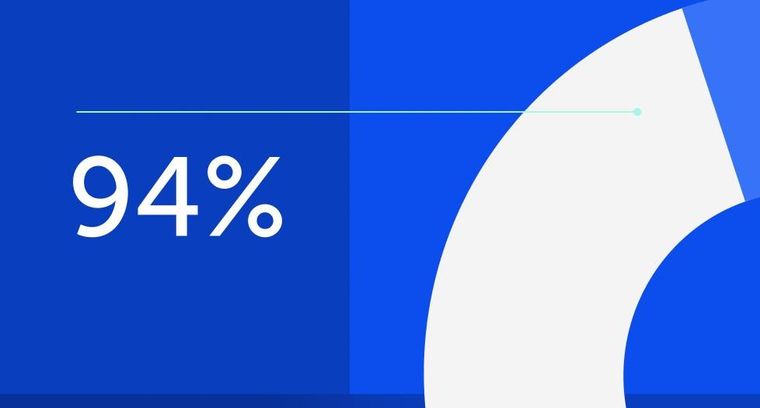
94% of researchers rate our articles as excellent or good
Learn more about the work of our research integrity team to safeguard the quality of each article we publish.
Find out more
REVIEW article
Front. Neurol., 07 September 2022
Sec. Dementia and Neurodegenerative Diseases
Volume 13 - 2022 | https://doi.org/10.3389/fneur.2022.929781
This article is part of the Research TopicAdvances in Integrative Medicine for Neurodegenerative Diseases: From Basic Research to Clinical PracticeView all 11 articles
Psychiatric and neurodegenerative diseases, including major depression disorder (MDD), bipolar disorder, and Alzheimer's disease, are a burden to society. Deficits of adult hippocampal neurogenesis (AHN) have been widely considered the main hallmark of psychiatric diseases as well as neurodegeneration. Herein, exploring applicable targets for improving hippocampal neural plasticity could provide a breakthrough for the development of new treatments. Emerging evidence indicates the broad functions of mitochondria in regulating cellular behaviors of neural stem cells, neural progenitors, and mature neurons in adulthood could offer multiple neural plasticities for behavioral modulation. Normalizing mitochondrial functions could be a new direction for neural plasticity enhancement. Exercise, a highly encouraged integrative method for preventing disease, has been indicated to be an effective pathway to improving both mitochondrial functions and AHN. Herein, the relative mechanisms of mitochondria in regulating neurogenesis and its effects in linking the effects of exercise to neurological diseases requires a systematic summary. In this review, we have assessed the relationship between mitochondrial functions and AHN to see whether mitochondria can be potential targets for treating neurological diseases. Moreover, as for one of well-established alternative therapeutic approaches, we summarized the evidence to show the underlying mechanisms of exercise to improve mitochondrial functions and AHN.
In recent decades, psychiatric or neurodegenerative diseases have attracted increased attention due to the growing number of patients. Psychiatric diseases such as depression disorder (MDD) and bipolar disorder usually see patients suffering from anxiety or depressive moods and changes in physical and emotional reactions that would be exacerbated by even minor environmental changes (1, 2). As for neurodegenerative diseases such as Alzheimer's disease (AD) and Parkinson's disease (PD), the progressive death of neurons commonly induces irreversible neuronal cognitive deficits, motor disability, and complex behavioral dysregulation (3). The unidentified etiology of those diseases strongly limited the development of drugs to prevent the progress of behavioral abnormality. From the angle of symptomatic treatment, it is urgent and necessary to explore supplementary or alternative medicine for improving brain functions.
Neural plasticity provides the ability for the central nervous system (CNS) to adapt to environmental challenges under physiological and pathological conditions (4). In the adult hippocampus, neural plasticity refers to neurogenesis and synaptic plasticity, both of which perform critical roles in regulating emotional and cognitive behaviors. Adult neurogenesis was widely considered as a structural plasticity through its regulation of the neuronal population in certain brain regions. As for the target of alternative or integrative medicine, improving hippocampal neurogenesis could serve as a key therapeutic paradigm against neurological disorders without a clear pathological mechanism (5–7). For this reason, evaluating the mechanism of adult neurogenesis could help explore an applicable pathway to treat neurological diseases.
Emerging evidence indicates mitochondria have a key function in regulating the activity and fate commitment of stem cells (8). In addition, mitochondria have been recognized as key mediators in response to development of neurological disease (9). Physical exercise is an effective way to prevent chronic diseases, including diabetes, neurodegeneration, and psychiatric disorders (10, 11). Through metabolic regulating, exercise is beneficial to mitochondrial functions. It is noteworthy that mitochondria could be the linker between exercise and neurogenesis. Given this, it is necessary to summarize the effects of mitochondria in neural functions and its roles in disease development. In this review, we summarized the functions of mitochondria to regulate adult hippocampal neurogenesis and its potential regulators. Furthermore, we discussed the linkage role of mitochondria to bridge physical exercise and brain functional improvement.
Adult neurogenesis is a temporal-spatial progress composed by the self-renewal fate commitment of neural stem cells (NSCs) as well as the maturation of neural progenitor cells (NPCs). In the hippocampus, neurogenesis provides the regenerative resources to clear panic memory (12, 13). The enhancement of hippocampal neurogenesis was shown to promote pattern separation behavior, which enables animals to discriminate between environmental cues related to stress experience (14). While declined hippocampal neurogenesis commonly results in an elevated fear response, which subsequently manifests as inappropriate, uncontrollable expression of fear in neutral and safe environments (15). These documents highlight the critical role of AHN in regulating antidepressant behaviors. The critical role of hippocampal neurogenesis in depressive moods could also be seen in an animal model of seizures, which was demonstrated to be triggered by antidepressants (16). The seizures animal model showed abnormal increase of adult neurogenesis with upregulated immature neuronal numbers in the hippocampal DG region (17). Another type of neural plasticity besides neurogenesis is synaptic plasticity, which includes synaptogenesis as well as synaptic functions like long-term potentiation (LTP) and pre-synaptic plasticity. Dysregulation of synaptic plasticity was also shown to be related to the development of neurological disorders. Immobilization-stressed mice presented intensified fear memory and enhanced long-term potentiation (LTP) (18). In terms of synaptic plasticity, adult neurogenesis can provide a regenerative resource to prevent the neurodegenerative progress and simultaneously enhance the ability in emotional regulation (19). Promoting the AHN was documented as an effective approach against psychiatric disorders, particularly depression. In vivo calcium imaging to record neuronal activity in the vDG (ventral dentate gyrus) demonstrated increased neurogenesis correlated to decreased activity of stress-responsive cells, which are active during attacks or while mice explore anxiogenic environments (20). Through conditional knockout of the Bcl-gene in NSCs, Sahay et al. established that there is enhanced AHN in mice and found that improving AHN was sufficient to prevent behavioral dysfunctions in a depression model (21). Additionally, blocking AHN with temozolomide (TMZ) could also result in the comprised therapeutic effects of antidepressants such as SSRIs (selective serotonin reuptake inhibitors) and ketamine (22, 23). Thus, exploring factors in regulating ANH would offer the new drug targets for treating neurological diseases.
Biological regulation of mitochondria involves multiple aspects, including their metabolism, biogenesis, fission, and fusion dynamics and degradation via autophagy. Accumulating evidence has been reported to show that all these biological events participate in the regulation of AHN at different levels or conditions (Table 1). Cell metabolism plays a fundamental role in multiple biological events, including energy supply, cell growth, differentiation, and death. During the self-renewal and differentiation process, stem cells undergo a dramatic metabolic reprogram. At an adult hippocampus, the metabolism pattern of NSCs undergoes the switch from glycolysis to oxidative phosphorylation (OXPHOS) following the process of neuronal differentiation (34). In mature neurons, mitochondrial OXPHOS provides high amounts of energy to meet the requirement of neuronal electrophysiological activities (35, 36). Numerous mitochondrial mediators could be applied as therapeutic targets not only for metabolic regulating but also to improve AHN.
Mitochondria have been primarily identified as cellular organelles that provide energy. In neurons, mitochondrial dysfunction is reported to be involved in multiple neurodegenerative or psychiatric diseases (37, 38). Dysregulated AHN induced by abnormal mitochondrial function is one of the main reasons to these diseases. According to the environmental changes, quiescent NSCs in the hippocampus are undergoing extensive changes along with proliferative activity, cellular growth, and synaptic growth. Adult NSCs display astroglia features, including 100% GFAP expression, as well as glycolytic cellular metabolisms pattern (39). Following neurogenesis, mature neurons require high amounts of ATP for their biological functions, such as presynaptic vesicle recycling. Mature neurons integrated in neural circuits are highly dependent on the mitochondrial electron transport chain (ETC) and OXPHOS (40–42). Single cell transcriptomics shows the dramatic upregulating profile of OXPHOS-related genes during the neural lineage commitment of hippocampal NSCs. Moreover, specific ablation of mitochondrial transcription factor A (Tfam) in adult NSCs reproduces multiple hallmarks of aging in the hippocampus, including declined neurogenesis. Such alteration could be reversed by pharmacological enhancement of mitochondrial function (34). The evidence suggests mitochondrial metabolism has a critical role in regulating hippocampal neurogenesis and relative physiological process. Suppressing mitochondrial OXPHOS could also affect the other types of adult stem cells. In hematopoietic stem cell (HSCs), deleting PTEN-like mitochondrial phosphatase Ptpmt1 could lead to defective hematopoiesis with impaired differentiation of HSCs (43). Additionally, the metabolic pattern of cells could shift from mitochondrial OXPHOS to glycolysis during the reprogramming process of the inducible pluripotent stem cells (iPSCs), indicating that mitochondria also act critically in embryonic stem cells (44). Generally, switching of mitochondrial function is commonly associated with the energetic demands of stem cells to meet the requirement of their self-renewal or differentiation. Most neurological drugs that are widely used in clinic reportedly have an effect on metabolic regulation. Indeed, mitochondria are widely reported as the target for improving brain functions. The brain functional recovery drug piracetam was documented to prevent declined neurogenesis via promoting mitochondrial metabolism in an aging model (34, 45). Antioxidants could also reserve the functions of mitochondria (46). However, it is noteworthy that exercise may elevate the level of radial oxidative species (ROS), which has been recently declared as a mechanism in antidiabetic effects (47). Since antioxidant and exercise can provide effects similar to those of mitochondria, a certain level of ROS might serve as the “second messenger” to promote the fate commitment of the NSCs at the physiological level (48). Hence, exercise could be an effective way to control the level of ROS into physiological reasonable by promoting mitochondrial OXPHOS.
Mitochondria are constantly varying between being fragmented or filamentous networks to adapt to the requirements of cellular functions. According to different energetic demands of the stem cells stage between self-renewal and differentiation, dynamics alterations of mitochondrial morphology are critical in regulating stemness (49). Mitochondrial elongation commonly occurs in aging skeletal muscle cells with increased mitochondrial fusion protein MFN1/2 and the accumulated mutation of mitochondrial DNA (50). Following the neural commitment of NSCs, mitochondria shift from the elongated morphology to fragmentation. On the cell metabolic level, increasing mitochondrial fragments are associated with enhanced OXPHOS and production of ROS, previously mentioned as the second messenger to stimulate downstream signaling like NRF2 and downregulate Notch1 for lineage commitment determination (48). Sirtuins were also considered as regulators to link mitochondrial dynamics with adult neurogenesis (25). Physical exercise, the well-known upregulator of SIRT3 and lipid metabolism, could enhance adult neurogenesis in an unpredictable chronic stress depression model (51). Hypoxia inducible factor (HIF) signaling also provides the link between oxygen levels and mitochondrial dynamics (52, 53). The activation of the HIF complex under hypoxia ensures that energy demands meet pathological conditions by increasing levels of glycolytic enzymes and inhibiting oxygen consumption (54). Such mechanisms also mediate self-derived neural repair under stress. In the hypoxia condition, activation of HIF induces NSCs proliferation and switched their migration in subventricular zone, which promotes regenerative progress in infarction region (55). HIF deletion, however, can impair the AHN and induce learning and memory deficit (56). Therefore, mitochondrial dynamics-mediated redox/oxidative status plays a key role in regulating AHN.
In starvation conditions, autophagy could be rapidly activated to provides a cell with nutrients to survive (57). The selective autophagy of mitochondria, also known as mitophagy, can be processed such that damaged or unwanted mitochondria require degradation (58). As differentiation of stem cells involves extensive cellular remodeling, autophagy ensures the elimination of unnecessary cellular components to maintain an optimal cellular status. It was demonstrated that pretreatment of antioxidant N-acetylcysteine (NAC) attenuated oxidative stress-induced NSCs' self-renewal disruption by suppressing autophagy signaling mTOR and decreased LC3B-II protein expression (59). In contrast, enhancing autophagy in aged satellite cells prevented the senescence and restored regenerative properties (60). Herein, it could hypothesize that mitochondrial morphology is another effect pathway to regulate mitochondrial dynamics in NSCs. At a physiological level, certain levels of mitophagy might be necessary for controlling the differentiation of adult NSCs. However, there is no systematic evidence that indicates the exact mechanisms of mitophagy to regulate the differentiation and self-renewal of adult NSCs.
At the cell level, mitochondrial alterations could be regarded as a hallmark for stem cell differentiation. Consistently, impairment of AHN is a well-established biological hallmark of psychiatric diseases and neurodegeneration at the tissue level. Such a relationship indicates that mitochondria could perform be a therapeutic target for neurological diseases. An increasing number of clinical reports have demonstrated substantial mitochondrial damage could contribute to the development of depression and cognitive impairments. Deletion of mtDNA in a child was associated with mitochondrial disease symptoms and mild–moderate unipolar depression (61). Blood sample measurement of mtDNA in bipolar disorder (BD) and MDD patients also showed a lower mtDNA copy number than in controls (62). Another report demonstrated a significant reduction of mtDNA copy numbers in combat PTSD (63), indicating mtDNA or the mitochondrial mass abnormality could be the general phenomena correlated with psychiatric diseases. On the other hand, mitochondria perform as the therapeutic target to psychiatric diseases. SSRIs (selective serotonin reuptake inhibitors) like the antidepressant fluoxetine could promote mitophagy by increasing colocalization of autophagosomes and mitochondria, which thereby eliminates damaged mitochondria in corticosterone-treated astrocytes (64). McCoy et al. compared high novelty responder rats (HRs), which show highly exploratory behavior in a novel environment as well as remarkable resilience to chronic mild stress, and low novelty responder rats (LRs), which are susceptible to chronic stress. They observed that LRs displayed higher cytochrome c oxidase (COX) activity in the dentate gyrus, prefrontal cortex, and dorsal raphe compared to HRs (65). Apart from selected brain regions, a declining skeletal muscle mitochondrial function in aging adults was also shown to be associated with clinically significant depressive symptoms (66). These lines of evidence support the critical regulatory roles of mitochondria in antidepressant functions.
Environmental factors could also induce psychiatric diseases via affecting mitochondrial functions. Glombik et al. reported that maternal stress leads to depression-like behaviors in the offspring of rats; they displayed brain mitochondrial abnormalities, including significant downregulation of Ndufv2 (complex I) (67). Animal studies have suggested that mitochondrial abnormalities were augmented by stress, indicating mitochondria are stress-response modulators and contribute to the stress-induced pathophysiology of psychiatric diseases (68). A possible mechanism might be an enhanced requirement of the neural activity during learning or memory coding, which could induce increased mitochondrial respiration and thereby produce more metabolic products to influence the signaling pathways downstream, e.g., ROS and RNS.
Accumulating evidence suggests that improving mitochondrial functions could help the treatment of neurodegeneration (69, 70). The complex I inhibitor rotenone could be utilized as a PD model for drug development (71). The impairment of complex I was associated with reduced ATP levels, oxidative stress, and calcium-mediated damage in such a pathological model (72). In post-mortem tissue of sporadic AD, scientists found mitochondrial dysfunction is correlated with decreased levels of ATP (73). Growing evidence indicated the medications targeting on mitochondria exert the therapeutic effects to neurodegenerative. Metformin, a type-2 diabetes drug approved by the FDA, was shown to enhance adult neurogenesis and showed promising effects on an animal model of AD and PD (74, 75). Another example is the glycogen-like peptide- (GLP-1) analog. It has been reported that the GLP-1 analog could promote adult neurogenesis and attenuate the behavioral dysfunctions in neurodegenerative disorders including PD and AD (76, 77). Herein, improving mitochondrial functions could also result in protective effects against neurodegeneration.
Alternative and integrative medicine are increasingly proposed as effective strategies to treat psychiatric and neurodegenerative disorders. Due to safety concerns regarding the tolerability and risk of medications (78), an effective alternative therapy is highly requested to attenuate the behavioral disorders. As for neurodegeneration, early prevention of the diseases is currently the most effective strategy due to the limited effects of drugs to halt or prevent the progress of the neuron death. Physical exercise is widely recognized as being part of a healthy lifestyle partly due to its promotion of the maintenance of lifelong mitochondrial quality control (79). Exercise has been increasingly reported for its improvement of adult neurogenesis in both physiological and pathological conditions (80–82). Exercise improves mitochondrial functions via its multiple biological effects. It was demonstrated that exercise promoted the production of brain-derived neurotrophic factor (BDNF) levels and could alter mitochondrial function, neuroplasticity, and the rate of apoptosis in the hippocampus and thereby prevented the occurrence of PTSD (83). In a maternal separation depression model, exercise could alter mitochondrial function, serotonin levels, and the rate of apoptosis (84). Herein, mitochondrial functions perform as the linkage between exercise and its neuroprotective effects.
In aged mice, physical exercise significantly increased DRP1 protein levels and elevated the rates of respiration and ROS production in mitochondria, which is suggestive of its potential in improving brain functions via its regulating mitochondrial electron transport chain function and dynamics (85). In an animal model of Alzheimer's disease, 1 h of swimming exercise for 6 days/week consolidated the intact of mitochondrial cristae and edges, raised the brain ATP production as well as the number of synapses by regulating the expression of GLUT1 and GLUT3 expression levels (86). Antidepressant action of running was highly correlated with an increase of hippocampal neurogenesis and plasticity (81). Compared with its promotion of NSCs' proliferation, the accelerating effects of exercise have a longer latency period (about 2 weeks) on the maturation of new neurons (87). Moreover, structural magnetic resonance imaging suggested hippocampus and brain cortex growth in schizophrenia patients and healthy controls after the endurance aerobic physical training. This evidence indicates exercise can also serve as a promising candidate for pathophysiology-based add-on interventions for schizophrenia (88). A recent study indicates that free wheel running could promote the activation of the quiescent NSCs in the hippocampus by regulating cellular ROS level (89). Therefore, exercise could engage broad effects of neural functions via multiple molecular mechanisms.
Exercise could exert multiple biological effects in additional to its roles in mitochondrial functions. Brain inflammation is another key target of exercise for neural tissue. A recent study showed the systematic regulatory mechanism of exercise influenced adult neurogenesis. Injecting plasma derived from voluntary running mice resulted in elevated density of hippocampal DCX+ neurons correlating with improved working memory, which were shown to rely on the inflammatory regulation via clusterin (90). This report further suggested the effects of exercise mediated AHN may depend on its effects on the peripheral circulation system. It was indicated that LPS could reduce the number of new neurons in aged but not adult mice, while such dysfunctions could be prevented by free wheel running (91). Exercise could also attenuate the inflammatory response in subjects with depression. A study on 61 university students assigned to 6 weeks of different models of exercise including high-intensity interval training (HIT), moderate continuous training (MCT), or no exercise (CON) suggested that MCT exercise could have a positive effect on the promotion of mental health by decreasing TNF-α level (92). Neuroinflammation has been suggested to negatively affect adult neurogenesis, and physical exercise could promote AHN by buffering the inflammation response in neural tissue (93). The activation of microglia mediated the proinflammatory factors, including interleukin-6, TNF-α, ROS, and nitric oxide, which all have anti-neurogenic properties (94). Table 2 summarizes the recent evidence in support of the effects of exercise, showing different patterns of mitochondrial biology as well as neuronal functions (Table 2). However, limited evidence has shown the possible role of mitochondria during exercise and their ability to mediate the functions of neural tissue, particularly adult NSCs.
Mitochondria are key organelles in the mediation of energy functions. Based on this mechanism, recent studies have demonstrated that mitochondria mediate multiple cellular behaviors that are far beyond energy supply, e.g., the fate commitment and proliferation of somatic stem cells as well as the reprogramming and differentiation process of pluripotent stem cells (104, 105). Improving mitochondrial function has also been considered a therapeutic strategy against neurological diseases. Therapeutic approaches targeting mitochondria should focus on future pre-clinical exploration for treating neurodegenerative and psychiatric disorders. Mitochondria play the critical roles in regulating stem cell behaviors including self-renew and fate commitment of the adult NSCs (Figure 1). Therefore, a systematic strategy to improve mitochondrial functions throughout the body is preferable; we should not only promote neuronal regeneration but also focus on regulating the NSCs environment, including the peripheral factors and the neurogenic niche. With such requirements, exercise is the ideal option, accompanied as it is by considerable healing effects and relatively few safety issues.
Figure 1. Exercise promotes adult neurogenesis via mitochondria. Physical exercise could enhance mitochondrial functions in adult neural stem cells. Exercise promotes the fission of mitochondria, biogenesis, and OXPHOS metabolism. These alterations result in the fate determination of NSCs from self-renewal to neural commitment, which thereby promotes adult neurogenesis in both physiological and pathological conditions.
All authors listed have made a substantial, direct, and intellectual contribution to the work and approved it for publication.
This work was supported by National Natural Science Foundation of China (32000835), and Open Research Fund of the State Key Laboratory of Cognitive Neuroscience and Learning (CNLZD2104).
The authors declare that the research was conducted in the absence of any commercial or financial relationships that could be construed as a potential conflict of interest.
All claims expressed in this article are solely those of the authors and do not necessarily represent those of their affiliated organizations, or those of the publisher, the editors and the reviewers. Any product that may be evaluated in this article, or claim that may be made by its manufacturer, is not guaranteed or endorsed by the publisher.
1. Cohen JA, Deblinger E, Mannarino AP, Steer RA. A multisite, randomized controlled trial for children with sexual abuse-related PTSD symptoms. J Am Acad Child Adolesc Psychiatry. (2004) 43:393–402. doi: 10.1097/00004583-200404000-00005
2. Raskind MA, Peskind ER, Kanter ED, Petrie EC, Radant A, Thompson CE, et al. Reduction of nightmares and other PTSD symptoms in combat veterans by prazosin: a placebo-controlled study. Am J Psychiatry. (2003) 160:371–3. doi: 10.1176/appi.ajp.160.2.371
3. Breijyeh Z, Karaman R. Comprehensive review on Alzheimer's disease: causes and treatment. Molecules. (2020) 25:25245789. doi: 10.3390/molecules25245789
4. Sharma N, Classen J, Cohen LG. Neural plasticity and its contribution to functional recovery. Handb Clin Neurol. (2013) 110:3–12. doi: 10.1016/B978-0-444-52901-5.00001-0
5. Adamec RE, Blundell J, Burton P. Relationship of the predatory attack experience to neural plasticity, pCREB expression and neuroendocrine response. Neurosci Biobehav Rev. (2006) 30:356–75. doi: 10.1016/j.neubiorev.2005.04.004
6. Nissen C, Holz J, Blechert J, Feige B, Riemann D, Voderholzer U, et al. Learning as a model for neural plasticity in major depression. Biol Psychiatry. (2010) 68:544–52. doi: 10.1016/j.biopsych.2010.05.026
7. Phillips ML, Ladouceur CD, Drevets WC. A neural model of voluntary and automatic emotion regulation: implications for understanding the pathophysiology and neurodevelopment of bipolar disorder. Mol Psychiatry 13 829. (2008) 833–57. doi: 10.1038/mp.2008.65
8. Khacho M, Harris R, Slack RS. Mitochondria as central regulators of neural stem cell fate and cognitive function. Nat Rev Neurosci. (2019) 20:34–48. doi: 10.1038/s41583-018-0091-3
9. Kim Y, Vadodaria KC, Lenkei Z, Kato T, Gage FH, Marchetto MC, et al. Mitochondria, metabolism, and redox mechanisms in psychiatric disorders. Antioxid Redox Signal. (2019) 31:275–317. doi: 10.1089/ars.2018.7606
10. Barnes JN. Exercise, cognitive function, and aging. Adv Physiol Educ. (2015) 39:55–62. doi: 10.1152/advan.00101.2014
11. Jenkins DW, Jenks A. Exercise and diabetes: a narrative review. J Foot Ankle Surg. (2017) 56:968–74. doi: 10.1053/j.jfas.2017.06.019
12. Clelland CD, Choi M, Romberg C, Clemenson GD Jr, Fragniere A, Tyers P, et al. A functional role for adult hippocampal neurogenesis in spatial pattern separation. Science. (2009) 325:210–3. doi: 10.1126/science.1173215
13. Kheirbek MA, Klemenhagen KC, Sahay A, Hen R. Neurogenesis and generalization: a new approach to stratify and treat anxiety disorders. Nat Neurosci. (2012) 15:1613–20. doi: 10.1038/nn.3262
14. Sahay A, Scobie KN, Hill AS, O'Carroll CM, Kheirbek MA, Burghardt NS, et al. Increasing adult hippocampal neurogenesis is sufficient to improve pattern separation. Nature. (2011) 472:466–70. doi: 10.1038/nature09817
15. Besnard A, Sahay A. Adult hippocampal neurogenesis, fear generalization, and stress. Neuropsychopharmacology. (2016) 41:24–44. doi: 10.1038/npp.2015.167
16. Gorska N, Slupski J, Cubala WJ, Wiglusz MS, Galuszko-Wegielnik M. Antidepressants in epilepsy. Neurol Neurochir Pol. (2018) 52:657–61. doi: 10.1016/j.pjnns.2018.07.005
17. Lybrand ZR, Goswami S, Zhu J, Jarzabek V, Merlock N, Aktar M, et al. A critical period of neuronal activity results in aberrant neurogenesis rewiring hippocampal circuitry in a mouse model of epilepsy. Nat Commun. (2021) 12:1423. doi: 10.1038/s41467-021-21649-8
18. Nijholt I, Farchi N, Kye M, Sklan EH, Shoham S, Verbeure B, et al. Stress-induced alternative splicing of acetylcholinesterase results in enhanced fear memory and long-term potentiation. Mol Psychiatry. (2004) 9:174–83. doi: 10.1038/sj.mp.4001446
19. Mahar I, Bambico FR, Mechawar N, Nobrega JN. Stress, serotonin, and hippocampal neurogenesis in relation to depression and antidepressant effects. Neurosci Biobehav Rev. (2014) 38:173–92. doi: 10.1016/j.neubiorev.2013.11.009
20. Anacker C, Luna VM, Stevens GS, Millette A, Shores R, Jimenez JC, et al. Hippocampal neurogenesis confers stress resilience by inhibiting the ventral dentate gyrus. Nature. (2018) 559:98–102. doi: 10.1038/s41586-018-0262-4
21. Hill AS, Sahay A, Hen R. Increasing adult hippocampal neurogenesis is sufficient to reduce anxiety and depression-like behaviors. Neuropsychopharmacology. (2015) 40:2368–78. doi: 10.1038/npp.2015.85
22. Ma Z, Zang T, Birnbaum SG, Wang Z, Johnson JE, Zhang CL, et al. TrkB dependent adult hippocampal progenitor differentiation mediates sustained ketamine antidepressant response. Nat Commun. (2017) 8:1668. doi: 10.1038/s41467-017-01709-8
23. Santarelli L, Saxe M, Gross C, Surget A, Battaglia F, Dulawa S, et al. Requirement of hippocampal neurogenesis for the behavioral effects of antidepressants. Science. (2003) 301:805–9. doi: 10.1126/science.1083328
24. Steib K, Schaffner I, Jagasia R, Ebert B, Lie DC. Mitochondria modify exercise-induced development of stem cell-derived neurons in the adult brain. J Neurosci. (2014) 34:6624–33. doi: 10.1523/JNEUROSCI.4972-13.2014
25. Iwata R, Casimir P, Vanderhaeghen P. Mitochondrial dynamics in postmitotic cells regulate neurogenesis. Science. (2020) 369:858–62. doi: 10.1126/science.aba9760
26. Owusu-Ansah E, Banerjee U. Reactive oxygen species prime Drosophila haematopoietic progenitors for differentiation. Nature. (2009) 461:537–41. doi: 10.1038/nature08313
27. Calvanese V, Lara E, Suarez-Alvarez B, Abu Dawud R, Vazquez-Chantada M, Martinez-Chantar ML, et al. Sirtuin 1 regulation of developmental genes during differentiation of stem cells. Proc Natl Acad Sci USA. (2010) 107:13736–41. doi: 10.1073/pnas.1001399107
28. Alavi MV, Fuhrmann N. Dominant optic atrophy, OPA1, and mitochondrial quality control: understanding mitochondrial network dynamics. Mol Neurodegener. (2013) 8:32. doi: 10.1186/1750-1326-8-32
29. Galan L, Gomez-Pinedo U, Guerrero A, Garcia-Verdugo JM, Matias-Guiu J. Amyotrophic lateral sclerosis modifies progenitor neural proliferation in adult classic neurogenic brain niches. BMC Neurol. (2017) 17:173. doi: 10.1186/s12883-017-0956-5
30. Muyderman H, Chen T. Mitochondrial dysfunction in amyotrophic lateral sclerosis - a valid pharmacological target? Br J Pharmacol. (2014) 171:2191–205. doi: 10.1111/bph.12476
31. Marti-Fabregas J, Romaguera-Ros M, Gomez-Pinedo U, Martinez-Ramirez S, Jimenez-Xarrie E, Marin R, et al. Proliferation in the human ipsilateral subventricular zone after ischemic stroke. Neurology. (2010) 74:357–65. doi: 10.1212/WNL.0b013e3181cbccec
32. Boekhoorn K, Joels M, Lucassen PJ. Increased proliferation reflects glial and vascular-associated changes, but not neurogenesis in the presenile Alzheimer hippocampus. Neurobiol Dis. (2006) 24:1–14. doi: 10.1016/j.nbd.2006.04.017
33. Jin K, Peel AL, Mao XO, Xie L, Cottrell BA, Henshall DC, et al. Increased hippocampal neurogenesis in Alzheimer's disease. Proc Natl Acad Sci USA. (2004) 101:343–7. doi: 10.1073/pnas.2634794100
34. Beckervordersandforth R, Ebert B, Schaffner I, Moss J, Fiebig C, Shin J, et al. Role of mitochondrial metabolism in the control of early lineage progression and aging phenotypes in adult hippocampal neurogenesis. Neuron. (2017) 93:60–573 e566. doi: 10.1016/j.neuron.2016.12.017
35. Kann O. The interneuron energy hypothesis: implications for brain disease. Neurobiol Dis. (2016) 90:75–85. doi: 10.1016/j.nbd.2015.08.005
36. Kwon SK, Sando R. 3rd, Lewis TL, Hirabayashi Y, Maximov A, Polleux F. LKB1 regulates mitochondria-dependent presynaptic calcium clearance and neurotransmitter release properties at excitatory synapses along cortical axons. PLoS Biol. (2016) 14:e1002516. doi: 10.1371/journal.pbio.1002516
37. Chen J, Vitetta L. Mitochondria could be a potential key mediator linking the intestinal microbiota to depression. J Cell Biochem. (2020) 121:17–24. doi: 10.1002/jcb.29311
38. Joshi AU, Minhas PS, Liddelow SA, Haileselassie B, Andreasson KI, Dorn GW, et al. Fragmented mitochondria released from microglia trigger A1 astrocytic response and propagate inflammatory neurodegeneration. Nat Neurosci. (2019) 22:1635–48. doi: 10.1038/s41593-019-0486-0
39. Alberini CM, Cruz E, Descalzi G, Bessieres B, Gao V. Astrocyte glycogen and lactate: New insights into learning and memory mechanisms. Glia. (2018) 66:1244–62. doi: 10.1002/glia.23250
40. Alle H, Roth A, Geiger JR. Energy-efficient action potentials in hippocampal mossy fibers. Science. (2009) 325:1405–8. doi: 10.1126/science.1174331
41. Attwell D, Laughlin SB. An energy budget for signaling in the grey matter of the brain. J Cereb Blood Flow Metab. (2001) 21:1133–45. doi: 10.1097/00004647-200110000-00001
42. Hall CN, Klein-Flugge MC, Howarth C, Attwell D. Oxidative phosphorylation, not glycolysis, powers presynaptic and postsynaptic mechanisms underlying brain information processing. J Neurosci. (2012) 32:8940–51. doi: 10.1523/JNEUROSCI.0026-12.2012
43. Yu WM, Liu X, Shen J, Jovanovic O, Pohl EE, Gerson SL, et al. Metabolic regulation by the mitochondrial phosphatase PTPMT1 is required for hematopoietic stem cell differentiation. Cell Stem Cell. (2013) 12:62–74. doi: 10.1016/j.stem.2012.11.022
44. Folmes CD, Nelson TJ, Martinez-Fernandez A, Arrell DK, Lindor JZ, Dzeja PP, et al. Somatic oxidative bioenergetics transitions into pluripotency-dependent glycolysis to facilitate nuclear reprogramming. Cell Metab. (2011) 14:264–71. doi: 10.1016/j.cmet.2011.06.011
45. Stockburger C, Miano D, Pallas T, Friedland K, Muller WE. Enhanced neuroplasticity by the metabolic enhancer piracetam associated with improved mitochondrial dynamics and altered permeability transition pore function. Neural Plast. (2016) 2016:8075903. doi: 10.1155/2016/8075903
46. Oyewole AO, Birch-Machin MA. Mitochondria-targeted antioxidants. FASEB J. (2015) 29:4766–71. doi: 10.1096/fj.15-275404
47. Watson JD. Type 2 diabetes as a redox disease. Lancet. (2014) 383:841–3. doi: 10.1016/S0140-6736(13)62365-X
48. Khacho M, Clark A, Svoboda DS, Azzi J, MacLaurin JG, Meghaizel C, et al. Mitochondrial dynamics impacts stem cell identity and fate decisions by regulating a nuclear transcriptional program. Cell Stem Cell. (2016) 19:232–47. doi: 10.1016/j.stem.2016.04.015
49. Chen H, Chan DC. Mitochondrial dynamics in regulating the unique phenotypes of cancer and stem cells. Cell Metab. (2017) 26:39–48. doi: 10.1016/j.cmet.2017.05.016
50. Berliocchi L, Maiaru M, Varano GP, Russo R, Corasaniti MT, Bagetta G, et al. Spinal autophagy is differently modulated in distinct mouse models of neuropathic pain. Mol Pain. (2015) 11:3. doi: 10.1186/1744-8069-11-3
51. Santos SS, Moreira JB, Costa M, Rodrigues RS, Sebastiao AM, Xapelli S, et al. The mitochondrial antioxidant sirtuin3 cooperates with lipid metabolism to safeguard neurogenesis in aging and depression. Cells. (2021) 11:11010090. doi: 10.3390/cells11010090
52. Chen X, Yao JM, Fang X, Zhang C, Yang YS, Hu CP, et al. Hypoxia promotes pulmonary vascular remodeling via HIF-1alpha to regulate mitochondrial dynamics. J Geriatr Cardiol. (2019) 16:855–71. doi: 10.11909/j.issn.1671-5411.2019.12.003
53. Jiang N, Zhao H, Han Y, Li L, Xiong S, Zeng L, et al. HIF-1alpha ameliorates tubular injury in diabetic nephropathy via HO-1-mediated control of mitochondrial dynamics. Cell Prolif. (2020) 53:e12909. doi: 10.1111/cpr.12909
54. Kim JW, Tchernyshyov I, Semenza GL, Dang CV. HIF-1-mediated expression of pyruvate dehydrogenase kinase: a metabolic switch required for cellular adaptation to hypoxia. Cell Metab. (2006) 3:177–85. doi: 10.1016/j.cmet.2006.02.002
55. Chen X, Zhou B, Yan T, Wu H, Feng J, Chen H, et al. Peroxynitrite enhances self-renewal, proliferation and neuronal differentiation of neural stem/progenitor cells through activating HIF-1alpha and Wnt/beta-catenin signaling pathway. Free Radic Biol Med. (2018) 117:158–67. doi: 10.1016/j.freeradbiomed.2018.02.011
56. Carrica L, Li L, Newville J, Kenton J, Gustus K, Brigman J, et al. Genetic inactivation of hypoxia inducible factor 1-alpha (HIF-1alpha) in adult hippocampal progenitors impairs neurogenesis and pattern discrimination learning. Neurobiol Learn Mem. (2019) 157:79–85. doi: 10.1016/j.nlm.2018.12.002
57. Leidal AM, Levine B, Debnath J. Autophagy and the cell biology of age-related disease. Nat Cell Biol. (2018) 20:1338–48. doi: 10.1038/s41556-018-0235-8
58. Gustafsson AB, Dorn GW 2nd. Evolving and expanding the roles of mitophagy as a homeostatic and pathogenic process. Physiol Rev. (2019) 99:853–92. doi: 10.1152/physrev.00005.2018
59. Xiong G, Zhao L, Yan M, Wang X, Zhou Z, Chang X. N-acetylcysteine alleviated paraquat-induced mitochondrial fragmentation and autophagy in primary murine neural progenitor cells. J Appl Toxicol. (2019) 39:1557–67. doi: 10.1002/jat.3839
60. Garcia-Prat L, Martinez-Vicente M, Perdiguero E, Ortet L, Rodriguez-Ubreva J, Rebollo E, et al. Autophagy maintains stemness by preventing senescence. Nature. (2016) 529:37–42. doi: 10.1038/nature16187
61. Koene S, Kozicz TL, Rodenburg RJ, Verhaak CM, de Vries MC, Wortmann S, et al. Major depression in adolescent children consecutively diagnosed with mitochondrial disorder. J Affect Disord. (2009) 114:327–32. doi: 10.1016/j.jad.2008.06.023
62. Tsujii N, Otsuka I, Okazaki S, Yanagi M, Numata S, Yamaki N, et al. Mitochondrial DNA copy number raises the potential of left frontopolar hemodynamic response as a diagnostic marker for distinguishing bipolar disorder from major depressive disorder. Front Psychiatry. (2019) 10:312. doi: 10.3389/fpsyt.2019.00312
63. Bersani FS, Morley C, Lindqvist D, Epel ES, Picard M, Yehuda R, et al. Mitochondrial DNA copy number is reduced in male combat veterans with PTSD. Prog Neuropsychopharmacol Biol Psychiatry. (2016) 64:10–7. doi: 10.1016/j.pnpbp.2015.06.012
64. Shu X, Sun Y, Sun X, Zhou Y, Bian Y, Shu Z, et al. The effect of fluoxetine on astrocyte autophagy flux and injured mitochondria clearance in a mouse model of depression. Cell Death Dis. (2019) 10:577. doi: 10.1038/s41419-019-1813-9
65. McCoy CR, Sabbagh MN, Huaman JP, Pickrell AM, Clinton SM. Oxidative metabolism alterations in the emotional brain of anxiety-prone rats. Prog Neuropsychopharmacol Biol Psychiatry. (2019) 95:109706. doi: 10.1016/j.pnpbp.2019.109706
66. Brown PJ, Brennan N, Ciarleglio A, Chen C, Garcia CM, Gomez S, et al. Declining skeletal muscle mitochondrial function associated with increased risk of depression in later life. Am J Geriatr Psychiatry. (2019) 27:963–71. doi: 10.1016/j.jagp.2019.03.022
67. Glombik K, Stachowicz A, Slusarczyk J, Trojan E, Budziszewska B, Suski M, et al. Maternal stress predicts altered biogenesis and the profile of mitochondrial proteins in the frontal cortex and hippocampus of adult offspring rats. Psychoneuroendocrinology. (2015) 60:151–62. doi: 10.1016/j.psyneuen.2015.06.015
68. Picard M, McManus MJ, Gray JD, Nasca C, Moffat C, Kopinski PK, et al. Mitochondrial functions modulate neuroendocrine, metabolic, inflammatory, and transcriptional responses to acute psychological stress. Proc Natl Acad Sci USA. (2015) 112:E6614–23. doi: 10.1073/pnas.1515733112
69. Beirne K, Rozanowska M, Votruba M. Photostimulation of mitochondria as a treatment for retinal neurodegeneration. Mitochondrion. (2017) 36:85–95. doi: 10.1016/j.mito.2017.05.002
70. Li PA, Hou X, Hao S. Mitochondrial biogenesis in neurodegeneration. J Neurosci Res. (2017) 95:2025–9. doi: 10.1002/jnr.24042
71. Radad K, Al-Shraim M, Al-Emam A, Wang F, Kranner B, Rausch WD, et al. Rotenone: from modelling to implication in Parkinson's disease. Folia Neuropathol. (2019) 57:317–26. doi: 10.5114/fn.2019.89857
72. Greenamyre JT, Sherer TB, Betarbet R, Panov AV. Complex I and Parkinson's disease. IUBMB Life. (2001) 52:135–41. doi: 10.1080/15216540152845939
73. Hoyer S. Oxidative energy metabolism in Alzheimer brain. Studies in early-onset and late-onset cases. Mol Chem Neuropathol. (1992) 16:207–24. doi: 10.1007/BF03159971
74. Barini E, Antico O, Zhao Y, Asta F, Tucci V, Catelani T, et al. Metformin promotes tau aggregation and exacerbates abnormal behavior in a mouse model of tauopathy. Mol Neurodegener. (2016) 11:16. doi: 10.1186/s13024-016-0082-7
75. Kang H, Khang R, Ham S, Jeong GR, Kim H, Jo M, et al. Activation of the ATF2/CREB-PGC-1alpha pathway by metformin leads to dopaminergic neuroprotection. Oncotarget. (2017) 8:48603–18. doi: 10.18632/oncotarget.18122
76. Bae CS, Song J. The role of glucagon-like peptide 1 (GLP1) in type 3 diabetes: GLP-1 controls insulin resistance, neuroinflammation and neurogenesis in the brain. Int J Mol Sci. (2017) 18:18112493. doi: 10.3390/ijms18112493
77. Grieco M, Giorgi A, Gentile MC, d'Erme M, Morano S, Maras B, et al. Glucagon-like peptide-1: a focus on neurodegenerative diseases. Front Neurosci. (2019) 13:1112. doi: 10.3389/fnins.2019.01112
78. Carvalho AF, Sharma MS, Brunoni AR, Vieta E, Fava GA. The safety, tolerability and risks associated with the use of newer generation antidepressant drugs: a critical review of the literature. Psychother Psychosom. (2016) 85:270–88. doi: 10.1159/000447034
79. Balan E, Schwalm C, Naslain D, Nielens H, Francaux M, Deldicque L. Regular endurance exercise promotes fission, mitophagy, and oxidative phosphorylation in human skeletal muscle independently of age. Front Physiol. (2019) 10:1088. doi: 10.3389/fphys.2019.01088
80. Choi SH, Bylykbashi E, Chatila ZK, Lee SW, Pulli B, Clemenson GD, et al. Combined adult neurogenesis and BDNF mimic exercise effects on cognition in an Alzheimer's mouse model. Science. (2018) 361:aan8821. doi: 10.1126/science.aan8821
81. Micheli L, Ceccarelli M, D'Andrea G, Tirone F. Depression and adult neurogenesis: Positive effects of the antidepressant fluoxetine and of physical exercise. Brain Res Bull. (2018) 143:181–93. doi: 10.1016/j.brainresbull.2018.09.002
82. Poulose SM, Miller MG, Scott T, Shukitt-Hale B. Nutritional factors affecting adult neurogenesis and cognitive function. Adv Nutr. (2017) 8:804–11. doi: 10.3945/an.117.016261
83. Seo JH, Park HS, Park SS, Kim CJ, Kim DH, Kim TW. Physical exercise ameliorates psychiatric disorders and cognitive dysfunctions by hippocampal mitochondrial function and neuroplasticity in post-traumatic stress disorder. Exp Neurol. (2019) 322:113043. doi: 10.1016/j.expneurol.2019.113043
84. Park SS, Park HS, Kim CJ, Baek SS, Kim TW. Exercise attenuates maternal separation-induced mood disorder-like behaviors by enhancing mitochondrial functions and neuroplasticity in the dorsal raphe. Behav Brain Res. (2019) 372:112049. doi: 10.1016/j.bbr.2019.112049
85. Gusdon AM, Callio J, Distefano G, O'Doherty RM, Goodpaster BH, Coen PM, et al. Exercise increases mitochondrial complex I activity and DRP1 expression in the brains of aged mice. Exp Gerontol. (2017) 90:1–13. doi: 10.1016/j.exger.2017.01.013
86. Pang R, Wang X, Pei F, Zhang W, Shen J, Gao X, et al. Regular exercise enhances cognitive function and intracephalic GLUT expression in Alzheimer's disease model mice. J Alzheimers Dis. (2019) 72:83–96. doi: 10.3233/JAD-190328
87. Patten AR, Sickmann H, Hryciw BN, Kucharsky T, Parton R, Kernick A, et al. Long-term exercise is needed to enhance synaptic plasticity in the hippocampus. Learn Mem. (2013) 20:642–7. doi: 10.1101/lm.030635.113
88. Maurus I, Hasan A, Roh A, Takahashi S, Rauchmann B, Keeser D, et al. Neurobiological effects of aerobic exercise, with a focus on patients with schizophrenia. Eur Arch Psychiatry Clin Neurosci. (2019) 269:499–515. doi: 10.1007/s00406-019-01025-w
89. Adusumilli VS, Walker TL, Overall RW, Klatt GM, Zeidan SA, Zocher S, et al. ROS dynamics delineate functional states of hippocampal neural stem cells and link to their activity-dependent exit from quiescence. Cell Stem Cell. (2021) 28:300–14 e306. doi: 10.1016/j.stem.2020.10.019
90. De Miguel Z, Khoury N, Betley MJ, Lehallier B, Willoughby D, Olsson N, et al. Exercise plasma boosts memory and dampens brain inflammation via clusterin. Nature. (2021) 600:494–9. doi: 10.1038/s41586-021-04183-x
91. Littlefield AM, Setti SE, Priester C, Kohman RA. Voluntary exercise attenuates LPS-induced reductions in neurogenesis and increases microglia expression of a proneurogenic phenotype in aged mice. J Neuroinflammation. (2015) 12:138. doi: 10.1186/s12974-015-0362-0
92. Paolucci EM, Loukov D, Bowdish DME, Heisz JJ. Exercise reduces depression and inflammation but intensity matters. Biol Psychol. (2018) 133:79–84. doi: 10.1016/j.biopsycho.2018.01.015
93. Ryan SM, Nolan YM. Neuroinflammation negatively affects adult hippocampal neurogenesis and cognition: can exercise compensate? Neurosci Biobehav Rev. (2016) 61:121–31. doi: 10.1016/j.neubiorev.2015.12.004
94. Voloboueva LA, Giffard RG. Inflammation, mitochondria, and the inhibition of adult neurogenesis. J Neurosci Res. (2011) 89:1989–96. doi: 10.1002/jnr.22768
95. Huang J, Wang X, Zhu Y, Li Z, Zhu YT, Wu JC, et al. Exercise activates lysosomal function in the brain through AMPK-SIRT1-TFEB pathway. CNS Neurosci Ther. (2019) 25:796–807. doi: 10.1111/cns.13114
96. Lezi E, Burns JM, Swerdlow RH. Effect of high-intensity exercise on aged mouse brain mitochondria, neurogenesis, and inflammation. Neurobiol Aging. (2014) 35:2574–83. doi: 10.1016/j.neurobiolaging.2014.05.033
97. Kang GM, Min SH, Lee CH, Kim JY, Lim HS, Choi MJ, et al. Mitohormesis in hypothalamic POMC neurons mediates regular exercise-induced high-turnover metabolism. Cell Metab. (2021) 33:334–49 e336. doi: 10.1016/j.cmet.2021.01.003
98. Ferreira AFF, Binda KH, Singulani MP, Pereira CPM, Ferrari GD, Alberici LC, et al. Physical exercise protects against mitochondria alterations in the 6-hidroxydopamine rat model of Parkinson's disease. Behav Brain Res. (2020) 387:112607. doi: 10.1016/j.bbr.2020.112607
99. Liu YJ, Cui ZY, Yang AL, Jallow AW, Huang HL, Shan CL, et al. Anti-apoptotic and pro-survival effect of exercise training on early aged hypertensive rat cerebral cortex. Aging. (2021) 13:20495–510. doi: 10.18632/aging.203431
100. Lai JH, Chen KY, Wu JC, Olson L, Brene S, Huang CZ, et al. Voluntary exercise delays progressive deterioration of markers of metabolism and behavior in a mouse model of Parkinson's disease. Brain Res. (2019) 1720:146301. doi: 10.1016/j.brainres.2019.146301
101. Park HS, Kim CJ, Kwak HB, No MH, Heo JW, Kim TW. Physical exercise prevents cognitive impairment by enhancing hippocampal neuroplasticity and mitochondrial function in doxorubicin-induced chemobrain. Neuropharmacology. (2018) 133:451–61. doi: 10.1016/j.neuropharm.2018.02.013
102. Navazani P, Vaseghi S, Hashemi M, Shafaati MR, Nasehi M. Effects of treadmill exercise on the expression level of BAX, BAD, BCL-2, BCL-XL, TFAM, and PGC-1alpha in the hippocampus of thimerosal-treated rats. Neurotox Res. (2021) 39:1274–84. doi: 10.1007/s12640-021-00370-w
103. Pan G, Zhang H, Zhu A, Lin Y, Zhang L, Ye B, et al. Treadmill exercise attenuates cerebral ischaemic injury in rats by protecting mitochondrial function via enhancement of caveolin-1. Life Sci. (2021) 264:118634. doi: 10.1016/j.lfs.2020.118634
104. Arrazola MS, Andraini T, Szelechowski M, Mouledous L, Arnaune-Pelloquin L, Davezac N, et al. Mitochondria in developmental and adult neurogenesis. Neurotox Res. (2019) 36:257–67. doi: 10.1007/s12640-018-9942-y
Keywords: mitochondria, exercise, psychiatric diseases, neurodegenerative diseases, adult neurogenesis
Citation: Sun L, Liu T, Liu J, Gao C and Zhang X (2022) Physical exercise and mitochondrial function: New therapeutic interventions for psychiatric and neurodegenerative disorders. Front. Neurol. 13:929781. doi: 10.3389/fneur.2022.929781
Received: 27 April 2022; Accepted: 21 June 2022;
Published: 07 September 2022.
Edited by:
Hansen Chen, Stanford University, United StatesReviewed by:
Colwyn Headley, Stanford University, United StatesCopyright © 2022 Sun, Liu, Liu, Gao and Zhang. This is an open-access article distributed under the terms of the Creative Commons Attribution License (CC BY). The use, distribution or reproduction in other forums is permitted, provided the original author(s) and the copyright owner(s) are credited and that the original publication in this journal is cited, in accordance with accepted academic practice. No use, distribution or reproduction is permitted which does not comply with these terms.
*Correspondence: Lina Sun, c3VuZmxtQDEyNi5jb20=; Xiaohui Zhang, eGh6aGFuZ0BibnUuZWR1LmNu; Chong Gao, Z2FvY0B6dWNjLmVkdS5jbg==
Disclaimer: All claims expressed in this article are solely those of the authors and do not necessarily represent those of their affiliated organizations, or those of the publisher, the editors and the reviewers. Any product that may be evaluated in this article or claim that may be made by its manufacturer is not guaranteed or endorsed by the publisher.
Research integrity at Frontiers
Learn more about the work of our research integrity team to safeguard the quality of each article we publish.