Neuroimmunol. (2009) 206:121–4. doi: 10.1016/j.jneuroim.2008.09.017
52. Strandwitz P. Neurotransmitter modulation by the gut microbiota. Brain Res. (2018) 1693:128–33. doi: 10.1016/j.brainres.2018.03.015
53. Imhann F, Vich Vila A, Bonder MJ, Fu J, Gevers D, Visschedijk MC, et al. Interplay of host genetics and gut microbiota underlying the onset and clinical presentation of inflammatory bowel disease. Gut. (2018) 67:108–19. doi: 10.1136/gutjnl-2016-312135
54. Sharon G, Sampson TR, Geschwind DH, Mazmanian SK. The central nervous system and the gut microbiome. Cell. (2016) 167:915–32. doi: 10.1016/j.cell.2016.10.027
55. Kim G, Gautier O, Tassoni-Tsuchida E, Ma XR, Gitler AD. ALS genetics: gains, losses, and implications for future therapies. Neuron. (2020) 108:822–42. doi: 10.1016/j.neuron.2020.08.022
56. DeJesus-Hernandez M, Mackenzie IR, Boeve BF, Boxer AL, Baker M, Rutherford NJ, et al. Expanded GGGGCC hexanucleotide repeat in noncoding region of C9ORF72 causes chromosome 9p-linked FTD and ALS. Neuron. (2011) 72:245–56. doi: 10.1016/j.neuron.2011.09.011
57. Renton AE, Majounie E, Waite A, Simón-Sánchez J, Rollinson S, Gibbs JR, et al. A hexanucleotide repeat expansion in C9ORF72 is the cause of chromosome 9p21-linked ALS-FTD. Neuron. (2011) 72:257–68. doi: 10.1016/j.neuron.2011.09.010
58. Manfredsson FP, Luk KC, Benskey MJ, Gezer A, Garcia J, Kuhn NC, et al. Induction of alpha-synuclein pathology in the enteric nervous system of the rat and non-human primate results in gastrointestinal dysmotility and transient CNS pathology. Neurobiol Dis. (2018) 112:106–18. doi: 10.1016/j.nbd.2018.01.008
59. Xiao W, Su J, Gao X, Yang H, Weng R, Ni W, et al. The microbiota-gut-brain axis participates in chronic cerebral hypoperfusion by disrupting the metabolism of short-chain fatty acids. Microbiome. (2022) 10:62. doi: 10.1186/s40168-022-01255-6
60. Johnson KV, Foster KR. Why does the microbiome affect behaviour? Nat Rev Microbiol. (2018) 16:647–55. doi: 10.1038/s41579-018-0014-3
61. Bellono NW, Bayrer JR, Leitch DB, Castro J, Zhang C, O'Donnell TA, et al. Enterochromaffin cells are gut chemosensors that couple to sensory neural pathways. Cell. (2017) 170:185–98.e16. doi: 10.1016/j.cell.2017.05.034
62. Gao K, Mu CL, Farzi A, Zhu WY. Tryptophan metabolism: a link between the gut microbiota and brain. Adv Nutr. (2020) 11:709–23. doi: 10.1093/advances/nmz127
63. El Oussini H, Scekic-Zahirovic J, Vercruysse P, Marques C, Dirrig-Grosch S, Dieterlé S, et al. Degeneration of serotonin neurons triggers spasticity in amyotrophic lateral sclerosis. Ann Neurol. (2017) 82:444–56. doi: 10.1002/ana.25030
64. Brown GC. The endotoxin hypothesis of neurodegeneration. J Neuroinflammation. (2019) 16:180. doi: 10.1186/s12974-019-1564-7
65. Bolte LA, Vich Vila A, Imhann F, Collij V, Gacesa R, Peters V, et al. Long-term dietary patterns are associated with pro-inflammatory and anti-inflammatory features of the gut microbiome. Gut. (2021) 70:1287–98. doi: 10.1136/gutjnl-2020-322670
66. Opal SM. Endotoxins and other sepsis triggers. Contrib Nephrol. (2010) 167:14–24. doi: 10.1159/000315915
67. Seth RK, Kimono D, Alhasson F, Sarkar S, Albadrani M, Lasley SK, et al. Increased butyrate priming in the gut stalls microbiome associated-gastrointestinal inflammation and hepatic metabolic reprogramming in a mouse model of Gulf War Illness. Toxicol Appl Pharmacol. (2018) 350:64–77. doi: 10.1016/j.taap.2018.05.006
68. Zhang J, Shang D. [Research progress of TLR4 signaling pathway and its targeted drugs]. Xi Bao Yu Fen Zi Mian Yi Xue Za Zhi. (2021) 37:657–62. doi: 10.13423/j.cnki.cjcmi.009231
69. Gorecki AM, Anyaegbu CC, Anderton RS. TLR2 and TLR4 in Parkinson's disease pathogenesis: the environment takes a toll on the gut. Transl Neurodegener. (2021) 10:47. doi: 10.1186/s40035-021-00271-0
70. Bowerman M, Vincent T, Scamps F, Perrin FE, Camu W, Raoul C, et al. Neuroimmunity dynamics and the development of therapeutic strategies for amyotrophic lateral sclerosis. Front Cell Neurosci. (2013) 7:214. doi: 10.3389/fncel.2013.00214
71. Correia AS, Patel P, Dutta K, Julien JP. Inflammation induces TDP-43 mislocalization and aggregation. PLoS ONE. (2015) 10:e0140248. doi: 10.1371/journal.pone.0140248
72. Zhang YG, Wu S, Yi J, Xia Y, Jin D, Zhou J, et al. Target intestinal microbiota to alleviate disease progression in amyotrophic lateral sclerosis. Clin Ther. (2017) 39:322–36. doi: 10.1016/j.clinthera.2016.12.014
73. Ma XR, Prudencio M, Koike Y, Vatsavayai SC, Kim G, Harbinski F, et al. TDP-43 represses cryptic exon inclusion in the FTD-ALS gene UNC13A. Nature. (2022) 603:124–30. doi: 10.1038/s41586-022-04424-7
74. Burberry A, Wells MF, Limone F, Couto A, Smith KS, Keaney J, et al. C9orf72 suppresses systemic and neural inflammation induced by gut bacteria. Nature. (2020) 582:89–94. doi: 10.1038/s41586-020-2288-7
75. Hsu BB, Gibson TE, Yeliseyev V, Liu Q, Lyon L, Bry L, et al. Dynamic modulation of the gut microbiota and metabolome by bacteriophages in a mouse model. Cell Host Microbe. (2019) 25:803–14.e5. doi: 10.1016/j.chom.2019.05.001
76. Shuwen H, Kefeng D. Intestinal phages interact with bacteria and are involved in human diseases. Gut Microbes. (2022) 14:2113717. doi: 10.1080/19490976.2022.2113717
77. Boiziau C, Nikolski M, Mordelet E, Aussudre J, Vargas-Sanchez K, Petry KG, et al. A peptide targeting inflammatory CNS lesions in the EAE rat model of multiple sclerosis. Inflammation. (2018) 41:932–47. doi: 10.1007/s10753-018-0748-0
78. Rosen DR. Mutations in Cu/Zn superoxide dismutase gene are associated with familial amyotrophic lateral sclerosis. Nature. (1993) 364:362. doi: 10.1038/364362c0
79. Sun J, Huang T, Debelius JW, Fang F. Gut microbiome and amyotrophic lateral sclerosis: a systematic review of current evidence. J Intern Med. (2021) 290:758–88. doi: 10.1111/joim.13336
80. Zhang Y, Ogbu D, Garrett S, Xia Y, Sun J. Aberrant enteric neuromuscular system and dysbiosis in amyotrophic lateral sclerosis. Gut Microbes. (2021) 13:1996848. doi: 10.1080/19490976.2021.1996848
81. Chan DG, Ventura K, Villeneuve A, Du Bois P, Holahan MR. Exploring the connection between the gut microbiome and parkinson's disease symptom progression and pathology: implications for supplementary treatment options. J Parkinsons Dis. (2022) 12:2339–52. doi: 10.3233/JPD-223461
82. Zhang L, Zhuang Z, Zhang G, Huang T, Fan D. Assessment of bidirectional relationships between 98 genera of the human gut microbiota and amyotrophic lateral sclerosis: a 2-sample Mendelian randomization study. BMC Neurol. (2022) 22:8. doi: 10.1186/s12883-021-02522-z
83. Nezami BG, Srinivasan S. Enteric nervous system in the small intestine: pathophysiology and clinical implications. Curr Gastroenterol Rep. (2010) 12:358–65. doi: 10.1007/s11894-010-0129-9
84. Obrenovich M, Jaworski H, Tadimalla T, Mistry A, Sykes L, Perry G, et al. The role of the microbiota-gut-brain axis and antibiotics in ALS and neurodegenerative diseases. Microorganisms. (2020) 8:784. doi: 10.3390/microorganisms8050784
85. Chua JP, De Calbiac H, Kabashi E, Barmada SJ. Autophagy and ALS: mechanistic insights and therapeutic implications. Autophagy. (2022) 18:254–82. doi: 10.1080/15548627.2021.1926656
86. Crippa V, Boncoraglio A, Galbiati M, Aggarwal T, Rusmini P, Giorgetti E, et al. Differential autophagy power in the spinal cord and muscle of transgenic ALS mice. Front Cell Neurosci. (2013) 7:234. doi: 10.3389/fncel.2013.00234
87. Kamioka M, Goto Y, Nakamura K, Yokoi Y, Sugimoto R, Ohira S, et al. Intestinal commensal microbiota and cytokines regulate Fut2(+) Paneth cells for gut defense. Proc Natl Acad Sci U S A. (2022) 119. doi: 10.1073/pnas.2115230119
88. Sun J, Mu Y, Jiang Y, Song R, Yi J, Zhou J, et al. Inhibition of p70 S6 kinase activity by A77 1726 induces autophagy and enhances the degradation of superoxide dismutase 1 (SOD1) protein aggregates. Cell Death Dis. (2018) 9:407. doi: 10.1038/s41419-018-0441-0
89. Shi N, Li N, Duan X, Niu H. Interaction between the gut microbiome and mucosal immune system. Mil Med Res. (2017) 4:14. doi: 10.1186/s40779-017-0122-9
90. Rodrigues Lima-Junior J, Sulzer D, Lindestam Arlehamn CS, Sette A. The role of immune-mediated alterations and disorders in ALS disease. Hum Immunol. (2021) 82:155–61. doi: 10.1016/j.humimm.2021.01.017
91. Page MJ, Kell DB, Pretorius E. The role of lipopolysaccharide-induced cell signalling in chronic inflammation. Chronic Stress. (2022) 6:24705470221076390. doi: 10.1177/24705470221076390
92. Kar F, Hacioglu C, Kar E, Donmez DB, Kanbak G. Probiotics ameliorates LPS induced neuroinflammation injury on Aβ 1-42, APP, γ-β secretase and BDNF levels in maternal gut microbiota and fetal neurodevelopment processes. Metab Brain Dis. (2022) 37:1387–99. doi: 10.1007/s11011-022-00964-z
93. Mohr AE, Crawford M, Jasbi P, Fessler S, Sweazea KL. Lipopolysaccharide and the gut microbiota: considering structural variation. FEBS Lett. (2022) 596:849–75. doi: 10.1002/1873-3468.14328
94. Tinkov AA, Martins AC, Avila DS, Gritsenko VA, Skalny AV, Santamaria A, et al. Gut microbiota as a potential player in mn-induced neurotoxicity. Biomolecules. (2021) 11:1292. doi: 10.3390/biom11091292
95. Donohoe DR, Collins LB, Wali A, Bigler R, Sun W, Bultman SJ, et al. The Warburg effect dictates the mechanism of butyrate-mediated histone acetylation and cell proliferation. Mol Cell. (2012) 48:612–26. doi: 10.1016/j.molcel.2012.08.033
96. Gomes C, Cunha C, Nascimento F, Ribeiro JA, Vaz AR, Brites D, et al. Cortical neurotoxic astrocytes with early ALS pathology and miR-146a deficit replicate gliosis markers of symptomatic SOD1G93A mouse model. Mol Neurobiol. (2019) 56:2137–58. doi: 10.1007/s12035-018-1220-8
97. Collins SM. A role for the gut microbiota in IBS. Nat Rev Gastroenterol Hepatol. (2014) 11:497–505. doi: 10.1038/nrgastro.2014.40
98. Sampson TR, Debelius JW, Thron T, Janssen S, Shastri GG, Ilhan ZE, et al. Gut microbiota regulate motor deficits and neuroinflammation in a model of Parkinson's disease. Cell. (2016) 167:1469–80.e12. doi: 10.1016/j.cell.2016.11.018
99. Stilling RM, Cryan JF. Host response: a trigger for neurodegeneration? Nat Microbiol. (2016) 1:16129. doi: 10.1038/nmicrobiol.2016.129
100. Wachsmuth HR, Weninger SN, Duca FA. Role of the gut-brain axis in energy and glucose metabolism. Exp Mol Med. (2022) 54:377–92. doi: 10.1038/s12276-021-00677-w
101. Akbari E, Asemi Z, Daneshvar Kakhaki R, Bahmani F, Kouchaki E, Tamtaji OR, et al. Effect of probiotic supplementation on cognitive function and metabolic status in Alzheimer's disease: a randomized, double-blind and controlled trial. Front Aging Neurosci. (2016) 8:256. doi: 10.3389/fnagi.2016.00256
102. Di Gioia D, Bozzi Cionci N, Baffoni L, Amoruso A, Pane M, Mogna L, et al. A prospective longitudinal study on the microbiota composition in amyotrophic lateral sclerosis. BMC Med. (2020) 18:153. doi: 10.1186/s12916-020-01607-9
103. Yang XD, Wang LK, Wu HY, Jiao L. Effects of prebiotic galacto-oligosaccharide on postoperative cognitive dysfunction and neuroinflammation through targeting of the gut-brain axis. BMC Anesthesiol. (2018) 18:177. doi: 10.1186/s12871-018-0642-1
104. Yang X, Yu D, Xue L, Li H, Du J. Probiotics modulate the microbiota-gut-brain axis and improve memory deficits in aged SAMP8 mice. Acta Pharm Sin B. (2020) 10:475–87. doi: 10.1016/j.apsb.2019.07.001
105. Haidry RJ, van Baar AC, Galvao Neto MP, Rajagopalan H, Caplan J, Levin PS, et al. Duodenal mucosal resurfacing: proof-of-concept, procedural development, and initial implementation in the clinical setting. Gastroint Endosc. (2019) 90:673–81.e2. doi: 10.1016/j.gie.2019.03.024
106. Włodarek D. Role of ketogenic diets in neurodegenerative diseases (Alzheimer's disease and Parkinson's disease). Nutrients. (2019) 11:169. doi: 10.3390/nu11010169
107. Goncharova PS, Davydova TK, Popova TE, Novitsky MA, Petrova MM, Gavrilyuk OA, et al. Nutrient effects on motor neurons and the risk of amyotrophic lateral sclerosis. Nutrients 13. (2021). doi: 10.3390/nu13113804
108. Chico L, Ienco EC, Bisordi C, Lo Gerfo A, Petrozzi L, Petrucci A, et al. Amyotrophic lateral sclerosis and oxidative stress: a double-blind therapeutic trial after curcumin supplementation. CNS Neurol Disord Drug Targets. (2018) 17:767–79. doi: 10.2174/1871527317666180720162029
109. Fitzgerald KC, O'Reilly ÉJ, Fondell E, Falcone GJ, McCullough ML, Park Y, et al. Intakes of vitamin C and carotenoids and risk of amyotrophic lateral sclerosis: pooled results from 5 cohort studies. Ann Neurol. (2013) 73:236–45. doi: 10.1002/ana.23820
110. Caruso G, Torrisi SA, Mogavero MP, Currenti W, Castellano S, Godos J, et al. Polyphenols and neuroprotection: therapeutic implications for cognitive decline. Pharmacol Therap. (2022) 232:108013. doi: 10.1016/j.pharmthera.2021.108013
111. Virmani A, Pinto L, Binienda Z, Ali S. Food nutrigenomics, and neurodegeneration–neuroprotection by what you eat! Mol Neurobiol. (2013) 48:353–62. doi: 10.1007/s12035-013-8498-3
112. Khoruts A, Sadowsky MJ. Understanding the mechanisms of faecal microbiota transplantation. Nat Rev Gastroenterol Hepatol. (2016) 13:508–16. doi: 10.1038/nrgastro.2016.98
113. Zhang C, Yan J, Xiao Y, Shen Y, Wang J, Ge W, et al. Inhibition of autophagic degradation process contributes to claudin-2 expression increase and epithelial tight junction dysfunction in TNF-α treated cell monolayers. Int J Mol Sci. (2017) 18:157. doi: 10.3390/ijms18010157
114. Lu G, Wen Q, Cui B, Li Q, Zhang F. Washed microbiota transplantation stopped the deterioration of amyotrophic lateral sclerosis: the first case report and narrative review. J Biomed Res. (2022) 37:69–76. doi: 10.7555/JBR.36.20220088
115. Untangled No ALS. 21: fecal transplants. Amyotroph Lateral Scler Frontotemporal Degener. (2013) 14:482–5. doi: 10.3109/21678421.2013.814981
116. Smaga I, Fierro D, Mesa J, Filip M, Knackstedt LA. Molecular changes evoked by the beta-lactam antibiotic ceftriaxone across rodent models of substance use disorder and neurological disease. Neurosci Biobehav Rev. (2020) 115:116–30. doi: 10.1016/j.neubiorev.2020.05.016
117. Santa-Cecília FV, Leite CA, Del-Bel E, Raisman-Vozari R. The neuroprotective effect of doxycycline on neurodegenerative diseases. Neurotox Res. (2019) 35:981–6. doi: 10.1007/s12640-019-00015-z
118. Reglodi D, Renaud J, Tamas A, Tizabi Y, Socías SB, Del-Bel E, et al. Novel tactics for neuroprotection in Parkinson's disease: role of antibiotics, polyphenols and neuropeptides. Prog Neurobiol. (2017) 155:120–48. doi: 10.1016/j.pneurobio.2015.10.004
119. Tikka T, Usenius T, Tenhunen M, Keinänen R, Koistinaho J. Tetracycline derivatives and ceftriaxone, a cephalosporin antibiotic, protect neurons against apoptosis induced by ionizing radiation. J Neurochem. (2001) 78:1409–14. doi: 10.1046/j.1471-4159.2001.00543.x
120. Corsini S, Tortora M, Nistri A. Nicotinic receptor activation contrasts pathophysiological bursting and neurodegeneration evoked by glutamate uptake block on rat hypoglossal motoneurons. J Physiol. (2016) 594:6777–98. doi: 10.1113/JP272591
121. Wang CH, Hsieh YH, Powers ZM, Kao CY. Defeating antibiotic-resistant bacteria: exploring alternative therapies for a postantibiotic era. Int J Mol Sci. (2020) 21:1061. doi: 10.3390/ijms21031061
122. Albillos A, de Gottardi A, Rescigno M. The gut-liver axis in liver disease: pathophysiological basis for therapy. J Hepatol. (2020) 72:558–77. doi: 10.1016/j.jhep.2019.10.003
123. Ghadge GD, Kay BK, Drigotas C, Roos RP. Single chain variable fragment antibodies directed against SOD1 ameliorate disease in mutant SOD1 transgenic mice. Neurobiol Dis. (2019) 121:131–7. doi: 10.1016/j.nbd.2018.08.021
124. Federici S, Kredo-Russo S, Valdés-Mas R, Kviatcovsky D, Weinstock E, Matiuhin Y, et al. Targeted suppression of human IBD-associated gut microbiota commensals by phage consortia for treatment of intestinal inflammation. Cell. (2022) 185:2879–98.e24. doi: 10.1016/j.cell.2022.07.003
Keywords: gut microbiota, gut-brain axis (GBA), intestinal barrier, autophagy, probiotics
Citation: Hong D, Zhang C, Wu W, Lu X and Zhang L (2023) Modulation of the gut–brain axis via the gut microbiota: a new era in treatment of amyotrophic lateral sclerosis. Front. Neurol. 14:1133546. doi: 10.3389/fneur.2023.1133546
Received: 29 December 2022; Accepted: 17 March 2023;
Published: 20 April 2023.
Edited by:
Hideyuki Takeuchi, Yokohama City University, JapanReviewed by:
Teleky Bernadette-Emoke, University of Agricultural Sciences and Veterinary Medicine of Cluj-Napoca, RomaniaCopyright © 2023 Hong, Zhang, Wu, Lu and Zhang. This is an open-access article distributed under the terms of the Creative Commons Attribution License (CC BY). The use, distribution or reproduction in other forums is permitted, provided the original author(s) and the copyright owner(s) are credited and that the original publication in this journal is cited, in accordance with accepted academic practice. No use, distribution or reproduction is permitted which does not comply with these terms.
*Correspondence: Liping Zhang, emxwaG14QDE2My5jb20=
†These authors have contributed equally to this work
Disclaimer: All claims expressed in this article are solely those of the authors and do not necessarily represent those of their affiliated organizations, or those of the publisher, the editors and the reviewers. Any product that may be evaluated in this article or claim that may be made by its manufacturer is not guaranteed or endorsed by the publisher.
Research integrity at Frontiers
Learn more about the work of our research integrity team to safeguard the quality of each article we publish.