- 1Division of Oncology, Department of Medicine, Siteman Cancer Center, Washington University in Saint Louis, St. Louis, MO, United States
- 2Department of Neurology, Washington University in Saint Louis, St. Louis, MO, United States
Chimeric antigen receptor (CAR)-T cell therapy has revolutionized the management of several life-threatening malignancies, often achieving durable sustained responses. The number of patients treated with this new class of cell-based therapy, along with the number of Food and Drug Association (FDA) approved indications, are growing significantly. Unfortunately Immune Effector Cell-Associated Neurotoxicity Syndrome (ICANS) can often occur after treatment with CAR-T cells, and severe ICANS can be associated with significant morbidity and mortality. Current standard treatments are mainly steroids and supportive care, highlighting the need for early identification. In the last several years, a range of predictive biomarkers have been proposed to distinguish patients at increased risk for developing ICANS. In this review, we discuss a systematic framework to organize potential predictive biomarkers that builds on our current understanding of ICANS.
Introduction
Development of cellular therapies has expanded rapidly since the FDA approval of the first commercial cellular therapy, tisagenlecleucel, in 2017. As of the time of this review, there are six FDA-approved products with nearly 3,000 clinical trials focused on experimental cellular therapies under active investigation, representing a 50% growth from the prior year (1). Of these, T cell based therapies remain the most common (52%) with a majority targeting CD19 (56%). The number of patients treated with cellular therapies also continues to increase with approximately 5,000 annually receiving CAR-T in the US (1). Unfortunately, between 15 and 65% of treated patients developed Immune Effector Cell-Associated Neurotoxicity Syndrome (ICANS) in the original randomized control trials (RTC) for the six approved agents (Table 1), and upwards of 55 to 69% has been reported in large real-world cohorts (16, 17).
Neurologic symptoms associated with ICANS includes mild confusion, inattention, impaired reading, disorientation, word-finding difficulty, delirium, and impaired consciousness. This wide range of symptoms can be clustered using American Society for Transplantation and Cellular Therapy (ASTCT) consensus grading (18). Low grade (grade 1–2) ICANS is characterized by mild loss of orientation, naming, commands, writing, or attention as assessed using the Immune-Effector Cell-Associated Encephalopathy (ICE) Tool (18). Most cases of grade 1 or 2 ICANS are self-limited and resolve with supportive care. The further presence of focal neurological weakness, seizure, depressed consciousness, define grade 3 ICANS and represent a medical emergency given the risk of rapid progression to grade 4 with associated coma, recurrent or prolonged seizures, or cerebral edema (18). Diffuse grade 4 cerebral edema remains a comparatively rare, but nonetheless a concerning risk for all agents (Table 1). Also notable are neurologic symptoms not included in current ICANS grading schema, including tremors, myoclonus, and intracranial hemorrhage. Early identification remains essential given the significant risk of morbidity and mortality associated with ICANS.
Treatment options remain limited; management is primarily through aggressive supportive care and high-dose steroids (19–21). As such, current management guidelines for ICANS stresses early identification by active monitoring and serial assessments. However, the optimal timing and dosing of steroids remains debated. Steroids are potent anti-inflammatory therapy that decrease immune cell proliferation, cytokine production, and cytotoxic activity, raising concern for impaired CAR-T efficacy. While some reports suggest no influence of steroids on CAR-T kinetics (22), other reports reveal cumulative dose and duration of steroids is associated with shorter progression-free and overall survival after cellular therapy (23). This has led to several ongoing early phase clinical trials aimed to identify targeted immunomodulatory or other steroid-sparing interventions that does not impair CAR-T cell efficacy. Given the significant risk associated with ICANS and limited treatment options to date, early identification remains essential.
ICANS in FDA-approved cellular therapies
ICANS was originally observed during phase I/II trials of all current FDA-approved cellular therapies. Incidence varied between different cellular therapies and even for the same agent when used for different indications (Table 1). The highest rates of neurotoxicity were observed in the original ZUMA trials for axicabtagene ciloleuce [axi-cel, 78% or 330/422 aggregated unique total patients, including 64% or 65/111 (ZUMA-1), 59% or 87/148 (ZUMA-5), and 60% or 102/170 (ZUMA-7)] and for brexucabtagene autoleuce [brexu-cel, 63% or 43/74 (ZUMA-2)]. Tisagenlecleucel (tisa-cel) by comparison had a lower reported incidence at 40% or 30/75 (ELIANA trial) while Idecabtagene vicleucel (ide-cel) and ciltacabtagene autoleucel (cilta-cel) had the lowest incidence of neurotoxicity. Symptom onset mainly ranged between 5 and 11 days, though earlier (median day 2) ICANS was reported for ide-cel (KarMMa trial).
Comparing between different agents suggests a relationship between the incidence of neurotoxicity, a given target, and the costimulatory domain used in a given agent. The highest rates are observed in CD19-directed agents using containing a CD28 co-stimulatory domain (e.g., axi-cel and brexu-cel), with lower rates observes for CD19-directed agents using containing a 4-1BB co-stimulatory domain (tisa-cel and liso-cel). Ide-cel and cilta-cel both target B cell maturation antigen (BCMA) and also use a 4-1BB costimulatory domain. In contrast to their CD19-directed peers, ide-cel and cilta-cel have the lowest incidence of neurotoxicity (Table 1). While the selected agent remains a major risk factor for ICANS, it is only one of several factors associated with ICANS risk after cellular therapy.
Predictive biomarkers for adverse events after cellular therapy
Predictive biomarkers aid in early diagnosis, optimal apportioning of clinical resources, early intervention including prophylactic or preemptive treatment for high risk patients, and patient risk stratification. Early success was observed for the closely related adverse event known as cytokine release syndrome (CRS) with efficacious targeted interventions for CRS now available. CRS is an exaggerated systemic inflammatory response which manifests within the first 96 h after treatment in between 40 and 90% of all patients (Table 1). Fever is the hallmark symptom with severe cases associated with hypotension requiring vasopressors, hypoxia requiring mechanical support, and end-organ damage (18). Intimately connected to early cytokine elevations, targeted intervention has ameliorated significant morbidity and mortality associated with severe CRS. Since ICANS was first observed, a similar concerted effort has been devoted to identify potential predictive biomarkers. Unfortunately predictive biomarkers for ICANS remained elusive until only recently, with significant gaps in mechanistic understanding.
Challenges in identifying and validating predictive biomarkers in ICANS are rooted in several factors: First, small multi-center or single center studies have limited sample size, and studies often lack power to infer relationships in the subgroup of patients with ICANS. Second, the incidence rate of ICANS varies widely between studies and agents, making it difficult to draw conclusions between studies. Third, patients who develop ICANS often (but not always) also have ongoing CRS, making it difficult to disentangling a putative ICANS specific biomarker. Fourth, it is unclear if risk factors for ICANS are different between adult and pediatric patients, confounded generalizability between groups. Finally, until recently there are no animal models of ICANS to study its pathophysiology and mechanism outside of retrospective correlative clinical studies. In the following sections we will review all major predictive biomarkers associated with neurotoxicity after cellular therapy while keeping these challenges in mind.
Three major classes of predictive biomarker classes for ICANS
Predictive biomarkers for ICANS may be clustered in one of three major categories: (1) host factors which increase a given patient's risk for ICANS, (2) cellular therapy factors related to the cellular product, and (3) inflammatory factors relating to intersection of the first two categories, including most importantly CRS (Figure 1). The following sections will review reported predictive biomarkers of ICANS risk and discuss caveats with the interpretation and application of these biomarkers.
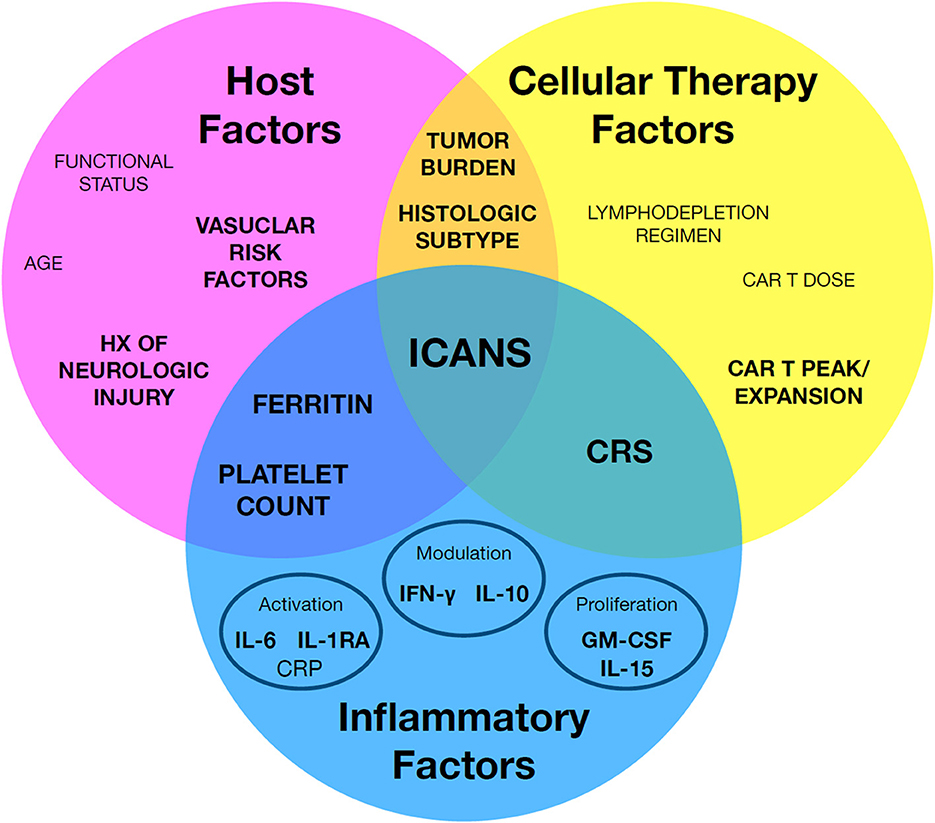
Figure 1. A framework to organize known immune effector cell-associated neurotoxicity syndrome (ICANS) risk factors. Individual factors may be broadly grouped by host factors, cellular therapy factors, and inflammatory factors. Notably, a subset of key factors bridge between classes, such as cytokine release syndrome (CRS). Factors with the strongest associated evidence are in highlighted in bold.
Host factors
Commonly reported host factors that can affect the development of ICANS include age, functional status, tumor burden, histologic subtype of tumor, neurologic comorbidities, and vascular risk factors.
Age
Age has been suggested as a risk factor for the development of ICANS (Table 2). However, evidence is conflicting and strongly biased by agent examined: The original ZUMA-1 clinical trial which lead to approval for axi-cel for DLBCL reported modest increased risk of neurologic events in patients ≥65 years old (n = 27, median age 69) vs. <65 years of age (n = 81, median age 55). Grade 3+ neurologic toxicities included delirium (11 vs. 0%), agitation (11 vs. 2%), and encephalopathy (30 vs. 22%), but interestingly not aphasia (0 vs. 10%) (50). Rubin et al. prospectively examined a cohort of 204 patients receiving axi-cel (median age 60 years) and found 58% developed neurotoxicity, with 43% developing grade 3+ neurotoxicity (39). Univariate regression revealed an association between age and ICANS (odds ratio (OR) 1.05). Once axi-cel was FDA approved for use, Jacobson et al. examined outcomes in a large international cohort of 1,297 patients who received the standard of care axi-cel, of which 739 (57%) would have been illegible for the original ZUMA-1 clinical trial (16) due to age or other ineglibility criteria. In the clinical practice, 55% of patients developed ICANS, with 24% developing grade 3+ ICANS. Multivariate analysis revealed a significant increased risk for patients aged 65 and older of developing any grade ICANS and grade 3+ ICANS with an odds ratio (OR) of 1.77 (95% CI, 1.39 to 2.26) and 1.38 (95% CI, 1.04–1.82) respectively. However, this relationship between older age and ICANS in patients treated with axi-cel has not been universally observed. Faramand and colleagues prospectively examined 75 patients (median age 63) treated with axi-cel and found no association with age. Similarly, Nastoupil and colleagues retrospectively analyzed results from the US Lymphoma CAR-T consortium totally 298 patients (median age 60, of whom, 275 were treated with axi-cel) and found no association with age and severe (Grade 3) neurotoxicity. No association with age has likewise been reported in smaller retrospective cohorts as well (40, 43, 48, 51). Like axi-cel, the investigational agent 19–28 z uses a CD28 co-stimulatory domain. Park et al. initially reported an association with age and ICANS in 51 adult patients (30), although this relationship was not observed in a second adult cohort of 51 patients (32). Overall, conflicting evidence exists for axi-cel and similar CD19-directed agents.
A relationship between age and ICANS was not observed for tisa-cel, either in the original trials (3) or later real-world cohorts (42, 43). Recently younger, not older age was reported associated with ICANS in a cohort of 15 patients (median age 59) treated with tisa-cel (44) (Table 2). Given the comparatively low incidence of ICANS for tisa-cel compared to axi-cel, statistical power is a limiting factor in drawing conclusions on the risk factors for ICANS development with tisa-cel. When examining an investigational CD19-directed CAR-T that also contains a 4-1BB costimulatory domain like tisa-cel, Gust et al. (28) also did not observe a significant relationship between neurotoxicity and age, but interestingly a trend for increased ICANS in younger, not older adult patients. Overall, age is an unclear risk factor with differences predominately related to agent. Larger studies are needed to understand the contributing factor of age to ICANS.
Functional status
Functional status, like age, is a risk factor reported predominately in studies that are heavily biased toward CD19-directed agents. It is measured using Eastern Cooperative Oncology Group performance status (ECOG PS), an established ordinal scale capturing a patient's level of medical independence and activity. An ECOG PS of 0 reflects a fully independent patient, while ECOG PS of 2 reflects an ambulatory patient who can carry out self-care but unable to work and up no more than 50% of waking hours. Faramand et al. (38) prospective study of patients treated with axi-cell did not observe an association between ECOG score and ICANS. In contrast, several large retrospective studies have demonstrated a positive association: Riedell and colleagues performed a retrospective multi-center study on 240 patients undergoing cellular therapy for a B cell lymphoma. They also observed ECOG PS ≥2 was associated with an increased risk of developing ICANS (p = 0.05, OR 5.05), even when controlling for treatment type i.e., axi-cel use. Jacobson and colleagues real-world cohort of 1,297 patients treated with axi-cel also observed a significant OR for baseline ECOG PS ≥2 and both any grade ICANS (OR 2.63; 95% CI, 1.40 to 4.93) and grade 3+ ICANS (3.23; 95% CI, 1.81 to 5.74) (16). Similarly, Iacaboni et al. examined 75 patients (median age 60) treated exclusively with tisa-cel in a retrospective Spanish cohort and found an association between ICANS and ECOG FS ≥1 (vs. 0) (42). Wudhikarn et al. only observed an increased likelihood of developing ICANS in patients with ECOG ≥2, but only in patients under the age of 60 (40). Gauthier and colleagues did not observe an association in their smaller retrospectively study (43). Taken together, ECOG PS remains a possible but weak factor mainly observed in larger cohorts with limited data beyond CD19-directed agents.
Tumor burden
Pre-infusion tumor burden has been reported as a risk factor for the development of neurotoxicity across several (17, 24, 28, 30, 32, 34) but not all studies (26, 36, 43, 44). Notably the methods used to measure tumor burden can vary widely from bone marrow assessments, to imaging-derived (including using positron emission tomography (PET), discussed below), to secondarily inferred from blood LDH levels (Table 3). As a result, tumor burden has been defined in different studies as a categorical variable (“high” vs. “low burden” based on marrow blasts or by the presence of secondary sites of disease), ordinal variable, and continuous variable highlighting the varied approaches used. To date, the association between tumor and risk for ICANS has been best characterized in adults with B cell lymphomas (17, 24, 28, 30, 32, 52) but not children (36). Studies reporting no association often limited by power (43, 44). Taken together, pre-infusion tumor burden is a possible risk factor, though standardization of measurement modality and larger studies comparing across agents is warranted.
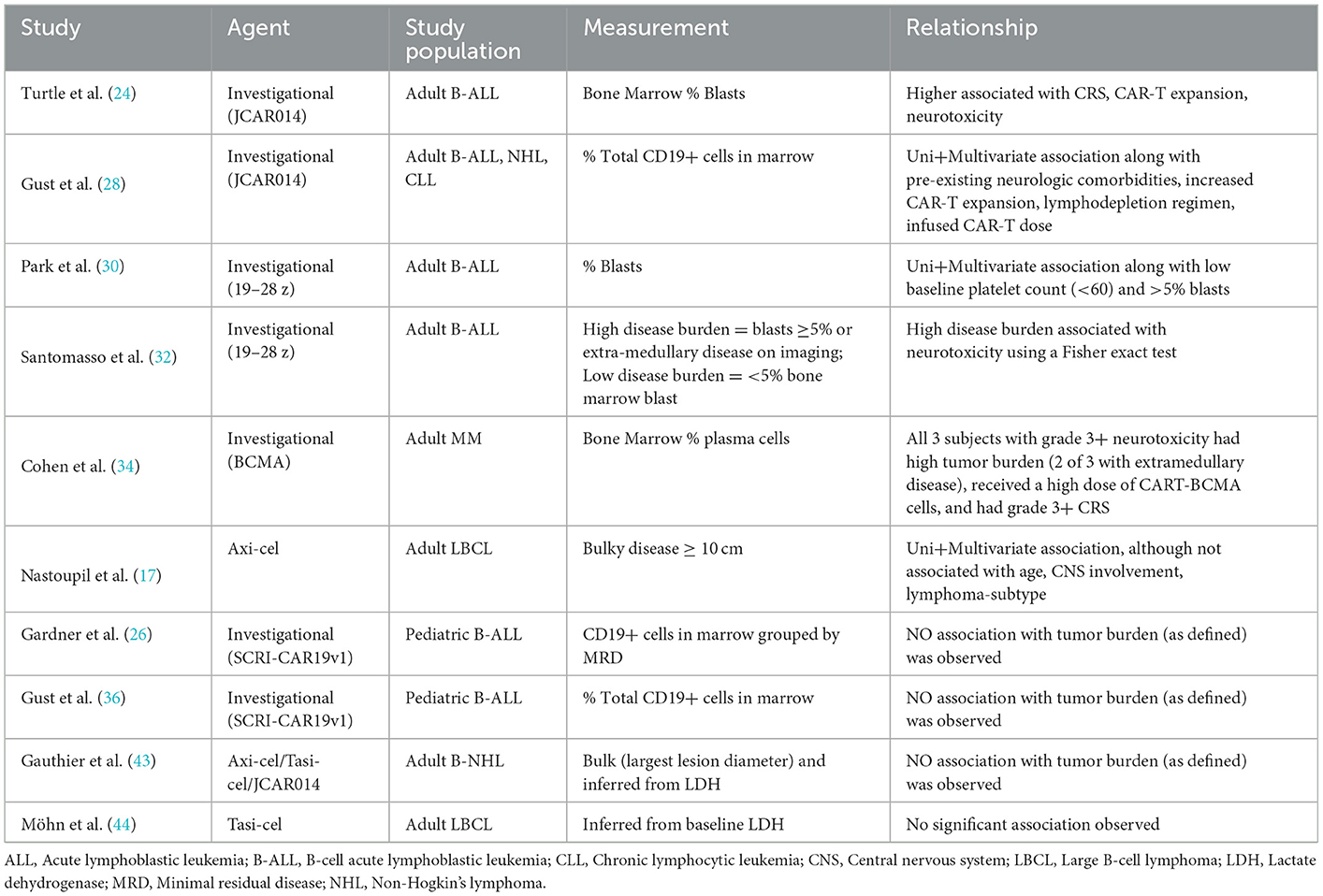
Table 3. Summary of studies reporting tumor burden including modality of measurement and reported relationship with neurotoxicity.
PET-derived assessment of total metabolic tumor volume (TMTV) has been proposed as a standardized means to quantify total active tumor volume and address some of the variability in tumor burden assessment highlighted above. TMTV is calculated from pre-treatment (i.e., baseline at recurrence) PET scans of patients with B cell lymphoma using a 41% maximum standardized uptake value threshold (53, 54). Unfortunately use of TMTV has had mixed to success to date (52, 54). Dean et al. retrospectively examined baseline PET scans of 96 patients treated with axi-cel and found no relationship between TMTV and ICANS (54), in line with earlier reports (55). Likewise, Iacoboni et al. retrospectively examined a cohort of 35 patients treated with an admixture of agents (breakdown not reported) also did not observe an association with ICANS risk (52). Nor did we see a relationship between TMTV and ICANS risk in our recent retrospective study of 30 patients (46). TMTV as a surrogate for tumor burden in B cell lymphomas remains of unclear utility at this time.
Histologic subtype of tumor
Until recently, only axi-cel's multiple indications permitted comparison of ICANS incidence between different histological subtypes while controlling for agent. The original ZUMA-1 [diffused large B cell lymphoma (DLBCL)], ZUMA-5 [follicular lymphoma (FL)], and most recently ZUMA-7 (2nd line for large B cell) clinical trials all reported comparable frequency of ICANS of approximately 60% (Table 1). However, the frequency of grade 3+ ICANS was approximately half for FL (ZUMA-5) vs. DLBCL (ZUMA-1). Rubin and colleagues prospective study of 204 patients categorically clustered by patient's histological subtype: aggressive (DLBL, primary mediastinal B-cell lymphoma) or indolent (follicular lymphoma, marginal zone lymphoma) histologic subtype (39). They found a univariate association between neurotoxicity and an aggressive histological subtype (p < 0.001), and included it in their multivariate prediction model. When comparing between different aggressive subtypes of lymphoma (DLBL, primary mediastinal B-cell lymphoma, transformed FL, germinal center B cell (GCB)-like, non-GCB, double/triple hit, or double expressor), no association was observed retrospectively in the US Lymphoma CAR-T consortium (17). With the recent approval of tisa-cel for follicular lymphoma, similar future comparisons will be possible [Table 1, (5)]. Taken together, histologic subtype is a possible factor, though confounding factors such as volume and extent of tumor burden, CAR-T expansion/peak, and selected agent make clear associations challenging to infer.
Vascular risk factors
The presence of microvascular and macrovascular insults, fluid attenuated inversion recovery (FLAIR) white matter lesions, and posterior reversible (leuko)encephalopathy syndrome (PRES) observed on imaging (56, 57) suggest an underlying vascular pathology may be at play in ICANS that leads to secondary central nervous system (CNS) injury after CRS and/or CAR-T expansion. Consistently reported endothelial risk factor for ICANS in adults are the agonist/antagonist pair of soluble vascular growth factors known as angiopoietin-1 (Ang-1) and angiopoietin-2 (Ang-2) supports this observation. Both Ang-1 and Ang-2 act on the tyrosine kinase receptor, Tie-2. Ang-1 stabilizes endothelium on binding to Tie-2, including closing inter-endothelial gaps (58). In contrast, high levels of Ang-2 inhibits Tie-2 activity resulting in increased endothelial permeability [(59); for review Saharinen et al. (60)]. Three studies which have examined levels of Ang-1, Ang-2, and the ratio of Ang-2/Ang-1 in axi-cel (38) and the investigational CD19-directed agents JCAR014 (28) and 19-28z (32) with generally congruent findings: Faramand et al. found baseline (pre-lymphodepletion) serum levels of Ang-2 (p = 0.0190) and Ang-2/Ang-1 ratio (p = 0.0056) were elevated in patients who developed grade 3+ neurotoxicity (F57). Among post-infusion factors, an elevated peak Ang-2/Ang-1 ratio (p = 0.0016) and depressed nadir Ang-1 level (p = 0.0298) were also associated with grade 3+ neurotoxicity (38). Gust and colleagues found a trend to increased baseline serum Ang-2/Ang-1 ratio (p = 0.09) and significant post-infusion peak Ang-2 (p = 0.0003), and Ang-2/Ang-1 ratio (p = 0.0014) elevations in patients with grade 4+ neurotoxicity, although no difference in nadir post-infusion Ang-1 was observed (p = 0.13) (28). Finally, Santomasso and colleagues also observed significant post-infusion day 7 Ang-2/Ang-1 ratio elevation (p = 0.0310), but not either day 7 Ang-2 elevation (p = 0.07) or day 7 Ang-1 depression (p = 0.053) in patients with grade 3+ neurotoxicity (32).
While the pre-treatment Ang-2/Ang-1 ratio may be a promising predictive biomarker for higher grade ICANS, there remain several caveats warranting examination. First, similar findings were not observed in pediatric populations receiving CD19-directed CAR-T agents, suggesting possible differences in pediatric vs. adult susceptibility factors (36). Second, other more non-specific plasma markers such as drops in platelet count and/or fibrinogen have had more varied success (Table 2). Third, data is limited for non-CD19 CAR-T cell agents. Finally, while suggestive of a link for vascular injury and subsequent ICANS risk, the mechanism remains unclear including the relationship of ICANS to blood brain barrier breakdown and systemic cytokine, myeloid, and/or CAR-T Infiltration into the CNS. Overall, Ang-1, Ang-2, and the ratio of Ang-2/Ang-1 remain a promising, if under-explored, risk factor for ICANS.
Neurologic comorbidities and injury
History of neurologic comorbidities or injury is another commonly reported risk factor for neurotoxicity (Table 4). Neurologic comorbidities may reflect prior CNS injury (e.g., seizures), peripheral nervous system (PNS) injury (e.g., neuropathy), CNS involvement of cancer (e.g., CNS lymphoma), or neurotoxic treatment exposure (e.g., CNS radiation, intrathecal methotrexate). Unfortunately, the definition of neurologic comorbidities varied significantly between studies. Gust and colleagues used an inclusive definition and found univariate (p = 0.006) and multivariate association (p = 0.002) when further controlling for tumor burden, increased CAR-T expansion, lymphodepletion regimen, and infused CAR-T dose in adults (28). A small recent adult cohort likewise clustered different sources of injury together and reported a possible univariate association (44). Gofshteyn and colleagues separately examined history of brain radiation, seizures, AED use, and pre-existing deficits in a pediatric cohort. Only pre-existing deficits (p = 0.01) was associated with encephalopathy, seizure, and aphasia, all key hallmarks of ICANS (31). Another small pediatric cohort observed 3 of 5 patients with history of pre-existing neurologic deficits developed ICANS (33). However, an association is not always observed in pediatric cohorts; Gust et al. did not observe a relationship when clustering different neurologic comorbidities except an abnormal MRI prior to treatment in a pediatric cohort (36). Overall, categorically-defined neurologic injury remains a possible risk factor, particularly in adults, though the underlying localization of injury remains unclear.
More recently neurofilament light chain (NfL) has been used to provide quantitative, rather than categorical, assessment of neural injury (Table 4). NfL is a structural component of long axons whose levels in cerebral spinal fluide (CSF) and blood increase after a neurological insult. Baseline elevations in NfL have been reported in two adult cohorts (45, 46), with elevations predating lymphodepletion. Levels further remain elevated throughout the course of treatment for up to 30 days after infusion (46). A similar relationship is not observed in a pediatric cohort, where global elevations were observed across all patients independent of ICANS risk (49). The disagreement between the adult (45, 46) and pediatric studies (49) may reflect different assay sensitivities or previous treatment dosing/regimens. Furthermore, like vascular injury markers listed above, the mechanistic link in ICANS between neuroaxonal injury, vascular injury, blood brain barrier breakdown, and CNS cytokine, myeloid, and/or CAR-T cross infiltration in ICANS remains unclear. While promising, further study is needed to account for possible confounding factors including neuropathy and systemic vascular injury unrelated to the blood brain barrier.
Cellular therapy factors
We previously discussed CD19-directed therapies, particularly those containing a CD28 costimulatory domain, have the highest incidence of ICANS (Table 1). This section summarizes additional cellular therapy-related risk factors known to be associated with neurotoxicity when controlling for the agent type. Additional risk factors include type/dose of lymphodepletion condition regimen, CAR T dose and expansion, are reviewed as follows:
Lymphodepletion
Cyclophosphamide and fludarabine containing regimens are commonly used for lymphodepletion prior to CAR-T cell infusion. The dose intensity of cyclophosphamide and addition of fludarabine are associated with improved CAR-T kinetics, expansion, and response in both pediatric and adult patients treated with CD19-directed agents (24–26, 35). Early studies demonstrated an association between the lymphodepletion regimen and ICANS in both univariate and multi-variate modeling (24, 25, 28). Notably all three of these studies all examined the same experimental agent, JCAR014. Unfortunately this relationship is not universally seen across CD19-directed agents and was not observed for investigational agents, including SCRI-CAR19v1 (26, 36) or 19–28 z (30, 35). Indeed a recent retrospective study of 152 patients treated with tisa-cel also revealed no association between low (termed “suboptimal” <13.8 mgxh/L) fludarabine exposure and ICANS (61). This variability may stem from a secondary relationship with neurotoxicity, where better lymphodepletion results in improved expansion and peak CAR-T levels, which in turn is associated with neurotoxicity. Fludarabine exposure is also known to cause a rare but separate neurotoxicity from ICANS, as was observed in the ZUMA-3 trial (10). Therefore, in select cases, misdiagnosis of neurotoxicity related to fludarabine may be another source of variability that confounds the clinical assessment for ICANS. Rates of ICANS in bendamustine and other fludarabine-free lymphodepletion regimes warrant further investigation. Overall, standardization of lymphodepletion regimens and monitoring/consideration for Flu-associated neurotoxicity remains key for risk modulation.
Dose of CAR T and expansion
Higher doses of infused CAR-T cells has been associated with increased risk for CRS and neurotoxicity, particularly in patients with high tumor burden [Tisa-cel: (42, 44); JCAR014: (24, 25, 28)]. This association was not observed for other CD19-directed agents such as the investigational 19–28 z (30, 35) or SCRI-CAR19v1 (26, 36). Overall CAR-T dose remains an unclear risk factor, particularly given the standardization of target dosing per patient weight.
In contrast to dose, the degree of expansion remains a consistent risk for the development of ICANS across multiple studies and agents (Table 2). Following infusion, CAR-T cells redistribute and proliferate rapidly, with the early expansion coupled to cytokine production and CRS risk. The duration of expansion varies, with CAR-T targeting leukemia and multiple myeloma associated with a slightly longer duration than those targeting lymphoma [for detailed comparison of kinetics, see Liu et al. (62)]. The association between expansion and ICANS risk was first observed in the original trials leading to approval of axi-cel (6, 7, 10), brexu-cel (9), liso-cel (A69), and several investigational CAR-T products including JCAR014 (24, 25, 28), 19–28 z (30, 32), SCRI-CAR19v1 (36). Of note 41 who examined 19–28 z in a pediatric cohort did not observe a relationship between neurotoxicity and expansion (p = 0.14), though this may reflect a small sample size (n = 25). Overall, rate of expansion and maximum CAR-T expansion capability remains a key risk factor for the development of neurotoxicity.
Inflammatory factors
Development and severity of CRS remains a key risk factor for ICANS (Table 2). Consorted effort has been devoted to understand the temporal cascade of systemic and when available, CSF inflammatory cascade after treatment with a given agent (21, 63). Here we highlight the two of the most commonly reported marker in each class using a standardized grouping before focusing in particular on pre-treatment risk factors.
Pro-(inflammatory) activation markers: IL-1 and IL-6
Post-treatment peak IL-6 elevations remain tightly coupled with the development of ICANS across multiple prospective and retrospective cohorts (Table 2). This includes the seminal trials leading the to the approval of axi-cel (6, 7, 10) and brexu-cel (9), but interestingly not tisa-cel (45). Elevations in IL-6 are a known hallmark of CRS. Given the high degree of association between CRS and ICANS, further correlation between IL-6 and ICANS is unsurprising though it remains unclear which level of contribution they provide to ICANS independently and specifically.
Along with IL-6, the IL-1 signaling pathway is considered a key mediator in CRS. Preclinical models (intraperitoneal Raji tumor cells injected SCID-beige subsequently treated with human 1928 z CAR T cells) demonstrated a tight coupling between the IL-1 signaling cascade and severe CRS through macrophage/monocyte activity (64). Of the two major agonist IL-1 ligands IL-1α and IL-1β, IL-1β is the predominately macrophage and monocyte activator with secondary effects on non-immune cells. Currently, the role of IL-1 and associated monocyte activation beyond CRS in neurotoxicity remains under active investigation. In preclinical studies, SGM3 mice treated with human CD19 CAR T cells demonstrated seizures and paralysis may be abrogated through IL-1 but not IL-6 blockade (65). This has led to active clinical investigation of IL-1 receptor antagonism using anakinra to intervene on the development of CRS and ICANS.
Pro-proliferation markers: IL-15 and GM-CSF
Along with IL-6, IL-15, which promotes T and NK cell proliferation and activation, has been observed to be consistently elevated in individuals who develop ICANS (6, 7, 25, 28–33). The myeloid proliferation and activation cytokine GM-CSF is likewise a commonly observed blood marker whose peak levels after treatment are associated with ICANS in several retrospective and prospective studies (6, 7, 9, 30, 32, 33).
Immuno-modulators: IL-10 and IFN-γ
Both IL-10 and IFN-γ are immune-modulators, with IL-10 traditionally thought of as an immunosuppressive regulator that decreases cytokine production and antigen presentation by antigen-presenting cells (66–68). However, high levels of IL-10 have been associated with IFN-γ-mediated CD8(+) T cell cytotoxicity (68, 69). Given known marked elevations in acute ICANS of IFN-γ, this second pathway paradoxical coupling to IFN-γ elevations is possible mechanism warranting further study.
Baseline systemic factors
A subset of studies have examined baseline blood markers in 19–28 z (32) and axi-cel (7, 10, 38, 46). Santomasso et al. found baseline elevations in IL-6, IL-10, GM-CSF, and G-CSF, but not ferritin, in patients treated with 19–28 z who went on to develop ICANS. Faramand et al. (38) also observed baseline elevations in IL-6 in patients treated with Axi-cel who went on to develop ICANS. In contrast to Santomasso et al., baseline ferritin elevations were observed in this study and in more recent studies (7, 38, 46). Overall, baseline IL-6 and ferritin elevations are a possible risk factor, though data is limited beyond a subset of CD19-directed agents.
Implications and future directions
Predictive biomarkers hold promise for the early, reliable, and rapid identification of patients most at risk for ICANS, despite ongoing limitations. While the mechanism underlying ICANS remains unclear, this review highlights the tight association between ICANS risk and key inflammatory, cellular therapy, and host factors in patients undergoing cellular therapy (Figure 1). Rubin and colleagues demonstrated the promise of a combined risk model for ICANS that assembles markers across each of the three major categories (39). Amidi et al. (70) recently expanded on this approach. Notably, neither study integrated vascular factors, neurologic injury, key cytokines, or type of cellular therapy agent in their respective models, holding promise these further additions may improve generalizability. Ongoing multi-omic approaches to identify new biomarkers (71), efforts to pool multi-center data as validation cohorts, and advances in preclinical mechanistic studies hold promise for a validated model that hones in on key pathologic pathways in the near future.
Author contributions
OB: study concept, visualization, and writing—original draft. AZ: writing—original draft. BA: project administration, supervision, and critical review. JD: supervision and critical review. AG: study concept, project administration, supervision, and critical review. All authors contributed to the article and approved the submitted version.
Conflict of interest
OB, BA, JD, and AG: Provisional New- Use Patent on use of blood NfL to predict ICANS.
The authors declare that the research was conducted in the absence of any commercial or financial relationships that could be construed as a potential conflict of interest.
Publisher's note
All claims expressed in this article are solely those of the authors and do not necessarily represent those of their affiliated organizations, or those of the publisher, the editors and the reviewers. Any product that may be evaluated in this article, or claim that may be made by its manufacturer, is not guaranteed or endorsed by the publisher.
References
1. Saez-Ibañez AR, Upadhaya S, Partridge T, Shah M, Correa D, Campbell J, et al. Landscape of cancer cell therapies: trends and real-world data. Nat Rev Drug Discov. (2022) 21:631–2. doi: 10.1038/d41573-022-00095-1'
2. Maude SL, Laetsch TW, Buechner J, Rives S, Boyer M, Bittencourt H, et al. Tisagenlecleucel in children and young adults with B-cell lymphoblastic leukemia. N Engl J Med. (2018) 378:439–48. doi: 10.1056/NEJMoa1709866
3. Schuster SJ, Bishop MR, Tam CS, Waller EK, Borchmann P, McGuirk JP, et al. JULIET, investigatorstisagenlecleucel in adult relapsed or refractory diffuse large B-cell lymphoma. N Engl J Med. (2019) 380:45–56. doi: 10.1056/NEJMoa1804980
4. Schuster SJ, Tam CS, Borchmann P, Worel N, McGuirk JP, Holte H, et al. Long-term clinical outcomes of tisagenlecleucel in patients with relapsed or refractory aggressive B-cell lymphomas (JULIET): a multicentre open-label single-arm phase 2 study. Lancet Oncol. (2021) 22:1403–15. doi: 10.1016/S1470-2045.21.00375-2
5. Fowler NH, Dickinson M, Dreyling M, Martinez-Lopez J, Kolstad A, Butler J, et al. Tisagenlecleucel in adult relapsed or refractory follicular lymphoma: the phase 2 ELARA trial. Nat Med. (2022) 28:325–32. doi: 10.1038/s41591-021-01622-0
6. Neelapu SS, Locke FL, Bartlett NL, Lekakis LJ, Miklos DB, Jacobson CA, et al. Axicabtagene ciloleucel CAR T-cell therapy in refractory large B-cell lymphoma. N Engl J Med. (2017) 377:2531–44. doi: 10.1056/NEJMoa1707447
7. Jacobson CA, Chavez JC, Sehgal AR, William BM, Munoz J, Salles G, et al. Axicabtagene ciloleucel in relapsed or refractory indolent non-Hodgkin lymphoma. (ZUMA-5): a single-arm multicentre phase 2 trial. Lancet Oncol. (2022) 23:91–103. doi: 10.1016/S1470-204500591-X
8. Locke FL, Miklos DB, Jacobson CA, Perales MA, Kersten MJ, Oluwole OO, et al. All ZUMA 7 investigators and contributing kite members, axicabtagene ciloleucel as second-line therapy for large B-cell lymphoma. N Engl J Med. (2022) 386:640–654. doi: 10.1056/NEJMoa2116133
9. Wang M, Munoz J, Goy A, Locke FL, Jacobson CA, Hill BT, et al. MKTE-X19 CART and cell therapy in relapsed or refractory mantle-cell lymphoma. N Engl J Med. (2020) 382:1331–42. doi: 10.1056/NEJMoa1914347
10. Shah BD, Ghobadi A, Oluwole OO, Logan AC, Boissel N, Cassaday RD, et al. RKTE.-,X19 for relapsed or refractory adult B-cell acute lymphoblastic leukaemia: phase 2 results of the single-arm open-label multicentre ZUMA-3 study. Lancet. (2021) 398:491–502. doi: 10.1016/S0140-6736.21.01222-8
11. Abramson JS, Palomba ML, Gordon LI, Lunning MA, Wang M, Arnason J, et al. Lisocabtagene maraleucel for patients with relapsed or refractory large B-cell lymphomas. (TRANSCEND NHL 001): a multicentre seamless design study. Lancet. (2020) 396:839–52. doi: 10.1016/S0140-6736.20.31366-0
12. Kamdar M Solomon SR, Arnason J, Johnston PB, Glass B, Bachanova V, et al. InvestigatorsLisocabtagene maraleucel versus standard of care with salvage chemotherapy followed by autologous stem cell transplantation as second-line treatment in patients with relapsed or refractory large B-cell lymphoma. (TRANSFORM): results from an interim analysis of an open-label randomised phase 3 trial. Lancet. (2022) 399:2294–308. doi: 10.1016/S0140-6736.22.00662-6
13. Munshi NC, Anderson LD Jr, Shah N, Madduri D, Berdeja J, Lonial S, et al. Idecabtagene vicleucel in relapsed and refractory multiple myeloma. N Engl J Med. (2021) 384:705–16. doi: 10.1056/NEJMoa2024850
14. Bristol Myers Squibb and 2seventy bio Announce Topline Results from KarMMa-3 Trial Showing Abecma. (idecabtagene vicleucel). Significantly Improves Progression-Free Survival Versus Standard Regimens in Relapsed and Refractory Multiple MyelomaPress Release. Available online at: https://news.bms.com/news/details/2022/Bristol-Myers-Squibb-and-2seventy-bio-Announce-Topline-Results-from-KarMMa-3-Trial-Showing-Abecma-idecabtagene-vicleucel-Significantly-Improves-Progression-Free-Survival-Versus-Standard-Regimens-in-Relapsed-and-Refractory-Multiple-Myeloma/default.aspx (accessed 11 December, 2022).
15. Berdeja JG, Madduri D, Usmani SZ, Jakubowiak A, Agha M, Cohen AD, et al. SCiltacabtagene autoleucel a B-cell maturation antigen-directed chimeric antigen receptor T-cell therapy in patients with relapsed or refractory multiple myeloma (CARTITUDE-1): a phase 1b/2 open-label study. Lancet. (2021) 398:314–24. doi: 10.1016/S0140-673600933-8
16. Jacobson CA, Locke FL, Ma L, Asubonteng J, Hu ZH, Siddiqi T, et al. Real-world evidence of axicabtagene ciloleucel for the treatment of large B cell lymphoma in the United States. Transplant Cell Ther. (2022) 28:581. doi: 10.1016/j.jtct.0026
17. Nastoupil LJ, Jain MD, Feng L, Spiegel JY, Ghobadi A, Lin Y, et al. Standard-of-care axicabtagene ciloleucel for relapsed or refractory large B-cell lymphoma: results from the US lymphoma CAR T consortium. J Clin Oncol. (2020) 38:3119–28. doi: 10.1200/JCO.19.02104
18. Lee DW, Santomasso BD, Locke FL, Ghobadi A, Turtle CJ, Brudno JN, et al. Consensus grading for cytokine release s.yndrome and neurologic toxicity associated with immune effector. Cells Biol Blood Marrow Transplant. (2019) 25:625–38. doi: 10.1016/j.bbmt.12 758.
19. Park JH, Rivière I, Gonen M, Wang X, Sénéchal B, Curran KJ, et al. Long-term follow-up of CD.19 CAR, therapy in acute lymphoblastic leukemia. N Engl J Med. (2018) 378:449–59. doi: 10.1056/NEJMoa1709919
20. Gust J, Taraseviciute A, Turtle CJ. Neurotoxicity Associated with CD19-targeted CAR-T cell therapies. CNS Drugs. (2018) 32 1091–101. doi: 10.1007/s40263-018-0582-9
21. Gust J, Ponce R, Liles WC, Garden GA, Turtle CJ. Cytokines in CART, Cell-associated neurotoxicity. Front Immunol. (2020) 11:577027. doi: 10.3389/fimmu.2020.577027
22. Liu S, Deng B, Yin Z, Pan J, Lin Y, Ling Z, et al. Corticosteroids do not influence the efficacy and kinetics of CAR-T cells for B-cell acute lymphoblastic leukemia. J Blood Cancer. (2020) 10:15. doi: 10.1038/s41408-020-0280-y
23. Strati P, Ahmed S, Furqan F, Fayad LE, Lee HJ, Iyer SP, et al. Prognostic impact of corticosteroids on efficacy of chimeric antigen receptor T-cell therapy in large B-cell lymphoma. Blood. (2021) 137:3272–6. doi: 10.1182/blood.2020008865
24. Turtle CJ, Hanafi LA, Berger C, Gooley TA, Cherian S, Hudecek M, et al. CD19 CAR- cells of defined CD4+:CD8+ composition in adult B cell ALL patients. J Clin Invest. (2016) 126:2123–38. doi: 10.1172/JCI85309
25. Turtle CJ, Hanafi LA, Berger C, Hudecek M, Pender B, Robinson E, et al. Immunotherapy of non-Hodgkin's lymphoma with a defined ratio of CD8+ and CD4+ CD19-specific chimeric antigen receptor-modified T cells. Sci Transl Med. (2016) 8:355ra. doi: 10.1126/scitranslmed.aaf8621
26. Gardner RA, Finney O, Annesley C, Brakke H, Summers C, Leger K, et al. Intent-to-treat leukemia remission by CD19 CAR T cells of defined formulation and dose in children and young adults. Blood. (2017) 129:3322–31. doi: 10.1182/blood-2017-02-769208
27. Turtle CJ, Hay KA, Hanafi LA Li D, Cherian S, Chen X, et al. Durable molecular remissions in chronic lymphocytic leukemia treated with CD19-specific chimeric antigen receptor-modified T cells after failure of ibrutinib. J Clin Oncol. (2017) 35:3010–20. doi: 10.1200/JCO.728519
28. Gust J, Hay KA, Hanafi LA, Li D, Myerson D, Gonzalez-Cuyar LF, et al. Endothelial activation and blood-brain barrier disruption in neurotoxicity after adoptive immunotherapy with CD19 CAR-T cells. Cancer Discov. (2017) 7:1404–19. doi: 10.1158/2159-8290.CD-17-0698
29. Kochenderfer JN, Somerville RPT, Lu T, Shi V, Bot A, Rossi J, et al. Lymphoma remissions caused by anti-CD19 chimeric antigen receptor T, cells are associated with high serum interleukin-15 levels. J Clin Oncol. (2017) 35:1803–13. doi: 10.1200/JCO.2016.71.3024.
30. Park JH, Santomasso B, Riviere I, Senechal B, Wang X, Purdon T, et al. Baseline and early post-treatment clinical and laboratory factors associated with severe neurotoxicity following 19–28z CAR T cells in adult patients with relapsed B ALL. J Clin Oncol. (2017) 35:7024. doi: 10.1200/JCO.2017.35.15_suppl.7024
31. Gofshteyn JS, Shaw PA, Teachey DT, Grupp SA, Maude S, Banwell B, et al. Neurotoxicity after CTL019 in a pediatric and young adult cohort. Ann Neurol. (2018) 84:537–46. doi: 10.1002/ana.25315
32. Santomasso BD, Park JH, Salloum D, Riviere I, Flynn J, Mead E, et al. Clinical and biological correlates of neurotoxicity associated with CAR T-cell therapy in patients with B-cell acute lymphoblastic leukemia. Cancer Discov. (2018) 8:958–71. doi: 10.1158/2159-8290.CD-17-1319
33. Shalabi H, Wolters PL, Martin S, Toledo-Tamula MA, Roderick MC, Struemph K, et al. Systematic evaluation of neurotoxicity in children and young adults undergoing CD22 chimeric antigen receptor T-cell therapy. J Immunother. (2018) 41:350–358. doi: 10.1097/CJI.0000000000000241
34. Cohen AD, Garfall AL, Stadtmauer EA, Melenhorst JJ, Lacey SF, Lancaster E, et al. B, cell maturation antigen-specific CAR T cells are clinically active in multiple myeloma. J Clin Invest. (2019) 129:2210–21. doi: 10.1172/JCI126397
35. Curran KJ, Margossian SP, Kernan NA, Silverman LB, Williams DA, Shukla N, et al. Toxicity and response after CD19-specific CAR, T-cell therapy in pediatric/young adult relapsed/refractory ball. Blood. (2019) 134:2361–2368. doi: 10.1182/blood
36. Gust J, Finney OC, Li D, Brakke HM, Hicks RM, Futrell RB, et al. Glial injury in neurotoxicity after pediatric CD19-directed chimeric antigen receptor T cell therapy. Ann Neurol. (2019) 86:42–54. doi: 10.1002/ana.25502
37. Karschnia P, Jordan JT, Forst DA, Arrillaga-Romany IC, Batchelor TT, Baehring JM, et al. Clinical presentation management and biomarkers of neurotoxicity after adoptive immunotherapy with CAR T cells. Blood. (2019) 133:2212–21. doi: 10.1182/blood-2018-12-893396
38. Faramand R, Jain M, Staedtke V, Kotani H, Bai R, Reid K. Tumor microenvironment composition and severe cytokine release syndrome. (CRS) influence toxicity in patients with large B-cell lymphoma treated with axicabtagene ciloleucel. Clin Cancer Res. (2020) 26:4823–31. doi: 10.1158/1078-0432.CCR-20-1434
39. Rubin DB, Al Jarrah A, Li K, LaRose S, Monk AD, Ali AB, et al. Clinical predictors of neurotoxicity after chimeric antigen receptor T-cell therapy. JAMA Neurol. (2020) 77:1536–42. doi: 10.1001/jamaneurol.2020.2703
40. Wudhikarn K, Bansal R, Khurana A, Hathcock MA, Braksick SA, Bennani NN, et al. Age defining immune effector cell associated neurotoxicity syndromes in aggressive large B, cell lymphoma patients treated with axicabtagene ciloleucel. Am J Hematol. (2021) 96:E427–30. doi: 10.1002/ajh.26330
41. Riedell PA, Hwang WT, Nastoupil LJ, Pennisi M, McGuirk JP, Maziarz RT, et al. Patterns of use outcomes and resource utilization among recipients of commercial axicabtagene ciloleucel and tisagenlecleucel for relapsed/refractory aggressive B, cell lymphomas. Transplant Cell Ther. (2022) 28:669–76. doi: 10.1016/j.jtct.07011
42. Iacoboni G, Villacampa G, Martinez-Cibrian N, Bailén R, Lopez Corral L, Sanchez JM, et al. Barba PGETH, GELTAMO, Spanish groups, Real-world evidence of tisagenlecleucel for the treatment of relapsed or refractory large B-cell lymphoma. Cancer Med. (2021) 10:3214–23. doi: 10.1002/cam4.3881
43. Gauthier J, Gazeau N, Hirayama AV, Hill JA, Wu V, Cearley A, et al. Impact of CD.19 CAR, T-cell product type on outcomes in relapsed or refractory aggressive B-NHL. Blood. (2022) 139:3722–31. doi: 10.1182/blood.2021014497
44. Möhn N, Bonda V, Grote-Levi L, Panagiota V, Fröhlich T, Schultze-Florey C, et al. Neurological management and work-up of neurotoxicity associated with CAR T cell therapy. Neurol Res Pract. (2022) 4:1. doi: 10.1186/s42466-021-00166-5
45. Schoeberl F, Tiedt S, Schmitt A, Blumenberg V, Karschnia P, Burbano VG, et al. Neurofilament light chain serum levels correlate with the severity of neurotoxicity after CART. cell treatment. Blood Adv. (2022) 6:3022–26. doi: 10.1182/bloodadvances.2021006144
46. Butt OH, Zhou AY, Caimi PF, Luckett PH, Wisch JK, Derenoncourt PR Assessment of pretreatment posttreatment evolution of neurofilament light chain levels in patients who develop immune effector cell-associated neurotoxicity syndrome. JAMA. (2022) 5:e223738. doi: 10.1001/jamaoncol.2022.3738
47. Qi Y, Zhao M, Hu Y, Wang Y, Li P, Cao J, et al. Efficacy and safety of CD19-specific CAR T cell-based therapy in B-cell acute lymphoblastic leukemia patients with CNSL. Blood. (2022) 139 3376–86. doi: 10.1182/blood.2021013733
48. Tang JP, Peters CW, Quiros C, Wang X, Klomhaus AM, Yamada RE, et al. Hypophosphatemia due to increased effector cell metabolic activity is associated with neurotoxicity symptoms in CD19-targeted CART cell therapy. Cancer Immunol Res. (2022) 3:OF1–8. doi: 10.1158/2326-6066.CIR-22-0418
49. Gust J, Rawlings-Rhea SD, Wilson AL, Tulberg NM, Sherman AL, Seidel KD, et al. Increase during neurotoxicity from high baseline levels in pediatric CD.19-CAR, T-cell patients. Blood Adv. (2022) 3:8119. doi: 10.1182./bloodadvances.2022008119
50. Neelapu SS, Jacobson CA, Oluwole OO, Munoz J, Deol A, Miklos DB, et al. Outcomes of older patients in ZUMA, 1 a pivotal study of axicabtagene ciloleucel in refractory large B-cell lymphoma. Blood. (2020) 135:2106–9. doi: 10.1182/blood.2019004162
51. Lin RJ, Lobaugh SM, Pennisi M, Chan HT, Batlevi Y, Ruiz JD, et al. Impact and safety of chimeric antigen receptor T-cell therapy in older vulnerable patients with relapsed/refractory large B-cell lymphoma. Haematologica. (2021) 106:255–8. doi: 10.3324/haematol.2019.243246
52. Iacoboni G, Simó M, Villacampa G, Catalá E, Carpio C, Díaz-Lagares C, et al. Prognostic impact of total metabolic tumor volume in large B-cell lymphoma patients receiving CART-cell therapy. Ann Hematol. (2021) 100:2303–10. doi: 10.1007/s00277-021-04560-6
53. Sasanelli M, Meignan M, Haioun C, Berriolo-Riedinger A, Casasnovas RO, Biggi A, et al. Pretherapy metabolic tumour volume is an independent predictor of outcome in patients with diffuse large B-cell lymphoma. Eur J Nucl Med Mol Imaging. (2014) 41:2017–22. doi: 10.1007/s00259-014-2822-7
54. Dean EA, Mhaskar RS, Lu H, Mousa MS, Krivenko GS, Lazaryan A, et al. High metabolic tumor volume is associated with decreased efficacy of axicabtagene ciloleucel in large B-cell lymphoma. Blood Adv. (2020) 4:3268–76. doi: 10.1182/bloodadvances.2020001900
55. Wang J, Hu Y, Yang S, Wei G, Zhao X, Wu W, et al. Role of fluorodeoxyglucose positron emission tomography/computed tomography in predicting the adverse effects of chimeric antigen receptor T, cell therapy in patients with non-hodgkin lymphoma. Biol Blood Marrow Transplant. (2019) 25:1098. doi: 10.1016/j.bbmt.2019.02.008.
56. Gust J, Ishak GE. Chimeric antigen receptor T-cell neurotoxicity neuroimaging: more than meets the eye. AJNR Am J Neuroradiol. (2019) 40:E50–E51. doi: 10.3174/ajnr.A6184
57. Strati P, Nastoupil LJ, Westin J, Fayad LE, Ahmed S, Fowler NH, et al. Clinical and radiologic correlates of neurotoxicity after axicabtagene ciloleucel in large B-cell lymphoma. Blood Adv. (2020) 4:3943–51. doi: 10.1182/bloodadvances.2020002228
58. Jeon BH, Khanday F, Deshpande S, Haile A, Ozaki M, Irani K, et al. Tie-ing the antiinflammatory effect of angiopoietin-1 to inhibition of NF-kappa B. Circ Res. (2003) 92:586–8. doi: 10.1161/01.RES.0000066881.04116.45
59. Hakanpaa L, Sipila T, Leppanen VM, Gautam P, Nurmi H, Jacquemet G, et al. Endothelial destabilization by angiopoietin-2 via integrin β1 activation. Nat Commun. (2015) 6:5962. doi: 10.1038/ncomms6962
60. Saharinen P, Eklund L, Alitalo K. Therapeutic targeting of the angiopoietin,-TIE, pathway. Nat Rev Drug Discov. (2017) 16:635–61. doi: 10.1038/nrd.2016.278
61. Fabrizio VA, Boelens JJ, Mauguen A, Baggott C, Prabhu S, Egeler E, et al. Optimal fludarabine lymphodepletion is associated with improved outcomes after CART and cell therapy. Blood Adv. (2022) 6:1961–8. doi: 10.1182/bloodadvances.2021006418
62. Liu C, Ayyar VS, Zheng X, Chen W, Zheng S, Mody H, et al. Model-based cellular kinetic analysis of chimeric antigen receptor-T, cells in humans. Clin Pharmacol Ther. (2021) 109:716–27. doi: 10.1002/cpt.2040
63. Morris EC, Neelapu SS, Giavridis T, Sadelain M. Cytokine release syndrome and associated neurotoxicity in cancer immunotherapy. Nat Rev Immunol. (2022) 22:85–96. doi: 10.1038/s41577-021-00547-6
64. Giavridis T, van der Stegen SJC, Eyquem J, Hamieh M, Piersigilli A, Sadelain MCART, et al. cell-induced cytokine release syndrome is mediated by macrophages and abated by IL-1 blockade. Nat Med. (2018) 24:731–738. doi: 10.1038/s41591-018-0041-7
65. Norelli M, Camisa B, Barbiera G, Falcone L, Purevdorj A, Genua M, et al. Monocyte-derived IL.-,1 and IL-6 are differentially required for cytokine-release syndrome and neurotoxicity due to CAR T cells. Nat Med. (2018) 24:739–48. doi: 10.1038/s41591-018-0036-4
66. Lippitz BE. Cytokine patterns in patients with cancer: a systematic review. Lancet Oncol. (2013) 14:e218–28. doi: 10.1016/S1470-2045.12.70582-X
67. Zhao S, Wu D, Wu P, Wang Z, Huang J. Serum IL-10 predicts worse outcome in cancer patients: a meta-analysis. PLoS ONE. (2015) 10:e0139598. doi: 10.1371/journal.pone.0139598
68. Ouyang W. O'Garra A. IL-10 Family Cytokines IL-10 and IL-22: from basic science to clinical. Translat Immun. (2019) 50:871–91. doi: 10.1016/j.immuni.03020
69. Mumm JB, Oft M. Pegylated IL-10 induces cancer immunity: the surprising role of IL-10 as a potent inducer of IFN-γ-mediated CD8. (+). T cell cytotoxicity. Bioessays. (2013) 35:623–31. doi: 10.1002/bies.201300004
70. Amidi Y, Eckhardt CA, Quadri SA, Malik P, Firme MS, Jones DK, et al. Forecasting immune effector cell-associated neurotoxicity syndrome after chimeric antigen receptor t-cell therapy. J Immunother Cancer. (2022) 10:e005459. doi: 10.1136/jitc-2022-005459
71. Diorio C, Shraim R, Myers R, Behrens EM, Canna S, Bassiri H, et al. Comprehensive Serum proteome profiling of cytokine release syndrome and immune effector cell-associated neurotoxicity syndrome patients with B-cell all receiving CAR, T19. Clin Cancer Res. (2022) 28:3804–3813. doi: 10.1158/1078-0432.CCR-22-0822
Keywords: ICANS, neurotoxicity, predictive biomarker, review, cellular therapies, CRS, cytokine release syndrome, cytokines
Citation: Butt OH, Zhou AY, Ances BM, DiPersio JF and Ghobadi A (2023) A systematic framework for predictive biomarkers in immune effector cell-associated neurotoxicity syndrome. Front. Neurol. 14:1110647. doi: 10.3389/fneur.2023.1110647
Received: 29 November 2022; Accepted: 23 January 2023;
Published: 10 February 2023.
Edited by:
Stella Bouziana, King's College Hospital NHS Foundation Trust, United KingdomReviewed by:
Umberto Pensato, Humanitas Research Hospital, ItalyMarsilio Adriani, 4D Pharma Plc, United Kingdom
Copyright © 2023 Butt, Zhou, Ances, DiPersio and Ghobadi. This is an open-access article distributed under the terms of the Creative Commons Attribution License (CC BY). The use, distribution or reproduction in other forums is permitted, provided the original author(s) and the copyright owner(s) are credited and that the original publication in this journal is cited, in accordance with accepted academic practice. No use, distribution or reproduction is permitted which does not comply with these terms.
*Correspondence: Omar H. Butt, b21hcmhidXR0JiN4MDAwNDA7d3VzdGwuZWR1