- 1Department of Histology and Embryology, School of Medicine, Shaoxing University, Zhejiang, China
- 2Institute of Neuroscience, Zhejiang University School of Medicine, Hangzhou, China
The pathogenesis of spinal cord injury (SCI) is complex. At present, there is no effective treatment for SCI, with most current interventions focused on improving the symptoms. Inflammation, apoptosis, autophagy, and oxidative stress caused by secondary SCI may instigate serious consequences in the event of SCI. The mammalian target of rapamycin (mTOR), as a key signaling molecule, participates in the regulation of inflammation, apoptosis, and autophagy in several processes associated with SCI. Quercetin can reduce the loss of myelin sheath, enhance the ability of antioxidant stress, and promote axonal regeneration. Moreover, quercetin is also a significant player in regulating the mTOR signaling pathway that improves pathological alterations following neuronal injury. Herein, we review the therapeutic effects of quercetin in SCI through its modulation of the mTOR signaling pathway and elaborate on how it can be a potential interventional agent for SCI.
Introduction
Spinal cord injury (SCI) is a serious lesion of the nervous system, which may be caused by falling, shooting, and vehicle collision (1, 2). The global incidence rate of SCI is about 10.4–83 per million per year (3). SCI pathological processes are categorized into two stages: primary and secondary injuries. The primary injury often has features of bone fragments and spinal ligament tears when the spine suffers from sudden trauma that can lead to spinal fracture and vertebral dislocation (4). Initial mechanical injury can cause an imbalance of axons, blood vessels, and cell membranes at the injured site (5). Magnetic resonance imaging scans have evidenced hemorrhage, cytotoxic edema, and spinal cord swelling after the primary SCI. Moreover, the intramedullary injury is gradually expanded to the rostral and caudal sides (6). Following the primary injury, there is the instigation of the secondary injury that can lead to chemical and mechanical damages. The secondary injury causes a series of responses, such as oxidative stress, excitotoxicity, inflammation, apoptosis, autophagy, and mitochondrial dysfunction (7–11). Owing to the primary injury being an initial and irreversible process, the secondary SCI has become an important treatment time window to prevent further damage. SCI can cause neuronal atrophy and synaptic abnormalities, concomitant with inflammation, apoptosis, and autophagy. Therefore, SCI treatment should be enacted as soon as possible to control the occurrence of inflammation, reduce apoptosis, and enhance autophagy. Besides, the formation of glial scar hinders the regeneration of axons.
Quercetin is a flavonoid compound and widely exists in the roots and fruits of edible plants, such as apples and onions (12, 13). Quercetin, as a dietary supplement, is safe and has less adverse effects (14). In addition, it has features of antioxidation, anti-inflammatory, promotes autophagy, inhibits apoptosis, and improves pathological changes related to the cardiovascular, liver, and kidney (15–17). Quercetin may be a potential drug for SCI in view of the above features. Multiple studies have demonstrated that the microenvironment and cell survival after SCI can be improved by regulating a number of signaling pathways, such as the mammalian target of rapamycin (mTOR), nuclear factor-kappa B (NF-κB), mitogen-activated protein kinase (MAPK), and phosphoinositide 3-kinase (PI3K) (18–20). The mTOR signaling pathway is involved in several pathological changes, such as inflammation, autophagy, apoptosis, and oxidative stress in the wake of SCI (21, 22). Thus, regulating the mTOR signaling pathway can be an important strategy to improve SCI. The review initially expounds on the pathological changes associated with SCI and the biological roles of the mTOR signaling pathway, before exploring the relationship between SCI and the mTOR signaling pathway. Furthermore, it will endeavor to elaborate on how quercetin can regulate the mTOR signaling pathway to ameliorate SCI.
The Pathological Changes After SCI
Signaling pathways are the significant players in inflammation, apoptosis, and autophagy after SCI. The aggravation of inflammatory response is a key factor in the development of the secondary SCI (23). Local microglia activation and leukocyte infiltration augment reactive oxygen species (ROS) to induce oxidative stress. Colony-stimulating factor 1 receptor inhibitor PLX5622 can downregulate the expressions of ROS and tumor necrosis factor-α (TNF-α) by inhibiting microglial activation and reducing the infiltration of leukocytes and monocytes (24). In addition, the products of an inflammatory response, such as interleukin-1α (IL-1α), TNF-α, and complement component 1q, can induce the activation of A1 astrocytes. The formation of A1 astrocytes is closely related to the activation of the Notch pathway. When the Notch pathway of astrocytes is activated, the Notch intracellular domain (NICD) is released into the cytoplasm, and free NICD binds to phosphorylated STAT3 to form an NICD-STAT3 complex, which then enters the nucleus and promotes the transformation of astrocytes to A1 phenotype (25). Interestingly, astrocytes can form a glial scar barrier to limit the spread of inflammation (26).
The triggering receptor expressed on myeloid cell 1 (TREM1) can be expressed in astrocytes and microglia and is overexpressed in the injured spinal cord tissue. The TREM1 activates toll-like receptors (TLRs)/myeloid differentiation primary response 88 (MyD88)/NF-κB signaling pathways to upregulate the expressions of IL-6, IL-1β, TNF-α,CCL2, and CXCL1 and intensify inflammatory response (17). The TREM1 knockout decreases the activity of TLRs/IκBα/NF-κB signaling pathway, implying that this signaling pathway activated by TREM1 can be a key player in the pathological development of SCI. Further, MyD88 activates the NF-κB kinase (IKK) inhibitor to promote the separation of the NF-κB/IκB complex and translocate p65 subunit to the nucleus. Moreover, p65 entering the nucleus can activate the release of IL-6, IL-1β, and TNF-α to aggravate inflammatory response (8). After SCI, there are the activations of a series of pathways by cell surface receptors, such as TREM1, along with the formation of ROS in cells that can instigate the inflammatory response. The advanced oxidation protein products (AOPPs) upregulate NOX4 expression to produce ROS (27). ROS can activate MAPK to promote the phosphorylation of p38 and c-Jun N-terminal kinase (JNK) and then activate NF-κB to induce the expression of NACHT-LRR-PYD domains-containing protein 3 (NLRP3), eventually causing neuroinflammatory response (28). Zinc can regulate the NLRP3 inflammatory factor by activating the nuclear factor erythroid 2-related factor 2 (Nrf2)/heme oxygenase-1 (HO-1) pathways, which in turn inhibits inflammatory reaction (29). The ROS and reactive nitrogen species (RNS) produced after SCI induce the oxidative stress response, causing destruction to protein, DNA, and lipid peroxidation (4). Thymosin beta 4 inhibits the TLR4/MyD88 signaling pathway to minimize the damage of oxidative stress induced by hydrogen peroxide to neural stem cells (30). Interestingly, the impairment of the TLRs/MyD88 signaling pathway is not only involved in mitigating inflammation, but also a significant player in antioxidant stress (30, 31). Also, the phosphorylations of PI3K and protein kinase B (Akt) activate the Nrf2/Keap1 signaling pathway to exert its antioxidant role (32). Tetramethylpyrazine can activate Akt to enhance Nrf2 expression. The activation of Nrf2 combined with the antioxidant response element (ARE), which upregulates HO-1, can mitigate inflammatory response and oxidative stress (33). Kelch-like ECH-associated protein (Keap1) can bind to Nrf2 and inhibit its activation. When Keap1 is silenced or inactivated, Nrf2 is released into the cytoplasm (34). However, p62 can destroy the ubiquitination modification of Nrf2 to separate Nrf2 from Keap1 and activate Nrf2 as well as its downstream pathways (35). The released Nrf2 then enters the nucleus and induces the expressions of HO-1, NAD(P)H-dependent quinone oxidoreductase 1 (NQO1), and glutamate-cysteine ligase catalytic subunit (GCLC) (34). Moreover, the AMP-activated protein kinase (AMPK) phosphorylation can upregulate the HO-1 expression (36). Oxidative stress induces HO-1 that can decrease the formation of ROS and RNS, and the phosphorylation of JNK, p38 MAPK, and IKK to inhibit inflammation (37).
In the wake of SCI, the damaged glial cells and neurons activate the apoptotic pathway through the mitochondria and death receptors, resulting in cell death (38). Furthermore, after SCI, lysosomal destruction causes autophagy flux disruption that increases endoplasmic reticulum stress and aggravates neuronal apoptosis (39). The ratio of beclin-1 and autophagy marker light chain 3 (LC3)II/LCI is increased after SCI (40). Interestingly, the activation of autophagy can reduce neuronal apoptosis (41). PI3K/Akt controls autophagy and apoptosis. The PI3K activated phosphorylates PIP2 and converts to PIP3, which then activates Akt (42). Also, the expressions of phosphatase and tensin homolog (PTEN) are significantly increased, which inhibits the Akt/mTOR signaling pathway and promotes apoptosis after SCI (21). PTEN inhibition can increase the activity of the downstream PI3K signaling pathway (43). Akt activates the mTOR to trigger the translation of downstream proteins by phosphorylating 4EBP1 and P70S6K (44). Berberine can inhibit the activation of mTOR/P70S6K/4EBP1, increase autophagy, and reduce apoptosis (45).
Understanding the pathological changes of the secondary SCI is very important for halting and treating SCI. The related mechanisms of the inflammatory response, oxidative stress, apoptosis, and autophagy are the main instigators of the secondary SCI. Inflammation, oxidative stress, and apoptosis can affect each other, and there is a close relationship between the related proteins that activate the signaling pathways of these lesions (Figure 1).
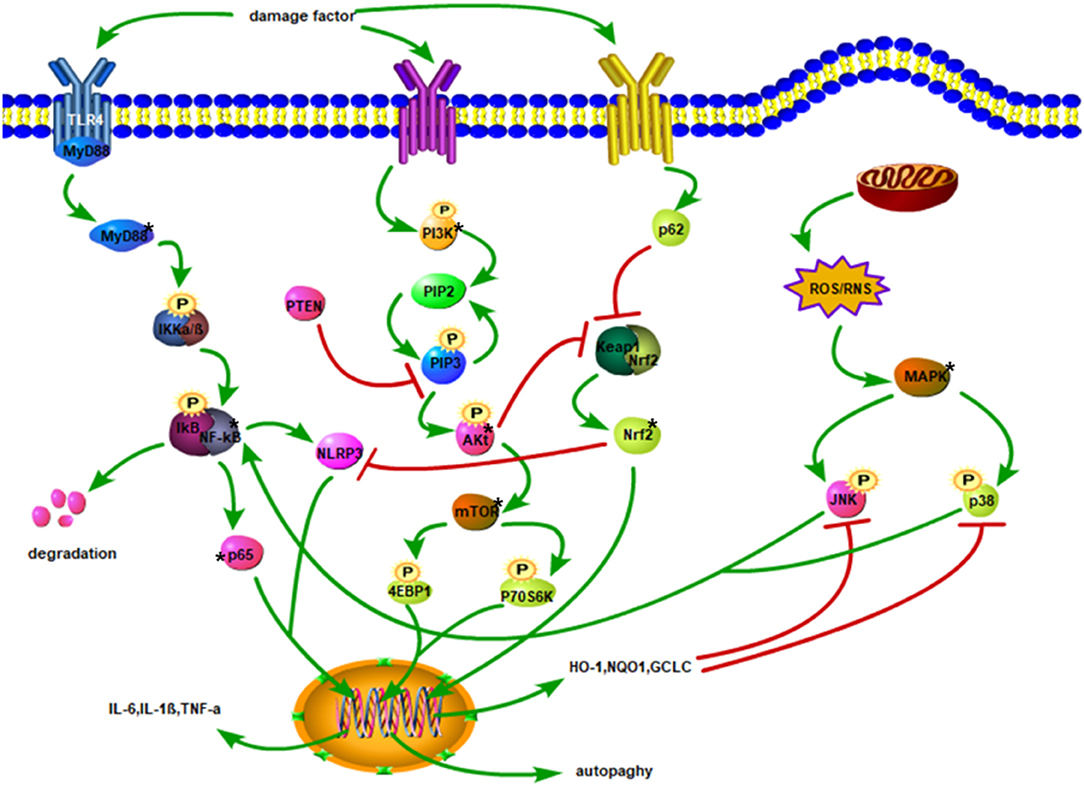
Figure 1. Injury factors activate TLR4/MyD88 and promote IKKα/β phosphorylation. The degradation of IκB facilitates the translocation of p65 of NF-κB to the nucleus, thereby increasing the expression of inflammatory factors. Phosphorylation of PI3K triggers the conversion of PIP2 to PIP3 and stimulates Akt phosphorylation. Akt activates mTOR to phosphorylate 4EBP1, and P70S6K to enhance autophagy. Activated p62 may inhibit Keap1 and Nrf2 complexes, promote the release of Nrf2 into the cytoplasm, and then translocate to the nucleus. After Nrf2 enters the nucleus, it upregulates the expressions of HO-1, NQO1, and GCLC, leading to oxidation impairment and hindering the phosphorylation of JNK and p38. Phosphorylated JNK and p38 activate NF-κB. Mitochondrial damage produces ROS and RNS, which activates the phosphorylation of JNK and p38 downstream of MAPK. The NF-κB stimulates NLRP3, and Nrf2 inhibits NLRP3 activation.
The mTOR Signaling Pathway
The Biological Structure of mTOR
In 1991, Heitman et al. discover the mTOR (46). Throughout evolution, the mTOR protein has been highly conserved. mTOR is a eukaryotic serine/threonine protein kinase with a 289-kDa serine, belonging to the PI3K-related kinase family (47, 48). Surprisingly, it does not have the activity of esterase kinase, but Ser/Thr protein kinase activity forms the catalytic subunits of the two different protein complexes called the mTOR complex 1 (mTORC1) and mTORC2 (49). The compositions of mTORC1 are catalytic subunits and three related proteins, Raptor, PRAS40, and mLST8/GbL. The mTORC2 includes mTOR and mLST8/GbL, along with Rictor, mSin1, and protor proteins (50).
The Biological Function of mTOR
The mTOR signaling is paramount to the occurrence and development of several diseases, most of which are caused by mTOR overactivation. In LPS-induced depressive-like behavior, the suppression of the GluN2A-extracellular signal-regulated kinase (ERK)-mTOR signaling pathway inhibits neuroinflammation (51). Also, a significant amount of ATP produced by glycolysisactivates the mTOR signal in microglia, upregulates BDNF and TNF-α levels, and aggravates neuroinflammation (52). During the perioperative period in diabetic rats, excessive activation of the mTOR may aggravate the progression of autophagy, neuroapoptosis, and cognitive dysfunction (53). However, during development, the mTOR signal is necessary for driving the growth of myelin sheath and can promote oligodendrocytes to form myelin sheath (54). Besides, the mTOR signaling pathway plays an important role in the occurrence and repair of SCI. L-leucine promotes axonal regeneration in the SCI region by activating the mTOR signal and increases the p-mTOR expression, while also enhanced axonal crossing to the chondroitin sulfate polysaccharide region (55). Further, mTOR activation promotes neurotrophic factor-3 expression to induce the regeneration of myelinated axons (56). The abnormal activation or inhibition of the PI3K/Akt/mTOR can cause cancer, immunity, aging, metabolism, and other diseases (57–60). Considering the aforementioned studies, an in-depth investigation of the mTOR pathway can open avenues in understanding its upstream and downstream mechanisms, as well as its regulation that can be translated to improve neurological conditions.
The mTOR Regulates Inflammatory Response
The mTOR and its downstream (i.e., the NF-κB) factors participate in the inflammatory response. Rapamycin can decrease the occurrence of the mTOR-mediated neuroinflammation by inhibiting the overactivation of the mTOR pathway to suppress the activations of microglia and astrocytes and protect the blood–brain barrier (61). The mTOR-mediated inflammatory reaction includes two aspects: (1) The mTOR may directly induce the release of inflammatory factors and regulate the metabolism of related immune cells to instigate inflammatory response (62). Further, the mTOR signaling pathway promotes the expression of inflammatory factors, such as IL-17A, IL-17F, IL-22, and TNF-α, to aggravate inflammatory response (63, 64). (2) The activation of the related signaling pathways associated with the mTOR intensifies the progression of inflammation. For instance, amyloid-β in Alzheimer's disease can promote the phosphorylations of mTOR and NF-κB, which then triggers the occurrence of inflammation. However, metformin, the activator of AMPK, reduces amyloid-β volume and improves neuroinflammation through AMPK/mTOR/S6K/Bace1 signaling pathways (65). Meanwhile, this study also shows that the occurrence of neuroinflammation is closely related to the NF-κB activity downstream of mTOR. Besides, activating the mTOR/NF-κB signaling pathway can enhance neuroinflammation and systemic response inflammation (64). Notably, the inhibition of phosphorylated NF-κB is concomitant to the repression of the mTOR signaling pathway in the nucleus, leading to the translocation of the NF-κB to the nucleus being restricted (66). In addition, the miR-124-3p in microglial exosomes can target the PDE4B gene and inhibit the mTOR signaling pathway to alleviate neuroinflammation after brain injury (67). Therefore, the activations of mTOR and downstream NF-κB can aggravate the inflammatory response, but through cascade reactions, inhibition of mTOR activation targeted can improve inflammation.
mTOR Participates in Apoptosis
The mTOR signal is a key to neuronal survival, and the curtailment of the mTORC1 activity can increase neuronal apoptosis (68). As a pleiotropic cytokine, G-CSF can play a neuroprotective role in cerebral ischemia. This is due to its ability to inhibit Bax expression and CC3 activation by stimulating the mTOR/P70S6K signaling pathway and reduce the loss of pro-apoptotic neurons (69). Moreover, the Akt/mTOR is a key regulatory pathway of apoptosis, and its activation can upregulate Bcl-2 expression, reduce the ratio of Bcl-2/Bax, and minimize the apoptosis of nerve cells after repeated cerebral ischemia–reperfusion (70). The translocation of the mitochondrial p53 in the hippocampal CA1 region induces neuronal apoptosis after transient global cerebral ischemia (71). Noteworthy is that the mTOR phosphorylation negatively regulates the p53 to inhibit its phosphorylation and curtail apoptosis, which in turn can minimize the infarct volume after cerebral ischemia (72).
mTOR Is Involved in Autophagy
Autophagy exists in both pathological and physiological processes. Abnormal inhibition or activation of the mTOR affects autophagy activity. For instance, the inhibition of the mTOR signal can cause the limited expression of the downstream protein, P70S6K, which in turn may increase neuronal autophagy (73). Also, the inhibition of the mTOR phosphorylation promotes LC3-II expression and increases the ratio of LC3-II/LC3-I, which is concomitant with autophagy increased (74). Although neurons have moderate autophagy in the process of maintaining homeostasis, long-term overactivated autophagy can cause serious damage to neurons. The activation of the AMPK can inhibit the mTOR phosphorylation and enhance autophagy. However, alpha-lipoic acid can block the AMPK/mTOR signaling pathway to inhibit autophagy development (75). As an energy sensor of cells, the AMPK is affected by the energy changes induced by oxidative stress, which then activates autophagy. Therefore, there is also a close relationship between oxidative stress and autophagy. Besides, the AMPK can impair mTOR phosphorylation, reduce s757-ULK1 phosphorylation, promote s317-ULK1 phosphorylation, and induce autophagy in cerebral ischemia–reperfusion injury (76). Interestingly, the AMPK can negatively regulate the mTOR to promote autophagy (77). The PI3K/Akt/mTOR/P70S6K signaling pathway is also involved in the regulation of neuronal autophagy. In particular, astragalus polysaccharides inhibit the activation of the PI3K/Akt/mTOR signaling pathway, upregulate the expression of a negative regulatory protein, PTEN, and lead to the augmentation of autophagy (78). mTOR-mediated autophagy can also inhibit the activation of inflammatory factors and participate in the regulation of inflammatory response. TNF-α can activate the Akt/mTOR signaling pathway, reduce autophagy flux, and promote the transformation of microglia to M1 phenotype (79). However, the downregulation of mTOR may enhance autophagy, inhibit the activation of microglia, reduce inflammation, and promote neuronal survival (80). Although some studies have shown that the inhibition of the mTOR signal can activate excessive autophagy and cause neuronal damage, an activated autophagy through the mTOR signal pathway can also help neurons to counter the effects of inflammation and apoptosis.
The mTOR Signaling Pathway and SCI
The regulation of the mTOR signaling pathway is important in nervous system diseases. Activating the Akt/mTOR and ERK/mTOR signaling pathways can promote the growth of neurites (81). Furthermore, G-CSF can reduce neuroinflammation and inhibit neuronal apoptosis by stimulating the mTOR/P70S6K pathway (69). Interestingly, blocking the 5-HT6 receptor-mediated mTOR signal activation may improve neuropathic pain and mitigate complications of cognitive impairment as evidenced in rats (82). Therefore, controlling the mTOR signaling pathway can change the processes related to inflammation, apoptosis, and autophagy in nervous system diseases.
The Relationship Between the mTOR Signaling and Inflammation in SCI
The mTOR and its downstream pathways can modulate the development of neuroinflammation after SCI (83). High-mobility group protein B1 (HMGB1) binds to the TLR4 overexpressed on the surface of activated microglia after SCI, which inhibits the AMPK phosphorylation and activates its downstream pathway, facilitating the expression of TNF-α and IL-1 to aggravate inflammatory response (84–86) (Figure 2). However, after SCI, activation of the AMPK reduces the mTOR phosphorylation level and increases the TFEB nuclear translocation level to improve the degree of injury, and this effect can be reversed by an AMPK inhibitor (87). Other studies have shown that the activation of the AMPK can phosphorylate TSC1/2 and Raptor and inhibit the mTORC1 activity (86, 88). The PI3K/Akt and MAPK signaling pathways are involved in the mTOR-mediated inflammatory response. TNF-α binds to its receptors that can activate the downstream PI3K/Akt pathway (89) (Figure 2). Activated microglia increase the NF-κB expression and IL-6 level by stimulating the PI3K/Akt/mTOR signaling pathway (90). Meanwhile, after SCI, MAPK phosphorylation promotes the phosphorylations of p38, JNK, and ERK and activates NF-κB and inflammatory response (27, 91). Therefore, the inhibition of mTOR signal can block the phosphorylation of IKKα/β and nuclear translocation of the NF-κB p65 to reduce the release of proinflammatory factors (88). Transcription factor EB (TFEB), as a downstream protein of mTOR, plays an important role in regulating inflammation. Notably, doxycycline-treated mice show significantly increased phosphorylated levels of mTOR, IKKα/β, and NF-κB, although TFEB expression is decreased (92). This suggests that mTOR may promote the nuclear translocation of NF-κB by inhibiting the TFEB (92) and thus corroborating the previous study of Wu et al. Also, mTOR can activate the STAT3 (93) and aggravate neuroinflammation and neuropathic pain after SCI (94, 95). More importantly, the STAT3 can upregulate TNF-α and IL-1 expressions through the IKK/NF-κB pathway (96).
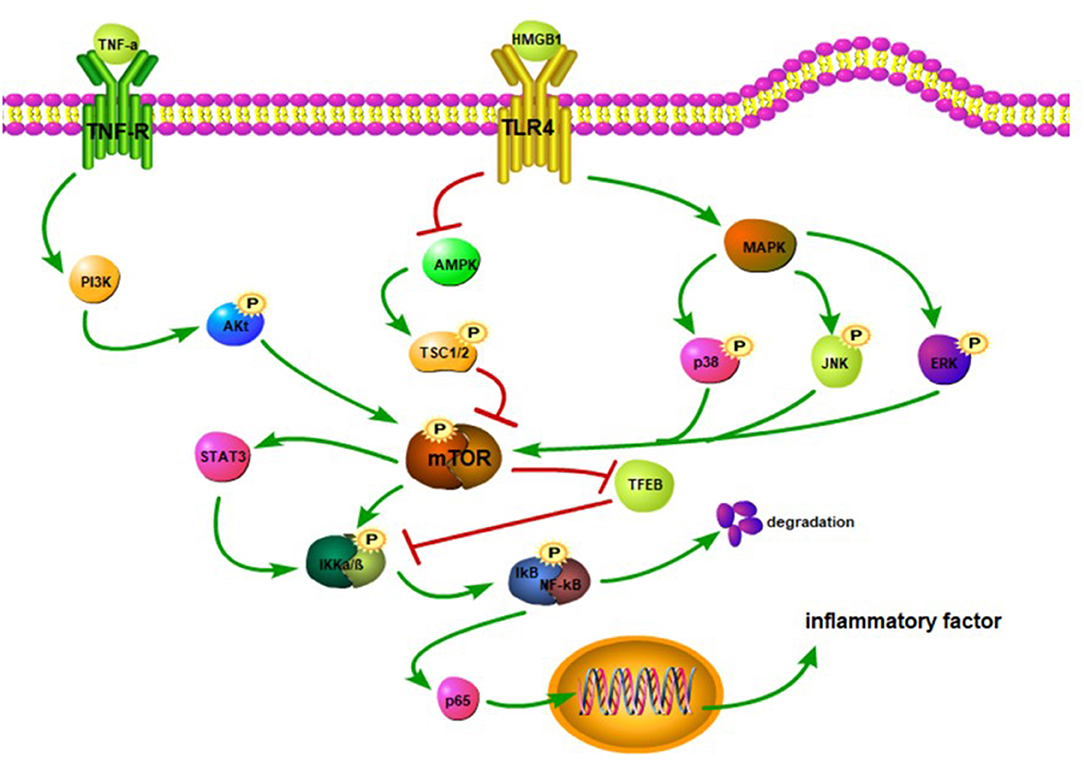
Figure 2. TNF-α binds to its receptor, activates PI3K, and promotes Akt phosphorylation. The Akt activates the mTOR. The HMGB1 binds to the TLR4 to inhibit the activation of the AMPK and trigger MAPK. The inhibition of the AMPK reduces the phosphorylation of TSC1/2, thereby hindering the activation of the mTOR. The MAPK promotes the phosphorylation of JNK, p38, and ERK to activate the mTOR. The mTOR stimulates STAT3 and facilitates the phosphorylation of IKKα/β. The mTOR can suppress the TFEB activation, which in turn may inhibit the IKKα/β phosphorylation. The activation of the NF-κB promotes the release of p65. When p65 is released into the cytoplasm, it translocates to the nucleus and upregulatesthe expression of inflammatory factors.
The Relationship Between the mTOR Signaling and Apoptosis in SCI
The regulation of cell apoptosis is often accompanied by phosphorylation of the mTOR and changes in the expression of upstream and downstream factors (Figure 3). SCI affects upstream factors to instigate a downregulation in the expressions, p-Akt and p-mTOR (97). In Sprague-Dawley rats with traumatic SCI, LC3, and Beclin1 expressions are decreased along with the accumulation of autophagy substrate (p62) and ubiquitinated proteins, subsequently leading to impaired autophagy activity. Moreover, following the activation of the Rheb/mTOR signaling pathway, the expression of the pro-apoptotic protein, Bax, is downregulated, whereas the expression of the anti-apoptotic protein, Bcl-2, is upregulated, and the number of TUNEL-positive neurons is decreased (98). Similarly, autophagy is increased, with apoptosis being decreased (31). By regulating the Akt/mTOR/HIF-α signaling pathway and reducing the phosphorylation of Akt/mTOR, the apoptotic-related proteins are reduced, which in turn may inhibit the apoptosis of motor neurons.
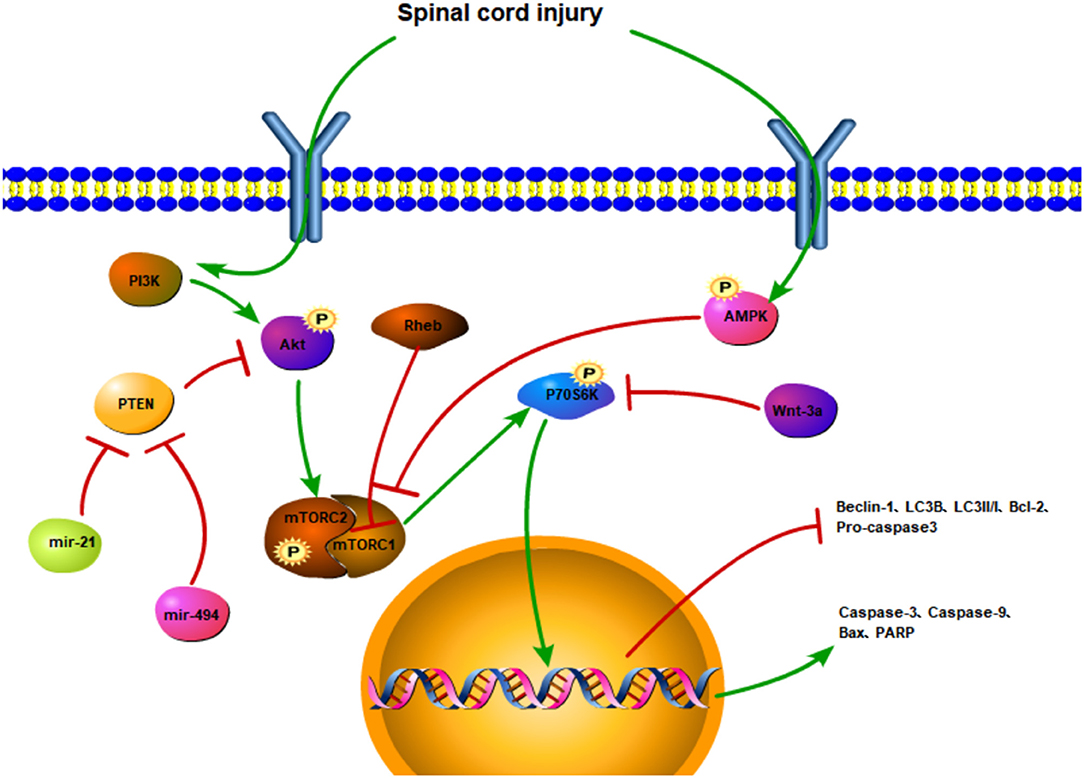
Figure 3. The regulatory mechanism of the mTOR signaling pathway and apoptotic autophagy after spinal cord injury. SCI activates the PI3K/Akt signaling pathway and promotes the phosphorylation of the mTOR. The PTEN inhibits the phosphorylation of the Akt and triggers the mTOR. However, miR-21 and mir-494 may hinder PTEN activation. SCI promotes AMPK phosphorylation and inhibits mTOR activation. Rheb can also inhibit mTORC1. The activation of mTOR upregulates the expressions of P70S6K, caspase-3, caspase-9, Bax, and PARP and downregulates the expressions of Beclin-1, LC3II/I, LC3B, Bcl-2, procaspase 3. Wnt-3a inhibits the phosphorylation of P70S6K.
The PTEN is a negative regulator of the Akt/mTOR pathway. By upregulating the miR-21, the overexpression of miR-21 decreases the expression of PTEN and activates the Akt/mTOR pathway. But the inhibition of the miR-21 can diminish the protective effect of neuronal apoptosis and inflammation induced by SCI. Therefore, the activation of the mir-21/PTEN/Akt/mTOR pathway may suppress neuronal apoptosis and inflammation (97). The neuroprotective effect of the miR-92a-3p is positively correlated with the activation of the PTEN/Akt/mTOR pathway after SCI (99). Also, the miR-494 can downregulate PTEN expression, improve the phosphorylations of Akt and mTOR, inhibit apoptosis, and enhance functional recovery after SCI. In addition, cleaved caspase-3, cleaved PARP, and Bax are downregulated, whereas procaspase-3, PARP, and Bcl-2 are upregulated (21).
The Relationship Between the mTOR Signaling and Autophagy in SCI
Autophagy plays a key role in neuroprotection and nerve regeneration in SCI (Figure 3). It promotes the recruitment of autophagosomes through mTOR-independent pathways, enhances the autophagic flux of neurons, inhibits cell apoptosis through the intrinsic mitochondrial-dependent pathway, curtails the expansion of the injured cavity, mitigates neuron loss, and ultimately improves SCI (100). MicroRNA-421-3P (miR-421-3p) targets mTOR, and the miR-421-3p can bind to the 3'untranslated region (3'UTR) of the mTOR to reduce the activity of the luciferase-mtor3'UTR construct and improve neuronal autophagy (101). Increasing autophagy and reducing apoptosis can induce axonal protection after SCI (102). More so, activating the AMPK mTOR signal axis and inhibiting the mTOR stimulation may enhance the autophagy level of microglia and promote the fusion of autophagosomes and lysosomes (103). Microglia clear myelin fragments protect residual myelin and promote axonal regeneration after SCI.
Both LC3 and p62 expressions are higher after SCI. However, this is not a representation of the complete activation of autophagy flux. The lysosomal membrane protein (i.e., LAMP-2), which is closely related to autophagy degradation, is significantly decreased after SCI, implying that the downstream degradation pathway of autophagy flux is damaged after SCI. Nonetheless, autophagy flux activation through the AMPK/mTOR pathway affects the microglial polarization (104). By activating the AMPK to suppress mTOR activation and inhibit the mTOR/P70S6K signaling pathway after SCI, the levels of p-AMPK, Beclin-1, and LC3B are increased, and the expressions of p-P70S6K and p-mTOR are decreased (105), together with the significant curtailment of caspase-3, caspase-9, and Bax protein levels (106). Moreover, the upregulated expression of autophagy markers and the downregulation of apoptotic marker expressions lead to neuronal protection (107). The inhibition of mTOR signal can upregulate the TFEB expression, increase autophagy flux, activate autophagy, and exert neuroprotectiveness (108).
Wnts affect the proliferation, composition, and survival of nerve cells. The Wnt-3a can inhibit the phosphorylation level of the P70S6K, which augments Beclin-1 and LC3II/I in the neurons of spinal cord. Chondroitin sulfate proteoglycan (CSPG) increases the expression level of neurons after SCI, which has a restrictive effect on the growth and regeneration of axons, causing the inhibition of axonal regeneration of neurons. Following Wnt-3a treatment, the expression level of CSPG in the neurons of spinal cord is significantly reduced, which facilitates axonal regeneration and improves functional recovery through autophagy activation (109).
Quercetin Regulates the mTOR Signaling Pathway to Improve SCI
Quercetin plays a neuroprotective role in neurodegeneration. In a Parkinson's disease animal model induced by rotenone, quercetin improves the degeneration of dopaminergic neurons through its anti-inflammatory and antioxidant abilities (110). Furthermore, quercetin minimizes the neurotoxicity caused by β-amyloid by activating the Sirtuin1/Nrf2/HO-1 pathway to decrease apoptosis (111). It also regulates neuronal survival and proliferation through the PI3K/Akt and ERK1/2 signaling pathways (112) and mitigates apoptosis by upregulating the expression of neurotrophic factors (NGF, BDNF) (113) to activate downstream pathways (114). Interestingly, quercetin can promote the expression of LC3II by inhibiting mTOR phosphorylation to enhance autophagy activity and promote the recovery of motor function (115). It can also activate the mTOR pathway to improve cognitive impairment after sleep deprivation (116). However, in severely injured neurons, low-dose quercetin may promote oxidation (112). Although a study shows quercetin to promote oxidative stress, multiple studies have demonstrated that it can improve nerve injury, especially through the mTOR signaling pathway.
The Biological Roles of Quercetin
The therapeutic effect of quercetin has been widely studied in different fields, such as cancer, cardiovascular, and kidney (15, 16, 117). Quercetin promotes the nuclear translocation of transcription factor EB, activates lysosomes, causes the accumulation of iron, and leads to the death of cancer cells (115). Quercetin can activate the FXR1/TGR5 signaling pathway to improve lipid metabolism in non-alcoholic fatty liver disease (118). In addition, quercetin may inhibit the NADPH oxidase by upregulating the HO-1 expression and minimizing ROS formation (119) to protect the kidney and pancreas (120). Besides, quercetin is significantly involved in the treatment of neurodegenerative diseases (Table 1). Quercetin increases the level of Nrf2 mRNA expression and promotes AMPKα and SIRT1 expressions to reduce the generation of ROS (133). Also, quercetin increases ERK and Akt phosphorylation by inhibiting protein threonine and serine–threonine phosphatase activity, thereby reducing neuronal apoptosis after cerebral ischemia–reperfusion injury (134). Mitochondrial fragmentation can lead to neuronal degeneration. However, quercetin can upregulate the expression of H2AX and mitochondrial transcription factor A to minimize the damages in the mitochondrial structure and activity, while also reducing axonal and neuronal detriments (135). Also, quercetin can reduce the damage of myelin loss to white matter after cerebral hypoperfusion by promoting the secretion of IL-10 by M2-like microglia (136). Quercetin decreases the apoptosis of cortical neurons, improves degenerative lesions of the central nervous system, and plays a neuroprotective role in Alzheimer's disease (137). Quercetin also improves the gray and white matter injuries after SCI, which may indicate its potentiality for SCI treatment. Furthermore, quercetin improves neuroinflammation by reducing the activation of STAT1 and NF-κB pathways to impair the polarization of macrophages or microglia to M1 (138) and mitigates the activation of astrocytes and microglia (135). After SCI, the death of oligodendrocytes causes demyelination, resulting in permanent neurological dysfunction. However, the use of quercetin mitigates the necrosis of oligodendrocytes by improving the living environment of oligodendrocytes, while also reducing myelin loss and axonal damage (138). The hemorrhage causes hemoglobin decomposition to form Fe2+, which then induces apoptosis and oxidative stress in the area of SCI. Nonetheless, quercetin can chelate Fe2+ and remove free iron to improve acute injury after SCI (139). Quercetin improves the electrophysiological function after acute SCI, increases the 5-HT-positive nerve fibers, and promotes axonal regeneration (140). Besides, in the early stage after acute SCI, the reactive astrocytes activated by quercetin stabilize the central nervous system and play a positive role in SCI. Also, isoquercetin, an analog of quercetin, inhibits the mitochondrial and endoplasmic reticulum stress in cells, reduces neuronal apoptosis, and exerts neuroprotection in acute SCI (141).
Quercetin Can Alleviate Inflammatory Response by Regulating the mTOR Signaling in SCI
Quercetin is involved in the regulation of mTOR-mediated inflammatory response and therefore can be a potential drug that improves pathological changes after SCI. For instance, TNF-α upregulates the expression of NF-κB and AP-1 to aggravate inflammation. However, quercetin treatment reverses this effect (142). Quercetin can inhibit the activation of MAPK, reduce the phosphorylation of JNK, p38, and ERK to inhibit the mTOR phosphorylation, leading to the downregulated expressions of IL-6, IL-1β, and TNF-α that alleviates inflammatory reaction (143, 144). Moreover, quercetin downregulates the TNF-α expression, inhibits the activation of hypothalamic–pituitary–adrenocortical axis, reduces neuroinflammation after stress, and improves cognitive impairment in mice (145). Following SCI, quercetin minimizes the expression of NLRP3 inflammation-related protein (146) and upregulates the BDNF expression to block the JAK2/STAT3 signaling pathway (140) (Figure 4). Noteworthy is that the mTOR/STAT3 pathway is involved in LPS-induced inflammatory response (147). Hence, quercetin can reduce inflammatory response by regulating the phosphorylation of mTOR and STAT3.
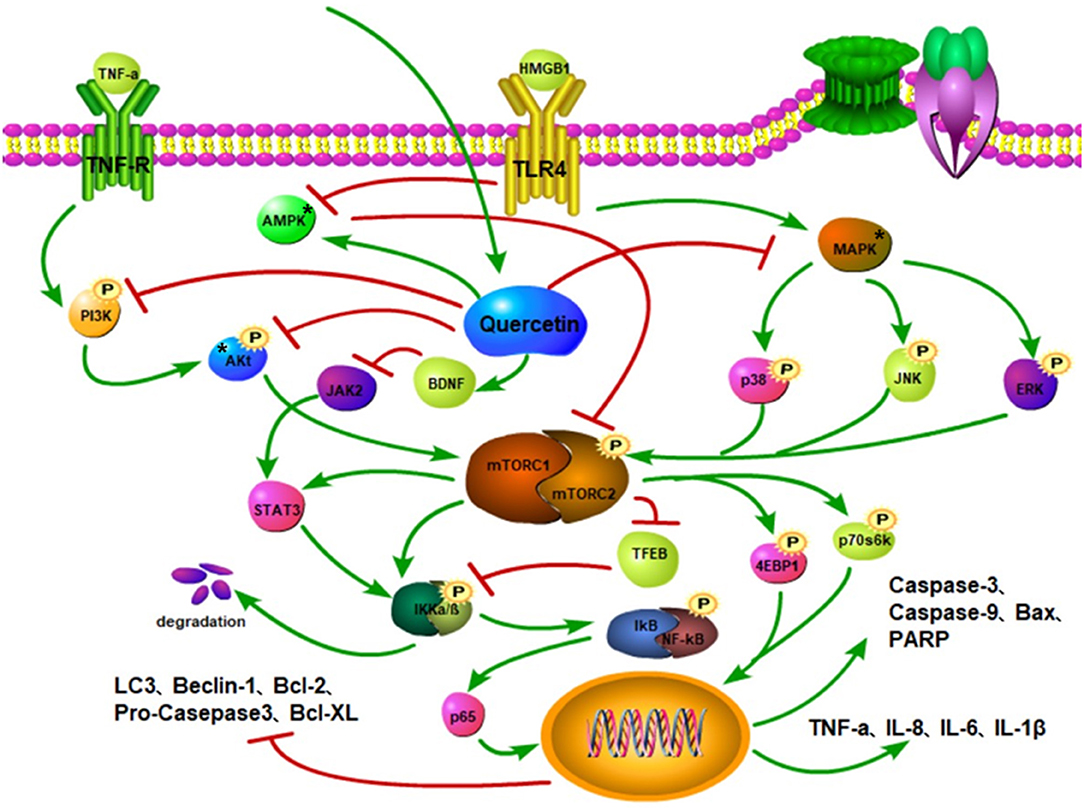
Figure 4. Quercetin inhibits the phosphorylation of PI3K and Akt and mitigates the activation of mTOR. Quercetin activates the AMPK to inhibit mTOR activation and stimulate autophagy. Quercetin suppresses the MAPK activation to curtail the phosphorylations of p38, JNK, and ERK, thereby reducing mTOR activation, while also increasing the ratio of LC3-II and autophagosomes. It also restricts BDNF to inhibit JAK activity and STAT3 activation. The inhibition of the mTOR activity reduces the activations of STAT3 and IKKα/β to promote the TFEB expression. The phosphorylations of IκB and NF-κB complexes attenuate the nuclear translocation of p65 and downregulate the expressions of pro-inflammatory factors, such as TNF-α, IL-8, and IL-6. Quercetin downregulates Bax, caspase-9, and caspase-3 protein expressions, inhibits the phosphorylations of Akt, mTOR, P70S6K, and 4E-BP1, and increases Beclin1, Bcl-2, and LC3-II protein levels through the PI3K/Akt/mTOR signaling pathway.
Quercetin can also inhibit the Akt/mTOR signaling pathway to decrease the mTOR phosphorylation (148), along with curtailing the phosphorylation of PI3K and Akt by inhibiting the formation of the TLR4/MyD88/PI3K complex, subsequently reducing the nuclear translocation of NF-κB (149) (Figure 4). Far more, the anti-inflammatory effect of quercetin is demonstrated to be time- and dose-dependent (149). Interestingly, quercetin can activate the AMPK pathway to inhibit the mTOR and reduce nuclear translocation of the NF-κB to improve inflammation (144, 150, 151).
Quercetin Can Inhibit Apoptosis and Upregulate Autophagy Reaction by Regulating the mTOR Signaling in SCI
Apoptosis and autophagy are the key players in SCI repair. Quercetin promotes the motor and electrophysiological functional recovery, astrocytic activation, and axonal regeneration after acute SCI (140). Moreover, it minimizes the formation of cavities at the injured site and pathological damage to nerve tissue, improves post-traumatic spinal cord edema (152), increases neuronal survival, promotes nerve regeneration (153), preserves the injured spinal cord tissue (154), and facilitates motor function recovery (139). The level of S-100β in serum is associated with the degree of nerve injury, neuroprotection, and regeneration. Quercetin significantly increases the level of S-100β that improves nerve injury (155). Following SCI, the employment of quercetin alters apoptotic and autophagy protein factors through the impairment of the mTOR signaling pathway (Figure 4). Also, quercetin considerably inhibits the augmentation of phosphorylated p38MAPK (p-p38MAPK) (156) and decreases the levels of TNF-α, IL-1β, Bcl-2, Bax, caspase-3, and caspase-9 expressions (157), leading to reduce spinal cord inflammation and impair apoptosis. The inhibition of the p38MAPK activity results in the mTOR diminution and causes an increase in autophagic activity (158). Both P70S6K and 4E-BP1, which are located in the downstream of mTOR, are dephosphorylated and increased caspase-3, caspase-9, and cleaved PARP (159). In nerve tissues, quercetin hinders the kinase activity of the mTOR in a dose-dependent manner, which improves the function of related signal axes and enhances cell degradation and self-renewal capabilities (160). Besides, quercetin mitigates the phosphorylated level of Akt, mTOR, and P70S6K and promotes motor function recovery, axonal regeneration, and energy metabolism following SCI. Also, it can inhibit apoptosis by activating the PI3K/Akt signaling pathway to increase Bcl-2 protein expression and decrease Bax protein expression (161). In addition, quercetin activates autophagy through the AMPK signaling pathway (162).
Conclusion and Future Prospect
The mTOR is involved in the pathological processes associated with SCI, and the regulation of the mTOR can significantly improve inflammation, apoptosis, and autophagy after SCI. Quercetin can play a neuroprotective role in nervous system diseases. Subsequent to SCI, quercetin can minimize inflammation and apoptosis and enhance autophagy by modulating the mTOR signaling pathway. This can reduce the secretion of inflammatory factors, inhibit microglial activation, increase autophagy processes, and lessen cell damage. Based on the aforementioned therapeutic effects, quercetin may be an interventional agent that regulates the mTOR signaling pathway in the treatment of SCI. Nevertheless, the effect and specific mechanism of quercetin in different stages of SCI still need further investigation using different animal models before it is employed in the clinical setting.
Author Contributions
XL designed the study. XW, YF, BB, YuZ, YoZ, TJ, and XL prepared the first draft of the manuscript and revised the manuscript. All authors approved the final version of the manuscript.
Funding
This study was supported by the Natural Science Foundation of Zhejiang Province (No. LY19H170001).
Conflict of Interest
The authors declare that the research was conducted in the absence of any commercial or financial relationships that could be construed as a potential conflict of interest.
Publisher's Note
All claims expressed in this article are solely those of the authors and do not necessarily represent those of their affiliated organizations, or those of the publisher, the editors and the reviewers. Any product that may be evaluated in this article, or claim that may be made by its manufacturer, is not guaranteed or endorsed by the publisher.
Abbreviations
SCI, spinal cord injury; mTOR, the mammalian target of rapamycin; NF-κB, nuclear factor-kappa B; MAPK, mitogen-activated protein kinase; PI3K, Phosphoinositide 3-kinase; ROS, reactive oxygen species; TNF-α, tumor necrosis factor alpha; IL-1α, interleukin-1α; NICD, the Notch intracellular domain; TREM1, triggering receptor expressed on myeloid cells 1; TLRs, toll-like receptors; MyD88, myeloid differentiation primary response 88; IKK, inhibitor of nuclear factor kappa-B kinase; IκB, inhibitor of NF-κB; AOPPs, advanced oxidation protein products; JNK, c-Jun N-terminal kinase; NLRP3, NACHT-LRR-PYD domains-containing protein 3; Nrf2, nuclear factor erythroid 2-related factor 2; HO-1, Heme oxygenase-1; ARE, antioxidant response element; Keap1, the Kelch-like ECH-associated protein; NQO1, NAD(P)H-dependent quinone oxidoreductase 1; GCLC, glutamate-cysteine ligase catalytic subunit; Akt, protein kinase B; AMPK, AMP-activated protein kinase; PTEN, phosphatase and tensin homolog; mTORC1, mTOR complex 1; RA, rheumatoid arthritis; TFEB, Transcription factor EB; LC3, light chain 3; CSPG, Chondroitin sulfate proteoglycan; ERK, extracellular signal-regulated kinase.
References
1. Stahel PF, VanderHeiden T, Finn MA. Management strategies for acute spinal cord injury: current options and future perspectives. Curr Opin Crit Care. (2012) 18:651–60. doi: 10.1097/MCC.0b013e32835a0e54
2. Witiw CD, Fehlings MG. Acute spinal cord injury. J Spinal Disord Tech. (2015) 28:202–10. doi: 10.1097/BSD.0000000000000287
3. Karsy M, Hawryluk G. Modern medical management of spinal cord injury. Curr Neurol Neurosci Rep. (2019) 19:65. doi: 10.1007/s11910-019-0984-1
4. Anjum A, Yazid MD, Fauzi Daud M, Idris J, Ng AMH, Selvi Naicker A, et al. Spinal cord injury: pathophysiology, multimolecular interactions, and underlying recovery mechanisms. Int J Mol Sci. (2020) 21:7533. doi: 10.3390/ijms21207533
5. Venkatesh K, Ghosh SK, Mullick M, Manivasagam G, Sen D. Spinal cord injury: pathophysiology, treatment strategies, associated challenges, future implications. Cell Tissue Res. (2019) 377:125–51. doi: 10.1007/s00441-019-03039-1
6. Freund P, Seif M, Weiskopf N, Friston K, Fehlings MG, Thompson AJ, et al. Traumatic spinal cord injury: from clinical assessment to neuroimaging biomarkers. Lancet Neurol. (2019) 18:1123–35. doi: 10.1016/S1474-4422(19)30138-3
7. Li Z, Wu F, Xu D, Zhi Z, Xu G. (2019). Inhibition of TREM1 reduces inflammation and oxidative stress after spinal cord injury (SCI) associated with HO-1 expressions. Biomed Pharmacother. 109:2014–21. doi: 10.1016/j.biopha.2018.08.159
8. Ma Z, Lu Y, Yang F, Li S, He X, Gao Y, et al. Rosmarinic acid exerts a neuroprotective effect on spinal cord injury by suppressing oxidative stress and inflammation via modulating the Nrf2/HO-1 and TLR4/NF-κB pathways. Toxicol Appl Pharmacol. (2020) 397:115014. doi: 10.1016/j.taap.2020.115014
9. Rong Y, Liu W, Wang J, Fan J, Luo Y, Li L, et al. stem cell-derived small extracellular vesicles attenuate apoptosis and neuroinflammation after traumatic spinal cord injury by activating autophagy. Cell Death Dis. (2019) 10:340. doi: 10.1038/s41419-019-1571-8
10. Wang Q, Cai H, Hu Z, Wu Y, Guo X, Li J, et al. Loureirin B promotes axon regeneration by inhibiting endoplasmic reticulum stress: induced mitochondrial dysfunction and regulating the Akt/GSK-3β pathway after spinal cord injury. J Neurotrauma. (2019) 36:1949–64. doi: 10.1089/neu.2018.5966
11. Yu CG, Bondada V, Joshi A, Reneer DV, Telling GC, Saatman KE, et al. Against excitotoxic hippocampal injury and traumatic spinal cord injury. J Neurotrauma. (2020) 37:2268–76. doi: 10.1089/neu.2020.7122
12. Khan H, Ullah H, Aschner M, Cheang WS, Akkol EK. Neuroprotective effects of quercetin in Alzheimer's disease. Biomolecules. (2019) 10:59. doi: 10.3390/biom10010059
13. Costa LG, Garrick JM, Roqu, è PJ, Pellacani C. Mechanisms of neuroprotection by quercetin: counteracting oxidative stress and more. Oxid Med Cell Longev. (2016) 2016:2986796. doi: 10.1155/2016/298679613
14. Andres S, Pevny S, Ziegenhagen R, Bakhiya N, Schäfer B, Hirsch-Ernst KI, et al. Safety Aspects of the Use of Quercetin as a Dietary,Supplement. Mol Nutr Food Res. (2018) 62. doi: 10.1002/mnfr.201700447
15. Mirsafaei L, Reiner Ž, Shafabakhsh R, Asemi Z. Molecular biological functions of quercetin as a natural solution for cardiovascular disease prevention and treatment. Plant Foods Hum Nutr. (2020) 75:307–15. doi: 10.1007/s11130-020-00832-0
16. Zeng H, Guo X, Zhou F, Xiao L, Liu J, Jiang C, et al. Quercetin alleviates ethanol-induced liver steatosis associated with improvement of lipophagy. Food Chem Toxicol. (2019) 125:21–8. doi: 10.1016/j.fct.2018.12.028
17. Yang H, Song Y, Liang YN, Li R. Quercetin treatment improves renal function and protects the kidney in a rat model of adenine-induced chronic kidney disease. Med Sci Monit. (2018) 24:4760–6. doi: 10.12659/MSM.909259
18. Xu S, Wang J, Zhong J, Shao M, Jiang J, Song J, et al. CD73 alleviates GSDMD-mediated microglia pyroptosis in spinal cord injury through PI3K/AKT/Foxo1 signaling. Clin Transl Med. (2021) 11:e269. doi: 10.1002/ctm2.269
19. Vargova I, Machova Urdzikova L, Karova K, Smejkalova B, Sursal T, Cimermanova V, et al. Involvement of mTOR pathways in recovery from spinal cord injury by modulation of autophagy and immune response. Biomedicines. (2021) 9:593. doi: 10.3390/biomedicines9060593
20. Su Y, Zong S, Wei C, Song F, Feng H, Qin A, et al. Salidroside promotes rat spinal cord injury recovery by inhibiting inflammatory cytokine expression and NF-κB and MAPK signaling pathways. J Cell Physiol. (2019) 234:14259–69. doi: 10.1002/jcp.28124
21. Zhu H, Xie R, Liu X, Shou J, Gu W, Gu S, et al. MicroRNA-494 improves functional recovery and inhibits apoptosis by modulating PTEN/AKT/mTOR pathway in rats after spinal cord injury. Biomed Pharmacother. (2017) 92:879–87. doi: 10.1016/j.biopha.2017.05.143
22. Kanno H, Ozawa H, Sekiguchi A, Yamaya S, Tateda S, Yahata K, et al. The role of mTOR signaling pathway in spinal cord injury. Cell Cycle. (2012) 11:3175–9. doi: 10.4161/cc.21262
23. Zeng H, Liu N, Yang YY, Xing HY, Liu XX, Li F, et al. Lentivirus-mediated downregulation of α-synuclein reduces neuroinflammation and promotes functional recovery in rats with spinal cord injury. J Neuroinflammation. (2019) 16:283. doi: 10.1186/s12974-019-1658-2
24. Li Y, Ritzel RM, Khan N, Cao T, He J, Lei Z, et al. microglial depletion after spinal cord injury reduces chronic inflammation and neurodegeneration in the brain and improves neurological recovery in male mice. Theranostics. (2020) 10:11376–403. doi: 10.7150/thno.49199
25. Qian D, Li L, Rong Y, Liu W, Wang Q, Zhou Z, et al. signal pathway suppresses the activation of neurotoxic A1 astrocytes after spinal cord injury. Cell Cycle. (2019) 18:3010–29. doi: 10.1080/15384101.2019.1667189
26. Kwiecien JM, Dabrowski W, Dabrowska-Bouta B, Sulkowski G, Oakden W, Kwiecien-Delaney CJ, et al. Prolonged inflammation leads to ongoing damage after spinal cord injury. PLoS ONE. (2020) 15:e0226584. doi: 10.1371/journal.pone.0226584
27. Liu Z, Yao X, Jiang W, Li W, Zhu S, Liao C, et al. oxidation protein products induce microglia-mediated neuroinflammation via MAPKs-NF-κB signaling pathway and pyroptosis after secondary spinal cord injury. J Neuroinflammation. (2020) 17:90. doi: 10.1186/s12974-020-01751-2
28. An Y, Zhang H, Wang C, Jiao F, Xu H, Wang X, et al. of ROS/MAPKs/NF-κB/NLRP3 and inhibition of efferocytosis in osteoclast-mediated diabetic osteoporosis. FASEB J. (2019) 33:12515–27. doi: 10.1096/fj.201802805RR
29. Li D, Tian H, Li X, Mao L, Zhao X, Lin J, et al. Zinc promotes functional recovery after spinal cord injury by activating Nrf2/HO-1 defense pathway and inhibiting inflammation of NLRP3 in nerve cells. Life Sci. (2020) 245:117351. doi: 10.1016/j.lfs.2020.117351
30. Li H, Wang Y, Hu X, Ma B, Zhang H. Thymosin beta 4 attenuates oxidative stress-induced injury of spinal cord-derived neural stem/progenitor cells through the TLR4/MyD88 pathway. Gene. (2019) 707:136–42. doi: 10.1016/j.gene.2019.04.083
31. Li Y, Guo Y, Fan Y, Tian H, Li K, Mei X. Melatonin enhances autophagy x and reduces apoptosis to promote locomotor recovery in spinal cord injury via the PI3K/AKT/mTOR signaling pathway. Neurochem Res. (2019) 44:2007–19. doi: 10.1007/s11064-019-02838-w
32. Li ST, Dai Q, Zhang SX, Liu YJ, Yu QQ, Tan F, et al. Attenuates LPS-induced inflammation in mouse macrophage RAW264.7 cells by inhibiting the JNK/NF-κB signaling pathway and activating the PI3K/Akt/Nrf2 pathway. Acta Pharmacol Sin. (2018) 39:1294–304. doi: 10.1038/aps.2017.143
33. Wang C, Wang P, Zeng W, Li W. Tetramethylpyrazine improves the recovery of spinal cord injury via Akt/Nrf2/HO-1 pathway. Bioorg Med Chem Lett. (2016) 26:1287–91. doi: 10.1016/j.bmcl.2016.01.015
34. Wang X, Ye L, Zhang K, Gao L, Xiao J, Zhang Y. Upregulation of microRNA−200a in bone marrow mesenchymal stem cells enhances the repair of spinal cord injury in rats by reducing oxidative stress and regulating Keap1/Nrf2 pathway. Artif Organs. (2020) 44:744–52. doi: 10.1111/aor.13656
35. Tan X, Yang Y, Xu J, Zhang P, Deng R, Mao Y, et al. via Modulation of the p62/Keap1/Nrf2 Pathway in Intracerebral Hemorrhage. Front Pharmacol. (2020) 10:1551. doi: 10.3389/fphar.2019.01551
36. Chen D, Wu YX, Qiu YB, Wan BB, Liu G, Chen JL, et al. Hyperoside suppresses hypoxia-induced A549 survival and proliferation through ferrous accumulation via AMPK/HO-1 axis. Phytomedicine. (2020) 67:153138. doi: 10.1016/j.phymed.2019.153138
37. Lee JA, Kim DJ, Hwang O. KMS99220 Exerts Anti-Inflammatory Effects, Activates the Nrf2 Signaling and Interferes with IKK, JNK and p38 MAPK via HO-1. Mol Cells. (2019) 42:702–10. doi: 10.14348/molcells.2019.0129
38. Abbaszadeh F, Fakhri S, Khan H. Targeting apoptosis and autophagy following spinal cord injury: Therapeutic approaches to polyphenols and candidate phytochemicals. Pharmacol Res. (2020) 160:105069. doi: 10.1016/j.phrs.2020.105069
39. Zhou K, Zheng Z, Li Y, Han W, Zhang J, Mao Y, et al. TFE3, a potential therapeutic target for Spinal Cord Injury via augmenting autophagy flux and alleviating ER stress. Theranostics. (2020) 10:9280–302. doi: 10.7150/thno.46566
40. Wang ZY, Lin JH, Muharram A, Liu WG. Beclin-1-mediated autophagy protects spinal cord neurons against mechanical injury-induced apoptosis. Apoptosis. (2014) 19:933–45. doi: 10.1007/s10495-014-0976-1
41. Cai Y, Yang E, Yao X, Zhang X, Wang Q, Wang Y, et al. FUNDC1-dependent mitophagy induced by tPA protects neurons against cerebral ischemia-reperfusion injury. Redox Biol. (2021) 38:101792. doi: 10.1016/j.redox.2020.101792
42. Alzahrani AS. PI3K/Akt/mTOR inhibitors in cancer: At the bench and bedside. Semin Cancer Biol. (2019) 59:125–32. doi: 10.1016/j.semcancer.2019.07.009
43. Hu M, Zhu S, Xiong S, Xue X, Zhou X. MicroRNAs and the PTEN/PI3K/Akt pathway in gastric cancer (Review). Oncol Rep. (2019) 41:1439–54. doi: 10.3892/or.2019.6962
44. Li K, Liu J, Song L, Lv W, Tian X, Li Z, Shi S. (2020). Effect of electroacupuncture treatment at dazhui (GV14) and mingmen (GV4) modulates the PI3K/AKT/mTOR signaling pathway in rats after spinal cord injury. Neural Plast. (2020) 2020:5474608. doi: 10.1155/2020/5474608
45. Li C, Guan XM, Wang RY, Xie YS, Zhou H, Ni WJ, et al. (2020). Berberine mitigates high glucose-induced podocyte apoptosis by modulating autophagy via the mTOR/P70S6K/4EBP1 pathway. Life Sci. (2020) 243:117277. doi: 10.1016/j.lfs.2020.117277
46. Heitman J, Movva NR, Hall MN. Targets for cell cycle arrest by the immunosuppressant rapamycin in yeast. Science. (1991) 253:905–9. doi: 10.1126/science.1715094
47. Laplante M, Sabatini DM. mTOR signaling at a glance. J Cell Sci. (2009) 122:3589–94. doi: 10.1242/jcs.051011
48. Chen Y, Zhou X. Research progress of mTOR inhibitors. Eur J Med Chem. (2020) 208:112820. doi: 10.1016/j.ejmech.2020.112820
49. Saxton RA, Sabatini DM. mTOR signaling in growth, metabolism, and disease. Cell. (2017) 168:960–76. doi: 10.1016/j.cell.2017.02.004
50. Peterson TR, Laplante M, Thoreen CC, Sancak Y, Kang SA, Kuehl WM, et al. an mTOR inhibitor frequently overexpressed in multiple myeloma cells and required for their survival. Cell. (2009) 137:873–86. doi: 10.1016/j.cell.2009.03.046
51. Francija E, Lukic I, Petrovic Z, Brkic Z, Mitic M, Radulovic J, et al. GluN2A-ERK-mTOR pathway confers a vulnerability to LPS-induced depressive-like behaviour. Behav Brain Res. (2022) 417:113625. doi: 10.1016/j.bbr.2021.113625
52. Hu Y, Mai W, Chen L, Cao K, Zhang B, Zhang Z, et al. mTOR-mediated metabolic reprogramming shapes distinct microglia functions in response to lipopolysaccharide and ATP. Glia. (2020) 68:1031–1045. doi: 10.1002/glia.23760
53. Chen X, Gao F, Lin C, Chen A, Deng J, Chen P, et al. mTOR-mediated autophagy in the hippocampus is involved in perioperative neurocognitive disorders in diabetic rats. CNS Neurosci Ther. (2021). doi: 10.1111./cns.13762
54. Fedder-Semmes KN, Appel B. The Akt-mTOR pathway drives myelin sheath growth by regulating cap-dependent translation. J Neurosci. (2021) 41:8532–44. doi: 10.1523/JNEUROSCI.0783-21.2021
55. Ma C, Teng L, Lin G, Guo B, Zhuo R, Qian X, et al. L-leucine promotes axonal outgrowth and regeneration via mTOR activation. FASEB J. (2021) 35:e21526. doi: 10.1096/fj.202001798RR
56. Liu Y, Kelamangalath L, Kim H, Han SB, Tang X, Zhai J, et al. NT-3 promotes proprioceptive axon regeneration when combined with activation of the mTor intrinsic growth pathway but not with reduction of myelin extrinsic inhibitors. Exp Neurol. (2016) 283(Pt A):73–84. doi: 10.1016/j.expneurol.2016.05.021
57. Tang ZL, Zhang K, Lv SC, Xu GW, Zhang JF, Jia HY. LncRNA MEG3 suppresses PI3K/AKT/mTOR signalling pathway to enhance autophagy and inhibit inflammation in TNF-α-treated keratinocytes and psoriatic mice. Cytokine. (2021) 148:155657. doi: 10.1016/j.cyto.2021.155657
58. Zhang X, Liu Z, Chen S, Li H, Dong L, Fu X. A new discovery: Total Bupleurum saponin extracts can inhibit the proliferation and induce apoptosis of colon cancer cells by regulating the PI3K/Akt/mTOR pathway. J Ethnopharmacol. (2022) 283:114742. doi: 10.1016/j.jep.2021.114742
59. Yuan T, Wang J, Shi C, Wang Y, Xia B, Xu W, et al. Downregulation of FAPP2 gene induces cell autophagy and inhibits PI3K/AKT/mTOR pathway in, T-,cell acute lymphoblastic leukemia. Hematol Oncol. (2021). doi: 10.1002./hon.2948
60. Wan M, Yin K, Yuan J, Ma S, Xu Q, Gao D, et al. YQFM alleviated cardiac hypertrophy by apoptosis inhibition and autophagy regulation via PI3K/AKT/mTOR pathway. J Ethnopharmacol. (2021) 2021:114835. doi: 10.1016/j.jep.2021.114835
61. Hodges SL, Lugo JN. Therapeutic role of targeting mTOR signaling and neuroinflammation in epilepsy. Epilepsy Res. (2020) 161:106282. doi: 10.1016/j.eplepsyres.2020.106282
62. Gu Y, Zhou H, Yang YH, Wang W, Qian B, Cheng F, et al. E. miR-99a regulates CD4+T cell differentiation and attenuates experimental autoimmune encephalomyelitis by mTOR-mediated glycolysis. Mol Ther Nucleic Acids. (2021) 26:1173–85. doi: 10.1016/j.omtn.2021.07.010
63. Chen S, van Tok MN, Knaup VL, Kraal L, Pots D, Bartels L, et al. mTOR blockade by rapamycin in spondyloarthritis: impact on inflammation and new bone formation in vitro and in vivo. Front Immunol. (2020) 10:2344. doi: 10.3389/fimmu.2019.02344
64. Sahan-Firat S, Temiz-Resitoglu M, Guden DS, Kucukkavruk SP, Tunctan B, Sari AN, et al. Protection by mTOR Inhibition on Zymosan-Induced Systemic Inflammatory Response and Oxidative/Nitrosative Stress: Contribution of mTOR/MEK1/ERK1/2/IKKβ/IκB-α/NF-κB Signalling Pathway. Inflammation. (2018) 41:276–98. doi: 10.1007/s10753-017-0686-2
65. Ou Z, Kong X, Sun X, He X, Zhang L, Gong Z, et al. (2018). Metformin treatment prevents amyloid plaque deposition and memory impairment in APP/PS1 mice. Brain Behav Immun. 69:351–63. doi: 10.1016/j.bbi.2017.12.009
66. Xu T, Liu J, Li XR, Yu Y, Luo X, Zheng X, et al. mTOR/NF-κB Pathway Mediates Neuroinflammation and Synaptic Plasticity in Diabetic Encephalopathy. Mol Neurobiol. (2021) 58:3848–62. doi: 10.1007/s12035-021-02390-1
67. Huang S, Ge X, Yu J, Han Z, Yin Z, Li Y, et al. miR-124-3p in microglial exosomes following traumatic brain injury inhibits neuronal inflammation and contributes to neurite outgrowth via their transfer into neurons. FASEB. (2018) 32:512–528. doi: 10.1096/fj.201700673R
68. Xu C, Yu L, Hou J, Jackson RJ, Wang H, Huang C, et al. PDK1 in the forebrain causes neuron loss and increased apoptosis during cortical development. Front Cell Neurosci. (2017) 11:330. doi: 10.3389/fncel.2017.00330
69. Dumbuya JS, Chen L, Shu SY, Ma L, Luo W, Wu L, et al. B. G-CSF attenuates neuroinflammation and neuronal apoptosis via the mTOR/p70SK6 signaling pathway in neonatal Hypoxia-Ischemia rat model. Brain Res. (2020) 1739:146817. doi: 10.1016/j.brainres.2020.146817
70. Xu J, Huai Y, Meng N, Dong Y, Liu Z, Qi Q, et al. P. L-3-n-butylphthalide activates Akt/mTOR signaling. inhibits neuronal apoptosis and autophagy and improves cognitive impairment in mice with repeated cerebral ischemia-reperfusion injury. Neurochem Res. (2017) 42:2968–81. doi: 10.1007/s11064-017-2328-3
71. Sun D, Wang W, Wang X, Wang Y, Xu X, Ping F, et al. bFGF plays a neuroprotective role by suppressing excessive autophagy and apoptosis after transient global cerebral ischemia in rats. Cell Death Dis. (2018) 9:172. doi: 10.1038/s41419-017-0229-7
72. Kilic U, Caglayan AB, Beker MC, Gunal MY, Caglayan B, Yalcin E, et al. Particular phosphorylation of PI3K/Akt on Thr308 via PDK-1 and PTEN mediates melatonin's neuroprotective activity after focal cerebral ischemia in mice. Redox Biol. (2017) 12:657–65. doi: 10.1016/j.redox.2017.04.006
73. Li X, Wang MH, Qin C, Fan WH, Tian DS, Liu JL. suppresses neuronal autophagy through the mTOR/p70S6K pathway and alleviates ischemic brain damage in mice. PLoS ONE. (2017) 12:e0188748. doi: 10.1371/journal.pone.0188748
74. Kuo CA, Wu SY, Lee CH, Lai YR, Lu CH, Chen PC, et al. Toona sinensis modulates autophagy and cytokines in lipopolysaccharide-induced RAW 264.7 macrophages. Biomed Pharmacother. (2020) 129:110386. doi: 10.1016/j.biopha.2020.110386
75. Zhou L, Cheng Y. Alpha-lipoic acid alleviated 6-OHDA-induced cell damage by inhibiting AMPK/mTOR mediated autophagy. Neuropharmacology. (2019) 155:98–103. doi: 10.1016/j.neuropharm.2019.04.009
76. Wang JF, Mei ZG, Fu Y, Yang SB, Zhang SZ, Huang WF, et al. Puerarin protects rat brain against ischemia/reperfusion injury by suppressing autophagy via the AMPK-mTOR-ULK1 signaling pathway. Neural Regen Res. (2018) 13:989–98. doi: 10.4103/1673-5374.233441
77. Ren ZL, Wang CD, Wang T, Ding H, Zhou M, Yang N, et al. Ganoderma lucidum extract ameliorates MPTP-induced parkinsonism and protects dopaminergic neurons from oxidative stress via regulating mitochondrial function, autophagy, and apoptosis. Acta Pharmacol Sin. (2019) 40:441–50. doi: 10.1038/s41401-018-0077-8
78. Tan Y, Yin L, Sun Z, Shao S, Chen W, Man X, et al. Astragalus polysaccharide exerts anti-Parkinson via activating the PI3K/AKT/mTOR pathway to increase cellular autophagy level in vitro. Int J Biol Macromol. (2020) 153:349–56. doi: 10.1016/j.ijbiomac.2020.02.282
79. Jin MM, Wang F, Qi D, Liu WW, Gu C, Mao CJ, et al. A critical role of autophagy in regulating microglia polarization in neurodegeneration. Front Aging Neurosci. (2018) 10:378. doi: 10.3389/fnagi.2018.00378
80. Yin P, Wang X, Wang S, Wei Y, Feng J, Zhu M. Maresin 1 improves cognitive decline and ameliorates inflammation in a mouse model of Alzheimer's disease. Front Cell Neurosci. (2019) 13:466. doi: 10.3389/fncel.2019.00466
81. Wen W, Wang Y, Li H, Xu H, Xu M, Frank JA, et al. Neurotrophic factor (MANF) regulates neurite outgrowth through the activation of Akt/mTOR and Erk/mTOR signaling pathways. Front Mol Neurosci. (2020) 13:560020. doi: 10.3389/fnmol.2020.560020
82. Martin PY, Doly S, Hamieh AM, Chapuy E, Canale V, Drop M, et al. mTOR activation by constitutively active serotonin6 receptors as new paradigm in neuropathic pain and its treatment. Prog Neurobiol. (2020) 193:101846. doi: 10.1016/j.pneurobio.2020.101846
83. Chen H, Xu G, Wu Y, Wang X, Wang F, Zhang Y. HBO-PC Promotes Locomotor by Reducing Apoptosis and Inflammation in SCI Rats: The Role of the mTOR Signaling Pathway. Cell Mol Neurobiol. (2021) 41:1537–47. doi: 10.1007/s10571-020-00921-3
84. Wan G, An Y, Tao J, Wang Y, Zhou Q, Yang R, et al. Micro RNA-129-5p alleviates spinal cord injury in mice via suppressing the apoptosis and inflammatory response through, HMGB1/TLR4/NF-κB. pathway. Biosci Rep. (2020) 40:BSR20193315. doi: 10.1042/BSR20193315
85. Chen J, Wang Z, Zheng Z, Chen Y, Khor S, Shi K, et al. Microglia/macrophage-derived FGF10 activate neuronal FGFR2/PI3K/Akt signaling and inhibit microglia/macrophages TLR4/NF-κB-dependent neuroinflammation to improve functional recovery after spinal cord injury. Cell Death Dis. (2017) 8:e3090. doi: 10.1038/cddis.2017.490
86. Zhang Z, Amorosa LF, Petrova A, Coyle S, Macor M, Nair M, et al. TLR4 counteracts BVRA signaling in human leukocytes via differential regulation of AMPK, mTORC1 and mTORC2. Sci Rep. (2019) 9:7020. doi: 10.1038/s41598-019-43347-8
87. Wu C, Chen H, Zhuang R, Zhang H, Wang Y, Hu X, et al. acid inhibits pyroptosis in spinal cord injury by augmenting autophagy via the AMPK-mTOR-TFEB signaling pathway. Int J Biol Sci. (2021) 17:1138–52. doi: 10.7150/ijbs.57825
88. Han CJ, Zheng JY, Sun L, Yang HC, Cao ZQ, Zhang XH, et al. The oncometabolite 2-hydroxyglutarate inhibits microglial activation via the AMPK/mTOR/NF-κB pathway. Acta Pharmacol Sin. (2019) 40:1292–302. doi: 10.1038/s41401-019-0225-9
89. Kong F, Lee BH, Wei K. 5-Hydroxymethylfurfural Mitigates Lipopolysaccharide-Stimulated Inflammation via Suppression of MAPK. NF-κB and mTOR Activation in RAW 264.7 Cells. Molecules. (2019) 24:275. doi: 10.3390/molecules24020275
90. Huang XY, Hu QP, Shi HY, Zheng YY, Hu RR, Guo Q. Everolimus inhibits PI3K/Akt/mTOR and NF-kB/IL-6 signaling and protects seizure-induced brain injury in rats. J Chem Neuroanat. (2021) 114:101960. doi: 10.1016/j.jchemneu.2021.101960
91. Zhou M, Xu W, Wang J, Yan J, Shi Y, Zhang C, et al. Boosting mTOR-dependent autophagy via upstream TLR4-MyD88-MAPK signalling and downstream NF-κB pathway quenches intestinal inflammation and oxidative stress injury. EBioMedicine. (2018) 35:345–60. doi: 10.1016/j.ebiom.2018.08.035
92. Wang X, Wang Q, Li W, Zhang Q, Jiang Y, Guo D, et al. TFEB-NF-κB inflammatory signaling axis: a novel therapeutic pathway of Dihydrotanshinone I in doxorubicin-induced cardiotoxicity. J Exp Clin Cancer Res. (2020) 39:93. doi: 10.1186/s13046-020-01595-x
93. Meng SS, Guo FM, Zhang XW, Chang W, Peng F, Qiu HB, et al. mTOR/STAT-3 pathway mediates mesenchymal stem cell-secreted hepatocyte growth factor protective effects against lipopolysaccharide-induced vascular endothelial barrier dysfunction and apoptosis. J Cell Biochem. (2019) 120:3637–50. doi: 10.1002/jcb.27642
94. Hu Z, Deng N, Liu K, Zhou N, Sun Y, Zeng W. CNTF-STAT3-IL-6 Axis mediates neuroinflammatory cascade across schwann cell-neuron-microglia. Cell Rep. (2020) 31:107657. doi: 10.1016/j.celrep.2020.107657
95. Wu WD, Wang LH, Wei NX, Kong DH, Shao G, Zhang SR, et al. MicroRNA-15a inhibits inflammatory response and apoptosis after spinal cord injury via targeting STAT3. Eur Rev Med Pharmacol Sci. (2019) 23:9189–98. doi: 10.26355/eurrev_201911_19409
96. Heo YJ, Choi SE, Jeon JY, Han SJ, Kim DJ, Kang Y, et al. Visfatin induces inflammation and insulin resistance via the nf-κb and stat3 signaling pathways in hepatocytes. J Diabetes Res. (2019) 2019:4021623. doi: 10.1155/2019/4021623
97. Sun T, Duan L, Li J, Guo H, Xiong M. Gypenoside XVII protects against spinal cord injury in mice by regulating the microRNA-21-mediated PTEN/AKT/mTOR pathway. Int J Mol Med. (2021) 48:146. doi: 10.3892/ijmm.2021.4979
98. Chen XB, Wang ZL, Yang QY, Zhao FY, Qin XL, Tang XE, et al. Diosgenin glucoside protects against spinal cord injury by regulating autophagy and alleviating apoptosis. Int J Mol Sci. (2018) 19:2274. doi: 10.3390/ijms19082274
99. He S, Wang Z, Li Y, Dong J, Xiang D, Ren L, et al. MicroRNA-92a-3p enhances functional recovery and suppresses apoptosis after spinal cord injury via targeting phosphatase and tensin homolog. Biosci Rep. (2020) 4:BSR20192743. doi: 10.1042/BSR20192743
100. Zhou K, Chen H, Xu H, Jia X. Trehalose Augments Neuron Survival and Improves Recovery from Spinal Cord Injury via mTOR-Independent Activation of Autophagy. Oxid Med Cell Longev. (2021) 2021:8898996. doi: 10.1155/2021/8898996
101. Wang J, Rong Y, Ji C, Lv C, Jiang D, Ge X, et al. MicroRNA-421-3p-abundant small extracellular vesicles derived from M2 bone marrow-derived macrophages attenuate apoptosis and promote motor function recovery via inhibition of mTOR in spinal cord injury. J Nanobiotechnology. (2020) 18:72. doi: 10.1186/s12951-020-00630-5
102. Pan D, Zhu S, Zhang W, Wei Z, Yang F, Guo Z, et al. Autophagy induced by Schwann cell-derived exosomes promotes recovery after spinal cord injury in rats. Biotechnol Lett. (2021) doi: 10.1007./s10529-021-03198-8
103. Wu YQ, Xiong J, He ZL, Yuan Y, Wang BN, Xu JY, et al. Metformin promotes microglial cells to facilitate myelin debris clearance and accelerate nerve repairment after spinal cord injury. Acta Pharmacol Sin. (2021).
104. Wang C, Wang Q, Lou Y, Xu J, Feng Z, Chen Y, et al. Salidroside attenuates neuroinflammation and improves functional recovery after spinal cord injury through microglia polarization regulation. J Cell Mol Med. (2018) 22:1148–66. doi: 10.1111/jcmm.13368
105. Lin S, Tian H, Lin J, Xu C, Yuan Y, Gao S, et al. Zinc promotes autophagy and inhibits apoptosis through AMPK/mTOR signaling pathway after spinal cord injury. Neurosci Lett. (2020) 736:135263. doi: 10.1016/j.neulet.2020.135263
106. Zhou Z, Chen S, Zhao H, Wang C, Gao K, Guo Y, et al. Probucol inhibits neural cell apoptosis via inhibition of mTOR signaling pathway after spinal cord injury. Neuroscience. (2016) 329:193–200. doi: 10.1016/j.neuroscience.2016.05.019
107. Guo Y, Wang F, Li H, Liang H, Li Y, Gao Z, He X. (2018). Metformin protects against Spinal cord injury by regulating autophagy via the mTOR signaling pathway. Neurochem Res. (2018) 43:1111–7. doi: 10.1007/s11064-018-2525-8
108. Yuan W, He X, Morin D, Barrière G, Liu X, Zhu L, et al. The neuroprotective impact of intermittent fasting on the acutely injured spinal cord. J Neurotrauma. (2021) 38:373–84. doi: 10.1089/neu.2020.7166
109. Gao K, Niu J, Dang X. Wnt-3a improves functional recovery through autophagy activation via inhibiting the mTOR signaling pathway after spinal cord injury. Neurosci Lett. (2020) 737:135305. doi: 10.1016/j.neulet.2020.135305
110. Sharma S, Raj K, Singh S. Neuroprotective effect of quercetin in combination with piperine against rotenone- and iron supplement-induced parkinson's disease in experimental rats. Neurotox Res. (2020) 37:198–209. doi: 10.1007/s12640-019-00120-z
111. Yu X, Li Y, Mu X. Effect of Quercetin on PC12 Alzheimer's Disease Cell Model Induced by Aβ25-35 and Its Mechanism Based on Sirtuin1/Nrf2/HO-1 Pathway. Biomed Res Int. (2020) 2020, 8210578. doi: 10.1155/2020/8210578
112. Zubčić K, Radovanović V, Vlainić J, Hof PR, Oršolić N, Šimić G, et al. PI3K/Akt and ERK1/2 signalling are involved in quercetin-mediated neuroprotection against copper-induced injury. Oxid Med Cell Longev. (2020) 2020:9834742. doi: 10.1155/2020/9834742
113. Li H, Chen FJ, Yang WL, Qiao HZ, Zhang SJ. Quercetin improves cognitive disorder in aging mice by inhibiting NLRP3 inflammasome activation. Food Funct. (2021) 12:717–725. doi: 10.1039/d0fo01900c
114. Yao RQ, Qi DS, Yu HL, Liu J, Yang LH, Wu XX. Quercetin attenuates cell apoptosis in focal cerebral ischemia rat brain via activation of BDNF-TrkB-PI3K/Akt signaling pathway. Neurochem Res. (2012) 37:2777–86. doi: 10.1007/s11064-012-0871-5
115. Wang ZX, Ma J, Li XY, Wu Y, Shi H, Chen Y, et al. p53-independent cancer cell death through lysosome activation by the transcription factor EB and Reactive Oxygen Species-dependent ferroptosis. Br J Pharmacol. (2021) 178:1133–48. doi: 10.1111/bph.15350
116. Zhao W, Wang J, Bi W, Ferruzzi M, Yemul S, Freire D, et al. Application of brain-targeting polyphenol compounds in sleep deprivation-induced cognitive dysfunction. Neurochem Int. (2015) 89:191–7. doi: 10.1016/j.neuint.2015.07.023
117. Reyes-Farias M, Carrasco-Pozo C. The anti-cancer effect of quercetin: molecular implications in cancer metabolism. Int J Mol Sci. (2019) 20:3177. doi: 10.3390/ijms20133177
118. Yang H, Yang T, Heng C, Zhou Y, Jiang Z, Qian X, et al. Quercetin improves nonalcoholic fatty liver by ameliorating inflammation, oxidative stress, and lipid metabolism in db/db mice. Phytother Res. (2019) 33:3140–52. doi: 10.1002/ptr.6486
119. Luo M, Tian R, Lu N. Quercetin Inhibited Endothelial Dysfunction and Atherosclerosis in Apolipoprotein E-Deficient Mice: Critical Roles for NADPH Oxidase and Heme Oxygenase-1. J Agric Food Chem. (2020) 68:10875–83. doi: 10.1021/acs.jafc.0c03907
120. El-Far AH, Lebda MA, Noreldin AE, Atta MS, Elewa YHA, Elfeky M, et al. Quercetin Attenuates Pancreatic and Renal D-Galactose-Induced Aging-Related Oxidative Alterations in Rats. Int J Mol Sci. (2020) 21:4348. doi: 10.3390/ijms21124348
121. Molaei A, Hatami H, Dehghan G, Sadeghian R, Khajehnasiri N. Synergistic effects of quercetin and regular exercise on the recovery of spatial memory and reduction of parameters of oxidative stress in animal model of Alzheimer's disease. EXCLI J. (2020) 19:596–612. doi: 10.17179/excli2019-2082
122. Paula PC, Angelica Maria SG, Luis CH, Gloria Patricia CG. Preventive Effect of Quercetin in a Triple Transgenic Alzheimer's Disease Mice Model. Molecules. (2019) 24:2287. doi: 10.3390/molecules24122287
123. Rifaai RA, Mokhemer SA, Saber EA, El-Aleem SAA, El-Tahawy NFG. Neuroprotective effect of quercetin nanoparticles: A possible prophylactic and therapeutic role in alzheimer's disease. J Chem Neuroanat. (2020) 107:101795. doi: 10.1016/j.jchemneu.2020.101795
124. Ay M, Luo J, Langley M, Jin H, Anantharam V, Kanthasamy A, et al. Molecular mechanisms underlying protective effects of quercetin against mitochondrial dysfunction and progressive dopaminergic neurodegeneration in cell culture and MitoPark transgenic mouse models of Parkinson's Disease. J Neurochem. (2017) 141:766–82. doi: 10.1111/jnc.14033
125. Wang WW, Han R, He HJ, Chen L, Gu Y, Xie C, et al. Administration of quercetin improves mitochondria quality control and protects the neurons in 6-OHDA-lesioned Parkinson's disease models. Aging (Albany NY). (2021) 13:11738–51. doi: 10.18632/aging.202868
126. El-Horany HE, El-Latif RN, ElBatsh MM, Emam MN. Ameliorative effect of quercetin on neurochemical and behavioral deficits in rotenone rat model of Parkinson's disease: modulating autophagy (Quercetin on Experimental Parkinson's Disease). J Biochem Mol Toxicol. (2016) 30:360–9. doi: 10.1002/jbt.21821
127. Sharma DR, Wani WY, Sunkaria A, Kandimalla RJ, Sharma RK, Verma D, et al. Quercetin attenuates neuronal death against aluminum-induced neurodegeneration in the rat hippocampus. Neuroscience. (2016) 324:163–76. doi: 10.1016/j.neuroscience.2016.02.055
128. Wu M, Liu F, Guo Q. Quercetin attenuates hypoxia-ischemia induced brain injury in neonatal rats by inhibiting TLR4/NF-κB signaling pathway. Int Immunopharmacol. (2019) 74:105704. doi: 10.1016/j.intimp.2019.105704
129. Li X, Wang H, Wen G, Li L, Gao Y, Zhuang Z, et al. By quercetin via mitochondrial function adaptation in traumatic brain injury: PGC-1α pathway as a potential mechanism. J Cell Mol Med. (2018) 22:883–91. doi: 10.1111/jcmm.13313
130. Lin MC, Liu CC, Liao CS, Ro JH. neuroprotective effect of quercetin during cerebral ischemic injury involves regulation of essential elements, transition metals, Cu/Zn ratio, antioxidant activity. Molecules. (2021) 26:6128. doi: 10.3390/molecules26206128
131. Park DJ, Kang JB, Shah FA, Jin YB, Koh PO. Quercetin attenuates decrease of thioredoxin expression following focal cerebral ischemia and glutamate-induced neuronal cell damage. Neuroscience. (2020) 428:38–49. doi: 10.1016/j.neuroscience.2019.11.043
132. Chakraborty J, Singh R, Dutta D, Naskar A, Rajamma U, Mohanakumar KP. Quercetin improves behavioral deficiencies, restores astrocytes and microglia, and reduces serotonin metabolism in 3-nitropropionic acid-induced rat model of Huntington's Disease. CNS Neurosci Ther. (2014) 20:10–9. doi: 10.1111/cns.12189
133. Yammine A, Zarrouk A, Nury T, Vejux A, Latruffe N, Vervandier-Fasseur D, et al. Prevention by dietary polyphenols (resveratrol, quercetin, apigenin) against 7-ketocholesterol-induced oxiapoptophagy in neuronal N2a cells: potential interest for the treatment of neurodegenerative and age-related diseases. Cells. (2020) 9:2346. doi: 10.3390/cells9112346
134. Wang YY, Chang CY, Lin SY, Wang JD, Wu CC, Chen WY, et al. Quercetin protects against cerebral ischemia/reperfusion and oxygen glucose deprivation/reoxygenation neurotoxicity. J Nutr Biochem. (2020) 83:108436. doi: 10.1016/j.jnutbio.2020.108436
135. Kang S, Piao Y, Kang YC, Lim S, Pak YK. Qi-activating quercetin alleviates mitochondrial dysfunction and neuroinflammation in vivo and in vitro. Arch Pharm Res. (2020) 43:553–66. doi: 10.1007/s12272-020-01238-x
136. Tan Z, Yang G, Qiu J, Yan W, Liu Y, Ma Z, et al. Through Regulating Microglial Phenotype Transformation to Mitigate Neuropsychiatric Symptoms in Mice with Vascular Dementia. Mol Neurobiol. (2022). doi: 10.1007./s12035-021-02712-3
137. Olayinka J, Eduviere A, Adeoluwa O, Fafure A, Adebanjo A, Ozolua R. Quercetin mitigates memory deficits in scopolamine mice model via protection against neuroinflammation and neurodegeneration. Life Sci. (2022) 292:120326. doi: 10.1016/j.lfs.2022.120326
138. Fan H, Tang HB, Shan LQ, Liu SC, Huang DG, Chen X, et al. Quercetin prevents necroptosis of oligodendrocytes by inhibiting macrophages/microglia polarization to M1 phenotype after spinal cord injury in rats. J Neuroinflammation. (2019) 16:206. doi: 10.1186/s12974-019-1613-2
139. Schültke E, Kendall E, Kamencic H, Ghong Z, Griebel RW, Juurlink BH. Quercetin promotes functional recovery following acute spinal cord injury. J Neurotrauma. (2003) 20:583–91. doi: 10.1089/089771503767168500
140. Wang Y, Li W, Wang M, Lin C, Li G, Zhou X, et al. Quercetin reduces neural tissue damage and promotes astrocyte activation after spinal cord injury in rats. J Cell Biochem. (2018) 119:2298–306. doi: 10.1002/jcb.26392
141. Lu T, Zhang C, Chai M, An Y. Isoquercetin ameliorates tunicamycin-induced apoptosis in rat dorsal root ganglion neurons via suppressing ROS-dependent endoplasmic reticulum stress. Biomed Pharmacother. (2016) 80:343–51. doi: 10.1016/j.biopha.2016.03.039
142. Chen T, Zhang X, Zhu G, Liu H, Chen J, Wang Y, et al. Quercetin inhibits TNF-α induced HUVECs apoptosis and inflammation via downregulating NF-kB and AP-1 signaling pathway in vitro. Medicine (Baltimore). (2020) 99:e22241. doi: 10.1097/MD.0000000000022241
143. Zhang Q, Wang X, Cao S, Sun Y, He X, Jiang B, et al. Berberine represses human gastric cancer cell growth in vitro and in vivo by inducing cytostatic autophagy via inhibition of MAPK/mTOR/p70S6K and Akt signaling pathways. Biomed Pharmacother. (2020) 128:110245. doi: 10.1016/j.biopha.2020.110245
144. Ye G, Lin C, Zhang Y, Ma Z, Chen Y, Kong L, et al. Quercetin alleviates neuropathic pain in the rat CCI model by mediating AMPK/MAPK pathway. J Pain Res. (2021) 14:1289–301. doi: 10.2147/JPR.S298727
145. Rinwa P, Kumar A. Quercetin along with piperine prevents cognitive dysfunction, oxidative stress and neuro-inflammation associated with mouse model of chronic unpredictable stress. Arch Pharm Res. (2017) 40:1166–75. doi: 10.1007/s12272-013-0205-4
146. Hu T, Lu XY, Shi JJ, Liu XQ, Chen QB, Wang Q, et al. Quercetin protects against diabetic encephalopathy via SIRT1/NLRP3 pathway in db/db mice. J Cell Mol Med. (2020) 24:3449–59. doi: 10.1111/jcmm.15026
147. Li L, Wan G, Han B, Zhang Z. Echinacoside alleviated LPS-induced cell apoptosis and inflammation in rat intestine epithelial cells by inhibiting the mTOR/STAT3 pathway. Biomed Pharmacother. (2018) 104:622–8. doi: 10.1016/j.biopha.2018.05.072
148. Lou M, Zhang LN, Feng J, Liu JH, Yang C, Li BF, et al. nanoparticles induced autophagy and apoptosis through AKT/ERK/Caspase-3 signaling pathway in human neuroglioma cells: In vitro and in vivo. Biomed Pharmacother. (2016) 84:1–9. doi: 10.1016/j.biopha.2016.08.055
149. Endale M, Park SC, Kim S, Kim SH, Yang Y, Cho JY, et al. Quercetin disrupts tyrosine-phosphorylated phosphatidylinositol 3-kinase and myeloid differentiation factor-88 association, and inhibits MAPK/AP-1 and IKK/NF-κB-induced inflammatory mediators production in RAW 264.7 cells. Immunobiology. (2013) 218:1452–67. doi: 10.1016/j.imbio.2013.04.019
150. Cao JH, Xue R, He B. Quercetin protects oral mucosal keratinocytes against lipopolysaccharide-induced inflammatory toxicity by suppressing the AKT/AMPK/mTOR pathway. Immunopharmacol Immunotoxicol. (2021) 43:519–26. doi: 10.1080/08923973.2021.1948565
151. Chen BL, Wang LT, Huang KH, Wang CC, Chiang CK, Liu SH. Quercetin attenuates renal ischemia/reperfusion injury via an activation of AMP-activated protein kinase-regulated autophagy pathway. J Nutr Biochem. (2014) 25:1226–34. doi: 10.1016/j.jnutbio.2014.05.013
152. Ocal O, Borcek AO, Pasaoglu O, Gundogdu AC, Kaplanoglu GT, Baykaner MK. Can Quercetin be an Option for Treatment of Spinal Cord Injury? An Experimental Study. Turk Neurosurg. (2019) 29:247–53. doi: 10.5137/1019-5149
153. Huang C, Fu C, Qi ZP, Guo WL, You D, Li R, et al. Localised delivery of quercetin by thermo-sensitive PLGA-PEG-PLGA hydrogels for the treatment of brachial plexus avulsion. Artif Cells Nanomed Biotechnol. (2020) 48:1010–21. doi: 10.1080/21691401.2020.1770265
154. Schültke E, Kamencic H, Skihar VM, Griebel R, Juurlink B. Quercetin in an animal model of spinal cord compression injury: correlation of treatment duration with recovery of motor function. Spinal Cord. (2010) 48:112–7. doi: 10.1038/sc.2009.111
155. Schültke E, Griebel RW, Juurlink BH. Quercetin administration after spinal cord trauma changes S-100 levels. Can J Neurol Sci. (2010) 37:223–8. doi: 10.1017/s0317167100009963
156. Song Y, Liu J, Zhang F, Zhang J, Shi T, Zeng Z. Antioxidant effect of quercetin against acute spinal cord injury in rats and its correlation with the p38MAPK/iNOS signaling pathway. Life Sci. (2013) 92:1215–21. doi: 10.1016/j.lfs.2013.05.007
157. Deng Y, Yang L, Xie Q, Yang F, Li G, Zhang G, et al. Protein kinase A is involved in neuropathic pain by activating the p38MAPK pathway to mediate spinal cord cell apoptosis. Mediators Inflamm. (2020) 2020:6420425. doi: 10.1155/2020/6420425
158. Yang L, Wu Y, Lin S, Dai B, Chen H, Tao X, et al. sPLA2-IB and PLA2R mediate insufficient autophagy and contribute to podocyte injury in idiopathic membranous nephropathy by activation of the p38MAPK/mTOR/ULK1ser757 signaling pathway. FASEB J. (2021) 35:e21170. doi: 10.1096/fj.202001143R
159. He Y, Cao X, Guo P, Li X, Shang H, Liu J, et al. Quercetin induces autophagy via FOXO1-dependent pathways and autophagy suppression enhances quercetin-induced apoptosis in PASMCs in hypoxia. Free Radic Biol Med. (2017) 103:165–76. doi: 10.1016/j.freeradbiomed.2016.12.016
160. Huang Y, Chen Y, Shaw AM, Goldfine H, Tian J, Cai J. Enhancing TFEB-mediated cellular degradation pathways by the mtorc1 inhibitor quercetin. Oxid Med Cell Longev. (2018) 2018:5073420. doi: 10.1155/2018/5073420
161. Du G, Zhao Z, Chen Y, Li Z, Tian Y, Liu Z, et al. Quercetin attenuates neuronal autophagy and apoptosis in rat traumatic brain injury model via activation of PI3K/Akt signaling pathway. Neurol Res. (2016) 38:1012–9. doi: 10.1080/01616412.2016.1240393
Keywords: quercetin, mTOR signaling pathway, spinal cord injury, inflammation, apoptosis, autophagy mTOR signaling pathway, autophagy
Citation: Wang X, Fu Y, Botchway BOA, Zhang Y, Zhang Y, Jin T and Liu X (2022) Quercetin Can Improve Spinal Cord Injury by Regulating the mTOR Signaling Pathway. Front. Neurol. 13:905640. doi: 10.3389/fneur.2022.905640
Received: 27 March 2022; Accepted: 06 April 2022;
Published: 20 May 2022.
Edited by:
Xifan Mei, First Affiliated Hospital of Liaoning Medical University, ChinaReviewed by:
Yajiang Yuan, Jinzhou Medical University, ChinaFrancesco Fornai, University of Pisa, Italy
Copyright © 2022 Wang, Fu, Botchway, Zhang, Zhang, Jin and Liu. This is an open-access article distributed under the terms of the Creative Commons Attribution License (CC BY). The use, distribution or reproduction in other forums is permitted, provided the original author(s) and the copyright owner(s) are credited and that the original publication in this journal is cited, in accordance with accepted academic practice. No use, distribution or reproduction is permitted which does not comply with these terms.
*Correspondence: Xuehong Liu, bGl1eHVlaDY1ODhAMTI2LmNvbQ==
†These authors have contributed equally to this work