- 1NeuroAging Laboratory (NEURAL), Clinical Neurosciences Research Laboratory (LINC), Health Research Institute of Santiago de Compostela (IDIS), Santiago de Compostela, Spain
- 2Centro Hospitalar e Universitário de Coimbra, Coimbra, Portugal
- 3Faculdade de Medicina da Universidade de Coimbra, Coimbra, Portugal
- 4Centro Neurociências e Biologia Celular, Coimbra, Portugal
- 5Neuroimaging and Biotechnology Laboratory (NOBEL), Clinical Neurosciences Research Laboratory (LINC), Health Research Institute of Santiago de Compostela (IDIS), Santiago de Compostela, Spain
- 6Neurovascular Research Laboratory, Vall d'Hebron Research Institute, Universitat Autònoma de Barcelona, Barcelona, Spain
- 7CNC-Center for Neuroscience and Cell Biology, CIBB-Centre for Innovative Biomedicine and Biotechnology, UC, Biotech Parque Tecnológico de Cantanhede, University of Coimbra, Coimbra, Portugal
Ischemic stroke is becoming one of the most common causes of death and disability in developed countries. Since current therapeutic options are quite limited, focused on acute reperfusion therapies that are hampered by a very narrow therapeutic time window, it is essential to discover novel treatments that not only stop the progression of the ischemic cascade during the acute phase, but also improve the recovery of stroke patients during the sub-acute or chronic phase. In this regard, several studies have shown that endothelial progenitor cells (EPCs) can repair damaged vessels as well as generate new ones following cerebrovascular damage. EPCs are circulating cells with characteristics of both endothelial cells and adult stem cells presenting the ability to differentiate into mature endothelial cells and self-renew, respectively. Moreover, EPCs have the advantage of being already present in healthy conditions as circulating cells that participate in the maintenance of the endothelium in a direct and paracrine way. In this scenario, EPCs appear as a promising target to tackle stroke by self-promoting re-endothelization, angiogenesis and vasculogenesis. Based on clinical data showing a better neurological and functional outcome in ischemic stroke patients with higher levels of circulating EPCs, novel and promising therapeutic approaches would be pharmacological treatment promoting EPCs-generation as well as EPCs-based therapies. Here, we will review the latest advances in preclinical as well as clinical research on EPCs application following stroke, not only as a single treatment but also in combination with new therapeutic approaches.
Introduction
Ischemic stroke remains as one of the leading causes of mortality and disability in Europe (1, 2). Following the ischemic insult, two large areas can be usually distinguished: the ischemic core, infarcted tissue with irreparable damage; and the penumbra area, surrounding the ischemic core, which contains hypoperfused tissue that is still viable (3). Following the occlusion of the blood vessel, the pathological paths of the ischemic cascade are activated, which eventually leads to neuronal death by either necrosis or apoptosis (4, 5). Hypoxic environment triggers glutamate excitotoxicity through a massive release of glutamate after injury, which provokes neurotoxicity by binding to N-methyl-D-aspartate receptors (NMDAR), promoting the entry of large amounts of Ca2+ in neurons (4–8). In addition, high concentrations of intracellular Ca2+ produce neuronal damage by increasing endoplasmic reticulum (ER) stress, reactive oxygen species (ROS), and depletion of ATP-depending processes (4, 5). Moreover, all together lead to the uncoupling of the mitochondrial electron chain, further increasing ROS and cellular death (4, 5). Overall, it is estimated that 2 million neurons die for every minute of obstruction (9).
Among others, mechanical thrombectomy and thrombolysis by the recombinant tissue plasminogen activator (rtPA) are effective therapeutic approaches by restoring the blood flow (10–17). However, these reperfusion therapies are hampered by a very narrow therapeutic time window, therefore it is necessary to search for alternatives and/or complementary treatments encompassed in, monitoring of cerebral homeostasis, reperfusion, neuroprotection and neurorepair strategies (12, 18, 19). In fact, angiogenesis and vasculogenesis represent two targets to obtain improvements in the outcome of patients following ischemic stroke (20–22). Whereas, angiogenesis means the sprouting of new blood vessels from uninjured ones, vasculogenesis is the de novo formation of blood vessels driven by endothelial progenitor cells (EPCs) (23). Importantly, it has been suggested that proinflammatory environments (e.g., following stroke) may enhance the proliferation and angiogenic function of EPCs (24). Therefore, both endothelial cell repair-regeneration and tissue neovascularization appear as key processes to restore the blood supply in the cortical areas affected by the vessel occlusion.
EPCs are circulating cells that exhibit both, progenitor- and stem-cell characteristics, since they have the capacity to differentiate into mature endothelial cells and self-renewing (25, 26). In the late 90's, Asahara et al. were the pioneers to identify EPCs in peripheral blood (27), and to determine their medullar origin (20). However, there is controversy nowadays regarding the origin of EPCs as both vascular and white adipocytic niches have been suggested over the years (28–31). The main function of EPCs is the maintenance of the endothelium by either releasing angiogenic growth factors or acting as a cellular reservoir for the replacement of injured endothelial cells (25, 32, 33). It is widely established that EPCs can be defined according to their angiogenic properties: early-outgrowth EPCs and late-outgrowth EPCs (25, 32, 33). On one hand, the early-outgrowth EPCs, or colony-forming unit endothelial cells (CFU-ECs), participate in the maintenance of the endothelium by the paracrine release of different pro-angiogenic factors (33). On the other hand, late-outgrowth EPCs, or endothelial colony-forming cells (ECFC), are characterized by their capacity to differentiate into mature endothelial cells, being the main player in the de novo formation of blood vessels (33). Late EPCs can also secrete several angiogenic factors, but in smaller quantities than early EPCs (34). Furthermore, these two types of EPCs can also be differentiated/classified by their in vitro characteristics: whereas early EPCs appear within a few days of culture and form colonies with spindle-shaped cells surrounding them, late EPCs appear at 2–3 weeks and show a cobblestone shape (34). Finally, kinase insert domain receptor (KDR) (also known as vascular endothelial growth factor receptor 2, VEGFR-2), CD34, and CD133 are the most common surface markers to detect EPCs (25). However, there are discrepancies regarding CD133 since it was also established as a specific marker for early EPCs (33). In addition, CD146 and von Willebrand factor (vWF) are characteristic markers of late EPCs (33). Overall, unfortunately, a unique marker to define EPCs clearly is still lacking.
Several studies have demonstrated that different factors mobilize EPCs from their niches, such as granulocyte macrophage-colony stimulating factor (GM-CSF) (35, 36), granulocyte-colony stimulating factor (G-CSF) (37, 38); vascular endothelial growth factor (VEGF) (39–42); stromal-derived factor-1α (SDF-1α) (41–44); and erythropoietin (EPO) (45). Interestingly, some of these factors (e.g., VEGF, GM-CSF, and G-CSF) are also released by EPCs as paracrine signaling, promoting angiogenesis and triggering the proliferation and migration of endothelial cells (46–48). Moreover, EPCs release different vasodilators and vasoconstrictors factors, such as nitric oxide (NO) through the endothelial nitric oxide synthase (eNOS) activity (34, 49), and the tPA (49); as well as anti-inflammatory molecules (50). In the recent years, the role of extracellular vesicles and exosomes has been described in cell-to-cell signaling (51–53). Interestingly, EPCs also release extracellular vesicles that participate in angiogenesis (53, 54). Therefore, all these features make EPCs an interesting target following cerebral ischemia as they modulate vasculogenesis and angiogenesis, either by stimulating re-endothelialization of injured vessels or by stimulating the formation of new ones (26, 55, 56). Specifically, hypoxia-inducible factor-1 (HIF-1) is up-regulated under hypoxic conditions, which activates the expression of VEGF and SDF-1α, among others; eventually inducing the homing of EPCs to the hypoxic tissues (44, 48, 55–58) (Figure 1). In addition to modulating vasculogenesis and angiogenesis, EPCs release different factors that reduce inflammation, promote neuronal survival, and maintain the myelin sheath integrity in the damaged tissue, the latter probably by regulating the survival and migration of oligodendrocytes through SDF-1α interaction (59, 60). In summary, early and late EPCs work synergistically for proper angiogenesis and revascularization: the high concentration of angiogenic factors and inflammatory cytokines in the ischemic injury area attracts early EPCs, then, early EPCs release different factors in a paracrine way that promote angiogenesis and recruit late EPCs to either restore the endothelium or form new vessels guided by the early EPCs (33) (Figure 1).
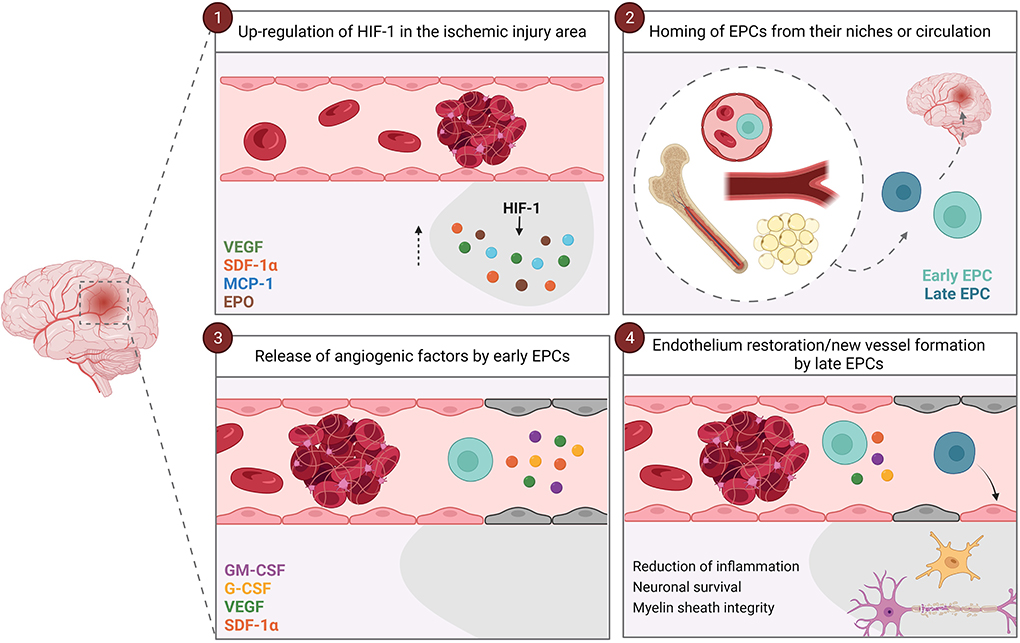
Figure 1. Vascular repair mediated by EPCs. After an ischemic stroke, HIF-1 is up-regulated and induces the expression of VEGF, SDF-1α, MCP-1 and EPO in the ischemic injury area (1). These factors induce the homing of EPCs from their niches (bone marrow, vascular niches or white adipose tissue) or circulation to the ischemic brain area (2). Firstly, early EPCs arrives at the site of injury, and paracrinally release factors that promote angiogenesis and recruit late EPCs (3). Once the late EPCs arrive at the ischemic lesion zone, they restore the endothelium or from new vessels guided by the early EPCs (4). In addition, both early and late EPCs reduce inflammation and promote neuronal survival and myelin sheath integrity. EPCs, endothelial progenitor cells; EPO, erythropoietin; G-CSF, granulocyte-colony stimulating factor; GM-CSF, granulocyte macrophage-colony stimulating factor; HIF-1, hypoxia-inducible factor-1; MCP-1, monocyte chemoattractant protein-1; SDF-1α, stromal-derived factor-1α; VEGF, vascular endothelial growth factor.
Therefore, EPCs appear as a promising target to find new therapies to overcome the ischemic damage, all thanks to their ability to both directly participate in blood vessel repair and remodeling, and indirectly release different trophic factors to stimulate the repairing processes following ischemic injury. Here, we will review the latest advances in preclinical as well as clinical research at EPCs application following stroke, not only as a single treatment but also in combination with new approaches.
Relationship between EPCs levels and the prognosis following stroke
For years, the quantification of EPCs has been proposed as a possible surrogate biomarker of cardiovascular function, since low circulating levels of EPCs were associated with increased cardiovascular risk (61–63). Indeed, although EPCs have been related to endothelial cell regeneration and neovascularization after tissue ischemia (61, 64–66), such cerebrovascular risk factors reduce the baseline levels of circulating EPCs (67). Accordingly, stroke patients displayed a lower number of EPCs at the ischemic event onset than their healthy counterparts (65); and such a reduced level of EPCs was negatively correlated with systolic blood pressure, diastolic blood pressure, triglycerides, LDL, and fasting blood glucose, all considered as vascular risk factors (67). Interestingly, proteomic analysis of samples from patients with ischemic stroke suggested that their EPCs were in a more advanced differentiation state and had a lower capacity for proliferation than those from healthy subjects (68). This study reveals that cerebral ischemia not only acts locally but also at systemic level, and such a general impact may be related to the functional impairment, since EPC-mediated protective vascular effects are mainly related to its proliferation ability. Clinical studies showed that the body counterattacks by increasing serum levels of pro-EPCs proliferation factors following ischemic stroke (69–72). Likewise, there is an increase in the levels of VEGF, SDF-1α, and active matrix metalloproteinase-9 (MMP-9) in the first 24 h after the onset, which are well-known molecules involved in EPC proliferation (73–75). Interestingly, the serum levels of these markers at 24 h correlated with the increment of EPCs during the first week (71, 76); although this raise can be negatively influenced by inflammatory factors such as leukocyte levels (76). Recently, high serum levels of VEGF at 3 months post-stroke have been associated with worse long-term (2-year) functional outcomes after injury (77), suggesting that long-term values of pro-EPCs proliferation factors might not be as beneficial as an acute increase.
Although controversial (78, 79), it has been widely observed that higher levels of circulating EPCs are related to a better prognosis following ischemic stroke (80–85). Indeed, our group was the first one to demonstrate the correlation between EPCs levels and both the injury progression and outcome following ischemic stroke (80). We observed that patients with higher EPCs numbers during the first week after ischemic stroke showed a reduction of the infarcted area and a neurological improvement at days 7 and 90; as well as a better outcome at 3 months compared to those with lower levels of EPCs (80). Importantly, patients with good outcome had higher numbers of EPCs than those with poor outcome on day 7 and at 3 months after ischemic stroke, but not at admission; and so, these results support the alleged beneficial role of EPCs mobilization following cerebral ischemia (80, 85). Curiously, it has been observed that the functional properties of EPCs to migrate and promote angiogenesis have more effect on both clinical outcome and in vivo angiogenesis than the absolute cell number (85–87); and that all EPC subsets would contribute similarly to this post-stroke angiogenesis (85). This can be observed in the positive association between functional properties of EPCs and increased flow diversion and cerebral blood flow following ischemic stroke (88). Nevertheless, the EPC-related cellular mechanisms underlying endogenous vascular repair, better neurological and functional outcome improvements and smaller growth of infarct volume are not fully understood. In the recent years, microRNAs (miRNAs) have been described to modulate the expression of several genes, gaining a special interest as therapeutic molecules (89). Accordingly, the profiling of miRNAs identified several ones potentially involved in the EPC-mediated angiogenesis and eventually good functional outcome following ischemic stroke (85). The analysis of CD34+ cells revealed that only 9 miRNAs were differentially expressed in the patients with a good outcome: the downregulated ones (hsa-miRNA-22-5p and has-miRNA-32-3p) induce cell senescence, and the upregulated ones (miRNA-27b24, miRNA-181d25, and miRNA-328) are involved in cell migration and angiogenesis (85). Interestingly, those mechanisms underlying the mobilization of CD34+ cells are also present following hemorrhagic stroke (90–92). Along these lines, a study using a mouse model of intracerebral hemorrhage (ICH) revealed that the single-nucleotide polymorphism (arginine-to-proline substitution) present at the codon 72 of the human Tp53 gene (a tumor suppressor gene) is directly related to endothelial cell survival, EPCs mobilization and neovascularization (93). Moreover, ICH patients carrying the arginine allele had a delayed neovascularization and impaired functional outcome compared to those patients with the proline allele (93). In summary, further studies are needed to decipher the mechanisms by which EPCs are associated with cellular, neurological, and functional improvements following ischemic stroke.
Targeting EPCs as a promising treatment following stroke
The direct use of EPCs as a possible treatment for ischemic damage has been studied for years in different animal models, in which intravenously EPC-based cell therapy was the most used one (Table 1). In addition, recent treatments based on the secretome of EPCs and drugs that mobilize EPCs from their physiological niches have been also addressed (Table 1). Regarding clinical studies, the number of published results using the direct administration of EPCs in human is scarce, and most of the studies are based on pharmacological therapies increasing the mobilization of circulating EPCs (Table 1).
Cell-based therapies using EPCs
In different animal models of middle cerebral artery occlusion (MCAO), the intravenous injection of late EPCs reduced the infarct volume and the number of apoptotic cells, promoted angiogenesis, neurogenesis, axonal rewiring and BBB protection at the site of injury, as well as a functional and behavioral improvement after ischemic injury (94, 95, 97, 99). Remarkably, the time-point post-ischemia treatment apparently has no effect on the beneficial outcomes, since some studies treated animals at 24 h (94, 95), and others at 2 h and then for 7 days (97). Recently, an interesting approach has been developed, in which EPCs were virally transfected with different cytokines to improve their therapeutic properties (59, 98). For example, EPCs transfected with adiponectin (98) or SDF-1α (59) have several benefits such as promoting angiogenesis, neurogenesis, remyelination, as well as attracting EPCs and other neuronal and oligodendrocyte progenitors to the cerebral ischemic tissues. Accordingly, this resulted in a larger vascular density, better functional recovery, and smaller infarct volume and apoptosis ratio when compared to animals treated with wild-type EPCs (59, 98). It is important to note that such pro-regenerative outcomes were seen at different time-window applications, 1 h (98), and 1 week (59); in different MCAO models, transient (98), and permanent (59); and even in animals with diabetes, a known cerebrovascular risk factor (98). Beyond ischemic stroke, intravenous injection of EPCs at 6 h post-ICH promoted positive effects by reducing edema, apoptosis, and BBB permeability; and increasing the expression of tight junction proteins (113). Moreover, the levels of pro-inflammatory cytokines such as interferon-γ, IL-6, and tumor necrosis factor-alpha were reduced, whereas those of anti-inflammatory cytokines such as transforming growth factor-β1 and IL-10 were increased (113). Importantly, the magnetic vectorization of EPCs has been tested with satisfactory results (114); which encourages to keep researching new approaches that improve the cell delivery to the ischemic area.
Despite these promising results in animal models of stroke, the number of published results and clinical trials regarding direct application of EPCs in patients is almost absent. This is remarkable when we have in mind the fact that EPCs can home to the damaged BBB and promote its restoration, which would be extremely beneficial for patients with ischemic damage. The work from Fang et al. exposed the outcomes after the intravenously transplantation of EPCs (100). Such results came from a small clinical trial (EPCs group, N = 5; placebo group, N = 6; NCT01468064 in ClinicalTrials.gov) over 4 years, where authors observed a slight improvement in neurological and motor functions following the application of autologous EPCs (100). Although no significant differences were found in those outcomes compared to control subjects (possibly due to the small group of the follow-up), the trend was promising enough to further study both neurological and functional benefits of EPCs infusion in a larger cohort. Similarly, previous I and II trials showed encouraging results following autologous transplantation of CD34+ cells in stroke patients, either at the acute/subacute phase (115, 116), or chronic phase (117). It is important to remark that the administration of different subtypes of EPCs (late or early) and/or their origin (bone marrow, cord blood or spleen) might influence the outcome following application and, therefore, they are aspects to have in mind when analyzing the data. Overall, this reinforces the need of increasing the number of clinical trials addressing the beneficial outcomes of EPCs transplantation following stroke.
Therapies based on EPCs-derived secretome/exosomes
Although promising, the use of cell therapies in stroke implies several risks such as emboli, immunological incompatibility, or infection (50). Therefore, new and safer research lines for the treatment of stroke are mandatory to avoid such issues. In this regard, the impressive work of Rosell et al. demonstrated for the first time that the infusion of cell-free conditioned medium (secretome) from late EPCs at 1 day post-ischemia was sufficient to increase capillary density in peri-infarct areas and eventually improve functional recovery in mice undergoing permanent MCAO (95). Subsequently, the therapeutic benefit of secretome was tested in a mouse model of prolonged cerebral hypoperfusion by bilateral stenosis of the common carotid artery (101). Although the secretome was administered at 24 h and 7 days after starting cerebral hypoperfusion, the results were similar to those obtained by Rosell et al.: increased vascular density, myelin, and mature oligodendrocytes in the white matter as well as improved cognitive function (101). More recently, the secretome of EPCs derived from stroke patients has proved to restore BBB function and promote angiogenesis in in vitro models of ischemia (118). Regarding neurogenesis, the interaction between neural stem cells (NSCs) and endothelial cells has been suggested for years (22, 95, 119–123). In this crosstalk, NSCs provide a vasculotrophic support for endothelial cells (ECs) under hypoxic conditions via the VEGF/HIF-1α axis (122), whereas either EPCs- or ECs-derived secretome protect NSCs against ischemic damage [(95, 123), respectively]. Interestingly, in vitro studies from Jing et al. revealed that the culture of NSCs with EPC-conditioned medium from hypoxic conditions increased their proliferation rate likely through VEGF secretion (124). Similarly, EPCs-derived secretome increases the proliferative rate of oligodendrocyte precursor cells (OPCs) and decreases their apoptosis, as well as enhances myelination under hypoxic environments either in vitro (101, 125) or in vivo (101). Several growth factors might be exerting the beneficial role mediated by the EPCs-derived secretome on oligodendrocyte remodeling following ischemic damage, such as C-X-C motif chemokine 12, VEGF and angiogenin, among others (101, 125, 126).
One of the components of the secretome of EPCs are exosomes, a type of extracellular vesicle that participates in cell-to-cell communication by releasing its contents, as mentioned above (51–53, 102). In the case of EPCs-derived exosomes, they are known to participate in angiogenesis and even protect the endothelium from suffering further damage or dysfunction under the hypoxia-reoxygenation process (53, 54, 127). Notably, EPCs-exosomes reduced ischemic damage and apoptosis, preserved cerebral blood flow and vascular microdensity, promoted angiogenesis and neurogenesis, as well as, improved neurological function in a mouse model of permanent MCAO (102). Interestingly, this beneficial effect was enhanced by using exosomes enriched in miRNA-126, a molecule involved in vascular function and angiogenesis (102). In fact, one of the beneficial effects of practicing exercise before the stroke is that it increases miRNA-126 levels in EPCs-exosomes, which correlates with reduced infarct volume and apoptosis and increased microvascular density in mice undergoing permanent MCAO (128). Likewise, EPCs-exosomes transfected with miRNA-126 were uptaken by astrocytes enhancing their survival rate and reducing both cellular toxicity and ROS generation under in vitro hypoxia (129). Other miRNAs also have beneficial roles following ischemia, such as the miRNA-210 whose inclusion into EPCs-exosomes boosted their beneficial roles by improving the mitochondrial function in endothelial cells following hypoxia/reoxygenation damage (130).
As previously stated, the therapeutic application of EPCs still has some concerns, including the large number of cells needed for the treatment and their potential to become tumorigenic; and so, the attention is being focused on the use of EPCs-derived exosomes/secretome. Unfortunately, this field is in a preclinical stage, although the experiments with extracellular vesicles have demonstrated to exert similar beneficial roles to the cell-based treatment (118). Therefore, clinical trials and studies on humans are needed to confirm preclinical data regarding the use of EPCs-derived exosomes and/or secretomes as a more reliable and safer approach than cell-based therapies.
Pharmacological treatments targeting EPCs
As mentioned previously, drug treatments to increase the number of EPCs appear as another interesting alternative to cell therapy. The application of GM-CSF, SDF-1α, statins, or EPO results in the mobilization of EPCs from their niches. Indeed, several works observed cellular and functional improvements following the administration of these drugs in rats undergoing either transitory (103, 104) or permanent MCAO (105). Overall, such pharmacological treatments resulted in a reduction of infarct volume, apoptosis, BBB disruption; an increase of angiogenesis and neurogenesis; an improvement of neurological function (103–105); and, interestingly, an increase in the levels of factors that induce homing of EPCs, such as VEGF or basic fibroblast growth factor (bFGF) in peripheral blood (105). Moreover, the levels of several growth factors involved in neurogenesis, such as glial cell-derived neurotrophic factor (GDNF), growth-associated protein-43 (GAP-43) and BDNF were also increased (104, 105). Importantly, there is a therapeutic synergy when combining GM-CSF with SDF-1α (105) or EPCs with EPO (104), which improves the effects of both drugs and cells. Notably, the injection of EPO-primed EPCs lead to an improvement in their migratory capacity, and it also had beneficial effects on the cerebral blood flow, apoptosis, and BBB disruption in a rat model of transient MCAO (96). Importantly, some of these drugs, and others, have been tested in patients where they showed therapeutic benefits. For example, patients treated with statins or citicoline (CDP-choline) after the stroke had a significant increase in the levels and mobilization of EPCs, which predicted with high sensitivity and specificity a good outcome at 3 months following injury (110, 131, 132). Likewise, the treatment with piperlongumine, an alkaloid extracted from Piper longum, exhibited anti-platelet aggregation and anti-inflammatory properties, and its administration significantly improved the functions of EPCs as well as reduced ischemic damage in an ischemic brain model of high-fat diet-fed mice (106). Although all these drugs promote the mobilization of EPCs, none is EPCs-specific as they induce homing of other progenitor cells and other beneficial effects. Therefore, cellular and functional improvements seen in patients with stroke treated with those drugs cannot be attributed exclusively to their effect on EPCs. The development of new drugs targeting exclusively EPCs is mandatory to confirm that the EPC-mediated beneficial effects are enough to promote functional recovery following ischemia.
Recently, the potential clinical use of the G-CSF is getting attention as it possesses a more specific activity spectrum (133). The G-CSF is a glycoprotein released by endothelium and immune cells that acts as a hematopoietic growth factor (134). Among other beneficial mechanisms following vascular injury, the G-CSF can promote angiogenesis by mobilizing EPCs from the bone marrow to the peripheral blood (135). This makes it a promising target to enhance vascular repair in CNS injuries. However, results in ischemic stroke patients could not find reliable improvements neither the clinical outcome nor infarct volume, even after a phase IIb trial (107–109, 111, 112). Noteworthy, a meta-analysis study did show a motor improvement, which was probably due to the increase in CD34+ counts, therefore, a higher mobilization of EPCs to the damaged area (136). Interestingly, there is an ongoing phase 1/2a clinical trial to evaluate the safety and efficacy of combining EPO and G-CSF treatments in patients with neurological diseases, including ischemic and hemorrhagic stroke (NCT02018406, ClinicalTrials.gov). Overall, further studies are mandatory to confirm the potential use of G-CSF as another alternative treatment following cerebral ischemia.
Concluding remarks
As shown here, there is compelling evidence that support the potential of EPCs as a therapeutic target. Data from both preclinical and clinical studies showed promising results that are being slowly translated to clinical trials. Although the clinical trials have reduced the expectations regarding functional and cognitive outcomes; they do have shown the safety of the cell-based therapy (injection of EPCs) and drug treatments (G-CSF). Those clinical trials were performed in small cohorts of patients, and so, more trials with higher numbers are needed to confirm undoubtedly the impact of such therapies following cerebral ischemia. Finally, several preclinical studies have highlighted the beneficial role of EPCs secretome/exosomes, but no clinical trials using EPCs secretome have been carried out so far. Therefore, the use of such approach may overcome the concerning regarding EPCs cell-based therapies and provide a more specific spectrum than drug treatments.
Author contributions
Conceptualization: AC, DR-S, and TS. Resources and supervision: LF, JC, and TS. Critical literature review: AC, MA-N, JMP-P, and DR-S. Writing—original draft preparation: AC, DR-S, JS-F, and TS. Writing—review and editing: AC, AO, MA-N, JS-F, PH, JP-P, AR, LF, JC, DR-S, and TS. Funding acquisition: DR-S, AC, LF, JC, and TS. All authors have read and agreed to the published version of the manuscript.
Funding
This study was partially supported by grants from the Xunta de Galicia (PH, JC, and TS: IN607A2018/3, TS: IN607D 2020/09, AC: IN606A-2021/015), the Science Ministry of Spain (TS: RTI2018-102165-B-I00 and RTC2019-007373-1), and the Instituto de Salud Carlos III (AR: PI19/00186 and RD21/0006/0007). Furthermore, this study was also supported by grants from the INTERREG Atlantic Area (LF and TS: EAPA_791/2018_ NEUROATLANTIC Project), INTER-REG V A España Portugal (POCTEP) (LF and TS: 0624_2IQBIONEURO_6_E), the European Regional Development Fund (ERDF), the PT2020 program (LF: FEDER, project LABEL: POCI-01-0247-FEDER-049268), and the Fundação para a Ciência e Tecnologia (LF: project ENDEAVOUR: EXPL/BTM-ORG/1348/2021). Moreover, DR-S (CD21/00166), MA-N (IFI18/00008), and TS (CPII17/00027) are recipients of Sara Borrell, iPFIS, and Miguel Servet contracts, respectively, from the Instituto de Salud Carlos III. The funders had no role in the study design, data collection and analysis, decision to publish, or preparation of the manuscript.
Acknowledgments
Figure 1 was made with the Biorender.com software.
Conflict of interest
The authors declare that the research was conducted in the absence of any commercial or financial relationships that could be construed as a potential conflict of interest.
Publisher's note
All claims expressed in this article are solely those of the authors and do not necessarily represent those of their affiliated organizations, or those of the publisher, the editors and the reviewers. Any product that may be evaluated in this article, or claim that may be made by its manufacturer, is not guaranteed or endorsed by the publisher.
References
1. Rodríguez-Castro E, López-Dequit I, Santamaría-Cadavid M, Arias-Rivas S, Rodríguez-Yáñez M, Pumar JM, et al. Trends in stroke outcomes in the last ten years in a European tertiary hospital. BMC Neurol. (2018) 18:164. doi: 10.1186/s12883-018-1164-7
2. Feigin VL, Vos T, Nichols E, Owolabi MO, Carroll WM, Dichgans M, et al. The global burden of neurological disorders: translating evidence into policy. Lancet Neurol. (2020) 19:255–65. doi: 10.1016/S1474-4422(19)30411-9
3. Ramos-Cabrer P, Campos F, Sobrino T, Castillo J. Targeting the ischemic penumbra. Stroke. (2011) 42:S7–11. doi: 10.1161/STROKEAHA.110.596684
4. Dirnagl U, Iadecola C, Moskowitz MA. Pathobiology of ischaemic stroke: an integrated view. Trends Neurosci. (1999) 22:391–7. doi: 10.1016/S0166-2236(99)01401-0
5. He J, Liu J, Huang Y, Tang X, Xiao H, Hu Z. Oxidative stress, inflammation, and autophagy: potential targets of mesenchymal stem cells-based therapies in ischemic stroke. Front Neurosci. (2021) 15:641157. doi: 10.3389/fnins.2021.641157
6. Castillo J, Dávalos A, Noya M. Progression of ischaemic stroke and excitotoxic aminoacids. Lancet. (1997) 349:79–82. doi: 10.1016/S0140-6736(96)04453-4
7. Dávalos A, Castillo J, Serena J, Noya M. Duration of glutamate release after acute ischemic stroke. Stroke. (1997) 28:708–10. doi: 10.1161/01.STR.28.4.708
8. Castellanos M, Sobrino T, Pedraza S, Moldes O, Pumar JM, Silva Y, et al. High plasma glutamate concentrations are associated with infarct growth in acute ischemic stroke. Neurology. (2008) 71:1862–8. doi: 10.1212/01.wnl.0000326064.42186.7e
9. Desai SM, Rocha M, Jovin TG, Jadhav AP. High variability in neuronal loss. Stroke. (2019) 50:34–7. doi: 10.1161/STROKEAHA.118.023499
10. Hacke W, Kaste M, Fieschi C, Toni D, Lesaffre E, von Kummer R, et al. Intravenous thrombolysis with recombinant tissue plasminogen activator for acute hemispheric stroke: the european cooperative acute stroke study (ECASS). JAMA. (1995) 274:1017–25. doi: 10.1001/jama.1995.03530130023023
11. Hacke W, Donnan G, Fieschi C, Kaste M, von Kummer R, Broderick JP, et al. Association of outcome with early stroke treatment: pooled analysis of ATLANTIS, ECASS, and NINDS rt-PA stroke trials. Lancet. (2004) 363:768–74. doi: 10.1016/S0140-6736(04)15692-4
12. Hacke W, Kaste M, Bluhmki E, Brozman M, Dávalos A, Guidetti D, et al. Thrombolysis with alteplase 3 to 4.5 hours after acute ischemic stroke. N Engl J Med. (2008) 359:1317–29. doi: 10.1056/NEJMoa0804656
13. Ahmed N, Wahlgren N, Brainin M, Castillo J, Ford GA, Kaste M, et al. Relationship of blood pressure, antihypertensive therapy, and outcome in ischemic stroke treated with intravenous thrombolysis: retrospective analysis from Safe Implementation of Thrombolysis in Stroke-International Stroke Thrombolysis Register (SITS-ISTR). Stroke. (2009) 40:2442–9. doi: 10.1161/STROKEAHA.109.548602
14. Sobrino T, Millán M, Castellanos M, Blanco M, Brea D, Dorado L, et al. Association of growth factors with arterial recanalization and clinical outcome in patients with ischemic stroke treated with tPA. J Thromb Haemost. (2010) 8:1567–74. doi: 10.1111/j.1538-7836.2010.03897.x
15. Wardlaw JM, Murray V, Berge E, del Zoppo GJ. Thrombolysis for acute ischaemic stroke. Cochrane Database Syst Rev. (2014) 2014:CD000213. doi: 10.1002/14651858.CD000213.pub3
16. Lambrinos A, Schaink AK, Dhalla I, Krings T, Casaubon LK, Sikich N, et al. Mechanical thrombectomy in acute ischemic stroke: a systematic review. Can J Neurol Sci. (2016) 43:455–60. doi: 10.1017/cjn.2016.30
17. Matsumoto S, Mikami T, Iwagami M, Briasoulis A, Ikeda T, Takagi H, et al. Mechanical thrombectomy and intravenous thrombolysis in patients with acute stroke: a systematic review and network meta-analysis. J Stroke Cerebrovasc Dis. (2022) 31:106491. doi: 10.1016/j.jstrokecerebrovasdis.2022.106491
18. Iglesias-Rey R, Rodríguez-Yáñez M, Rodríguez-Castro E, Pumar JM, Arias S, Santamaría M, et al. Worse outcome in stroke patients treated with rt-PA without early reperfusion: associated factors. Transl Stroke Res. (2018) 9:347–55. doi: 10.1007/s12975-017-0584-9
19. Yang JL, Yang YR, der Chen S. The potential of drug repurposing combined with reperfusion therapy in cerebral ischemic stroke: a supplementary strategy to endovascular thrombectomy. Life Sci. (2019) 236:116889. doi: 10.1016/j.lfs.2019.116889
20. Asahara T, Masuda H, Takahashi T, Kalka C, Pastore C, Silver M, et al. Bone marrow origin of endothelial progenitor cells responsible for postnatal vasculogenesis in physiological and pathological neovascularization. Circ Res. (1999) 85:221–8. doi: 10.1161/01.RES.85.3.221
21. Hayashi T, Noshita N, Sugawara T, Chan PH. Temporal profile of angiogenesis and expression of related genes in the brain after ischemia. J Cereb Blood Flow Metab. (2003) 23:166–80. doi: 10.1097/01.WCB.0000041283.53351.CB
22. Liu XS, Zhang ZG, Zhang RL, Gregg S, Morris DC, Wang Y, et al. Stroke induces gene profile changes associated with neurogenesis and angiogenesis in adult subventricular zone progenitor cells. J Cereb Blood Flow Metab. (2007) 27:564–74. doi: 10.1038/sj.jcbfm.9600371
23. Arenillas JF, Sobrino T, Castillo J, Dávalos A. The role of angiogenesis in damage and recovery from ischemic stroke. Curr Treat Options Cardiovasc Med. (2007) 9:205–12. doi: 10.1007/s11936-007-0014-5
24. Rosell A, Arai K, Lok J, He T, Guo S, Navarro M, et al. Interleukin-1β augments angiogenic responses of murine endothelial progenitor cells in vitro. J Cerebral Blood Flow Metabol. (2009) 29:933–43. doi: 10.1038/jcbfm.2009.17
25. Hristov M, Erl W, Weber P. Endothelial progenitor cells: isolation and characterization. Trends Cardiovasc Med. (2003) 13:201–6. doi: 10.1016/S1050-1738(03)00077-X
26. Yoder MC. Human endothelial progenitor cells. Cold Spring Harb Perspect Med. (2012) 2:a006692. doi: 10.1101/cshperspect.a006692
27. Asahara T, Murohara T, Sullivan A, Silver M, van der Zee R, Li T, et al. Isolation of putative progenitor endothelial cells for angiogenesis. Science. (1997) 275:964–7. doi: 10.1126/science.275.5302.964
28. Lin RZ, Moreno-Luna R, Muñoz-Hernandez R, Li D, Jaminet SCS, Greene AK, et al. Human white adipose tissue vasculature contains endothelial colony-forming cells with robust in vivo vasculogenic potential. Angiogenesis. (2013) 16:735–44. doi: 10.1007/s10456-013-9350-0
29. Solomon I, O'Reilly M, Ionescu L, Alphonse RS, Rajabali S, Zhong S, et al. Functional differences between placental micro- and macrovascular endothelial colony-forming cells. Stem Cells Transl Med. (2016) 5:291–300. doi: 10.5966/sctm.2014-0162
30. Green L, Ofstein RH, Rapp B, Saadatzadeh MR, Bhavsar JR, Fajardo A, et al. Adult venous endothelium is a niche for highly proliferative and vasculogenic endothelial colony-forming cells. J Vasc Surg. (2017) 66:1854–63. doi: 10.1016/j.jvs.2016.11.059
31. Iba T, Naito H, Shimizu S, Rahmawati FN, Wakabayashi T, Takakura N. Isolation of tissue-resident endothelial stem cells and their use in regenerative medicine. Inflamm Regen. (2019) 39:1–8. doi: 10.1186/s41232-019-0098-9
32. Malinovskaya NA, Komleva YK, Salmin VV, Morgun AV, Shuvaev AN, Panina YA, et al. Endothelial progenitor cells physiology and metabolic plasticity in brain angiogenesis and blood-brain barrier modeling. Front Physiol. (2016) 7:599. doi: 10.3389/fphys.2016.00599
33. Banno K, Yoder MC. Tissue regeneration using endothelial colony-forming cells: promising cells for vascular repair. Pediatr Res. (2018) 83:283–90. doi: 10.1038/pr.2017.231
34. Hur J, Yoon CH, Kim HS, Choi JH, Kang HJ, Hwang KK, et al. Characterization of two types of endothelial progenitor cells and their different contributions to neovasculogenesis. Arterioscler Thromb Vasc Biol. (2004) 24:288–93. doi: 10.1161/01.ATV.0000114236.77009.06
35. Cho HJ, Kim HS, Lee MM, Kim DH, Yang HJ, Hur J, et al. Mobilized endothelial progenitor cells by granulocyte-macrophage colony-stimulating factor accelerate reendothelialization and reduce vascular inflammation after intravascular radiation. Circulation. (2003) 108:2918–25. doi: 10.1161/01.CIR.0000097001.79750.78
36. Wang QR, Wang F, Zhu WB, Lei J, Huang YH, Wang BH, et al. GM-CSF accelerates proliferation of endothelial progenitor cells from murine bone marrow mononuclear cells in vitro. Cytokine. (2009) 45:174–8. doi: 10.1016/j.cyto.2008.12.002
37. Peichev M, Naiyer AJ, Pereira D, Zhu Z, Lane WJ, Williams M, et al. Expression of VEGFR-2 and AC133 by circulating human CD34+ cells identifies a population of functional endothelial precursors. Blood. (2000) 95:952–8. doi: 10.1182/blood.V95.3.952.003k27_952_958
38. Lin X, Wang H, Li X. Mobilization of endothelial progenitor cells promotes angiogenesis after full thickness excision by AMD3100 combined with G-CSF in diabetic mice by SDF-1/CXCR4 axis. Diab Vasc Dis Res. (2021) 18:473. doi: 10.1177/14791641211002473
39. Asahara T, Takahashi T, Masuda H, Kalka C, Chen D, Iwaguro H, et al. VEGF contributes to postnatal neovascularization by mobilizing bone marrow-derived endothelial progenitor cells. EMBO J. (1999) 18:3964–72. doi: 10.1093/emboj/18.14.3964
40. Hattori K, Dias S, Heissig B, Hackett NR, Lyden D, Tateno M, et al. Vascular endothelial growth factor and angiopoietin-1 stimulate postnatal hematopoiesis by recruitment of vasculogenic and hematopoietic stem cells. J Exp Med. (2001) 193:1005–14. doi: 10.1084/jem.193.9.1005
41. Moore MAS, Hattori K, Heissig B, Shieh JH, Dias S, Crystal RG, et al. Mobilization of endothelial and hematopoietic stem and progenitor cells by adenovector-mediated elevation of serum levels of SDF-1, VEGF, and angiopoietin-1. Ann N Y Acad Sci. (2001) 938:36–47. doi: 10.1111/j.1749-6632.2001.tb03572.x
42. Fox A, Smythe J, Fisher N, Tyler MPH, McGrouther DA, Watt SM, et al. Mobilization of endothelial progenitor cells into the circulation in burned patients. Br J Surg. (2008) 95:244–51. doi: 10.1002/bjs.5913
43. Hattori K, Heissig B, Tashiro K, Honjo T, Tateno M, Shieh JH, et al. Plasma elevation of stromal cell-derived factor-1 induces mobilization of mature and immature hematopoietic progenitor and stem cells. Blood. (2001) 97:3354–60. doi: 10.1182/blood.V97.11.3354
44. Ceradini DJ, Kulkarni AR, Callaghan MJ, Tepper OM, Bastidas N, Kleinman ME, et al. Progenitor cell trafficking is regulated by hypoxic gradients through HIF-1 induction of SDF-1. Nat Med. (2004) 10:858–64. doi: 10.1038/nm1075
45. Heeschen C, Aicher A, Lehmann R, Fichtlscherer S, Vasa M, Urbich C, et al. Erythropoietin is a potent physiologic stimulus for endothelial progenitor cell mobilization. Blood. (2003) 102:1340–6. doi: 10.1182/blood-2003-01-0223
46. Rehman J, Li J, Orschell CM, March KL. Peripheral blood “endothelial progenitor cells” are derived from monocyte/macrophages and secrete angiogenic growth factors. Circulation. (2003) 107:1164–9. doi: 10.1161/01.CIR.0000058702.69484.A0
47. Cheng CC, Chang SJ, Chueh YN, Huang TS, Huang PH, Cheng SM, et al. Distinct angiogenesis roles and surface markers of early and late endothelial progenitor cells revealed by functional group analyses. BMC Genomics. (2013) 14:1–10. doi: 10.1186/1471-2164-14-182
48. Yan F, Liu X, Ding H, Zhang W. Paracrine mechanisms of endothelial progenitor cells in vascular repair. Acta Histochem. (2022) 124:151833. doi: 10.1016/j.acthis.2021.151833
49. Shirota T, He H, Yasui H, Matsuda T. Human endothelial progenitor cell-seeded hybrid graft: proliferative and antithrombogenic potentials in vitro and fabrication processing. Tissue Eng. (2004) 9:127–36. doi: 10.1089/107632703762687609
50. Alwjwaj M, Rais Reskiawan AK, Bayraktutan U. The secretome of endothelial progenitor cells: a potential therapeutic strategy for ischemic stroke. Neural Regen Res. (2021) 16:1483–9. doi: 10.4103/1673-5374.303012
51. Kourembanas S. Exosomes: vehicles of intercellular signaling, biomarkers, and vectors of cell therapy. Annu Rev Physiol. (2015) 77:13–27. doi: 10.1146/annurev-physiol-021014-071641
52. Tkach M, Théry C. Communication by extracellular vesicles: where we are and where we need to go. Cell. (2016) 164:1226–32. doi: 10.1016/j.cell.2016.01.043
53. Hayakawa K, Chan SJ, Mandeville ET, Park JH, Bruzzese M, Montaner J, et al. Protective Effects of Endothelial Progenitor Cell-Derived Extracellular Mitochondria in Brain Endothelium. Stem Cells. (2018) 36:1404–10. doi: 10.1002/stem.2856
54. Xing Z, Zhao C, Liu H, Fan Y. Endothelial progenitor cell-derived extracellular vesicles: a novel candidate for regenerative medicine and disease treatment. Adv Healthc Mater. (2020) 9:2000255. doi: 10.1002/adhm.202000255
55. Esquiva G, Grayston A, Rosell A. Revascularization and endothelial progenitor cells in stroke. Am J Physiol Cell Physiol. (2018) 315:C664–74. doi: 10.1152/ajpcell.00200.2018
56. Kietzmann T. Hypoxia-inducible erythropoietin expression: details matter. Haematologica. (2020) 105:2704–6. doi: 10.3324/haematol.2020.261966
57. Mojsilovic-Petrovic J, Callaghan D, Cui H, Dean C, Stanimirovic DB, Zhang W. Hypoxia-inducible factor-1 (HIF-1) is involved in the regulation of hypoxia-stimulated expression of monocyte chemoattractant protein-1 (MCP-1/CCL2) and MCP-5 (Ccl12) in astrocytes. J Neuroinflammation. (2007) 4:12. doi: 10.1186/1742-2094-4-12
58. de Francesco EM, Lappano R, Santolla MF, Marsico S, Caruso A, Maggiolini M. HIF-1α/GPER signaling mediates the expression of VEGF induced by hypoxia in breast cancer associated fibroblasts (CAFs). Breast Cancer Res. (2013) 15:bcr3458. doi: 10.1186/bcr3458
59. Li Y, Chang S, Li W, Tang G, Ma Y, Liu Y, et al. Cxcl12-engineered endothelial progenitor cells enhance neurogenesis and angiogenesis after ischemic brain injury in mice. Stem Cell Res Therapy. (2018) 9:1–15. doi: 10.1186/s13287-018-0865-6
60. Santo S, di Seiler S, Andres R, Widmer HR. Endothelial progenitor cells conditioned medium supports number of GABAergic neurons and exerts neuroprotection in cultured striatal neuronal progenitor cells. Cell Transplant. (2019) 28:367–78. doi: 10.1177/0963689719835192
61. Hill JM, Zalos G, Halcox JPJ, Schenke WH, Waclawiw MA, Quyyumi AA, et al. Circulating endothelial progenitor cells, vascular function, and cardiovascular risk. N Engl J Med. (2003) 348:593–600. doi: 10.1056/NEJMoa022287
62. Bitterli L, Afan S, Bühler S, Disanto S, Zwahlen M, Schmidlin K, et al. Endothelial progenitor cells as a biological marker of peripheral artery disease. Vasc Med. (2016) 21:3–11. doi: 10.1177/1358863X15611225
63. Hayek SS, MacNamara J, Tahhan AS, Awad M, Yadalam A, Ko YA, et al. Circulating progenitor cells identify peripheral arterial disease in patients with coronary artery disease. Circ Res. (2016) 119:564–71. doi: 10.1161/CIRCRESAHA.116.308802
64. Zhang ZG, Zhang L, Jiang Q, Chopp M. Bone marrow-derived endothelial progenitor cells participate in cerebral neovascularization after focal cerebral ischemia in the adult mouse. Circ Res. (2002) 90:284–8. doi: 10.1161/hh0302.104460
65. Ghani U, Shuaib A, Salam A, Nasir A, Shuaib U, Jeerakathil T, et al. Endothelial progenitor cells during cerebrovascular disease. Stroke. (2005) 36:151–3. doi: 10.1161/01.STR.0000149944.15406.16
66. Werner N, Nickenig G. Influence of cardiovascular risk factors on endothelial progenitor cells: limitations for therapy? Arterioscler Thromb Vasc Biol. (2006) 26:257–66. doi: 10.1161/01.ATV.0000198239.41189.5d
67. Deng Y, Wang J, He G, Qu F, Zheng M. Mobilization of endothelial progenitor cell in patients with acute ischemic stroke. Neurol Sci. (2018) 39:437–43. doi: 10.1007/s10072-017-3143-y
68. Brea D, Rodríguez-González R, Sobrino T, Rodríguez-Yañez M, Blanco M, Castillo J. Proteomic analysis shows differential protein expression in endothelial progenitor cells between healthy subjects and ischemic stroke patients. Neurol Res. (2011) 33:1057–63. doi: 10.1179/1743132811Y.0000000038
69. Bogoslovsky T, Spatz M, Chaudhry A, Maric D, Luby M, Frank J, et al. Stromal-derived factor-1[alpha] correlates with circulating endothelial progenitor cells and with acute lesion volume in stroke patients. Stroke. (2011) 42:618–25. doi: 10.1161/STROKEAHA.110.596007
70. Bogoslovsky T, Spatz M, Chaudhry A, Maric D, Luby M, Frank J, et al. Circulating CD133+CD34+ progenitor cells inversely correlate with soluble ICAM-1 in early ischemic stroke patients. J Transl Med. (2011) 9:145. doi: 10.1186/1479-5876-9-145
71. Sobrino T, Pérez-Mato M, Brea D, Rodríguez-Yáñez M, Blanco M, Castillo J. Temporal profile of molecular signatures associated with circulating endothelial progenitor cells in human ischemic stroke. J Neurosci Res. (2012) 90:1788–93. doi: 10.1002/jnr.23068
72. Xue L, Chen H, Zhang T, Chen J, Geng Z, Zhao Y. Changes in serum vascular endothelial growth factor and endostatin concentrations associated with circulating endothelial progenitor cells after acute ischemic stroke. Metab Brain Dis. (2017) 32:641–8. doi: 10.1007/s11011-017-9953-z
73. Li DW, Liu ZQ, Wei J, Liu Y, sen Hu L. Contribution of endothelial progenitor cells to neovascularization (review). Int J Mol Med. (2012) 30:1000–6. doi: 10.3892/ijmm.2012.1108
74. Morancho A, Ma F, Barceló V, Giralt D, Montaner J, Rosell A. Impaired vascular remodeling after endothelial progenitor cell transplantation in MMP9-deficient mice suffering cortical cerebral ischemia. J Cereb Blood Flow Metab. (2015) 35:1547–51. doi: 10.1038/jcbfm.2015.180
75. Sobrino T, Rodríguez-Yáñez M, Campos F, Iglesias-Rey R, Millán M, de la Ossa NP, et al. Association of high serum levels of growth factors with good outcome in ischemic stroke: a multicenter study. Transl Stroke Res. (2020) 11:653–63. doi: 10.1007/s12975-019-00747-2
76. Golab-Janowska M, Paczkowska E, Machalinski B, Kotlega D, Meller A, Safranow K, et al. Elevated inflammatory parameter levels negatively impact populations of circulating stem cells (CD133+), early endothelial progenitor cells (CD133+/VEGFR2+), and fibroblast growth factor in stroke patients. Curr Neurovasc Res. (2019) 16:19–26. doi: 10.2174/1567202616666190129164906
77. Åberg ND, Wall A, Anger O, Jood K, Andreasson U, Blennow K, et al. Circulating levels of vascular endothelial growth factor and post-stroke long-term functional outcome. Acta Neurol Scand. (2020) 141:405–14. doi: 10.1111/ane.13219
78. Martí-Fàbregas J, Crespo J, Delgado-Mederos R, Martínez-Ramírez S, Peña E, Marín R, et al. Endothelial progenitor cells in acute ischemic stroke. Brain Behav. (2013) 3:649. doi: 10.1002/brb3.175
79. Martí-Fàbregas J, Delgado-Mederos R, Crespo J, Peña E, Marín R, Jiménez-Xarrié E, et al. Circulating endothelial progenitor cells and the risk of vascular events after ischemic stroke. PLoS ONE. (2015) 10:0124895. doi: 10.1371/journal.pone.0124895
80. Sobrino T, Hurtado O, Moro MÁ, Rodríguez-Yáñez M, Castellanos M, Brea D, et al. The increase of circulating endothelial progenitor cells after acute ischemic stroke is associated with good outcome. Stroke. (2007) 38:2759–64. doi: 10.1161/STROKEAHA.107.484386
81. Chu K, Jung KH, Lee ST, Park HK, Sinn DI, Kim JM, et al. Circulating endothelial progenitor cells as a new marker of endothelial dysfunction or repair in acute stroke. Stroke. (2008) 39:1441–7. doi: 10.1161/STROKEAHA.107.499236
82. Yip HK, Chang LT, Chang WN, Lu CH, Liou CW, Lan MY, et al. Level and value of circulating endothelial progenitor cells in patients after acute ischemic stroke. Stroke. (2008) 39:69–74. doi: 10.1161/STROKEAHA.107.489401
83. Cesari F, Nencini P, Nesi M, Caporale R, Giusti B, Abbate R, et al. Bone marrow-derived progenitor cells in the early phase of ischemic stroke: relation with stroke severity and discharge outcome. J Cereb Blood Flow Metab. (2009) 29:1983–90. doi: 10.1038/jcbfm.2009.178
84. Bogoslovsky T, Chaudhry A, Latour L, Maric D, Luby M, Spatz M, et al. Endothelial progenitor cells correlate with lesion volume and growth in acute stroke. Neurology. (2010) 75:2059–62. doi: 10.1212/WNL.0b013e318200d741
85. Sargento-Freitas J, Aday S, Nunes C, Cordeiro M, Gouveia A, Silva F, et al. Endothelial progenitor cells enhance blood-brain barrier permeability in subacute stroke. Neurology. (2018) 90:e127–34. doi: 10.1212/WNL.0000000000004801
86. Navarro-Sobrino M, Rosell A, Hernandez-Guillamon M, Penalba A, Ribó M, Alvarez-Sabín J, et al. Mobilization, endothelial differentiation and functional capacity of endothelial progenitor cells after ischemic stroke. Microvasc Res. (2010) 80:317–23. doi: 10.1016/j.mvr.2010.05.008
87. Navarro-Sobrino M, Hernández-Guillamon M, Fernandez-Cadenas I, Ribó M, Romero IA, Couraud PO, et al. The angiogenic gene profile of circulating endothelial progenitor cells from ischemic stroke patients. Vasc Cell. (2013) 5:3. doi: 10.1186/2045-824X-5-3
88. Sargento-Freitas J, Aday S, Nunes C, Cordeiro M, Gouveia A, Silva F, et al. Endothelial Progenitor Cells influence acute and subacute stroke hemodynamics. J Neurol Sci. (2018) 385:119–25. doi: 10.1016/j.jns.2017.12.028
89. Hanna J, Hossain GS, Kocerha J. The potential for microRNA therapeutics and clinical research. Front Genet. (2019) 10:478. doi: 10.3389/fgene.2019.00478
90. Sobrino T, Arias S, Pérez-Mato M, Agulla J, Brea D, Rodríguez-Yáñez M, et al. Cd34+ progenitor cells likely are involved in the good functional recovery after intracerebral hemorrhage in humans. J Neurosci Res. (2011) 89:979–85. doi: 10.1002/jnr.22627
91. Paczkowska E, Gołab-Janowska M, Bajer-Czajkowska A, MacHalińska A, Ustianowski P, Rybicka M, et al. Increased circulating endothelial progenitor cells in patients with haemorrhagic and ischaemic stroke: the role of endothelin-1. J Neurol Sci. (2013) 325:90–9. doi: 10.1016/j.jns.2012.12.005
92. Piás-Peleteiro J, Pérez-Mato M, López-Arias E, Rodríguez-Yáñez M, Blanco M, Campos F, et al. Increased endothelial progenitor cell levels are associated with good outcome in intracerebral hemorrhage. Sci Rep. (2016) 6:28724. doi: 10.1038/srep28724
93. Rodríguez C, Sobrino T, Agulla J, Bobo-Jiménez V, Ramos-Araque ME, Duarte JJ, et al. Neovascularization and functional recovery after intracerebral hemorrhage is conditioned by the Tp53 Arg72Pro single-nucleotide polymorphism. Cell Death Differ. (2017) 24:144–54. doi: 10.1038/cdd.2016.109
94. Moubarik C, Guillet B, Youssef B, Codaccioni JL, Piercecchi MD, Sabatier F, et al. Transplanted late outgrowth endothelial progenitor cells as cell therapy product for stroke. Stem Cell Rev Rep. (2011) 7:208–20. doi: 10.1007/s12015-010-9157-y
95. Rosell A, Morancho A, Navarro-Sobrino M, Martínez-Saez E, Hernández-Guillamon M, Lope-Piedrafita S, et al. Factors secreted by endothelial progenitor cells enhance neurorepair responses after cerebral ischemia in mice. PLoS ONE. (2013) 8:73244. doi: 10.1371/journal.pone.0073244
96. Garrigue P, Hache G, Bennis Y, Brige P, Stalin J, Pellegrini L, et al. Erythropoietin pretreatment of transplanted endothelial colony-forming cells enhances recovery in a cerebral ischemia model by increasing their homing ability: a SPECT/CT study. J Nucl Med. (2016) 57:1798–804. doi: 10.2967/jnumed.115.170308
97. Hong Y, Yu Q, Kong Z, Wang M, Zhang R, Li Y, et al. Exogenous endothelial progenitor cells reached the deficient region of acute cerebral ischemia rats to improve functional recovery via Bcl-2. Cardiovasc Diagn Ther. (2020) 10:695–704. doi: 10.21037/cdt-20-329
98. Wang M, Li Y, Zhang R, Zhang S, Feng H, Kong Z, et al. Adiponectin-transfected endothelial progenitor cells have protective effects after 2-hour middle-cerebral artery occlusion in rats with type 2 diabetes mellitus. Front Neurol. (2021) 12:630681. doi: 10.3389/fneur.2021.630681
99. Kadir RRA, Alwjwaj M, Bayraktutan U. Treatment with outgrowth endothelial cells protects cerebral barrier against ischemic injury. Cytotherapy. (2022) 24:489–99. doi: 10.1016/j.jcyt.2021.11.005
100. Fang J, Guo Y, Tan S, Li Z, Xie H, Chen P, et al. Autologous endothelial progenitor cells transplantation for acute ischemic stroke: a 4-year follow-up study. Stem Cells Transl Med. (2019) 8:14–21. doi: 10.1002/sctm.18-0012
101. Maki T, Morancho A, Segundo PMS, Hayakawa K, Takase H, Liang AC, et al. Endothelial progenitor cell secretome and oligovascular repair in a mouse model of prolonged cerebral hypoperfusion. Stroke. (2018) 49:1003–10. doi: 10.1161/STROKEAHA.117.019346
102. Wang J, Chen S, Zhang W, Chen Y, Bihl JC. Exosomes from miRNA-126-modified endothelial progenitor cells alleviate brain injury and promote functional recovery after stroke. CNS Neurosci Ther. (2020) 26:1255–65. doi: 10.1111/cns.13455
103. Lee ST, Chu K, Jung KH, Ko SY, Kim EH, Sinn DI, et al. Granulocyte colony-stimulating factor enhances angiogenesis after focal cerebral ischemia. Brain Res. (2005) 1058:120–8. doi: 10.1016/j.brainres.2005.07.076
104. Pellegrini L, Bennis Y, Guillet B, Velly L, Garrigue P, Sabatier F, et al. Therapeutic benefit of a combined strategy using erythropoietin and endothelial progenitor cells after transient focal cerebral ischemia in rats. Neurol Res. (2013) 35:937–47. doi: 10.1179/1743132813Y.0000000235
105. Wang ML, Zhang LX, Wei JJ, Li LL, Zhong WZ, Lin XJ, et al. Granulocyte colony-stimulating factor and stromal cell-derived factor-1 combination therapy: a more effective treatment for cerebral ischemic stroke. Int J Stroke. (2020) 15:743–54. doi: 10.1177/1747493019879666
106. Dong XH, Peng C, Zhang YY, Jiang Y, Yang LJ, He JB, et al. Low-dose piperlongumine rescues impaired function of endothelial progenitor cells and reduces cerebral ischemic injury in high-fat diet-fed mice. Front Pharmacol. (2021) 12:689880. doi: 10.3389/fphar.2021.689880
107. Schäbitz WR, Laage R, Vogt G, Koch W, Kollmar R, Schwab S, et al. AXIS: a trial of intravenous granulocyte colony-stimulating factor in acute ischemic stroke. Stroke. (2010) 41:2545–51. doi: 10.1161/STROKEAHA.110.579508
108. Alasheev AM, Belkin AA, Leiderman IN, Ivanov RA, Isakova TM. Granulocyte-colony-stimulating factor for acute ischemic stroke: a randomized controlled trial (STEMTHER). Transl Stroke Res. (2011) 2:358–65. doi: 10.1007/s12975-011-0091-3
109. Floel A, Warnecke T, Duning T, Lating Y, Uhlenbrock J, Schneider A, et al. Granulocyte-colony stimulating factor (G-CSF) in stroke patients with concomitant vascular disease–a randomized controlled trial. PLoS ONE. (2011) 6:19767. doi: 10.1371/journal.pone.0019767
110. Sobrino T, Rodríguez-González R, Blanco M, Brea D, Pérez-Mato M, Rodríguez-Yáñez M, et al. CDP-choline treatment increases circulating endothelial progenitor cells in acute ischemic stroke. Neurol Res. (2011) 33:572–7. doi: 10.1179/016164110X12807570510176
111. Ringelstein EB, Thijs V, Norrving B, Chamorro A, Aichner F, Grond M, et al. Granulocyte colony-stimulating factor in patients with acute ischemic stroke: results of the AX200 for Ischemic Stroke trial. Stroke. (2013) 44:2681–7. doi: 10.1161/STROKEAHA.113.001531
112. Mizuma A, Yamashita T, Kono S, Nakayama T, Baba Y, Itoh S, et al. Phase II trial of intravenous low-dose granulocyte colony-stimulating factor in acute ischemic stroke. J Stroke Cerebrovasc Dis. (2016) 25:1451–7. doi: 10.1016/j.jstrokecerebrovasdis.2016.01.022
113. Zhang R, Yang J, Yuan J, Song B, Wang Y, Xu Y. The therapeutic value of bone marrow-derived endothelial progenitor cell transplantation after intracerebral hemorrhage in rats. Front Neurol. (2017) 8:174. doi: 10.3389/fneur.2017.00174
114. Carenza E, Barceló V, Morancho A, Levander L, Boada C, Laromaine A, et al. In vitro angiogenic performance and in vivo brain targeting of magnetized endothelial progenitor cells for neurorepair therapies. Nanomed Nanotechnol Biol Med. (2014) 10:225–34. doi: 10.1016/j.nano.2013.06.005
115. Moniche F, Gonzalez A, Gonzalez-Marcos JR, Carmona M, Piñero P, Espigado I, et al. Intra-arterial bone marrow mononuclear cells in ischemic stroke: a pilot clinical trial. Stroke. (2012) 43:2242–4. doi: 10.1161/STROKEAHA.112.659409
116. Banerjee S, Bentley P, Hamady M, Marley S, Davis J, Shlebak A, et al. Intra-arterial immunoselected CD34+ stem cells for acute ischemic stroke. Stem Cells Transl Med. (2014) 3:1322. doi: 10.5966/sctm.2013-0178
117. Sung PH, Lin HS, Lin WC, Chang CC, Pei SN, Ma MC, et al. Intra-carotid arterial transfusion of autologous circulatory derived CD34+ cells for old ischemic stroke patients- a phase I clinical trial to evaluate safety and tolerability. Am J Transl Res. (2018) 10:2975–89.
118. Loiola RA, García-Gabilondo M, Grayston A, Bugno P, Kowalska A, Duban-Deweer S, et al. Secretome of endothelial progenitor cells from stroke patients promotes endothelial barrier tightness and protects against hypoxia-induced vascular leakage. Stem Cell Res Ther. (2021) 12:552. doi: 10.1186/s13287-021-02608-y
119. Ward NL, LaManna JC. The neurovascular unit and its growth factors: coordinated response in the vascular and nervous systems. Neurol Res. (2004) 26:870–83. doi: 10.1179/016164104X3798
120. Ohab JJ, Fleming S, Blesch A, Carmichael ST. A neurovascular niche for neurogenesis after stroke. J Neurosci. (2006) 26:13007–16. doi: 10.1523/JNEUROSCI.4323-06.2006
121. Yamashita T, Ninomiya M, Acosta PH, García-Verdugo JM, Sunabori T, Sakaguchi M, et al. Subventricular zone-derived neuroblasts migrate and differentiate into mature neurons in the post-stroke adult striatum. J Neurosci. (2006) 26:6627–36. doi: 10.1523/JNEUROSCI.0149-06.2006
122. Roitbak T, Li L, Cunningham LA. Neural stem/progenitor cells promote endothelial cell morphogenesis and protect endothelial cells against ischemia via HIF-1alpha-regulated VEGF signaling. J Cereb Blood Flow Metab. (2008) 28:1530–42. doi: 10.1038/jcbfm.2008.38
123. Zhou S, Gao B, Sun C, Bai Y, Cheng D, Zhang Y, et al. Vascular endothelial cell-derived exosomes protect neural stem cells against ischemia/reperfusion injury. Neuroscience. (2020) 441:184–96. doi: 10.1016/j.neuroscience.2020.05.046
124. Jing J, Jiang H, Zhang L. Endothelial progenitor cells promote neural stem cell proliferation in hypoxic conditions through VEGF via the PI3K/AKT pathway. J Recept Signal Transduct Res. (2022) 18:1–7. doi: 10.1080/10799893.2021.2019275
125. Zhou N, Wang L, Fu P, Cui Z, Ge Y, Jiang F, et al. Conditioned medium-preconditioned EPCs enhanced the ability in oligovascular repair in cerebral ischemia neonatal rats. Stem Cell Res Ther. (2021) 12:118. doi: 10.1186/s13287-021-02157-4
126. Miyamoto N, Pham LDD, Seo JH, Kim KW, Lo EH, Arai K. Crosstalk between cerebral endothelium and oligodendrocyte. Cell Mol Life Sci. (2014) 71:1055. doi: 10.1007/s00018-013-1488-9
127. Wang J, Chen S, Ma X, Cheng C, Xiao X, Chen J, et al. Effects of endothelial progenitor cell-derived microvesicles on hypoxia/reoxygenation-induced endothelial dysfunction and apoptosis. Oxid Med Cell Longev. (2013) 2013:572729. doi: 10.1155/2013/572729
128. Wang J, Liu H, Chen S, Zhang W, Chen Y, Yang Y, et al. Moderate exercise has beneficial effects on mouse ischemic stroke by enhancing the functions of circulating endothelial progenitor cell-derived exosomes. Exp Neurol. (2020) 330:113325. doi: 10.1016/j.expneurol.2020.113325
129. Halurkar MS, Wang J, Chen S, Bihl JC. EPC-EXs improve astrocyte survival and oxidative stress through different uptaking pathways in diabetic hypoxia condition. Stem Cell Res Therapy. (2022) 13:1–10. doi: 10.1186/s13287-022-02766-7
130. Ma X, Wang J, Li J, Ma C, Chen S, Lei W, et al. Loading MiR-210 in endothelial progenitor cells derived exosomes boosts their beneficial effects on hypoxia/reoxygeneation-injured human endothelial cells via protecting mitochondrial function. Cell Physiol Biochem. (2018) 46:664–75. doi: 10.1159/000488635
131. Sobrino T, Blanco M, Pérez-Mato M, Rodríguez-Yáñez M, Castillo J. Increased levels of circulating endothelial progenitor cells in patients with ischaemic stroke treated with statins during acute phase. Eur J Neurol. (2012) 19:1539–46. doi: 10.1111/j.1468-1331.2012.03770.x
132. Golab-Janowska M, Paczkowska E, Machalinski B, Meller A, Kotlega D, Safranow K, et al. Statins therapy is associated with increased populations of early endothelial progenitor (CD133+/VEGFR2+) and Endothelial (CD34-/CD133- /VEGFR2+) cells in patients with acute ischemic stroke. Curr Neurovasc Res. (2018) 15:120–8. doi: 10.2174/1567202615666180611120546
133. Lazarus HM, Gale RP. G-CSF and GM-CSF are different. Which one is better for COVID-19? Acta Haematol. (2021) 144:355–9. doi: 10.1159/000510352
134. Ushach I, Zlotnik A. Biological role of granulocyte macrophage colony-stimulating factor (GM-CSF) and macrophage colony-stimulating factor (M-CSF) on cells of the myeloid lineage. J Leukoc Biol. (2016) 100:481–9. doi: 10.1189/jlb.3RU0316-144R
135. Natori T, Sata M, Washida M, Hirata Y, Nagai R, Makuuchi M. G-CSF stimulates angiogenesis and promotes tumor growth: potential contribution of bone marrow-derived endothelial progenitor cells. Biochem Biophys Res Commun. (2002) 297:1058–61. doi: 10.1016/S0006-291X(02)02335-5
Keywords: angiogenesis, cell therapy, endothelial progenitor cells, secretome, stroke, vasculogenesis, cerebral ischemia
Citation: Custodia A, Ouro A, Sargento-Freitas J, Aramburu-Núñez M, Pías-Peleteiro JM, Hervella P, Rosell A, Ferreira L, Castillo J, Romaus-Sanjurjo D and Sobrino T (2022) Unraveling the potential of endothelial progenitor cells as a treatment following ischemic stroke. Front. Neurol. 13:940682. doi: 10.3389/fneur.2022.940682
Received: 10 May 2022; Accepted: 15 August 2022;
Published: 08 September 2022.
Edited by:
Ken Arai, Massachusetts General Hospital and Harvard Medical School, United StatesReviewed by:
Nunzio Vicario, University of Catania, ItalySavneet Kaur, The Institute of Liver and Biliary Sciences (ILBS), India
Yongting Wang, Shanghai Jiao Tong University, China
Copyright © 2022 Custodia, Ouro, Sargento-Freitas, Aramburu-Núñez, Pías-Peleteiro, Hervella, Rosell, Ferreira, Castillo, Romaus-Sanjurjo and Sobrino. This is an open-access article distributed under the terms of the Creative Commons Attribution License (CC BY). The use, distribution or reproduction in other forums is permitted, provided the original author(s) and the copyright owner(s) are credited and that the original publication in this journal is cited, in accordance with accepted academic practice. No use, distribution or reproduction is permitted which does not comply with these terms.
*Correspondence: Daniel Romaus-Sanjurjo, ZGFuaWVsLnJvbWF1cy5zYW5qdXJqbyYjeDAwMDQwO3Nlcmdhcy5lcw==; Tomás Sobrino, dG9tYXMuc29icmluby5tb3JlaXJhcyYjeDAwMDQwO3Nlcmdhcy5lcw==