Corrigendum: A prosperous application of hydrogels with extracellular vesicles release for traumatic brain injury
- Department of Neurosurgery, The Second Affiliated Hospital, Zhejiang University School of Medicine, Hangzhou, China
Traumatic brain injury (TBI) is one of the leading causes of disability worldwide, becoming a heavy burden to the family and society. However, the complexity of the brain and the existence of blood-brain barrier (BBB) do limit most therapeutics effects through simple intravascular injection. Hence, an effective therapy promoting neurological recovery is urgently required. Although limited spontaneous recovery of function post-TBI does occur, increasing evidence indicates that exosomes derived from stem cells promote these endogenous processes. The advantages of hydrogels for transporting drugs and stem cells to target injured sites have been discussed in multitudinous studies. Therefore, the combined employment of hydrogels and exosomes for TBI is worthy of further study. Herein, we review current research associated with the application of hydrogels and exosomes for TBI. We also discuss the possibilities and advantages of exosomes and hydrogels co-therapies after TBI.
Introduction
Every year, more than fifty million individuals worldwide suffer from traumatic brain injury (TBI). One in two people will experience one or more brain injuries of varying degrees in their lifetime (1). Many survivors of TBI left distinct types of permanent neurological deficits, which affect their ability to take care of themselves in their daily lives. TBI brings a heavy economic burden to the family and society. There are constantly new therapeutics verified in preclinical trials. However, no current medical intervention explicitly improves the prognosis of TBI in both preclinical and clinical trials (2). Traditional therapies only aim at several mechanisms of TBI. More importantly, inefficiency and side effects limit the development and use of these treatments.
Nevertheless, the emergence and rapid development of emerging treatment methods in recent years may break the current dilemma. The extracellular matrix (ECM) is a substance with a specific structure and function existing in all tissues and organs. It provides physical support for cells and transduces signals associated with cell proliferation, adhesion, and migration (3). Hydrogels can simulate ECM, and more vitally, can synergize with drugs or cell therapies. At present, the advantages of hydrogels for the treatment of TBI were prominent in many preclinical trials. The development and application of this new material have brought new hope for TBI treatment. Additionally, exosomes (a kind of vesicles with membrane structure) have been proved to play a vital role in intercellular communication. Therefore, research on exosome therapy in TBI also has become a hot topic in recent years.
In this review, we discuss recent advances that describe the basic properties of hydrogels and consider the use of hydrogels for the treatment of TBI and for amplification of effects of drugs and cell therapies to improve neurological recovery after TBI. Moreover, we discuss evidence of how unmodified or modified exosomes affect neurological outcomes when used as therapy for TBI. At last, we prospect opportunities for and challenges in a combined hydrogel-based exosome therapy to applications for TBI. It is worth noting that, as a part of the central nervous system, the situation of spinal cord injury (SCI) is similar to TBI. Therefore, in the review of the application of hydrogels and exosomes, we also include research on the application in SCI.
Pathophysiology of TBI
According to damages of neuronal tissues, TBI can be divided into two main categories: primary injury and secondary injury. Primary injury occurs within seconds to minutes due to direct mechanical forces. The primary injury could lead to hemorrhages such as epidural hematoma, subdural hematoma, subarachnoid hemorrhage, intraventricular hemorrhage; focal cerebral contusions; traumatic axonal injury caused by shearing of white matter tracts; and cerebral edema. The secondary injury occurs after initial injury, characterized by the expansion of damage from the center of the trauma (4). Given the irreversible tissue damage and cell death caused by primary injury, we only focus on the mechanism of secondary injury. So far, numerous factors in secondary injury have been investigated, such as excitotoxicity, oxidative stress, neuroinflammation, apoptosis, axonal degeneration, and formation of cavity and glial scar (5) (Figure 1).
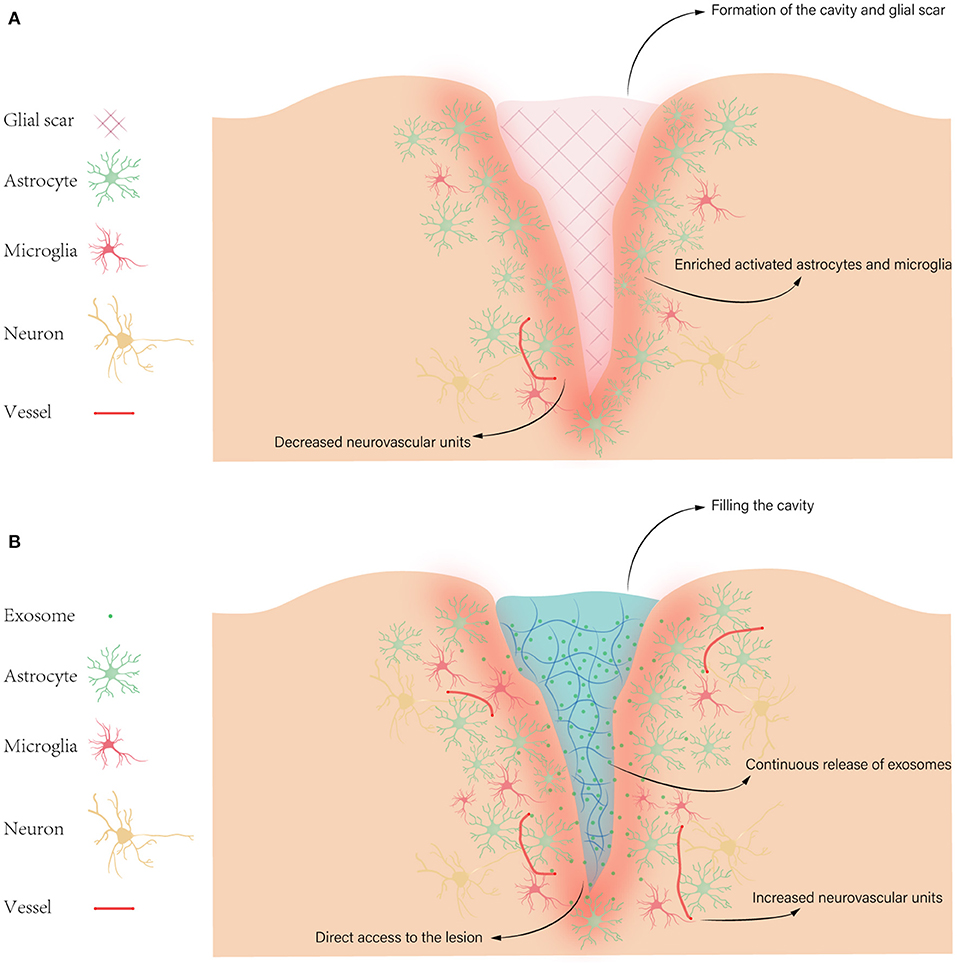
Figure 1. Pathophysiology of TBI. (A) Some pathophysiology of secondary injury after TBI, such as formation of the cavity and glial scar, enriched activated astrocytes and microglia, and decreased neurovascular units. (B) The possible advantages of hydrogels for exosome treatment in TBI, like filling the cavity, direct access to the lesion, increased neurovascular units, and continuous release of exosomes. Then exosomes target various cellular and molecular pathophysiological states, such as excitotoxicity, oxidative stress, neuroinflammation, and apoptosis.
Hydrogels
Hydrogels are cross-linked macromolecular networks that contain a large amount of water, making these materials friendly to water-enriched biological environments (6). Early work on hydrogels can be traced back to the mid-1930s (7). Due to high moisture content and potentials for biomedical applications, hydrogels have received a great deal of attention in recent years. Hydrogels are held together in many ways to form polymeric structures, including chemical bonds and intermolecular forces. Natural polymers such as hyaluronic acid (HA), gelatin, alginates, chitosan, pectin, collagen, and fibrin can be directly employed to prepare hydrogels. However, potential problems like immunogenicity and histocompatibility may limit the applications of natural hydrogels. Additionally, compared with natural hydrogels, synthetic hydrogels have better controllability, histocompatibility, and immunogenicity (8). Therefore, recent articles mainly report synthetic hydrogels.
Structure and Mechanical Properties of Hydrogels
Before preparing a hydrogel, the structure and mechanical properties of the hydrogel should be considered, such as strength, stiffness, mesh size, and porosity. The effects of hydrogels are closely related to their characteristics, such as cytocompatibility, histocompatibility, and the release of therapeutic agents. For instance, the mechanical properties affect the viability and function of encapsulated cells, associating with the efficiency of cell therapy (9–16).
The mechanical strength and stiffness of hydrogels are measured by the compressive modulus directly determined by the cross-link density. And the percent composition of monomers, the polymer molecular mass, and the total amount of cross-linker are closely related to the hydrogels' cross-link density. Therefore, we can adjust the compressive modulus by changing these properties (17–22). The mechanical strength of hydrogels should match the target tissue. As for brain tissue, the ideal hydrogel is soft. In other words, the brain cell prefers the hydrogel with a low compressive modulus (≤3.8 kPa) (15). Many studies have revealed that neurons tend to grow on substrates with softer surfaces (0.1–1.0 kPa), while astrocytes and oligodendrocytes prefer stiffer compressive moduli (0.5–10 kPa) (10, 11, 23–28). These studies demonstrate that the mechanical characteristic of hydrogels plays a vital role in the proliferation and growth of brain cells. Due to the degradation and the loss of cross-links, the compressive modulus of hydrogels will gradually decrease, leading to the destruction of integrity (19, 29, 30). It is rare to see adult brain tissue suffering stretching or shearing force unless under extreme conditions. Thus, we believe that the compressive modulus deserves more attention in the mechanical properties of hydrogel when applied to TBI. As some studies point out that, mild TBI, neuroinflammation, hypertension, and even neural cell migration may cause a rise in the local or overall pressure of brain tissue (31–33).
Mesh size is defined as the distance between the cross-link points in the hydrogel, which is measured in angstroms (Å), usually from 10 to 150Å (8). It is a nanoscopic physical characteristic of the hydrogel, contributing to mechanical properties like stiffness. Given the need for exchanging nutrients and wastes with the surrounding environment, mesh size is a vital agent to consider when designing hydrogels. Moreover, diffusion rates are closely related to the size of the molecule and the mesh, determining the exchange efficiency of fluids and small molecules. By contrast, the migration of larger molecules and cells is affected by the degradation of hydrogels (34, 35).
The function of hydrogels also relates to macroscopic architecture, like interconnected pores or the general shape and size. Some cells and tissues serve functions based on large spaces within hydrogels. Therefore, the pore size is employed to measure the interior space of hydrogels. The formation of the pore can be natural or intentional. Many studies have successfully created varied sizes of pores by various complex methods (36–42). The size of interconnected pores in hydrogels regulates cell-specific growth and differentiation. Two studies prove the neurite outgrowth promoted before hydrogels degradation (41, 43). This characteristic can be deployed in the reconstruction of neural circuits. The general shape should be considered first when designing a hydrogel. Before gelation, most hydrogels will form any shape desired because of their mobility. To reconstruct a neural circuit, the hydrogel can be made as a strand to link two remote regions. The size of hydrogels must be considered due to the limited intracranial space. The swelling of hydrogels mentioned in many studies may lead to a sustained increase in size. But Lampe et al. reported the restrained swelling of hydrogels induced by the counterforce from surrounding brain tissue. And degradation of hydrogels also slowed down the increase in size caused by swelling (44).
Application of Hydrogels in TBI
We review the literature on applications of hydrogels in TBI treatment and divide them into three categories: 1. Hydrogel alone; 2. Hydrogel as a drug delivery tool; 3. Hydrogel as a cell therapy delivery tool. Due to the limited effect, there are few reports about using hydrogel alone. Zhang et al. injected a hydrogel form of urinary bladder matrix (UBM) derived from porcine bladder tissue into the brains of TBI rats. They observed the good biocompatibility of UBM within the brain tissue, the reduced volume of the lesion and the relief of myelin disruption, improving vestibulomotor function (45). Similarly, Yun et al. prepared a hydrogel form of ECM from normal porcine brain tissue to inject into the brains of TBI mice, reducing lesion volume and improving neuro-behavioral function (3). As the focus of this review, we describe the related research of hydrogels in drug and cell therapies in the following part.
Drug Delivery With Hydrogels
As excellent means of transportation with controllable degradation behavior, hydrogels are suitable tools to deliver drugs, playing significant roles at the target site within a specific time according to requirements. Based on secondary injury mechanisms post-TBI, factors such as oxidative stress and neuroinflammation can persist for a long time. Therefore, many studies on drug delivery with hydrogels in TBI use antioxidant or anti-inflammatory drugs (Table 1). Qian et al. developed an injectable TM/PC hydrogel composed of triglycerol monostearate (TM), hydrophobic poly (propylene sulfide) 120 (PPS120) and curcumin. They demonstrated that the TM/PC (PPS120 and curcumin) hydrogel responded effective immediately to the microenvironment after TBI, slowly releasing the drug up to 14 days and significantly reducing the ROS (46). The high porosity of hydrogels provides resident space for molecules and permits the release of molecules from the hydrogels' network at different rates. Thus, the release profile of the molecule can be modulated by mesh size changed by the crosslinking density of hydrogels. Some researchers take advantage of this feature to achieve the sustained release of ferulic acid, significantly inhibiting the oxidative stress enhanced in the early stage after TBI (47).
Compared to intravascular administration, the injectable hydrogels bypass the BBB and direct contact with the injured site. Moreover, the release rate of drugs in hydrogels can be controlled. These characteristics of hydrogels may reduce the amount of drug-using and avoid the occurrence of side effects. It has advantages in the treatment of diseases like TBI where damage factors exist for a prolonged period. Jeong et al. placed dexamethasone in a hydrogel constructed by PEG-bis-AA/HA [photo-cross-linkable poly (ethylene) glycol-bis-(acryloyloxy acetate)/hyaluronic acid], demonstrating that compared with traditional intraperitoneal injection, this new drug delivery strategy markedly reduced the dosage of dexamethasone, as much as half above (48).
The formation of the cystic cavity post-TBI is common. This unfavorable environment is one of the factors inhibiting nerve regeneration. As a cavity implant with great plasticity, the hydrogel can perfectly fill the capsule cavity owing to its physical properties and create a favorable microenvironment beneficial to nerve regeneration and functional recovery post-TBI. He et al. have similar findings in the rat model of spinal cord injury (SCI). As a drug delivery platform, the brain-derived neurotrophic factor-modified hyaluronan-methylcellulose (HAMC) hydrogel slowly released anti-inflammatory peptides and nerve growth factors, which inhibited post-traumatic neuroinflammation and promoted axon regeneration in a longer time (8 weeks) (49). Although the formation of glial scars dominated by reactive astrocytes is essential for limiting the spread of inflammation, it is thought to hinder the regeneration of axons after trauma. Maclean FL et al. have developed a new self-assembling peptide hydrogel carrying anti-inflammatory macromolecule fucoidan. Compared with the untreated group, this hydrogel markedly reduced glial scar formation. On the one hand, the hydrogel filled the cyst left after trauma and supported the surrounding brain tissue, avoiding further damage caused by the collapse of the injured area. On the other hand, the presence of fucoidan reduced reactive astrocytes production (50).
Tissue Engineering Scaffolds From Hydrogels
Stem cell therapy is one of the options in TBI therapies. However, the matrix environment caused by the secondary injury post-TBI is not conducive to the growth and proliferation of stem cells, greatly affecting their survival after transplantation. Further, this harmful environment may induce stem cells, such as endogenous neuro stem cells, to differentiate into astrocytes instead of neurons (51, 52). The possible immunogenicity of transplanted stem cells also limits the application. However, at present, the application of injectable hydrogels in TBI has brought dawn to stem cell therapy. Injectable hydrogels provide a favorable niche for enhancing stem cell therapy by improving the survival rate of stem cells (53). We summarize the application of hydrogels as stem cell scaffolds in TBI (Table 2).
One of the difficulties in stem cell therapy is that the stem cell's growth, proliferation, and differentiation require a suitable microenvironment. But there are great differences in the microenvironment of tissues in the human body. Under pathological conditions, the damaged area accumulates substances unfavorable for the survival of stem cells. Therefore, the survival rate of implanted stem cells has been unsatisfactory for a long time. As mentioned earlier, as a synthetic material, hydrogels have strong plasticity. With the continuous development of material science, we can now build hydrogels with materials with different strengths and stiffness according to tissue specificity. As the scaffold for stem cells, we can change the mesh size and porosity of hydrogels to adapt stem cells in different sizes. The porous structure is also conducive to the exchange of nutrients, oxygen, and carbon dioxide and the emission of metabolites, providing a friendly environment for the survival, proliferation, and diffusion of stem cells (58). Yao et al. believe stem cell scaffolds should have a series of basic features that support the growth of transplanted cells and match the microenvironment of brain tissue, including rapid gelation process, high water content, porosity, and appropriate rheological behavior and degradation properties. The injectable hydrogel synthesized in this way will have low immunogenicity and cause minimal inflammation in vivo. For instance, the gelatin-hydroxyphenyl hydrogel of GO0.1UHRP0.5U has sufficient moisture (more than 90%) and appropriate rheological properties (100–1,000 Pa), meeting the physiological characteristics of brain tissue and reducing frictional stimulation to surrounding tissues. Proper gelation time (6 min) also avoids the loss of stem cells caused by longer gelation progress (56). Zhang et al. point out that the rapid flow of cerebrospinal fluid, the mechanical injury, and the reactive inflammation at the lesion induce the high loss rate and low survival rate of transplanted cells (57). Hydrogels mimic the function of the extracellular matrix. The porous structure and suitable pore size improve the survival rate of stem cells and affect the behavior of transplanted cells, such as proliferation and differentiation, promoting the growth of stem cells. Moreover, Xue et al. have tried co-transplantation of neural stem cells and endothelial progenitor cells. Results showed that revascularization, tissue repair, and generation of neural cells were all improved (62).
Another advantage of hydrogels in stem cell therapy is that hydrogels can carry with other macromolecular substances or be modified by certain chemical groups during the synthesis process. These macromolecular substances or chemical groups give hydrogels additional functions, supporting the cellular behaviors of stem cells. For example, SDF-1 and its receptor CXCR4 are key molecules for the survival, migration, and differentiation of many stem cells like neural stem cells or mesenchymal stem cells. Therefore, some employed the slow-release property of SDF-1 in the pores of hydrogels to provide a suitable microenvironment for the survival, migration, and differentiation of transplanted cells (53, 55, 61, 63). Immunogenicity is one of the reasons for the low survival rate of transplanted stem cells. Some researchers have made full use of this advantage of hydrogels to reduce immune rejection. Alvarado-Velez et al. designed a FasL-agarose hydrogel. The sustained release of FasL at the injury site induced apoptosis of CD8+ T cells, improving the survival rate of mesenchymal stem cells (MSCs). Meanwhile, they demonstrated that the neurotrophic factors NGF and BDNF significantly upregulated when used FasL-agarose hydrogel, indicating a better therapeutic effect promoted by prolonged MSC survival (54). However, some have adopted distinct design ideas. Betancur et al. constructed a CS-GAG hydrogel to maintain the undifferentiated state of neural stem cells within 4 weeks. Undifferentiated stem cells inhibited the proliferation of reactive astrocytes post-TBI through paracrine action (60). Xu et al. constructed a hydrogel containing semaphorin 3A (Sema3A) gradient, inducing substantial migration of endogenous neural progenitor cells into the hydrogel and promoting their differentiation to regenerate cortical tissue (64).
Exosome
Current research suggests that exosomes as endosome-derived membrane-bound vesicles can be released by cells in all living systems under any conditions (65). Exosomes are composed of uniform lipid bilayer membranes, containing several vital proteins, including CD63, CD8, CD9, and endosomal membrane proteins flotillin and ALIX. The most significant function of exosomes is carrying macromolecular substances such as proteins, lipids, and nucleic acids to participate in intercellular communication. Among the cargo of exosomes, miRNA is the most well-studied. And there is substantial evidence that miRNA is central to the treatment effects of exosomes. Mature miRNAs have a double-stranded structure, one or both of them will bind to argonaute 2 (Ago2) and be integrated into the RNA-induced silencing complex to cleave the target mRNA or inhibit translational repression (66–68).
Several types of neural cells release exosomes. The glutamatergic synaptic activity in mature neurons promotes the release of exosomes which carry with molecules to modulate neuronal function (65). Exosomes participate in the communication between neurons and endothelial cells, regulating the BBB (59). Oligodendrocytes and astrocytes also communicate with neurons through exosomes (69). The release of exosomes in oligodendrocytes and astrocytes is regulated by the level of cytoplasmic calcium and the concentration of potassium chloride, respectively. And glutamate released by neurons increases exosomes production in oligodendrocytes (70, 71). The exosomes containing the myelin proteins from oligodendrocytes are often around axons, coordinating axon myelination (70, 72, 73). Moreover, oligodendrocyte exosomes transfer superoxide dismutase and catalase to neurons to increase the vitality of neurons under hypoxia-glucose deprivation (OGD) conditions (72). There is evidence indicating that the extensive action of astrocytes depends on the exosomes. The exosomes carrying miR-26 released by astrocytes may inhibit glycogen synthase kinase 3β, an effective inhibitor of axon remodeling and synaptic plasticity (74, 75). In addition, some evidence suggests that astrocytes exosomes transfer prion protein (PrP) to hypoxia and glucose deprivation neurons to prevent neuronal death (76).
It was recorded for the first time that intravenous injection of MSC-derived exosomes in rodent models with TBI significantly increased neurovascular remodeling at the site of the lesion, improving the neurological, behavioral, and cognitive outcomes. The therapeutic gain is equivalent to MSC therapy (77–79). Immunogenicity or histocompatibility also should be considered for exosome therapy. Although there is currently limited data reporting noticeable adverse immune reactions (65).
Exosome therapy is currently commonly administered via intravenous or nasal. Many related studies support that under the existing route of administration, exosomes need to cross the BBB to interact with target cells in brain tissue (80–84). Systemic administration of exosomes may promote endogenous neural circuitry reconstruction, white matter remodeling, oligodendrogenesis, angiogenesis, and neurogenesis to improve neurological outcomes. In vitro studies show that exosomes derived from MSCs or fibroblasts directly strengthen the outgrowth of dendrites and axons (85, 86). The exosomes from brain endothelial cells of rodents and humans and neural stem cells of adult rodents have been observed promoting neurogenesis and angiogenesis in the process of injury recovery (87–89). In addition to directly affecting nerve repair, exosomes also have indirect effects. In a cerebral ischemia rat model, exogenous exosomes from MSC stimulate astrocytes to release endogenous exosomes assisting exogenous exosomes to promote the growth of cortical neurons (90). Many studies have indicated that the intercellular communication of exosomes participated in the inflammation after brain injury (91–93). And the neuroinflammation was downregulated by injecting MSC-derived exosomes (94, 95). The preclinical study of the TBI model receiving exosome therapy also describes the improved neurological function during the recovery period (92). Moreover, exosomes transfer microglia/macrophages toward anti-inflammatory phenotypes directly by macromolecular substances, such as miRNAs and proteins, or indirectly by upregulating pro-inflammatory factors, such as TNF-α and IL-1β (83, 86, 89, 90, 96).
The main challenge of TBI exosome therapy currently is to develop exosomes targeting specific cells in the central nervous system more effectively than cell-derived exosomes. The application of virus-derived peptides makes it possible for exosomes to target specific cells. Exosomes expressing virus-derived peptides can span the BBB, binding to the corresponding receptors on the surface of cells and importing molecules such as miRNA and siRNA (97–99). However, the virus-derived peptides perhaps induce immune response (100). Studies have shown that exosomes modified by chemically synthesized peptides could avoid immunogenicity (101, 102). Apparently, due to the capacity of carrying and editing, treatment methods based on exosomes have unlimited possibilities.
Application Prospects of Hydrogel With Exosomes in TBI Therapies
Although some studies have proved that exosomes take effects by crossing the BBB and targeting specific cells, there is evidence suggesting that the existing methods of administration fail to improve the prognosis of TBI (103). An early study finished by Harting et al. using intravenous injection of BMSC-derived exosomes indicated that only a few exosomes would reach the brain (104). In addition, under the traditional route of administration, exosomes have short duration of action and require frequent administration to achieve therapeutic effects, limiting clinical transformation. Because of these limitations, it is worthy to improve the delivery method of exosomes.
As we reviewed above, hydrogels have been extensively studied as scaffolds for stem cell therapy of TBI, obtaining many positive results. And the method of encapsulating exosomes with hydrogels has been applied in several fields such as bone and cartilage regeneration (105, 106), diabetic chronic wound healing (107, 108), and cardiac repair (109, 110). Moreover, some research on spinal cord injury (SCI) repair attempting to embed exosomes into injectable hydrogels confirmed the effective retention and slow release of exosomes at the target site, with enhanced angiogenesis and neural function (111–114).
Here, we summarize the possible advantages of hydrogels for exosome treatment in TBI: 1. Direct access to the lesion. Compared to the application of exosomes via intravenous or nasal routes, in situ injections of hydrogels can directly reach the lesion and act at the injured site, avoiding the influence of BBB, and improving the treatment efficiency of exosomes. 2. Filling the cyst cavity. The formation of the cystic cavity post-TBI is quite common, unfavorable for nerve repair and regeneration at the injured site. It is difficult to change this with the traditional route of administration of exosomes, while hydrogels can perfectly fill the cyst cavity and provide a better microenvironment for nerve regeneration. 3. Editability. As a synthetic material, hydrogels have excellent editability. Its pore size can be controlled to adapt to varied sizes of exosomes by selecting various materials and changing synthesis conditions. In addition, it is foreseeable that by selecting materials with different characteristics or adding different chemical group modifications, hydrogels can also obtain additional capacities, such as regulating the release conditions and release curves of exosomes and creating a favorable microenvironment. Recently, Staufer et al. developed a fully synthetic extracellular vesicle and demonstrated its therapeutic function, remarking a new level of editability of exosomes (115). In the future, by combining the two highly programmable tools of hydrogels and exosomes, we have great reason to believe that the treatment of TBI will usher in the dawn.
Of course, there are also foreseeable drawbacks in therapy of using hydrogels and exosomes. 1. Although the advantage of hydrogels in the treatment of brain trauma is to fill the cavity formed after trauma and directly contact the lesion, the accompanying side effect of hydrogels may be the mechanical damage to the surrounding brain tissue. Compared with the traditional covalent gel, the dynamic hydrogel is injectable and gelling in situ, substantially reducing mechanical damage during implantation. However, injectable hydrogels are not perfect. These hydrogels usually experience problems with gelation kinetics, such as coagulating too quickly in the syringe, gelling so slow that the drugs release prematurely, or heterogeneous gelation caused by poor mixing. Therefore, dynamic hydrogels capable of seamlessly transition between solid-like and liquid-like during injection draw much attention (6). 2. Risks of the implant. Most hydrogel matrices used recently are synthetic materials, reducing the risk of rejection after implantation compared with bio-derived materials. However, risks of hydrogels still exist objectively, such as immune response and infection. As for exosomes, immune reaction may also be a disadvantage of our concern, as described above in this review. 3. In addition, injectable hydrogels still have problems to be solved before clinical trials. For example, most researchers directly left empty cavities in TBI animal models, injecting hydrogels into them. Although they present positive results, it only illustrates that injectable hydrogels are suitable for TBI patients who need surgery, which undoubtedly restricts the application of hydrogels. However, the majority of TBI patients only take conservative treatment in clinical. For such patients, the validity and safety of injectable hydrogels need more vivo trials.
Although the injectable hydrogel reduces mechanical damage during implantation compared with the traditional gel, the possibility of mechanical damage still exists objectively. We believe that this damage is due to the material properties of hydrogels. Some characteristics can help us evaluate and reduce the mechanical damage. 1. The mechanical strength of hydrogels. Matthew reveals that if the storage moduli of the hydrogel similar to the brain (140–620 Pa), the hydrogel can maintain tight apposition to brain tissue (55). In addition, to prevent it from being squeezed out by the surrounding tissue, the deformation resistance of the hydrogel can be adjusted by the chemically modified matrix. Yao et al. also proposes that the hydrogel possesses high water content (>90%) and appropriate rheological behavior (100–1,000 Pa) can reduce the frictional irritation to the surrounding tissue (56). 2. Swelling behavior. When certain materials such as HA are used for hydrogel preparation, the hydrogel shows water intake capacity. The rapid and high swelling behavior may stress the surrounding tissues, causing secondary mechanical damage (116). However, we can choose a different material ratio to synthesize hydrogels with appropriate swelling ability to reduce this type of damage.
Based on the experience of hydrogels with drugs or stem cell therapies before, the ideal characteristics for the hydrogel include the following: 1. Be injectable and be able to gel in situ. 2. Mechanical properties comparable to brain tissue to minimize mechanical injury. 3. Be biocompatible and biodegradable to reduce risks of immune response and support tissue regeneration. 4. Be made of a material that appropriate mesh size allows sustained release of exosomes (117).
Conclusion
We briefly review the pathophysiological mechanism of TBI and describe the potential new treatment strategies for TBI. The therapies for TBI are problematic due to the modes of drug or cell delivery. Hydrogels have the potential of conveying drugs or cells to the target injured sites and improving their effects. The therapeutic gain of exosome therapy may be equivalent to stem cell therapy. And the low immunogenicity of exosomes suggests that the adverse effects associated with current stem cell therapies can be reduced. Evidence from studies regarding the employment of exosomes and hydrogels co-therapies in TBI are lacking. Although we believe co-therapies employing exosomes and hydrogels can potentially ameliorate TBI, it still needs to be confirmed by thorough multitudinous studies in the future.
Author Contributions
YC, JL, and WY: conceptualization. YC: writing—original draft preparation. JL and WY: writing—review and editing. All authors have read and agreed to the published version of the manuscript.
Conflict of Interest
The authors declare that the research was conducted in the absence of any commercial or financial relationships that could be construed as a potential conflict of interest.
Publisher's Note
All claims expressed in this article are solely those of the authors and do not necessarily represent those of their affiliated organizations, or those of the publisher, the editors and the reviewers. Any product that may be evaluated in this article, or claim that may be made by its manufacturer, is not guaranteed or endorsed by the publisher.
References
1. Maas A, Menon DK, Adelson PD, Andelic N, Bell MJ, Belli A, et al. Traumatic brain injury: integrated approaches to improve prevention, clinical care, and research. Lancet Neurol. (2017) 16:987–1048. doi: 10.1016/S1474-4422(17)30371-X
2. Yang Y, Yang H, Yang Y, Ma Y. Exosomal microRNAs have great potential in the neurorestorative therapy for traumatic brain injury. Exp Neurol. (2022) 352:114026. doi: 10.1016/j.expneurol.2022.114026
3. Wu Y, Wang J, Shi Y, Pu H, Leak RK, Liou A, et al. Implantation of brain-derived extracellular matrix enhances neurological recovery after traumatic brain injury. Cell Transplant. (2017) 26:1224–34. doi: 10.1177/0963689717714090
4. Jarrahi A, Braun M, Ahluwalia M, Gupta RV, Wilson M, Munie S, et al. Revisiting traumatic brain injury: from molecular mechanisms to therapeutic interventions. Biomedicines. (2020) 8:389. doi: 10.3390/biomedicines8100389
5. Ng SY, Lee A. Traumatic brain injuries: pathophysiology and potential therapeutic targets. Front Cell Neurosci. (2019) 13:528. doi: 10.3389/fncel.2019.00528
6. Correa S, Grosskopf AK, Lopez Hernandez H, Chan D, Yu AC, Stapleton LM, et al. Translational applications of hydrogels. Chem Rev. (2021) 121:11385–457. doi: 10.1021/acs.chemrev.0c01177
7. Peppas NA, Hoffman AS. Hydrogels. In: Wagner WR, Sakiyama-Elbert SE, Zhang G, Yaszemski MJ, editors. Biomaterials Science. 4th ed., Academic Press (2020). p. 153–66. doi: 10.1016/B978-0-12-816137-1.00014-3
8. Aurand ER, Lampe KJ, Bjugstad KB. Defining and designing polymers and hydrogels for neural tissue engineering. Neurosci Res. (2012) 72:199–213. doi: 10.1016/j.neures.2011.12.005
9. Bryant SJ, Anseth KS. Hydrogel properties influence ECM production by chondrocytes photoencapsulated in poly (ethylene glycol) hydrogels. J Biomed Mater Res. (2002) 59:63–72. doi: 10.1002/jbm.1217
10. Flanagan LA, Ju YE, Marg B, Osterfield M, Janmey PA. Neurite branching on deformable substrates. Neuroreport. (2002) 13:2411–5. doi: 10.1097/00001756-200212200-00007
11. Engler AJ, Sen S, Sweeney HL, Discher DE. Matrix elasticity directs stem cell lineage specification. Cell. (2006) 126:677–89. doi: 10.1016/j.cell.2006.06.044
12. Kraehenbuehl TP, Zammaretti P, Van der Vlies AJ, Schoenmakers RG, Lutolf MP, Jaconi ME, et al. Three-dimensional extracellular matrix-directed cardioprogenitor differentiation: systematic modulation of a synthetic cell-responsive PEG-hydrogel. Biomaterials. (2008) 29:2757–66. doi: 10.1016/j.biomaterials.2008.03.016
13. Comolli N, Neuhuber B, Fischer I, Lowman A. In vitro analysis of PNIPAAm-PEG, a novel, injectable scaffold for spinal cord repair. Acta Biomater. (2009) 5:1046–55. doi: 10.1016/j.actbio.2008.10.008
14. Chatterjee K, Lin-Gibson S, Wallace WE, Parekh SH, Lee YJ, Cicerone MT, et al. The effect of 3D hydrogel scaffold modulus on osteoblast differentiation and mineralization revealed by combinatorial screening. Biomaterials. (2010) 31:5051–62. doi: 10.1016/j.biomaterials.2010.03.024
15. Lampe KJ, Mooney RG, Bjugstad KB, Mahoney MJ. Effect of macromer weight percent on neural cell growth in 2D and 3D nondegradable PEG hydrogel culture. J Biomed Mater Res Part A. (2010) 94:1162–71. doi: 10.1002/jbm.a.32787
16. Young JL, Engler AJ. Hydrogels with time-dependent material properties enhance cardiomyocyte differentiation in vitro. Biomaterials. (2011) 32:1002–9. doi: 10.1016/j.biomaterials.2010.10.020
17. Anseth KS, Bowman CN, Brannon-Peppas L. Mechanical properties of hydrogels and their experimental determination. Biomaterials. (1996) 17:1647–1657. doi: 10.1016/0142-9612(96)87644-7
18. He S, Yaszemski MJ, Yasko AW, Engel PS, Mikos AG. Injectable biodegradable polymer composites based on poly(propylene fumarate) crosslinked with poly(ethylene glycol)-dimethacrylate. Biomaterials. (2000) 21:2389–94. doi: 10.1016/S0142-9612(00)00106-X
19. Anseth KS, Metters AT, Bryant SJ, Martens PJ, Elisseeff JH, Bowman CN. In situ forming degradable networks and their application in tissue engineering and drug delivery. J Control Release. (2002) 78:199–209. doi: 10.1016/S0168-3659(01)00500-4
20. Sawhney AS, Pathak CP, Hubbell JA. Bioerodible hydrogels based on photopolymerized poly(ethylene glycol)-co-poly(.alpha.-hydroxy acid) diacrylate macromers. Macromolecules. (2002) 26:581–7. doi: 10.1021/ma00056a005
21. Martens PJ, Bryant SJ, Anseth KS. Tailoring the degradation of hydrogels formed from multivinyl poly(ethylene glycol) and poly(vinyl alcohol) macromers for cartilage tissue engineering. Biomacromolecules. (2003) 4:283–92. doi: 10.1021/bm025666v
22. Lin CC, Anseth KS. PEG hydrogels for the controlled release of biomolecules in regenerative medicine. Pharm Res. (2009) 26:631–43. doi: 10.1007/s11095-008-9801-2
23. Georges PC, Miller WJ, Meaney DF, Sawyer ES, Janmey PA. Matrices with compliance comparable to that of brain tissue select neuronal over glial growth in mixed cortical cultures. Biophys J. (2006) 90:3012–8. doi: 10.1529/biophysj.105.073114
24. Brännvall K, Bergman K, Wallenquist U, Svahn S, Bowden T, Hilborn J, et al. Enhanced neuronal differentiation in a three-dimensional collagen-hyaluronan matrix. J Neurosci Res. (2007) 85:2138–46. doi: 10.1002/jnr.21358
25. Saha K, Keung AJ, Irwin EF, Li Y, Little L, Schaffer DV, et al. Substrate modulus directs neural stem cell behavior. Biophys J. (2008) 95:4426–38. doi: 10.1529/biophysj.108.132217
26. Banerjee A, Arha M, Choudhary S, Ashton RS, Bhatia SR, Schaffer DV, et al. The influence of hydrogel modulus on the proliferation and differentiation of encapsulated neural stem cells. Biomaterials. (2009) 30:4695–9. doi: 10.1016/j.biomaterials.2009.05.050
27. Hynes SR, Rauch MF, Bertram JP, Lavik EB. A library of tunable poly(ethylene glycol)/poly(L-lysine) hydrogels to investigate the material cues that influence neural stem cell differentiation. J Biomed Mater Res Part A. (2009) 89:499–509. doi: 10.1002/jbm.a.31987
28. Seidlits SK, Khaing ZZ, Petersen RR, Nickels JD, Vanscoy JE, Shear JB, et al. The effects of hyaluronic acid hydrogels with tunable mechanical properties on neural progenitor cell differentiation. Biomaterials. (2010) 31:3930–40. doi: 10.1016/j.biomaterials.2010.01.125
29. Metters A. Fundamental studies of a novel, biodegradable PEG-b-PLA hydrogel. Polymer. (2000) 41:3993–4004. doi: 10.1016/S0032-3861(99)00629-1
30. Hawkins AM, Milbrandt TA, Puleo DA, Hilt JZ. Synthesis and analysis of degradation, mechanical and toxicity properties of poly(β-amino ester) degradable hydrogels. Acta Biomater. (2011) 7:1956–64. doi: 10.1016/j.actbio.2011.01.024
31. Cayre M, Canoll P, Goldman JE. Cell migration in the normal and pathological postnatal mammalian brain. Prog Neurobiol. (2009) 88:41–63. doi: 10.1016/j.pneurobio.2009.02.001
32. Broder JS. Head computed tomography interpretation in trauma: a primer. Psychiatr Clin N Am. (2010) 33:821–54. doi: 10.1016/j.psc.2010.08.006
33. Yuh EL, Dillon WP. Intracranial hypotension and intracranial hypertension. Neuroimaging Clin N Am. (2010) 20:597–617. doi: 10.1016/j.nic.2010.07.012
34. Jeong B, Bae YH, Lee DS, Kim SW. Biodegradable block copolymers as injectable drug-delivery systems. Nature. (1997) 388:860–2. doi: 10.1038/42218
35. Bjugstad KB, Lampe K, Kern DS, Mahoney M. Biocompatibility of poly(ethylene glycol)-based hydrogels in the brain: an analysis of the glial response across space and time. J Biomed Mater Res Part A. (2010) 95:79–91. doi: 10.1002/jbm.a.32809
36. Guan J, Fujimoto KL, Sacks MS, Wagner WR. Preparation and characterization of highly porous, biodegradable polyurethane scaffolds for soft tissue applications. Biomaterials. (2005) 26:3961–71. doi: 10.1016/j.biomaterials.2004.10.018
37. Stachowiak AN, Bershteyn A, Tzatzalos E, Irvine DJ. Bioactive hydrogels with an ordered cellular structure combine interconnected macroporosity and robust mechanical properties. Adv Mater. (2005) 17:399–403. doi: 10.1002/adma.200400507
38. Katz JS, Burdick JA. Hydrogel mediated delivery of trophic factors for neural repair. Wiley Interdiscip Rev Nanomed Nanobiotechnol. (2009) 1:128–39. doi: 10.1002/wnan.10
39. Keskar V, Gandhi M, Gemeinhart EJ, Gemeinhart RA. Initial evaluation of vascular ingrowth into superporous hydrogels. J Tissue Eng Regen Med. (2009) 3:486–90. doi: 10.1002/term.183
40. Keskar V, Marion NW, Mao JJ, Gemeinhart RA. In vitro evaluation of macroporous hydrogels to facilitate stem cell infiltration, growth, and mineralization. Tissue Eng Part A. (2009) 15:1695–707. doi: 10.1089/ten.tea.2008.0238
41. Namba RM, Cole AA, Bjugstad KB, Mahoney MJ. Development of porous PEG hydrogels that enable efficient, uniform cell-seeding and permit early neural process extension. Acta Biomater. (2009) 5:1884–97. doi: 10.1016/j.actbio.2009.01.036
42. Spiller KL, Holloway JL, Gribb ME, Lowman AM. Design of semi-degradable hydrogels based on poly(vinyl alcohol) and poly(lactic-co-glycolic acid) for cartilage tissue engineering. J Tissue Eng Regen Med. (2011) 5:636–47. doi: 10.1002/term.356
43. Zhong J, Chan A, Morad L, Kornblum HI, Fan G, Carmichael ST. Hydrogel matrix to support stem cell survival after brain transplantation in stroke. Neurorehabil Neural Repair. (2010) 24:636–44. doi: 10.1177/1545968310361958
44. Lampe KJ, Kern DS, Mahoney MJ, Bjugstad KB. The administration of BDNF and GDNF to the brain via PLGA microparticles patterned within a degradable PEG-based hydrogel: Protein distribution and the glial response. J Biomed Mater Res Part A. (2011) 96:595–607. doi: 10.1002/jbm.a.33011
45. Zhang L, Zhang F, Weng Z, Brown BN, Yan H, Ma XM, et al. Effect of an inductive hydrogel composed of urinary bladder matrix upon functional recovery following traumatic brain injury. Tissue Eng Part A. (2013) 19:1909–18. doi: 10.1089/ten.tea.2012.0622
46. Qian F, Han Y, Han Z, Zhang D, Zhang L, Zhao G, et al. In situ implantable, post-trauma microenvironment-responsive, ROS depletion hydrogels for the treatment of traumatic brain injury. Biomaterials. (2021) 270:120675. doi: 10.1016/j.biomaterials.2021.120675
47. Dong GC, Kuan CY, Subramaniam S, Zhao JY, Sivasubramaniam S, Chang HY, et al. A potent inhibition of oxidative stress induced gene expression in neural cells by sustained ferulic acid release from chitosan based hydrogel. Mater Sci Eng C Mater Biol Applic. (2015) 49:691–699. doi: 10.1016/j.msec.2015.01.030
48. Jeong DU, Bae S, Macks C, Whitaker J, Lynn M, Webb K, et al. Hydrogel-mediated local delivery of dexamethasone reduces neuroinflammation after traumatic brain injury. Biomed Mater. (2021) 16:035002. doi: 10.1088/1748-605X/abc7f1
49. He Z, Zang H, Zhu L, Huang K, Yi T, Zhang S, et al. An anti-inflammatory peptide and brain-derived neurotrophic factor-modified hyaluronan-methylcellulose hydrogel promotes nerve regeneration in rats with spinal cord injury. Int J Nanomed. (2019) 14:721–32. doi: 10.2147/IJN.S187854
50. Maclean FL, Wang Y, Walker R, Horne MK, Williams RJ, Nisbet DR. Reducing astrocytic scarring after traumatic brain injury with a multifaceted anti-inflammatory hydrogel system. ACS Biomater Sci Eng. (2017) 3:2542–9. doi: 10.1021/acsbiomaterials.7b00524
51. Fan B, Wei Z, Yao X, Shi G, Cheng X, Zhou X, et al. Microenvironment imbalance of spinal cord injury. Cell Transplant. (2018) 27:853–66. doi: 10.1177/0963689718755778
52. Mukherjee N, Adak A, Ghosh S. Recent trends in the development of peptide and protein-based hydrogel therapeutics for the healing of CNS injury. Soft Matter. (2020) 16:10046–64. doi: 10.1039/D0SM00885K
53. Ma S, Zhou J, Huang T, Zhang Z, Xing Q, Zhou X, et al. Sodium alginate/collagen/stromal cell-derived factor-1 neural scaffold loaded with BMSCs promotes neurological function recovery after traumatic brain injury. Acta Biomater. (2021) 131:185–97. doi: 10.1016/j.actbio.2021.06.038
54. Alvarado-Velez M, Enam SF, Mehta N, Lyon JG, LaPlaca MC, Bellamkonda RV. Immuno-suppressive hydrogels enhance allogeneic MSC survival after transplantation in the injured brain. Biomaterials. (2021) 266:120419. doi: 10.1016/j.biomaterials.2020.120419
55. Zheng Y, Wu G, Chen L, Zhang Y, Luo Y, Zheng Y, et al. Neuro-regenerative imidazole-functionalized GelMA hydrogel loaded with hAMSC and SDF-1α promote stem cell differentiation and repair focal brain injury. Bioact Mater. (2020) 6:627–37. doi: 10.1016/j.bioactmat.2020.08.026
56. Yao M, Gao F, Xu R, Zhang J, Chen Y, Guan F. A dual-enzymatically cross-linked injectable gelatin hydrogel loaded with BMSC improves neurological function recovery of traumatic brain injury in rats. Biomater Sci. (2019) 7:4088–98. doi: 10.1039/C9BM00749K
57. Zhang K, Shi Z, Zhou J, Xing Q, Ma S, Li Q, et al. Potential application of an injectable hydrogel scaffold loaded with mesenchymal stem cells for treating traumatic brain injury. J Mater Chem B. (2018) 6:2982–92. doi: 10.1039/C7TB03213G
58. Jahanbazi Jahan-Abad A, Sahab Negah S, Hosseini Ravandi H, Ghasemi S, Borhani-Haghighi M, Stummer W, et al. Human neural stem/progenitor cells derived from epileptic human brain in a self-assembling peptide nanoscaffold improve traumatic brain injury in rats. Mol Neurobiol. (2018) 55:9122–38. doi: 10.1007/s12035-018-1050-8
59. Xu B, Zhang Y, Du XF, Li J, Zi HX, Bu JW, et al. Neurons secrete miR-132-containing exosomes to regulate brain vascular integrity. Cell Res. (2017) 27:882–97. doi: 10.1038/cr.2017.62
60. Betancur MI, Mason HD, Alvarado-Velez M, Holmes PV, Bellamkonda RV, Karumbaiah L. Chondroitin sulfate glycosaminoglycan matrices promote neural stem cell maintenance and neuroprotection post-traumatic brain injury. ACS Biomater Sci Eng. (2017) 3:420–30. doi: 10.1021/acsbiomaterials.6b00805
61. Shi W, Huang CJ, Xu XD, Jin GH, Huang RQ, Huang JF. Transplantation of RADA16-BDNF peptide scaffold with human umbilical cord mesenchymal stem cells forced with CXCR4 and activated astrocytes for repair of traumatic brain injury. Acta Biomater. (2016) 45:247–61. doi: 10.1016/j.actbio.2016.09.001
62. Xue S, Wu G, Zhang HT, Guo YW, Zou YX, Zhou ZJ, et al. Transplantation of adipocyte-derived stem cells in a hydrogel scaffold for the repair of cortical contusion injury in rats. J Neurotrauma. (2015) 32:506–15. doi: 10.1089/neu.2014.3480
63. Addington CP, Heffernan JM, Millar-Haskell CS, Tucker EW, Sirianni RW, Stabenfeldt SE. Enhancing neural stem cell response to SDF-1α gradients through hyaluronic acid-laminin hydrogels. Biomaterials. (2015) 72:11–9. doi: 10.1016/j.biomaterials.2015.08.041
64. Xu Z, Wang W, Ren Y, Zhang W, Fang P, Huang L, et al. Regeneration of cortical tissue from brain injury by implantation of defined molecular gradient of semaphorin 3A. Biomaterials. (2018) 157:125–35. doi: 10.1016/j.biomaterials.2017.12.010
65. Zhang ZG, Buller B, Chopp M. Exosomes - beyond stem cells for restorative therapy in stroke and neurological injury. Nat Rev Neurol. (2019) 15:193–203. doi: 10.1038/s41582-018-0126-4
66. Macfarlane LA, Murphy PR. MicroRNA: biogenesis, function and role in cancer. Curr Genomics. (2010) 11:537–61. doi: 10.2174/138920210793175895
67. Meister G. Argonaute proteins: functional insights and emerging roles. Nat Rev Genet. (2013) 14:447–59. doi: 10.1038/nrg3462
68. Mantel PY, Hjelmqvist D, Walch M, Kharoubi-Hess S, Nilsson S, Ravel D, et al. Infected erythrocyte-derived extracellular vesicles alter vascular function via regulatory Ago2-miRNA complexes in malaria. Nat Commun. (2016) 7:12727. doi: 10.1038/ncomms12727
69. Borroto-Escuela DO, Agnati LF, Bechter K, Jansson A, Tarakanov AO, Fuxe K. The role of transmitter diffusion and flow versus extracellular vesicles in volume transmission in the brain neural-glial networks. Philos Trans R Soc Lond Ser B Biol Sci. (2015) 370:20140183. doi: 10.1098/rstb.2014.0183
70. Krämer-Albers EM, Bretz N, Tenzer S, Winterstein C, Möbius W, Berger H, et al. Oligodendrocytes secrete exosomes containing major myelin and stress-protective proteins: trophic support for axons?. Proteomics Clin Appl. (2007) 1:1446–1461. doi: 10.1002/prca.200700522
71. Wang S, Cesca F, Loers G, Schweizer M, Buck F, Benfenati F, et al. Synapsin I is an oligomannose-carrying glycoprotein, acts as an oligomannose-binding lectin, and promotes neurite outgrowth and neuronal survival when released via glia-derived exosomes. J Neurosci. (2011) 31:7275–90. doi: 10.1523/JNEUROSCI.6476-10.2011
72. Frühbeis C, Fröhlich D, Kuo WP, Amphornrat J, Thilemann S, Saab AS, et al. Neurotransmitter-triggered transfer of exosomes mediates oligodendrocyte-neuron communication. PLoS Biol. (2013) 11:e1001604. doi: 10.1371/journal.pbio.1001604
73. Frühbeis C, Fröhlich D, Kuo WP, Krämer-Albers EM. Extracellular vesicles as mediators of neuron-glia communication. Front Cell Neurosci. (2013) 7:182. doi: 10.3389/fncel.2013.00182
74. Liu Y, Li D, Liu Z, Zhou Y, Chu D, Li X, et al. Targeted exosome-mediated delivery of opioid receptor Mu siRNA for the treatment of morphine relapse. Sci Rep. (2015) 5:17543. doi: 10.1038/srep17543
75. Lafourcade C, Ramírez JP, Luarte A, Fernández A, Wyneken U. MiRNAs in astrocyte-derived exosomes as possible mediators of neuronal plasticity. J Exp Neurosci. (2016) 10:1–9. doi: 10.4137/JEN.S39916
76. Guitart K, Loers G, Buck F, Bork U, Schachner M, Kleene R. Improvement of neuronal cell survival by astrocyte-derived exosomes under hypoxic and ischemic conditions depends on prion protein. Glia. (2016) 64:896–910. doi: 10.1002/glia.22963
77. Xin H, Li Y, Cui Y, Yang JJ, Zhang ZG, Chopp M. Systemic administration of exosomes released from mesenchymal stromal cells promote functional recovery and neurovascular plasticity after stroke in rats. J Cereb Blood Flow Metab. (2013) 33:1711–5. doi: 10.1038/jcbfm.2013.152
78. Xin H, Li Y, Liu Z, Wang X, Shang X, Cui Y. MiR-133b promotes neural plasticity and functional recovery after treatment of stroke with multipotent mesenchymal stromal cells in rats via transfer of exosome-enriched extracellular particles. Stem Cells. (2013) 31:2737–46. doi: 10.1002/stem.1409
79. Zhang Y, Chopp M, Meng Y, Katakowski M, Xin H, Mahmood A, et al. Effect of exosomes derived from multipluripotent mesenchymal stromal cells on functional recovery and neurovascular plasticity in rats after traumatic brain injury. J Neurosurg. (2015) 122:856–67. doi: 10.3171/2014.11.JNS14770
80. Betzer O, Perets N, Angel A, Motiei M, Sadan T, Yadid G, et al. In vivo neuroimaging of exosomes using gold nanoparticles. ACS Nano. (2017) 11:10883–93. doi: 10.1021/acsnano.7b04495
81. Otero-Ortega L, Laso-García F, Gómez-de Frutos MD, Rodríguez-Frutos B, Pascual-Guerra J, Fuentes B, et al. White matter repair after extracellular vesicles administration in an experimental animal model of subcortical stroke. Sci Rep. (2017) 7:44433. doi: 10.1038/srep44433
82. Xiong Y, Mahmood A, Chopp M. Emerging potential of exosomes for treatment of traumatic brain injury. Neural Regen Res. (2017) 12:19–22. doi: 10.4103/1673-5374.198966
83. Yuan D, Zhao Y, Banks WA, Bullock KM, Haney M, Batrakova E, et al. Macrophage exosomes as natural nanocarriers for protein delivery to inflamed brain. Biomaterials. (2017) 142:1–12. doi: 10.1016/j.biomaterials.2017.07.011
84. Webb RL, Kaiser EE, Scoville SL, Thompson TA, Fatima S, Pandya C, et al. Human neural stem cell extracellular vesicles improve tissue and functional recovery in the murine thromboembolic stroke model. Transl Stroke Res. (2018) 9:530–9. doi: 10.1007/s12975-017-0599-2
85. Zhang Y, Kim MS, Jia B, Yan J, Zuniga-Hertz JP, Han C, et al. Hypothalamic stem cells control ageing speed partly through exosomal miRNAs. Nature. (2017) 548:52–7. doi: 10.1038/nature23282
86. Tassew NG, Charish J, Shabanzadeh AP, Luga V, Harada H, Farhani N, et al. Exosomes mediate mobilization of autocrine Wnt10b to promote axonal regeneration in the injured CNS. Cell Rep. (2017) 20:99–111. doi: 10.1016/j.celrep.2017.06.009
87. Zhang Y, Chopp M, Liu XS, Katakowski M, Wang X, et al. Exosomes derived from mesenchymal stromal cells promote axonal growth of cortical neurons. Mol Neurobiol. (2017) 54:2659–73. doi: 10.1007/s12035-016-9851-0
88. Haqqani AS, Delaney CE, Tremblay TL, Sodja C, Sandhu JK, Stanimirovic DB. Method for isolation and molecular characterization of extracellular microvesicles released from brain endothelial cells. Fluids Barriers CNS. (2013) 10:4. doi: 10.1186/2045-8118-10-4
89. András IE, Leda A, Contreras MG, Bertrand L, Park M, Skowronska M, et al. Extracellular vesicles of the blood-brain barrier: role in the HIV-1 associated amyloid beta pathology. Mol Cell Neurosci. (2017) 79:12–22. doi: 10.1016/j.mcn.2016.12.006
90. Xin H, Wang F, Li Y, Lu QE, Cheung WL, et al. Secondary release of exosomes from astrocytes contributes to the increase in neural plasticity and improvement of functional recovery after stroke in rats treated with exosomes harvested from MicroRNA 133b-overexpressing multipotent mesenchymal stromal cells. Cell Transpl. (2017) 26:243–57. doi: 10.3727/096368916X693031
91. Balusu S, Van Wonterghem E, De Rycke R, Raemdonck K, Stremersch S, Gevaert K, et al. Identification of a novel mechanism of blood-brain communication during peripheral inflammation via choroid plexus-derived extracellular vesicles. EMBO Mol Med. (2016) 8:1162–83. doi: 10.15252/emmm.201606271
92. Esenwa CC, Elkind MS. Inflammatory risk factors, biomarkers and associated therapy in ischaemic stroke. Nat Rev Neurol. (2016) 12:594–604. doi: 10.1038/nrneurol.2016.125
93. Couch Y, Akbar N, Davis S, Fischer R, Dickens AM, Neuhaus AA, et al. Inflammatory stroke extracellular vesicles induce macrophage activation. Stroke. (2017) 48:2292–6. doi: 10.1161/STROKEAHA.117.017236
94. Kim DK, Nishida H, An SY, Shetty AK, Bartosh TJ, Prockop DJ. Chromatographically isolated CD63+CD81+ extracellular vesicles from mesenchymal stromal cells rescue cognitive impairments after TBI. Proc Natl Acad Sci USA. (2016) 113:170–5. doi: 10.1073/pnas.1522297113
95. Drommelschmidt K, Serdar M, Bendix I, Herz J, Bertling F, Prager S, et al. Mesenchymal stem cell-derived extracellular vesicles ameliorate inflammation-induced preterm brain injury. Brain Behav Immunity. (2017) 60:220–32. doi: 10.1016/j.bbi.2016.11.011
96. Chopp M, Li Y. Treatment of neural injury with marrow stromal cells. Lancet Neurol. (2002) 1:92–100. doi: 10.1016/S1474-4422(02)00040-6
97. Kumar P, Wu H, McBride JL, Jung KE, Kim MH, Davidson BL, et al. Transvascular delivery of small interfering RNA to the central nervous system. Nature. (2007) 448:39–43. doi: 10.1038/nature05901
98. Alvarez-Erviti L, Seow Y, Yin H, Betts C, Lakhal S, Wood MJ. Delivery of siRNA to the mouse brain by systemic injection of targeted exosomes. Nat Biotechnol. (2011) 29:341–5. doi: 10.1038/nbt.1807
99. Yang J, Zhang X, Chen X, Wang L, Yang G. Exosome mediated delivery of miR-124 promotes neurogenesis after ischemia. Mol Ther Nucleic Acids. (2017) 7:278–87. doi: 10.1016/j.omtn.2017.04.010
100. Marcus ME, Leonard JN. FedExosomes: engineering therapeutic biological nanoparticles that truly deliver. Pharmaceuticals. (2013) 6:659–80. doi: 10.3390/ph6050659
101. Zhuang X, Xiang X, Grizzle W, Sun D, Zhang S, Axtell RC, et al. Treatment of brain inflammatory diseases by delivering exosome encapsulated anti-inflammatory drugs from the nasal region to the brain. Mol Ther. (2011) 19:1769–79. doi: 10.1038/mt.2011.164
102. Tian T, Zhang HX, He CP, Fan S, Zhu YL, Qi C, et al. Surface functionalized exosomes as targeted drug delivery vehicles for cerebral ischemia therapy. Biomaterials. (2018) 150:137–49. doi: 10.1016/j.biomaterials.2017.10.012
103. Turtzo LC, Budde MD, Dean DD, Gold EM, Lewis BK, Janes L, et al. Failure of intravenous or intracardiac delivery of mesenchymal stromal cells to improve outcomes after focal traumatic brain injury in the female rat. PLoS ONE. (2015) 10:e0126551. doi: 10.1371/journal.pone.0126551
104. Harting MT, Jimenez F, Xue H, Fischer UM, Baumgartner J, Dash PK, et al. Intravenous mesenchymal stem cell therapy for traumatic brain injury. J Neurosurg. (2009) 110:1189–97. doi: 10.3171/2008.9.JNS08158
105. Yang S, Zhu B, Yin P, Zhao L, Wang Y, Fu Z, et al. Integration of human umbilical cord mesenchymal stem cells-derived exosomes with hydroxyapatite-embedded hyaluronic acid-alginate hydrogel for bone regeneration. ACS Biomater Sci Eng. (2020) 6:1590–602. doi: 10.1021/acsbiomaterials.9b01363
106. Zhang FX, Liu P, Ding W, Meng QB, Su DH, Zhang QC, et al. Injectable mussel-inspired highly adhesive hydrogel with exosomes for endogenous cell recruitment and cartilage defect regeneration. Biomaterials. (2021) 278:121169. doi: 10.1016/j.biomaterials.2021.121169
107. Shi Q, Qian Z, Liu D, Sun J, Wang X, Liu H, et al. GMSC-Derived exosomes combined with a chitosan/silk hydrogel sponge accelerates wound healing in a diabetic rat skin defect model. Front Physiol. (2017) 8:904. doi: 10.3389/fphys.2017.00904
108. Wang C, Wang M, Xu T, Zhang X, Lin C, Gao W, et al. Engineering bioactive self-healing antibacterial exosomes hydrogel for promoting chronic diabetic wound healing and complete skin regeneration. Theranostics. (2019) 9:65–76. doi: 10.7150/thno.29766
109. Han C, Zhou J, Liang C, Liu B, Pan X, Zhang Y, et al. Human umbilical cord mesenchymal stem cell derived exosomes encapsulated in functional peptide hydrogels promote cardiac repair. Biomaterials science. (2019) 7:2920–2933. doi: 10.1039/C9BM00101H
110. Zou Y, Li L, Li Y, Chen S, Xie X, Jin X, et al. Restoring cardiac functions after myocardial infarction-ischemia/reperfusion via an exosome anchoring conductive hydrogel. ACS Appl Mater Interfaces. (2021) 13:56892–56908. doi: 10.1021/acsami.1c16481
111. Li L, Zhang Y, Mu J, Chen J, Zhang C, Cao H, et al. Transplantation of human mesenchymal stem-cell-derived exosomes immobilized in an adhesive hydrogel for effective treatment of spinal cord injury. Nano Lett. (2020) 20:4298–305. doi: 10.1021/acs.nanolett.0c00929
112. Cao Y, Xu Y, Chen C, Xie H, Lu H, Hu J. Local delivery of USC-derived exosomes harboring ANGPTL3 enhances spinal cord functional recovery after injury by promoting angiogenesis. Stem Cell Res Ther. (2021) 12:20. doi: 10.1186/s13287-020-02078-8
113. Cheng J, Chen Z, Liu C, Zhong M, Wang S, Sun Y, et al. Bone mesenchymal stem cell-derived exosome-loaded injectable hydrogel for minimally invasive treatment of spinal cord injury. Nanomedicine. (2021) 16:1567–79. doi: 10.2217/nnm-2021-0025
114. Luo Z, Peng W, Xu Y, Xie Y, Liu Y, Lu H, et al. Exosomal OTULIN from M2 macrophages promotes the recovery of spinal cord injuries via stimulating Wnt/β-catenin pathway-mediated vascular regeneration. Acta Biomater. (2021) 136:519–32. doi: 10.1016/j.actbio.2021.09.026
115. Staufer O, Dietrich F, Rimal R, Schröter M, Fabritz S, Boehm H, et al. Bottom-up assembly of biomedical relevant fully synthetic extracellular vesicles. Sci Adv. (2021) 7:eabg6666. doi: 10.1126/sciadv.abg6666
116. Liu Y, Hsu YH, Huang AP, Hsu SH. Semi-Interpenetrating polymer network of hyaluronan and chitosan self-healing hydrogels for central nervous system repair. ACS Appl Mater Interfaces. (2020) 12:40108–20. doi: 10.1021/acsami.0c11433
Keywords: hydrogels, exosomes, traumatic brain injury, biocompatible materials, therapy
Citation: Chen Y, Lin J and Yan W (2022) A Prosperous Application of Hydrogels With Extracellular Vesicles Release for Traumatic Brain Injury. Front. Neurol. 13:908468. doi: 10.3389/fneur.2022.908468
Received: 30 March 2022; Accepted: 05 May 2022;
Published: 02 June 2022.
Edited by:
Ulises Gomez-Pinedo, Health Research Institute of Hospital Clínico San Carlos, SpainReviewed by:
Francisco Javier Sancho-Bielsa, University of Castilla-La Mancha, SpainDoddy Denise Ojeda-Hernández, Universidad Complutense de Madrid, Spain
Copyright © 2022 Chen, Lin and Yan. This is an open-access article distributed under the terms of the Creative Commons Attribution License (CC BY). The use, distribution or reproduction in other forums is permitted, provided the original author(s) and the copyright owner(s) are credited and that the original publication in this journal is cited, in accordance with accepted academic practice. No use, distribution or reproduction is permitted which does not comply with these terms.
*Correspondence: Jingquan Lin, MTIwMTg0NjkmI3gwMDA0MDt6anUuZWR1LmNu; Wei Yan, eXdpc3l3JiN4MDAwNDA7emp1LmVkdS5jbg==