- 1Department of Neurology, Mass General Brigham, Boston, MA, United States
- 2Harvard Medical School, Boston, MA, United States
The association between idiopathic Parkinson's disease, a paradigmatic dopamine-deficiency syndrome, and problems in the estimation of time has been studied experimentally for decades. I review that literature, which raises a question about whether and if dopamine deficiency relates not only to the motor slowness that is an objective and cardinal parkinsonian sign, but also to a compromised neural substrate for time perception. Why does a clinically (motorically) significant deficiency in dopamine play a role in the subjective perception of time's passage? After a discussion of a classical conception of basal ganglionic control of movement under the influence of dopamine, I describe recent work in healthy mice using optogenetics; the methodology visualizes dopaminergic neuronal firing in very short time intervals, then allows for correlation with motor behaviors in trained tasks. Moment-to-moment neuronal activity is both highly dynamic and variable, as assessed by photometry of genetically defined dopaminergic neurons. I use those animal data as context to review a large experimental experience in humans, spanning decades, that has examined subjective time perception mainly in Parkinson's disease, but also in other movement disorders. Although the human data are mixed in their findings, I argue that loss of dynamic variability in dopaminergic neuronal activity over very short intervals may be a fundamental sensory aspect in the pathophysiology of parkinsonism. An important implication is that therapeutic response in Parkinson's disease needs to be understood in terms of short-term alterations in dynamic neuronal firing, as has already been examined in novel ways—for example, in the study of real-time changes in neuronal network oscillations across very short time intervals. A finer analysis of a treatment's network effects might aid in any effort to augment clinical response to either medications or functional neurosurgical interventions in Parkinson's disease.
Introduction
Early in a parkinsonian syndrome, a family member or someone in regular contact with the patient often reports unmistakable slowing in movement, typically over a span of months or years. The patient herself may not be aware of any significant change in speed affecting activities of life, such that the diagnosis of parkinsonism may come as a surprise, met with skepticism of the diagnosis. The quantification of motor slowness in parkinsonism has been the subject of many types of investigation (1). But such objective measurement either may not or may only indirectly address the subjective experience of time's passing—what has been termed an individual's temporal cognition (2) or time perception (3). This paper examines subjective time perception in parkinsonism in light of observations of neuronal activity in substantia nigra pars compacta (SNc) during tasks, as assessed by fiber photometry of genetically defined dopaminergic neurons (DANs). The animal data introduce a consideration that has been variously addressed in human studies: do patients with idiopathic Parkinson's disease (PD) have a disease-related perturbation of their own moment-to-moment perception of time?
Dopamine (DA), Basal Ganglionic Control of Movement in General (Classical Conception), and PD
Projections from midbrain (particularly, SNc) release DA at the level of the corpus striatum with contrary effects, mediated by two different, G-protein-linked DA receptor families located on spiny projection striatal neurons. Striatal projections to downstream sites (and cortical projections to striatum) have been understood to operate by way of so-called direct and indirect pathways. In an evolutionarily conserved way (4), the direct pathway inhibits, whereas the indirect pathway disinhibits, globus pallidus pars interna and the substantia nigra pars reticulata (GPi/SNr), which together are major inhibitory output nuclei from the basal ganglia. GPi/SNr output has distinct effects on thalamic nuclei to which they project, with ensuing inhibition (via indirect pathway) or disinhibition (via direct pathway) of thalamocortical drive (5). Additionally, in the normal state, DA, acting via distinct G-protein receptor subfamilies, facilitates direct pathway activity and inhibits indirect pathway activity. An additional “hyperdirect” pathway involves two excitatory projections in series, first from cortex to subthalamic nucleus (STN), then from STN to GPi/SNr, but the pathway may not be under DA influence (6).
The principal pathologic finding in PD is degeneration of DANs in the ventrolateral SNc (7). Striatal DA deficiency provides a rationale for use of the DA precursor, levodopa, as well as DA agonists in PD. As has been studied in animal models (selective SNc neuronal loss after exposure to 6-hydoxydopamine in rats or 1-methyl-4-phenyl-1,2,3,6-tetrahydropyridine in primates), DA depletion results in consistently observed alterations, including increased firing rates of STN, GPi, and SNr neurons (8). The classical conception maintains that the multisynaptic indirect pathway between corpus striatum and thalamic nuclei becomes overactive in the context of DAN degeneration. When levodopa- or agonist-associated complications arise (e.g., dyskinesia), ablation or deep-brain, high-frequency stimulation (DBS) of GPi or STN has been associated with convincing clinical benefits, including reduction in medication-associated dyskinesia and improvement in cardinal PD signs, including motor slowness.
The classical conception, despite its heuristic value, does not account for other known aspects of DA neurotransmission. As has been observed in early vertebrate species and mammals (4, 9), SNc neurons are activated by salient visual and other sensory stimuli. The influence of the environment on DAN firing has implicated DA neurotransmission in motor learning, in which action in the world is evaluated in an ongoing way. Specifically, DANs may code for deviations between predicted and real outcomes (10). A reasonable question arises as to how DANs track the time that passes from one event to another so as to determine a deviation or “error” from an anticipation or prediction.
A DA Clock?
If an animal were trained to move to a target after a self-timed interval following a start-timing cue, then what neural mechanisms operate to determine the amount of time that precedes the movement to target? One can measure the time interval between a start cue and an ensuing movement objectively. But for self-timing to occur, some kind of subjective or internal time estimation must take place. SNc and DA may be involved in determining internal clock speed in time frames of seconds up to 2 min (2, 11–13).
Hamilos et al. (14) trained head-fixed mice to make self-timed movements to receive juice rewards (see Figure 1). Mice only received juice if they waited a prescribed interval (>3 s) before taking their first lick. After training, fiber photometry recorded the activity of SNc DANs expressing the calcium-sensitive fluorophore GCaMP6f. To control for optical artifacts, the authors simultaneously recorded co-expressed, calcium-insensitive fluorophores (“tdTomato” signals). DAN GCaMP6f fluorescence transiently increased after the start cue, then, during the self-timed interval, an up-slope or ramp-up of GCaMP6f fluorescence occurred, with a minimum time of onset of the ramp-up just after the cue-associated transient increase in firing. Slope of the ramp-up differed among trials in which mice moved early or late in relation to the cue. Differences in the steepness of ramping highly predicted the relative earliness or relative lateness of the first lick: steeply rising fluorescence preceded early licking; slowly rising fluorescence preceded later licking. TdTomato signals did not exhibit such ramping (see Figure 2).

Figure 1. Hamilos et al. (14), with permission (Creative Commons Attribution License permits unrestricted use and redistribution provided that the original author and source are credited). Abbreviations are those used in the text. Schematic of self-timed movement task in Hamilos et al. (14). After a light cue, a mouse must wait 3.3 s before initiating a movement (a lick) for liquid reward. Termination of any single trial occurs at 7 s, although the intertrial interval (ITI) ends at 17 s.
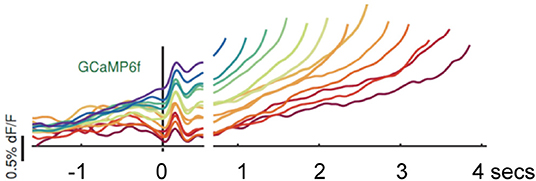
Figure 2. Hamilos et al. (14), with permission (Creative Commons Attribution License permits unrestricted use and redistribution provided that the original author and source are credited). Abbreviations are those used in the text. Average DAN GCaMP6f responses from 12 mice. The different colored traces correspond to averaged trial responses with different first-lick times after onset of a light cue. Averaged traces are aligned relative to both the start-timing cue (left of x-axis break) and timing of the first lick (right of x-axis break). The break in the x-axis indicates the change in plot alignment. Slope of the various colored traces correlates with time to first lick (steeper slope associated with earlier lick after 3.3 s; shallower slope associated with later lick after 3.3 s). dF/F: change in fluorescence intensity relative to resting fluorescence intensity.
DAN firing has effects on striatal neurons (dorsolateral striatum, DLS, in mice), as can be observed by simultaneous photometry of DLS. Optogenetic activation or inhibition of DANs, using light levels simulating physiological variations, resulted in contrary downstream striatal effects associated with different delays to movement within the time frame of a trial. DAN activation shifted the distribution of self-timed movements earlier (associated with a steeper slope of ramp-up); inhibition shifted distribution to a later time (associated with a shallower slope of ramp-up). The ramping signal unfolds over seconds and may precede first lick by as long as 10 s. Supra-physiological DAN activation caused large and sustained increases in DLS activity associated with immediate, non-purposeful body movements and disrupted task performance. An implication, corroborated by activations and inhibitions in the physiological range, is that DAN firing normally does not cause or suppress movement, but rather that DAN firing modulates a propensity to movement.
In the context of the experiments as described, propensity to movement relates either to the expectation of reward or, alternatively, to an ongoing evaluation of variability in that expectation, including situations in which reward prediction happens to be wrong. As has been described dating to the 1980's (10), DANs fire transiently in response to unpredicted rewards and to cues that correctly predict reward, but they pause when an expected reward does not occur. Only part of the work of an internal, neural clock is temporal measurement per se; another part may relate to the prediction of future salient events within very short, antecedent time frames.
An extensive time-perception literature (we focus on human studies in what follows) has specifically addressed short-term time perception in parkinsonism. How do the observations in mice, that DANs exhibit dynamic changes in firing over extremely short intervals, color our view of what DA deficiency entails for fleeting temporal perception in human disease?
Clinical Literature Review
Strategy
The literature search was conducted in accord with Preferred Reporting Items for Systematic Reviews and Meta-Analyses (PRISMA) guidelines. As of September 22, 2021, EMBASE and MEDLINE databases, interrogated with the search terms “temporal,” “perception,” and “Parkinson,” yielded 515 references. I added 69 papers selected from a PubMed search dating to 1971 and after review of bibliographies of seminal articles or reviews (1–3, 15–24). See Figure 3 for the results of the screening and selection process. A total of 84 studies in humans were studied; all were written in English. Supplementary Tables 1, 2 section provides details regarding all 84 investigations.
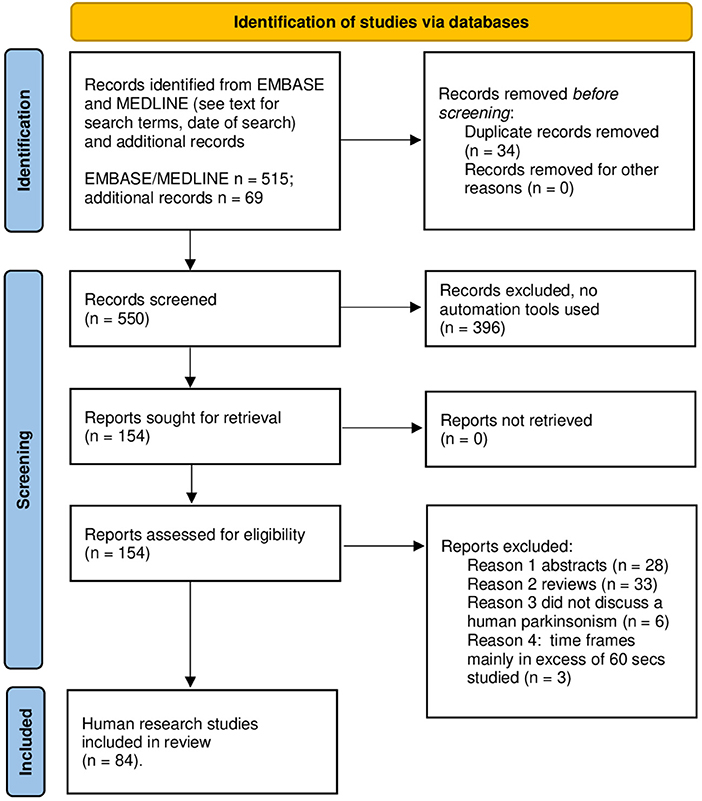
Figure 3. PRISMA 2020 template for the search and selection strategy used in this review. Review of literature according PRISMA 2020 flow sheet.
Overview of Citations
General Comments
Clinical historical information—e.g., sidedness of onset (since PD typically presents asymmetrically), disease duration, degree of response to levodopa, clinical staging of parkinsonism and its severity in the OFF-anti-PD medication state (e.g., less or poor levodopa intervals during ongoing treatment, “OFF meds”) and the ON anti-PD medication state (e.g., good or better levodopa response intervals during ongoing treatment, “ON meds”) states, presence or absence of levodopa-associated dyskinesia, variation of dyskinesia in relation to dosing during a day, specifics of all PD medications and other concomitant non-PD drugs, medical comorbidities (e.g., subcortical white matter disease, illnesses other than PD, inter alia)—tends not to be detailed systematically or consistently in papers reviewed, although there is often an attempt to control for memory deficits in PD patients, as ascertained by various neuropsychological instruments. As has been discussed elsewhere (22), clinical variability likely contributes to disparities in reported data. In addition, although clinical assessments in the sample often used standard PD rating scales, there are inherent limitations in such instruments—e.g., a United Parkinson's Disease Rating Scale (UPDRS) score for bradykinesia may not reliably correlate with observed time perception disturbances (25).
Explicit vs. Implicit Timing Tasks
Coull and Nobre (26) and Avanzino et al. (27) offer a distinction that usefully, if only broadly organizes one's approach to the interval-timing literature. An explicit timing task requires that a subject provide a specific estimation of a time interval. How subjects report an interval varies among studies, as I will examine, but the gist of an explicit timing task is to provide an estimate of elapsed time. By comparison, an implicit timing task does not require a specific estimation of how long a stimulus or an action lasts. Yet, a subject uses timing information either to accomplish a task or to predict when something will occur in relationship to a prior event. Again, variations in implicit timing tasks vary paper by paper, but the gist of an implicit timing task relates to a process by which, for example, temporal expectations underpin the accomplishment of a task. A delay in temporal processing may explain variance from controls in intrinsic timing tasks.
A criticism which Coull and Nobre acknowledge, is that one cannot dismiss the possibility that implicit timing, especially across sub-second intervals, may contribute to accomplishing an explicit timing task. Yet, the extrinsic/intrinsic distinction allows one to examine any given study in relation to others of similar, basic methodology. Coull and Nobre add that in both explicit and implicit timing categories, performance may have either “motor” or “perceptual” features: e.g., the press of a button over some duration (a motor aspect of an explicit task); judgment of a longer vs. shorter interval in relationship to another interval (a perceptual aspect of an explicit task); an action based on memory of a preceding time interval (a motor aspect of an implicit task); or, a prediction or expectation within a stipulated time frame (a perceptual aspect of an implicit task). In what follows, I address papers according to the explicit-implicit distinction, although each study in either category has its own nuances (for details per study, see Supplementary Tables 1, 2). With respect to anticipation resulting from an implicit temporal estimation, as I discuss below, a very different literature review than the current one would be necessary, since a number of studies—many of which enlist functional brain imaging—finds that prediction and expectation involve more areas of brain than those under direct DA influence.
Explicit Timing: Estimates of Time Duration
Consistent with earlier work (15–18, 28–33), Malapani et al. (17) studied OFF meds vs. ON meds states [see also (34)]. A peak-interval timing procedure was their index of time estimation. As described both by Malapani et al. (17) and by Matell and Meck (35), peak-interval timing involves a subject watching a series of images on a screen while remaining “aware” of the amount of time an image remains on the screen [e.g., 8 vs. 21 s (secs)]; then the subject is instructed that an image will appear for either 8 or 21 s. The subject is asked to press a button based on when she expects that the image will disappear. She is not supposed to subdivide duration in any fashion (no counting to herself, for example). During training, the subject is told for just a fraction of the trials that the button was pushed early, late, or accurately. After training, accuracy (button press at 8 or 21 s) and variability around either peak time are studied. Based on extensive experience in animals and humans, accuracy is often quite reasonable, but variability increases proportionally to the duration being timed (a scalar property). Systematic under- or overestimation of time tends to self-correct with training, but not all studies describe the scalar property (36).
Malapani et al. (17) reported that patients OFF meds show significantly impaired accuracy for both the 8- and 21-s peaks, with a tendency to err on the late side after 8 s and to err on the early side before 21 s. In addition, also in the OFF meds state, the variability around peaks was greater around the 8-s peak than around the 21-s peak—a non-scalar variability. Such changes were not observed either in the ON-meds state or in controls. Corroborating Pastor et al. (33), ON states differed from OFF states in terms of PD patients' time estimations. Malapani et al. concluded that dopaminergic deregulation—specifically, the OFF state—is associated with distortion of accuracy of time estimation, greater variance around peak times, and loss of scalar property. All three aspects have bearing on the subject of explicit timing tasks, perhaps especially their non-scalar property (34, 36–38), although not all studies agree that the three observations hold true in PD or other parkinsonisms [for counterpoints, see (39–41)].
Applying a explicit timing protocol used in their experience with transcranial magnetic stimulation (42, 43) noted time estimation migrations [as described by Malapani et al., (17)]–i.e., overestimation of 5-s intervals and underestimation of 15-s intervals–in PD patients OFF meds/OFF deep-brain stimulation (DBS) of subthalamic nuclei (STN) vs. the same patients OFF meds/ON DBS of STN, as well as ON meds/OFF DBS of STN. Other DBS experience suggests that effects on time duration estimation vary in relationship to the frequency of stimulation delivered to STN (44). With PD treatment (either ON meds or ON DBS of STN), time estimations improved, but did not fully correct (43, 45). Merchant et al. (37) reported two subgroups of PD OFF meds patients—one group with highly variable and the other with variability near control values in time estimation; variances improved in the ON-meds state.
Errors in the determination of duration have been thought to point to a dysfunction of a hypothetical internal clock operative in the millisecond (msec) range, as many groups have attested (15, 16, 28, 46–50). The hypothetical clock may err in non-PD contexts. In an interesting comparison of young, normal controls, elderly controls, PD patients OFF meds, as well as presymptomatic and symptomatic patients with Huntington's disease, time estimations erred (both too fast and too slow) among all cohorts except the young controls (51). Fielding et al. (52) also observed that visual saccade generation in cued and uncued contexts was impaired in both PD and Huntington's disease.
Explicit Timing: Estimation, Production, Reproduction, and Variations
The tests used to quantify subjective estimates of duration vary methodologically (22). In time estimation tasks, an interval is presented, then a subject is asked to estimate its length to the nearest second (verbally). In time production tasks, a subject is asked to perform some movement (e.g., pressing a button at start and end) for period of time that they are asked to produce (e.g., 30, 60, 120 s). In time reproduction tasks, a subject is presented a target interval visually or aurally, then, later, they are asked to perform some movement (e.g., sustained key press) for the amount of time that the target interval had been presented. The peak-interval timing procedure used by Malapani et al., (17) is a kind of time reproduction task. Time production and reproduction tests have been performed concomitantly or in variations of either (34, 39, 53, 54). In addition, there are bisection and trisection tasks: in a bisection, a subject is asked to learn two durations (e.g., a short and long one; a trisection involves three durations), then to judge whether another, new duration—one that lasts somewhere between the short and long—is closer to the short or to the long interval (55). What one learns from such subtly different tests has been debated (56, 57), and the various methods used across studies may contribute to their mixed or sometimes contradictory results.
Allman and Meck (38) offer a different cautionary note. The ON-meds state in PD has not been uniformly associated with improvement in temporal perception tasks compared to the OFF-meds state (31). In the mid-1990's, with the advent of the treatment of PD with DBS, especially of STN (pallidal DBS has not been studied in depth with respect to timing), additional subtleties have been observed (58), as we discuss, below. In some reports, PD patients (both ON and OFF meds) exhibited nearly normal timing-task performance (29, 41, 59). There has even been contrary results from the same research group: Perbal et al. and Perbal-Hatif (49, 50) reported migration effects similar to Malapani et al. (17), but Pouthas and Perbal (39) corroborated Malapani et al. only for time production, not reproduction tasks. Other investigations have examined short-term time estimations when different time frames were examined in one as opposed to separate session/s (54), in non-PD movement disorders (52, 60, 61), and in association with event-related-potential or electroencephalographic parameters (62–66).
Perhaps as a consequence of contrary data using diverse methodologies, investigators have considered other context dependencies—e.g., study of auditory or visual stimuli in time estimations ON meds (55, 67); of timing cues during gait both ON and OFF meds (68); or of time production vs. reproduction tasks both ON and OFF meds (34) or both ON and OFF meds or ON and OFF DBS of STN (45).
Using a trisection method in which subjects needed to decide whether time intervals were short (200 ms), medium (550 ms), or long (900 ms), Zhang et al. (69) found that PD subjects had difficulties in discriminating short- and medium-time epochs, but, interestingly, they exhibited impulsive decision strategies that appeared to bias them toward premature responses. In a temporal bisection task, Mioni et al. (70, 71) asked PD patients to “memorize” 400- and 1,600-ms intervals, then later to judge whether a new stimulus was closer to 400 ms or to 1,600 ms (the stimuli lasted 400, 600, 800, 1,000, 1,200, 1,400, and 1,600 ms, and were presented randomly). A so-called Weber ratio (WR) quantified variability in responses, PD subjects tended to underestimate time and to exhibit widely distributed individual WR's, the latter suggesting lower sensitivity to time estimation generally. Reminiscent of Malapani et al. (17), Terao et al. (72) found that time reproduction of very short time durations was longer in PD subjects [and even longer in Progressive Supranuclear Palsy (PSP) subjects] and shorter for long-time durations (even shorter in PSP). Terao et al.'s methodology included various tests in concert with time reproduction tasks.
Temporal misperception, as opposed to general cognition measured by neuropsychological testing, may be subtle in its manifestations, and may not be rectified with anti-PD treatments. Bernardinis et al. (73) found anomalous time estimations, especially in long rather than sub-sec time frames, that did not improve with either meds or DBS of STN [see also (45, 58)]; and varying DBS parameters had no effect on duration- and beat-based timing (74). Alternatively, Wojtecki et al. (44) observed improvements in time production and reproduction associated with high-, but not low-frequency STN of DBS. Honma et al. (75) argue that stopwatch training even in medicated PD patients can improve time production tasks as well as performance in go-no-go tasks (associated with decreased impulsivity).
Diverse observations notwithstanding, is DA deficiency itself responsible for any timing disturbance? In correlation with quantified striatal DA transporter (DaT) deficits, studying both time production (33, 53) and time reproduction (17), Honma et al. (57) found that, 0–5 s after a cue, time production overestimated, then began to underestimate time. The underestimation continued to increase from 10 to 300 s. In time reproduction, PD patients overestimated time in the first 2 s, then normalized in comparison to controls. Striatal DaT deficits correlated with underestimation beyond 10 s in reproduction tasks.
Implicit Timing: Processing Delay or Other Aberrancy in Processing
Various groups (16, 28–30, 41, 68, 76) have scrutinized the relationship in PD between a cue (e.g., a visual prompt) and cued action (a reaction based on the prompt, but after a delay period). De Lancy Horne (76) studied a population of PD patients who had undergone thalamotomy, a procedure associated with improvement in tremor and rigidity in PD. A mean-square root of reaction times reduced significant variance in the data, but, overall, PD patients exhibited slower reaction times in two of three tasks compared to age-matched controls, but different lengths of delay period (all ≥10 s) did not affect the slowness of reaction times. By comparison, another early paper examining non-linear components of visual evoked potentials (VEPs) found differences between controls and both untreated and treated PD when examining phase-shifting VEP components presented simultaneously, suggesting that processing of visual information is disturbed in PD (77). Investigators (31, 78) have also examined various stimulus-to test (cue-to-cued-response intervals). During those intervals, Sagar et al. (78) introduced other stimuli. Subjects with PD showed disproportionate deficits in content recognition—viz., in the actual content of a recollection—only at the shorted stimulus-to-test intervals, in comparison to subjects with Alzheimer's disease whose deficits manifested at all stimulus-to-test intervals.
Rammsayer and Classen (79, 80) studied information processing in PD over msec time frames by way of subjective estimations of durations of stimuli: impaired processing in those very short times, they argued, was a trait marker of decreased DA activity rather than a state marker of clinical symptomatology. The rationale behind examining activity inside 500 msecs dates to a contention from the late 19th century that, across extremely short time intervals, information processing is not mediated cortically, but rather by way of subcortical structures (80, 81). Length of scrutinized time frames varies in the early literature (15, 32); Lange et al. (53) asked patients to judge time intervals of 10, 30, and 60 s—up to two orders of magnitude greater than the interval studied by Rammsayer and Classen.
Riesen and Schnider (40) studied short temporal processing (~1 s) in patients ON meds (various daily doses among subjects) by asking them to determine the longer duration of sequentially presented images. The authors determined that, for PD patients, two stimuli must be separated by a longer interval compared to controls in order for them to perceive events as separate. In Shipley et al. (82), using a method that attempted to determine the minimum stimulus duration to discern an order embedded in simple visual stimuli (viz., a sequence of letters; images displayed from 100 to 700 msecs), PD patients ON meds exhibited, as in Riesen and Schnider, a difficulty in monitoring and distinguishing temporally distinct events in the short term. Johnson et al. (83) focused on what they called inspection time in PD (both ON and OFF meds—viz., inspection of graphical variations of the Greek letter pi). They found that in both the ON- and OFF-meds states, PD patients required longer inspection times by about a third compared to controls, although the overall inspection times for both PD groups and controls were <200 ms.
In a study of “foreperiods” before cued involuntary and voluntary motor reactions, Jurkowski et al. (84) found that for older persons without PD and for those with PD OFF meds compared to young controls, foreperiod time had very little effect on voluntary reaction time (which was delayed in response to all antecedent foreperiods, 1.0 s, 2.5 s, 4 s, 6.5 s, in both the elderly and in PD). When involuntary reaction was studied (latency to eye blink in response to a puff of air), the short (1 s) and longest foreperiod (6 s) each was associated with a quicker eyeblink (compared to the 2.5-s and 4-s foreperiods), although latencies were nevertheless longer in the elderly and in PD patients when compared to controls. As Bloxham et al. (31) had speculated years before, in general, patients with PD—perhaps the elderly as well—perform as if they were carrying out another task at the same time, but they do not dual task efficiently [for a contrary, contemporary opinion see (85, 86)].
A foreperiod methodology has been used as well by Tomassini et al. (87) who employed Bayesian analysis to observe that DA deficiency was associated with increased subjective uncertainty about predictions in time. Zokaei et al. (88), also using a variation on foreperiod, introduced a temporal orienting cue to help divert attention from distractors; they found that the cue did not help PD subjects OFF meds, but did help ON meds. The authors concluded that the benefits of cueing may relate to specific processing demands of a task.
Altered integrations, in PD, have been variously reported between sensory input [including a study with distractor stimuli (89)], motor performance, and memory or other cognitive tasks [(58, 60, 62, 68, 90–105), see (64, 65, 106) for reviews; Table 1 summarizes these particular studies]. In the spirit of context-dependent studies in explicit timing tasks (described above), PD may increase the perceived duration not of time, but of an action [(45), but see (105) for a contrary view]; may corroborate a migration effect (overestimation of short intervals, underestimation of long ones), especially when an emotionally salient event is being timed (71); may not increase the time of anticipatory eye movements (98); may shorten latency to acoustic startle (96); may prolong the perception of syntax in a piece of music (91); may prolong time spent in the appreciation of works of art (107); and may alter the subjective rating of an aural rhythm's complexity, depending on beat frequency (84).
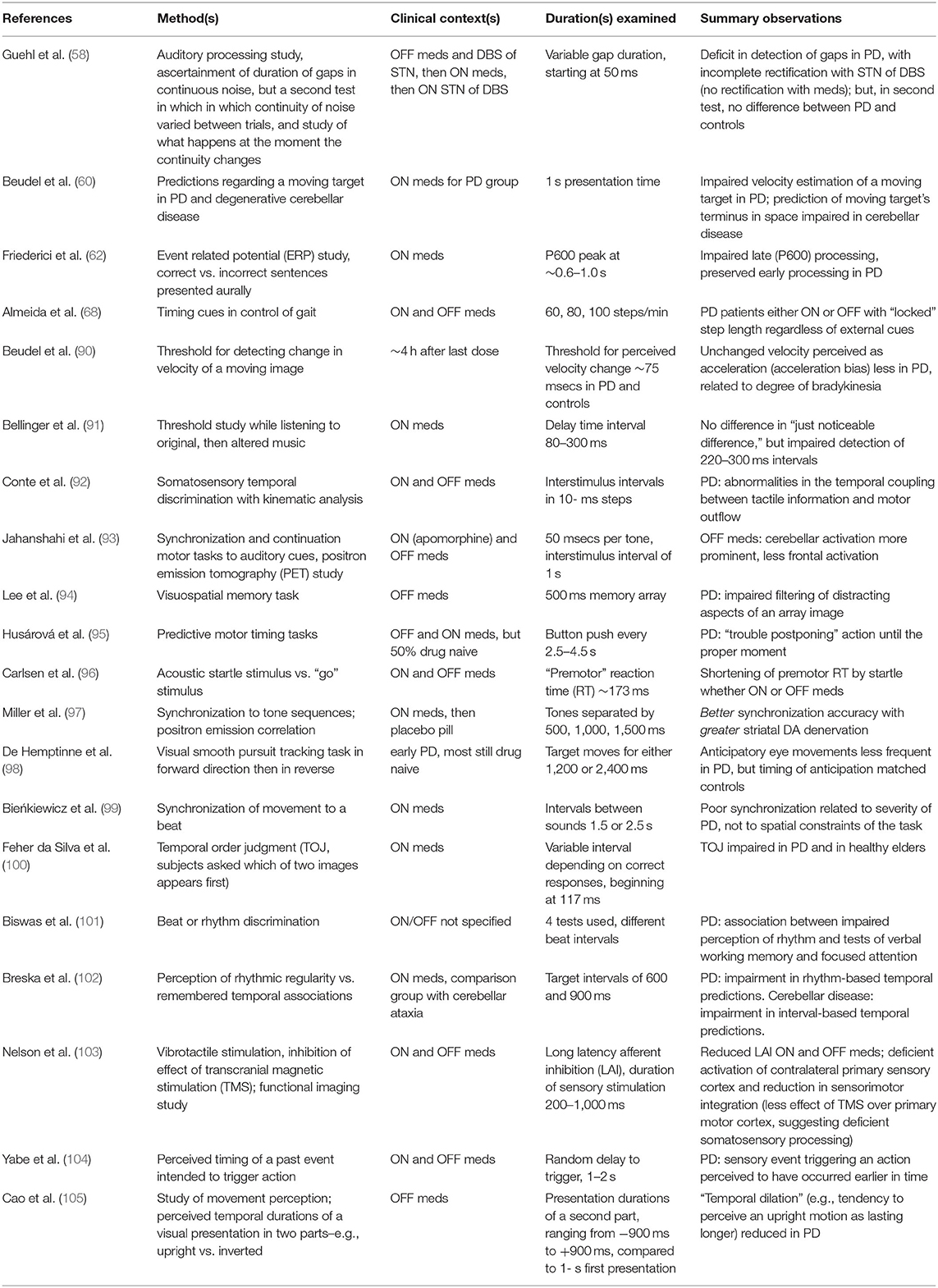
Table 1. Representative implicit timing studies regarding altered somatomotor processing (all vs. controls, with other intergroup analyses, as indicated).
Degos et al. (108) studied visual saccades initiated after variable foreperiods before movement in PD (both ON and OFF meds) and PARKIN-associated parkinsonism (the PARKIN gene, also known as PRKN, encodes an ubiquitin ligase; various mutations of the gene have been associated with an autosomal recessive parkinsonism, typically of juvenile onset). Latency to saccades decreased with increased foreperiod durations for all groups, both PD patients ON and OFF meds and controls, after controlling for motor reaction time. The implication is that implicit monitoring of elapsed time appears to be functional in PD. Yet, a within-group analysis found that the influence of an antecedent (“n-minus-1”) foreperiod was evident among controls and PARKIN-associated parkinsonians, but not among PD subjects both ON and OFF meds: what would appear to be an impairment in short-term memory for a previous foreperiod could not be attributed, in the authors' view, to a general slowness of processing if the immediately preceding foreperiod effect for an oculomotor task did not materially differ between any of the groups.
Implicit Timing: Perception of Movements in Time
An elegant study by Fiorio et al. (109) studied unilateral bradykinetic-rigid PD (OFF meds) neurophysiologically. They induced index finger abduction or wrist flexion by stimulation of the motor point of the first dorsal interosseus muscle (FDI) or flexor carpi radialis (FCR). Subjects, all blindfolded, were asked to report whether pairs of stimuli separated by various time intervals resulted in finger abduction (action of FDI), wrist flexion (action of FCR), or both. The shortest time interval at which subjects reported two discrete movements in time was called the temporal discrimination movement threshold (TDMT), specific to the proprioceptive domain. Comparisons were made between the affected and unaffected sides in PD patients and in PD patients vs. controls. Mean TDMT was higher in the affected than the unaffected arm (111 ms and 95 ms, respectively); PD patients had higher TDMT's than controls only on the affected side (111 ms and 88 ms, respectively). Lyoo et al. (110) used a different TDMT protocol, but observed that, among 30 de novo cases of multiple system atrophy, an atypical parkinsonism with subtypes (e.g., akinetic-rigid, ataxic), significantly increased thresholds correlated with bradykinesia ratings. TDMT has also been studied in asymptomatic and symptomatic PINK 1 heterozygotes in a comparison with symptomatic homozygotes (the PINK 1 gene encodes a mitochondrial protein kinase and has been linked to autosomal recessive parkinsonism): when compared to controls and asymptomatic heterozygotes, all symptomatic cohorts demonstrated higher TDMTs (111). Lee et al. (112) corroborate TDMT abnormalities, especially in OFF-state gait freezing, a particularly bothersome and dramatic PD phenomenon.
Anti-PD treatment targeting motor deficits may or may not correct observed implicit timing alterations compared to controls. When compared ON meds and OFF meds, PD subjects may not exhibit improved threshold discrimination (59). Consider, by comparison, the seeming contradiction that medications improved discrimination thresholds, but DBS of STN may not (83, 113).
Nevertheless, temporal discrimination thresholds have been examined in various ways and at different body locations in PD (25, 73, 90–92, 113–117). PD patients as well as subjects with non-PD movement disorders may exhibit implicit timing alterations (50, 51, 61, 116). Such data provide support for the idea that a movement disorder may involve higher-order sensory dysfunction (117).
A Comment on Predictive Timing
A question arises in light of the implicit timing literature whether predictions of future events are a function of implicit timing, explicit timing, or a combination of both. Ivry and Keele (32, 102), as corroborated by other studies [e.g., (118–120)], suggest that prediction of events likely involves more than a single neural mechanism—and not all those mechanisms or networks may be under direct DA influence. For example, past temporal regularity may allow a person to anticipate a future event, but, if such regularity is not present or is not perceived, then neuropsychological study (102), functional imaging and electrophysiological studies [some touched upon in this review, including (26, 27, 62, 64–66, 93, 114)] suggest that multiple cortical and subcortical domains may be involved, depending on specific context. Breska and Ivry (102) summarize neuroimaging studies that highlight the role of cortical (e.g., left inferior parietal lobe, supplementary motor area, and right inferior frontal cortex) and cerebellar nodes—striatal locales to a lesser degree—in experimental paradigms that attempt to reproduce prediction in time. This review does not significantly broach that expanding literature, although consideration of DANs in time keeping and prediction is not therefore obviated.
Discussion
The subjective estimation of time intervals (either in explicit or implicit timing tasks) has been studied extensively in PD. The studies included in this review are not at all univocal in their conclusions, but they altogether point to an open question, relevant both to basic and clinical science: why might DA play a role—or why does an otherwise clinically significant deficiency in DA play a role—in timekeeping at all?
In response, consider DANs and the determination of inter-event durations in the context of expected reward. As Hamilos et al. (14) observe, DANs fire in steep or gradual ramped fashions that predict, respectively, when earlier or later movement will occur. What information can be said to be communicated by different slopes of firing—or, put differently, what do slopes inform about the context in which the animal finds itself at any given time? DANs do not trigger movement per se, as was corroborated when DANs were optogenetically activated at physiological levels. A first-pass resemblance to interval timing in which a clock system senses duration in the world, then inputs to an “accumulator” (a kind of working memory), as has been studied in the Pavlovian conditional response (121), suggests that DAN ramping relates to the probability of response in specific contexts or circumstances.
Only a minority of SNc neurons can be visualized at a time in a photometric study of SNc in mice, but it is plausible that the extreme loss of DANs in clinically manifest PD (7) may result in a dys-calibration of accumulator function. The consequence would not necessarily be an inability to move, but rather a skewed probability function that could manifest variously–either as slowness, impulsivity, perseveration, or some combination of all three features. In clinical PD phenomenology, slowness does in fact mix with other aspects, as one observes in a festinating gait, which combines both fast and slow aspects. Likewise, in gait freezing, the stance is not a fixed motionlessness in space; it is often apparent that a PD patient is trying to step forward, though the perseverative effort is both fitful and unsuccessful.
With respect to the provocative, but not universally accepted observation that PD patients overestimate very short time intervals and underestimate longer ones, some background discussion is in order. Karl Vierordt's The Experimental Study of the Time Sense, published in German in 1868 (122), introduced a psychological “law” in which, for example (based on study of himself, but corroborated by other data reported in the book), durations < ~2.5 s are overestimated and those longer than ~2.5 s are underestimated normally, with an “indifference” point (at ~2.5 s), when estimation of duration is transiently veridical. The validity of Vierordt's law, instances of its violation, and the variability of the indifference point (depending on durations studied, extending to many minutes) have been reviewed (123), and iterative Bayesian analysis has been applied to some of the original 1868 data to show that the law's validity varies with testing protocols, especially when durations are randomly ordered (124). Variation in manner of study certainly characterizes the studies included in this literature review. One should not conclude that the variability forces one to dismiss the idea of a temporal-sensory disturbance, since there are interesting suggestions that time misperception may underpin not only PD, but also other movement disorders (e.g., Huntington's disease and dystonia), as somatosensory threshold data and many other, though by no means all, studies that address implicit timing tasks have demonstrated, perhaps more consistently than in studies of explicit timing.
The goal of treatment in a parkinsonism is to allow for the greatest possible functional benefit in real time. The adjective “real” is essential: time judgments in life are not completely veridical compared to an objective clock, and, in real life, desires, plans, goals, and past experience (in both short and sometimes very long time frames) color both moment-to-moment and overall survival.
Recent data suggest that primates use Bayesian strategies by which preceding events alter probabilities of later ones in a trial-by-trial, ongoing fashion, all as a function of cortical representations of elapsed time (125). At the core of such a strategy is an incremental procedure in which differences in temporally successive predictions drive learning, as opposed to decisions made on the basis of past prediction errors of temporally isolated events (126). Mikhael and Gershman (24) have reviewed dominant models in the DA-and-timing literature—Table 2 provides a summary based on their paper and this review. They usefully observe that all modeling contends with minimizing differences between true and estimated values (e.g., of time; of a reward in time, associated with a “reward prediction error”) as a function of different gradients (a term also used in 127) that represent, as in any ongoing analysis, the effect of antecedents on future judgments of difference between the estimated and the true. It may not be the case that successive trials necessarily lead to a zero difference between truth and estimation, and, more probably, a zero difference never happens, neither absolutely nor in perpetuity. It should not surprise that, in real life, judgments can err in either direction, both toward the veridical or very far from it (but bidirectional updating continues, by definition). Mikhael and Gershman (24) further observe that modulation of timekeeping mediated by DA would have an effect on learning in the above iterative sense, especially in contexts that are either highly complex (“noisy”) or stochastic (random).
An important therapeutic implication is that current therapy to spare DA, or to enhance DA agonism, or the long-term, high-frequency stimulation of non-SNc sites may simply not rectify the inherently dynamic capacity of DANs in the pre-disease state. Loss of temporal dynamism has wide ranging network effects. If we consider that A8, A9, and A10 DA cell groups not only project to striatum, but also receive afferents from diverse locations, including the striatum itself (see Table 2 for a summary), DA denervation in PD represents both and output and input problem: not only is there loss of DA innervation of relevant pathways to striatum, then to downstream sites, but also the role of DANs in afferent integration is compromised—the latter may be associated with time misperceptions in movement disorders not themselves associated with selective DAN degeneration.
A greater probabilistic understanding of how pre-planned or spontaneous movement begins to transpire across very short time frames may inform new, more exquisitely variable, and highly time-sensitive deliveries of treatment. For example, as reviewed by Jenkinson and Brown (134) and corroborated by Hamilos et al. (14) in their data presented above, increases in striatal DA happen at 500–600 msecs after DAN discharges that constitute a phasic burst. In association with phasic DAN discharges, striatal interneuronal oscillations the beta range [13–30 hertz (Hz)] dampen in an intermittent or “scalloped” fashion. In PD, reduced beta synchrony may be a marker for motor impairment. It may further be the case that there are different ranges of beta oscillations—e.g., low beta (with mean frequency of ~15 Hz) and high beta (mean frequency of ~26 Hz)—with the most robust motor improvements in the ON state associated with dampened low-beta, but not high-beta oscillation (135). Dating to early work on synchronization of neural activity [reviewed in (136); see (66) for a discussion of electroencephalographic synchronization specifically in a timing task in PD], temporal patterns have been understood as a repository of information useful in understanding both normal physiology and pathophysiology: temporal coding, based on patterns observed in short-me frames, along with their distributed effects (e.g., striatal beta oscillations), could lead to refined ideas about what specifically changes in an anti-PD treatment (e.g., dampening specifically of low-beta oscillation) and the relation between a treatment-related change and other temporal coding associations (e.g., coupling between beta and other frequency oscillations in different time epochs or across different anatomical locations).
I acknowledge obvious limitations in my review. To concentrate on the role of DA in subjective time perception is not to say that DANs are the only timekeepers in the central nervous system, nor do DANs exclusively clock time. Inspecting the various theories posed in the DA-timing literature (Table 3), as Cruz and Paton (145) have opined in a different context, one notes that DA signaling must be heterogeneous (it does not merely keep time). Further, DA effects must be spatiotemporally ramified if any one of the theories is even partly valid. Transitions in behavior, mnemonic encoding, reinforcement learning, conditioned and unconditioned response, and ongoing correlations between explicit and implicit perception of the world are functions that intuitively should involve much of the brain as one gleans from even a cursory inspection of the predictive timing literature.
The subjective perception of time simply does not “belong” to a specific brain region (146); among the papers in this review, even in the context of DAN loss in PD, other subcortical structures (perhaps cerebellum in particular) may intervene to compensate for a DA-related timing deficit (80). Pertinent, however, to DANs and striatum in particular, Cruz and Paton (145) editorialize on recent work (147) that leverages high-resolution visualization of signaling directed to the richly DA-innervated striatum, including its striatal sensorimotor and associative or limbic domains: Hamid et al. (147) describe “wave-like spatiotemporal activity” across striatum, with DA transients organizing into local striatal clusters, which appear to be tailored to task demands. Of interest in relationship to the mouse data presented at the start of this review, DA ramped signaling may pass to striatal subregions in relation to demands of a task in real time. DA dynamism is temporally varied in very short time frames at the level of SNc and, downstream, it exhibits elegant and intricate spatial ramification to accomplish intended action.
Conclusions
Animal study suggests a role for DA in timing related to planned movement. The subjective perception of time informs the bradykinesia or slowness that characterizes PD. Loss of dynamic variability in DA neuronal activity in very short time frames may be fundamental to the pathophysiology of parkinsonism and perhaps other disorders manifesting incorrectly timed coordination or sequencing of purposeful movements. In a literature review spanning 40 years, clinical studies have concentrated on how PD patients perceive time compared to controls (a preliminary conclusion might be that temporal perception is variable, as even normal, daily experience corroborates: why can the same interval of veridical time seem to pass so quickly or so very slowly?).
More importantly, why does time perception matter in the first place? Mixed results in clinical research do not quite address the “why” question, which has been more fully examined in animal work. One should not conclude that the variability in clinical data forces one to dismiss the idea of a temporal-perceptual disturbance in PD. DA-mediated time perception is most obvious in scenarios where motor planning and associated reward are studied in a Pavlov-inspired experimental design. In human study, an important therapeutic implication is that current therapy to spare DA, or to enhance DA agonism, or the long-term, high-frequency stimulation of non-SNc sites may not rectify the inherently dynamic capacity of DANs in the pre-disease state. Loss of dopaminergic temporal dynamism has wide ranging network effects. DA denervation in PD represents both and output and input problem: not only is there loss of DA innervation of relevant pathways to striatum, thence to downstream sites, but also the role of DANs in afferent integration is compromised—the latter may be associated with time misperceptions associated with the disordered planning and execution of movement, not only in parkinsonism, but perhaps also in other movement disorders not associated specifically with DAN loss in SNc.
Author Contributions
EM, with much advice from others noted in the acknowledgment section, conceived and conducted the review, wrote, and revised the article.
Conflict of Interest
The author declares that the research was conducted in the absence of any commercial or financial relationships that could be construed as a potential conflict of interest.
Publisher's Note
All claims expressed in this article are solely those of the authors and do not necessarily represent those of their affiliated organizations, or those of the publisher, the editors and the reviewers. Any product that may be evaluated in this article, or claim that may be made by its manufacturer, is not guaranteed or endorsed by the publisher.
Acknowledgments
The author very gratefully acknowledges Allison E. Hamilos, Ph.D., who is currently completing her M.D/Ph.D. at Harvard Medical School. Her input was invaluable. Only her clinical obligations during the M.D. phase of her education prevented her from contributing as an outright co-author. Dr. John A. Assad's input, especially in the earliest phase of this study, is also duly acknowledged. In the Department of Neurobiology at Harvard Medical School, Dr. Assad supervised and co-authored Hamilos's work cited in this article.
Supplementary Material
The Supplementary Material for this article can be found online at: https://www.frontiersin.org/articles/10.3389/fneur.2022.927160/full#supplementary-material
References
1. Bologna M, Parparella G, Fasano A, Hallett M, Berardelli A. Evolving concepts on bradykinesia. Brain. (2020) 143:727–50. doi: 10.1093/brain/awz344
2. Gibbon J, Malapani C, Dale CL, Gallistel CR. Toward a neurobiology of temporal cognition: advances and challenges. Curr Opin Neurobiol. (1997) 7:170–84. doi: 10.1016/S0959-4388(97)80005-0
3. Meck WH. Neuropharmacology of timing and time perception. Cogn Brain Res. (1996) 3:227–42. doi: 10.1016/0926-6410(96)00009-2
4. Grillner S, Robertson B. The basal ganglia over 500 million years. Curr Biol. (2016) 26:R1088–100. doi: 10.1016/j.cub.2016.06.041
5. Delong MR. Primate models of movement disorders of basal ganglionic origin. Trends Neurosci. (1990) 13:281–5. doi: 10.1016/0166-2236(90)90110-V
6. Oswal A, Brown P, Litvak V. Synchronized neural oscillations and the pathophysiology of Parkinson's disease. Curr Opin Neurol. (2103) 26:662–70. doi: 10.1097/WCO.0000000000000034
7. Fearnley JM, Lees AJ. Ageing and Parkinson's disease: substantia nigra regional selectivity. Brain. (1991) 114:2283–301. doi: 10.1093/brain/114.5.2283
8. Wichmann T, Delong MR. Functional and pathophysiological models of the basal ganglia. Curr Opin Neurobiol. (1996) 6:751–8. doi: 10.1016/S0959-4388(96)80024-9
9. Grillner S. Evolution of the vertebrate motor system—from forebrain to spinal cord. Curr Opin Neurobiol. (2021) 71:11–8. doi: 10.1016/j.conb.2021.07.016
10. Schultz W, Dayan P, Montague PR. A neural substrate of prediction and reward. Science. (1997) 275:1594–9. doi: 10.1126/science.275.5306.1593
11. Dews PB, Morse WH. Some observations on an operant in human subjects and its modification by dextroamphetamine. J Exp Anal Behav. (1958) 1:359–64. doi: 10.1901/jeab.1958.1-359
12. Maricq AV, Church RM. The differential effects of haloperidol and methamphetamine on time estimation in the rat. Psychopharmacology. (1983) 79:10–5. doi: 10.1007/BF00433008
13. Meck WH. Affinity for the dopamine D2 receptor predicts neuroleptic potency in decreasing the speed of an internal clock. Pharmacol Biochem Behav. (1986) 25:1185–9. doi: 10.1016/0091-3057(86)90109-7
14. Hamilos AE, Spedicato G, Hong Y, Sun F, Li Y, Assad JA. Slowly evolving dopaminergic activity modulates the moment-to-moment probability of reward-related self-timed movements. ELife. (2021) 10:10–23. doi: 10.7554/eLife.62583
15. Artieda J, Pastor MA, Lacruz F, Obeso JA. Temporal discrimination is abnormal in Parkinson's disease. Brain. (1992) 115:199–210. doi: 10.1093/brain/115.1.199
16. O'Boyle DJ, Freeman JS, Cody FWJ. The accuracy and precision of time of self-paced repetitive movements in subjects with Parkinson's disease. Brain. (1996) 119:51–70. doi: 10.1093/brain/119.1.51
17. Malapani C, Rakitin B, Meck WH, Deweer B, Dubois B, Gibbon, et al. Coupled temporal memories in Parkinson's disease: a dopamine-related dysfunction. J Cogn Neurosci. (1998) 10:316–31. doi: 10.1162/089892998562762
18. Lucas M, Chaves F, Teixeira S, Carvalho D, Peresutti D, Bittencourt J, et al. Time perception impairs sensory-motor integration in Parkinson's disease. Int Arch Med. (2013) 3:39. doi: 10.1186/1755-7682-6-39
19. Parker KL, Lamichhane D, Caetano MS, Narayanan NS. Executive dysfunction in Parkinson's disease and timing deficits. Front Integr Neurosci. (2013) 7:75. doi: 10.3389/fnint.2013.00075
20. Allman MJ, Teki S, Griffiths TD, Meck WH. Properties of the internal clock: first- and second-order principles of subjective time. Annu Rev Physiol. (2014) 65:743–71. doi: 10.1146/annurev-psych-010213-115117
21. Costa A, Peppe A, Mazzù I, Longarzo M, Caltagirone C, Carlesimo GA. Dopamine treatment and cognitive functioning in individuals with Parkinson's disease: the “cognitive flexibility” hypothesis seems to work. Behav Neurol. (2014) 2014:260896. doi: 10.1155/2014/260896
22. Jones CRG, Jahanshahi M. Contributions of the basal ganglia to temporal processing: evidence from Parkinson's disease. Timing Time Percept. (2014) 2:87–127. doi: 10.1163/22134468-00002009
23. Soares S, Atallah BV, Paton JJ. Midbrain dopamine neurons control judgment of time. Science. (2016) 354:1273–7. doi: 10.1126/science.aah5234
24. Mikhael JG, Gershman SJ. Adapting the flow of time with dopamine. J Neurophysiol. (2019) 121:1748–60. doi: 10.1152/jn.00817.2018
25. Lee MJ, Son JS, Lee LH, Kim SJ, Lyoo CH, Lee MS. Impact of prolonged temporal discrimination threshold on finger movements of Parkinson's disease. PLoS ONE. (2016) 11:e0167034. doi: 10.1371/journal.pone.0167034
26. Coull JT, Nobre AC. Dissociating explicit timing from temporal expectation with fMRI. Curr Opin Neurobiol. (2008) 18:137–44. doi: 10.1016/j.conb.2008.07.011
27. Avanzino L, Pelosin E, Vicario CM, Lagravinese G, Abbruzzese G, Martino D. Time processing and motor control in movement disorders. Front Neurosci. (2016) 10:631. doi: 10.3389/fnhum.2016.00631
28. Freeman JS, Cody FWJ, Schady W. The influence of external cues upon the rhythm of voluntary movements in Parkinson's disease. J Neurol Neurosurg Psychiatry. (1993) 53:1078–84. doi: 10.1136/jnnp.56.10.1078
29. Duchek JM, Balota DA, Ferraro FR. Component analysis of a rhythmic finger tapping task in individuals with senile dementia of the Alzheimer's type and in individuals with Parkinson's disease. Neuropsychology. (1994) 8:218–26. doi: 10.1037/0894-4105.8.2.218
30. Harrington DL, Haaland KY, Hermanowicz N. Temporal processing in the basal ganglia. Neuropsychology. (1998) 12:3–12. doi: 10.1037/0894-4105.12.1.3
31. Bloxham CA, Dick DJ, Moore M. Reaction times and attention in Parkinson's disease. J Neurol Neurosurg Psychiatry. (1987) 50:1178–83. doi: 10.1136/jnnp.50.9.1178
32. Ivry RB, Keele SW. Timing functions of the cerebellum. J Cogn Neurosci. (1989) 1:136–52. doi: 10.1162/jocn.1989.1.2.136
33. Pastor MA, Artieda J, Jahanshahi M, Obeso JA. Time estimation and reproduction is abnormal in Parkinson's disease. Brain. (1992) 116:211–25. doi: 10.1093/brain/115.1.211
34. Jones CR, Malone TJ, Dirnberger G, Edwards M, Jahanshahi M. Basal ganglia, dopamine and temporal processing: performance on three timing tasks on and off medication in Parkinson's disease. Brain Cogn. (2008) 68:30–41. doi: 10.1016/j.bandc.2008.02.121
35. Matell MS, Meck WH. Neuropsychological mechanisms of interval timing behavior. Bioessays. (2000) 22:94–103. doi: 10.1002/(SICI)1521-1878(200001)22:1<94::AID-BIES14>3.0.CO;2-E
36. Shea-Brown E, Rinzel J, Rakitin BC, Malapani C. A firing rate model of parkinsonian deficits in interval timing. Brain Res. (2006) 1070:189–201. doi: 10.1016/j.brainres.2005.10.070
37. Merchant H, Luciana M, Hooper C, Majestic S, Tuite P. Interval timing and Parkinson's disease: heterogeneity in temporal performance. Exp Brain Res. (2008) 184:233–48. doi: 10.1007/s00221-007-1097-7
38. Allman MJ, Meck WH. Pathophysiological distortions in time perception and time performance. Brain. (2012) 135:656–77. doi: 10.1093/brain/awr210
39. Pouthas V, Perbal S. Time perception depends on accurate clock mechanisms as well as unimpaired attention and memory processes. Acta Neurobiol Exp. (2004) 64:367–85.
40. Riesen JM, Schnider A. Time estimation in Parkinson' disease: normal long duration estimation despite impaired short duration discrimination. J Neurol. (2001) 248:27–35. doi: 10.1007/s004150170266
41. Spencer RMC, Ivry RB. Comparison of patients with Parkinson's disease or cerebellar lesions in the production of periodic movements involving event-based or emergent timing. Brain Cogn. (2005) 58:84–93. doi: 10.1016/j.bandc.2004.09.010
42. Koch G, Oliveri M, Torriero S, Caltagirone C. Underestimation of time perception after repetitive transcranial magnetic stimulation. Neurol. (2003) 60:1844–6. doi: 10.1212/WNL.60.11.1844
43. Koch G, Brusa L, Caltagirone C, Oliveri M, Peppe A, Tiraboschi P, et al. Subthalamic deep brain stimulation improves times perception in Parkinson's disease. NeuroReport. (2004) 15:1071–3. doi: 10.1097/00001756-200404290-00028
44. Wojtecki L, Elben S, Timmerman L, Reck C, Maarouf M, Jorgens S, et al. Modulation of human time processing by subthalamic deep brain stimulation. PLoS ONE. (2011) 6:12. doi: 10.1371/journal.pone.0024589
45. Torta DME, Castelli L, Latini-Corazzini L, Banche A, Lopiano L, Geminiani G. Dissociation between time reproduction and actions and of intervals in patients with Parkinson's disease. J Neurol. (2010) 257:1356–61. doi: 10.1007/s00415-010-5532-5
46. Malapani C, Deweer B, Gibbon J. Separating storage from retrieval dysfunction of temporal memory in Parkinson's disease. J Cogn Neurosci. (2002) 14:311–22. doi: 10.1162/089892902317236920
47. Harrington DL, Haaland KY. Neural underpinnings of temporal processing: a review of focal lesion, pharmacological, and functional imaging research. Rev Neurosci. (1998) 10:91–116. doi: 10.1515/REVNEURO.1999.10.2.91
48. Harrington DL, Haaland KY, Knight RT. Cortical networks underlying mechanisms of time perception. J Neurosci. (1998) 18:1085–95. doi: 10.1523/JNEUROSCI.18-03-01085.1998
49. Perbal S, Deweer B, Pilion B, Vidaihet M, Dubois B, Pouthas V. Effects of internal clock and memory disorders on duration reproductions and duration productions in patients with Parkinson's disease. Brain Cogn. (2005) 58:35–48. doi: 10.1016/j.bandc.2005.02.003
50. Perbal-Hatif S. A neuropsychological approach to time estimation. Dial Clin Neurosci. (2012) 14:425–32. doi: 10.31887/DCNS.2012.14.4/sphatif
51. Wild-Wall N, Willemssen R, Falkenstein M, Beste C. Time estimation in healthy ageing and neurodegenerative basal ganglia disorders. Neurosci Lett. (2008) 442:34–8. doi: 10.1016/j.neulet.2008.06.069
52. Fielding J, Georgious-Karistianis N, Millist L, White O. Temporal variation in the control of goal-directed visuospatial attention in basal ganglia disorder. Neurosci Res. (2006) 54:57–65. doi: 10.1016/j.neures.2005.10.007
53. Lange KW, Tucha O, Steup A, Gsell W, Naumann M. Subjective time estimation in Parkinson's disease. J Neural Transm Suppl. (1995) 46:433–8.
54. Koch G, Costa A, Brusa L, Peppe A, Gatto I, Torriero S, et al. Impaired reproduction of second but not millisecond time intervals in Parkinson's disease. Neuropsychologia. (2008) 46:1305–13. doi: 10.1016/j.neuropsychologia.2007.12.005
55. Smith JG, Harper DN, Gittings D, Abernethy D. The effect of Parkinson's disease on time estimation as a function of stimulus duration range and modality. Brain Cogn. (2007) 64:130–43. doi: 10.1016/j.bandc.2007.01.005
56. Mioni G, Stabium F, McClintock SM, Grondin S. Different methods for reproducing time, different results. Atten Percept Psychophys. (2014) 76:675–81. doi: 10.3758/s13414-014-0625-3
57. Honma M, Kuroda T, Futamura A, Shiromaru A, Kawamura M. Dysfunctional counting of mental time in Parkinson's disease. Sci Rep. (2016) 6:25421. doi: 10.1038/srep25421
58. Guehl D, Burbaud P, Lorenzi D, Ramos C, Bioulac B, Semal C, et al. Auditory temporal processing in Parkinson's disease. Neuropsychologia. (2008) 46:2326–35. doi: 10.1016/j.neuropsychologia.2008.03.007
59. Wearden JH, Smith-Spark JH, Cousins R, Edelstyn NMJ, Cody FWJ, O'Boyle DJ. Stimulus timing by people with Parkinson's disease. Brain Cogn. (2008) 67:264–79. doi: 10.1016/j.bandc.2008.01.010
60. Beudel M, Galama S, Leenders KL, de Jong BM. Time estimation in Parkinson's disease and degenerative cerebellar disease. NeuroReport. (2008) 19:1055–9. doi: 10.1097/WNR.0b013e328303b7b9
61. Högl B, Agostino PV, Peralta MC, Gershanik O, Golombek DA. Alterations in time estimation in multiple system atrophy. Basal Ganglia. (2014) 4:95–9. doi: 10.1016/j.baga.2014.06.004
62. Friederici AD, Kotz SA, Werheid K, Hein G, Yves von Cramon D. Syntactic comprehension in Parkinson's disease: investigating early automatic and late integrational processes using event-related potentials. Neuropsychology. (2003) 17:133–42. doi: 10.1037/0894-4105.17.1.133
63. Pfeuty M, Ragot R, Pouthas V. Relationship between CNV and timing of an upcoming event. Neurosci Lett. (2005) 382:106–11. doi: 10.1016/j.neulet.2005.02.067
64. Meck WH, Penney TB, Pouthas V. Cortico-striatal representation of time in animals and humans. Curr Opin Neurobiol. (2008) 18:145–52. doi: 10.1016/j.conb.2008.08.002
65. Kotz SA, Schwartze M, Schmidt-Kassow M. Non-motor basal ganglia functions: a review and proposal for a model of sensory predictability in auditory language perception. Cortex. (2009) 45:982–90. doi: 10.1016/j.cortex.2009.02.010
66. Singh A, Cole RC, Espinoza AI, Evans A, Cao S, Cavanagh JF, et al. Timing variability and midfrontal ~4 Hz rhythms correlate with cognition in Parkinson's disease. Npj Parkinson's Disease. (2021) 7:14. doi: 10.1038/s41531-021-00158-x
67. Harrington DL, Castillo GN, Reed JD, Song DD, Litvan I, Lee RR. Dissociation of neural mechanisms for intersensory timing deficits in Parkinson's disease. Timing Time Percept. (2014) 2:145–68. doi: 10.1163/22134468-00002025
68. Almeida QJ, Frank JS, Roy EA, Patla AE, Jog MS. Dopaminergic modulation of timing control and variability of gait of Parkinson's disease. Mov Disord. (2007) 22:1735–42. doi: 10.1002/mds.21603
69. Zhang J, Nombela C, Wolpe N, Barker RA, Rowe JB. Time on timing: dissociating premature responding from internal sensitivity in Parkinson's disease. Mov Disord. (2016) 31:1163–72. doi: 10.1002/mds.26631
70. Mioni G, Capizzi M, Vallesi A, Correa Á, DiGiacopo R, Stablum F. Dissociating explicit and implicit timing in Parkinson's disease patients: evidence from bisection and foreperiod tasks. Front Hum Neurosci. (2018) 12:17. doi: 10.3389/fnhum.2018.00017
71. Mioni G, Meligrana L, Grondin S, Perini F, Bartolomei L, Stablum F. Effects of emotional facial expression on time perception in patients with Parkinson's disease. J Int Neuropsychol Soc. (2016) 22:890–9. doi: 10.1017/S1355617715000612
72. Terao Y, Honma M, Asahara Y, Tokushige SI, Furubayashi T, Miyazaki T, et al. Time distortion in Parkinsonism. Front Neurosci. (2021) 15:648814. doi: 10.3389/fnins.2021.648814
73. Bernardinis M, Atashzar SF, Jog MS, Patel RV. Differential temporal perception abilities in Parkinson's disease based on timing magnitude. Sci Rep. (2019) 9:19638. doi: 10.1038/s41598-019-55827-y
74. Cope TE, Grube M, Mandal A, Cooper FE, Brechany U, Burn DJ, et al. Subthalamic deep brain stimulation in Parkinson's disease no significant effect on perceptual timing in the hundreds of milliseconds range. Neuropsychologia. (2014) 57:29–37. doi: 10.1016/j.neuropsychologia.2014.02.021
75. Honma M, Murakami H, Yabe Y, Kuroda T, Futamura A, Sugimoto A, et al. Stopwatch training improves cognitive functions in patients with Parkinson's disease. J Neurosci Res. (2021) 99:1325–36. doi: 10.1002/jnr.24812
76. De Lancy Horne DJ. Performance on delayed response tasks by patients with parkinsonism. J Neurol Neurosurg Psychiatry. (1971) 34:192–4. doi: 10.1136/jnnp.34.2.192
77. Bodis-Wollner I, Harnois C, Bobak P, Mylin L. On the possible role of temporal delays of afferent processing in Parkinson's disease. J Neural Transm Suppl. (1983) 19:243–52.
78. Sagar HJ, Sullivan EV, Gabrieli JD, Corkin S, Growdon JH. Temporal ordering and short-term memory deficits in Parkinson's disease. Brain. (1988) 111:525–39. doi: 10.1093/brain/111.3.525
79. Rammsayer T, Classen W. Impaired temporal discrimination in Parkinson's disease: temporal processing of brief durations as an indicator of degeneration of dopaminergic neurons in the basal ganglia. Int J Neurosci. (1997) 91:45–55. doi: 10.3109/00207459708986364
80. Rammsayer TH. Neuropharmacological evidence for different timing mechanisms in humans. Q J Exp Psychology. (1999) 52B:273–86.
81. Münsterberg H. Der Zeitsinn. In: Beiträge zur Experimentellen Psychologie. Heft 2. Freiburg: Akademische Verlagsbuchhandlung von JCB Mohr (1889). p. 1–68.
82. Shipley BA, Deary IJ, Tan J, Christie G, Starr JM. Efficiency of temporal order discrimination as an indicator of bradyphrenia in Parkinson's disease: the inspection time loop task. Neuropsychologia. (2002) 40:1488–93. doi: 10.1016/S0028-3932(01)00195-6
83. Johnson AM, Almeida QJ, Stough C, Thompson JC, Singrayer R, Jog MS. Visual inspection time in Parkinson's disease: deficits in early stages of cognitive processing. Neuropsychologia. (2004) 42:577–83. doi: 10.1016/j.neuropsychologia.2003.10.011
84. Jurkowski AJ, Stepp E, Hackley SA. Variable foreperiod deficits in Parkinson's disease: dissociation across reflexive and voluntary behaviors. Brain Cogn. (2005) 58:49–61. doi: 10.1016/j.bandc.2004.09.008
85. Vikene K, Skeie GO, Specht K. Subjective judgments of rhythmic complexity in Parkinson's disease: higher baseline, preserved relative ability, and modulated by tempo. PLoS ONE. (2019) 14:e02221752. doi: 10.1371/journal.pone.0221752
86. Vikene K, Skeie GO, Specht K. Abnormal phasic activity in saliency network, motor areas, and basal ganglia in Parkinson's disease during rhythm perception. Hum Brain Mapp. (2019) 40:916–27. doi: 10.1002/hbm.24421
87. Tomassini A, Pollak TA, Edwards MJ, Bestmann S. Learning from the past and expecting the future in Parkinsonism: dopaminergic influence on predictions about the timing of future events. Neuropsychologia. (2019) 127:9–18. doi: 10.1016/j.neuropsychologia.2019.02.003
88. Zokaei N, Gillebert CR, Chauvin JJ, Gresh D, Board AG, Rolinski M, et al. Temporal orienting in Parkinson's disease. Eur J Neurosci. (2021) 53:2713–25. doi: 10.1111/ejn.15114
89. Slagter HA, van Wouwe NC, Kanoff K, Grasman RPPP, Claassen DO, van den Wildenberg WPM, et al. Dopamine and temporal attention: an attentional blink study in Parkinson's disease patients on and off medications. Neuropsychologia. (2016) 91:407–14. doi: 10.1016/j.neuropsychologia.2016.09.006
90. Beudel M, de Geus CM, Leenders KL, de Jong BM. Acceleration bias in visually perceived velocity change and effects of Parkinson's bradykinesia. NeuroReport. (2013) 24:773–8. doi: 10.1097/WNR.0b013e328363f739
91. Bellinger D, Altenmüller E, Volkmann J. Perception of time in music in patients with Parkinson's disease—the processing of musical syntax compensates for rhythmic deficits. Front Neurosci. (2017) 11:68. doi: 10.3389/fnins.2017.00068
92. Conte A, Belvisi D, Tartaglia M, Cortese FN, Baione V, Battista E, et al. Abnormal temporal coupling of tactile perception and motor action in Parkinson's disease. Front Neurol. (2017) 8:249. doi: 10.3389/fneur.2017.00249
93. Jahanshahi M, Jones ERG, Zijlmans J, Katzenschlage R, Lee L, Quinn N, et al. Dopaminergic modulation of striato-frontal connectivity during motor timing in Parkinson's disease. Brain. (2010) 133:727–45. doi: 10.1093/brain/awq012
94. Lee E-Y, Cowan N, Vogel EK, Rolan T, Valle-Inclán F, Haxkley SA. Visual working memory deficits in patients with Parkinson's disease are due to both reduced storage capacity and impaired ability to filter out irrelevant information. Brain. (2010) 133:727–45. doi: 10.1093/brain/awq197
95. Husárová I, Lungu OV, Mareček R, Miki M, Gescheidt T, Krupa P, et al. Functional imaging of the cerebellum and basal ganglia during predictive motor timing in early Parkinson's disease. J Neuroimaging. (2014) 24:45–53. doi: 10.1111/j.1552-6569.2011.00663.x
96. Carlsen AN, Almeida QJ, Franks IM. Using a startling acoustic stimulus to investigate underlying mechanisms of bradykinesia in Parkinson's disease. Neuropsychologia. (2013) 51:392–9. doi: 10.1016/j.neuropsychologia.2012.11.024
97. Miller NS, Kwak Y, Bohnen NI, Müller MLTM, Dayalu P, Seidler RD. The pattern of striatal dopaminergic denervation explains sensorimotor synchronization accuracy in Parkinson's disease. Behav Brain Res. (2013) 257:100–10. doi: 10.1016/j.bbr.2013.09.032
98. De Hemptinne C, Ivanoiu A, Lefèvre P, Missal M. How does Parkinson's disease and aging affect temporal expectation and the implicit timing of eye movements? Neuropsychologia. (2013) 51:340–8. doi: 10.1016/j.neuropsychologia.2012.10.001
99. Bieńkiewicz MMN, Craig CM. Parkinson's is time on your side? Evidence for difficulties with sensorimotor synchronization. Front Neurol. (2015) 6:249. doi: 10.3389/fneur.2015.00249
100. Feher da Silva C, Silva Morgero KC, Manzini Mota A, Pimentel Piemonte ME, Chrysóstomo Baldo MV. Aging and Parkinson's disease as functional models of temporal order perception. Neuropsychologia. (2015) 75:1–9. doi: 10.1016/j.neuropsychologia.2015.09.029
101. Biswas A, Hegde S, Jhunjhunwala K, Kumar Pal P. Two sides of the same coin: impairment in perception of temporal components of rhythm and cognitive function in Parkinson's disease. Basal Ganglia. (2016) 6:63–70. doi: 10.1016/j.baga.2015.12.001
102. Breska A, Ivry RB. Double dissociation of single-interval and rhythmic temporal prediction in cerebellar degeneration and Parkinson's disease. Proc Natl Acad Sci USA. (2018) 115:12283–8. doi: 10.1073/pnas.1810596115
103. Nelson AJ, Hoque T, Gunraj C, Chen R. Altered somatosensory processing in Parkinson's disease and modulation by dopaminergic medications. Parkinsonism Rel Disord. (2018) 53:76–81. doi: 10.1016/j.parkreldis.2018.05.002
104. Yabe Y, Goodale MA, MacDonald PA. Investigating the perceived timing of sensory events triggering actions in patients with Parkinson's disease and the effects of dopaminergic therapy. Cortex. (2019) 115:309–23. doi: 10.1016/j.cortex.2019.02.009
105. Cao R, Ye X, Chen X, Zhang L, Chen X, Tian Y, et al. Exploring biological motion processing in Parkinson's disease using temporal dilation. PLoS ONE. (2015) 10:e0138502. doi: 10.1371/journal.pone.0138502
106. Harrington DL, Castillo GN, Greenberg PA, Song DD, Lessig S, Lee RR, et al. Neurobehavioral mechanisms of temporal processing deficits in Parkinson's disease. PLoS ONE. (2011) 6:e17461. doi: 10.1371/journal.pone.0017461
107. Motta MR, Tumas V, Bueno JLO. Time perception of an artwork's manipulation is distorted by patients with Parkinson's disease. Front Integr Neurosci. (2019) 13:6. doi: 10.3389/fnint.2019.00006
108. Degos B, Ameqrane I, Rivaud-Péchoux S, Pouget P, Missal M. Short-term temporal memory in idiopathic and Parkin-associated Parkinson's disease. Sci Rep. (2018) 16:7637. doi: 10.1038/s41598-018-25751-8
109. Fiorio M, Stanzani C, Rothwell JC, Bhatia KP, Moretto G, Fiaschi A, et al. Defective temporal discrimination of passive movements in Parkinson's disease. Neurosci Lett. (2007) 417:312–5. doi: 10.1016/j.neulet.2007.02.050
110. Lyoo CH, Lee SY, Song TJ, Lee MS. Abnormal temporal discrimination threshold in patients with multiple system atrophy. Mov Disord. (2007) 22:556–9. doi: 10.1002/mds.21111
111. Fiorio M, Valente EM, Gambarin M, Bentivoglio AR, Ialongo T, Albanese A, et al. Subclinical sensory abnormalities in unaffected PINK1 heterozygotes. J Neurol. (2008) 255:1372–7. doi: 10.1007/s00415-008-0923-6
112. Lee M-S, Kim H-S, Lyoo C-H. “Off” gait freezing and temporal discrimination threshold in patients with Parkinson's disease. Neurol. (2005) 64:670–4. doi: 10.1212/01.WNL.0000151961.14861.BA
113. Conte A, Modugno N, Lena F, Dispenza S, Gandolfi B, Iezzi E, et al. Subthalamic nucleus stimulation and somatosensory temporal discrimination in Parkinson's disease. Brain. (2010) 133:2656–63. doi: 10.1093/brain/awq191
114. Lyoo CH, Ryu YH, Lee MJ, Lee MS. Striatal dopamine loss and discriminative sensory dysfunction in Parkinson's disease. Acta Neurol Scand. (2012) 126:344–9. doi: 10.1111/j.1600-0404.2012.01657.x
115. Di Biasio F, Conte A, Bologna M, Iezzi E, Rocchi L, Modugno N. Does the cerebellum intervene in the abnormal temporal discrimination in Parkinson's disease? Parkinsonism Relat Disord. (2015) 21:789–92. doi: 10.1016/j.parkreldis.2015.04.004
116. Conte A, Ferrazzano G, Belvisi D, Manzo N, Battista E, Li Voti P, et al. Somatosensory temporal discrimination in Parkinson's disease, dystonia and essential tremor: pathophysiological and clinical implications. Clin. Neurophysiol. (2018) 29:1849–53. doi: 10.1016/j.clinph.2018.05.024
117. Lee MS, Lee MJ, Conte A, Berardelli A. Abnormal somatosensory temporal discrimination in Parkinson's disease: pathophysiological correlates and role in motor control deficits. Clin Neurophysiol. (2018) 129:442–7. doi: 10.1016/j.clinph.2017.11.022
118. Ivry RB, Spencer RM, Zalaznik HN, Diedrichsen J. The cerebellum and event timing. Ann N Y Acad Sci. (2002) 978:302–17. doi: 10.1111/j.1749-6632.2002.tb07576.x
119. Shin JC, Ivry RB. Spatial and temporal sequence learning in patients with Parkinson's disease or cerebellar lesions. J Cogn Neurosci. (2003) 15:1232–43. doi: 10.1162/089892903322598175
120. Praamstra P, Kourtis D, Kwok HF, Ooostenveld R. Neurophysiology of implicit timing in serial choice reaction-time performance. J Neurosci. (2006) 26:5448–55. doi: 10.1523/JNEUROSCI.0440-06.2006
121. Gallistel CR, Gibbon J. Time, rate, and conditioning. Psychological Rev. (2000) 107:289–344. doi: 10.1037/0033-295X.107.2.289
122. Vierordt Karl. Der Zeitsinn nach Versuchen. Tübingen: Verlag der H. Laupp'schen Buchhandlung (1868).
123. Lejeune H, Wearden JH. Vierordt's the experimental study of the time sense 1868 and its legacy. Eur J Cogn Psychol. (2009) 21:941–60. doi: 10.1080/09541440802453006
124. Glasauer S, Shi Z. The origin of Vierordt's law: the experimental protocol matters. PsyCh Journal. (2021) 10:732–41. doi: 10.1002/pchj.464
125. Jazayeri M, Shadlen MN. A neural mechanism for sensing and reproducing a time interval. Curr Biol. (2015) 25:2599–609. doi: 10.1016/j.cub.2015.08.038
126. Sutton RS. Learning to predict by methods of temporal differences. Mach Learn. (1988) 3:9–44. doi: 10.1007/BF00115009
127. Killeen PR, Fetterman JG. A behavioral theory of timing. Psychol Rev. (1988) 95:274–95. doi: 10.1037/0033-295X.95.2.274
128. Machado A. Learning the temporal dynamics of behavior. Psychol Rev. (1997) 104:241–65. doi: 10.1037/0033-295X.104.2.241
129. Fortin C. Attentional time-sharing in interval timing. In: Meck WH, editor. Functional and Neural Mechanisms of Interval Timing. Boca Raton, FL: CRC Press (2003). p. 235–60.
130. Simen P, Balci F, De Souza L, Cohen JD, Holmes P. A model of interval timing by neural integration. J Neurosci. (2011) 31:9238–53. doi: 10.1523/JNEUROSCI.3121-10.2011
131. Teixeira S, Magalhäes F, Marinho V, Velasques B, Ribeiro P. Proposal for using time estimation training for the treatment of Parkinson's disease. Med Hypotheses. (2016) 95:58–61. doi: 10.1016/j.mehy.2016.08.012
132. Luzardo A, Alonso E, Mondragón E. A rescorla-wagner drift-diffusion model of conditioning and timing. PLoS Comput Biol. (2017) 13:e1005796. doi: 10.1371/journal.pcbi.1005796
133. Ratcliff R, McKoon G. The diffusion decision model: theory and data for two-choice decision tasks. Neural Comput. (2008) 20:873–922. doi: 10.1162/neco.2008.12-06-420
134. Jenkinson N, Brown P. New insights into the relationship between dopamine, beta oscillations and motor function. Trends Neurosci. (2011) 34:611–8. doi: 10.1016/j.tins.2011.09.003
135. López-Azcárate J, Tainta M, Rodríguez-Oroz MC, Valencia M, Gonzáles R, Guiridi J, et al. Coupling between beta and high-frequency activity in the human subthalamic nucleus may be a pathophysiological mechanism in Parkinson's disease. J Neurosci. (2010) 301:6667–77. doi: 10.1523/JNEUROSCI.5459-09.2010
136. Singer W. Synchronization of cortical activity and its putative role in information processing and learning. Ann Rev Physiol. (1993) 55:349–74. doi: 10.1146/annurev.ph.55.030193.002025
137. Nieuwenhuys R. Survey of chemically defined cell groups pathways [section entitled Monoamines: Dopamine]. In: Nieuwenhuys R, editor. Chemoarchitecture of the Brain. Berlin: Springer Verlag (1985). p. 14–8.
138. Björklund A, Dunnett SB. Dopamine neuron systems in the brain: an update. Trends Neurosci. (2007) 30:194–202. doi: 10.1016/j.tins.2007.03.006
139. Jhou TC, Fields HL, Baxter MG, Saper CB, Holland PC. The rostromedial tegmental nucleus (RMTg), a GABAergic afferent to midbrain dopamine neurons, encodes aversive stimuli and inhibits motor responses. Neuron. (2009) 61:786–800. doi: 10.1016/j.neuron.2009.02.001
140. Graybiel AM. Templates for neural dynamics in the striatum: striasomes and matrisomes. In: Shepherd GM and Grillner S, editors. Handbook of Brain Microcircuits 2nd ed. New York, NY: Oxford University Press (2018). p. 133–41.
141. Hikosaka O. The habenula: from stress evasion to value-based decision-making. Nature Rev Neurosci. (2010) 11:503–13. doi: 10.1038/nrn2866
142. Beier KT, Steinberg EE, DeLoach KE, Xie S, Miyamachi K, Schwarz L, et al. Circuit architecture of VTA dopamine neurons revealed by systematic input-output mapping. Cell. (2015) 162:622–34. doi: 10.1016/j.cell.2015.07.015
143. Kitai ST, Shepard PD, Callaway JC, Scroggs R. Afferent modulation of dopamine neuron firing patterns. Curr Opin Neurobiol. (1999) 9:690–7. doi: 10.1016/S0959-4388(99)00040-9
144. Floresco SB, West AR, Ash B, Moore H, Grace AA. Afferent modulation of dopamine neuron firing differentially regulates tonic and phasic dopamine transmission. Nat Neurosci. (2003) 6:968–73. doi: 10.1038/nn1103
145. Cruz BF, Paton JJ. Dopamine gives credit where credit is due. Neuron. (2021) 109:1915–7. doi: 10.1016/j.neuron.2021.05.033
146. Lad SS, Hurley RA, Taber KH. Temporal processing: neural correlates and clinical relevance. J Neurospychiatry Clin Neurosci. (2020) 32, A6:105–8. doi: 10.1176/appi.neuropsych.19120342
Keywords: parkinsonism, dopamine, subjective perception, time estimation, reward prediction
Citation: Miyawaki EK (2022) Review: Subjective Time Perception, Dopamine Signaling, and Parkinsonian Slowness. Front. Neurol. 13:927160. doi: 10.3389/fneur.2022.927160
Received: 23 April 2022; Accepted: 16 June 2022;
Published: 08 July 2022.
Edited by:
Giovanni Abbruzzese, University of Genoa, ItalyReviewed by:
Laura Avanzino, University of Genoa, ItalyLiu Dave Liu, Baylor College of Medicine, United States
Copyright © 2022 Miyawaki. This is an open-access article distributed under the terms of the Creative Commons Attribution License (CC BY). The use, distribution or reproduction in other forums is permitted, provided the original author(s) and the copyright owner(s) are credited and that the original publication in this journal is cited, in accordance with accepted academic practice. No use, distribution or reproduction is permitted which does not comply with these terms.
*Correspondence: Edison K. Miyawaki, ZWRpc29uX21peWF3YWtpQGhtcy5oYXJ2YXJkLmVkdQ==