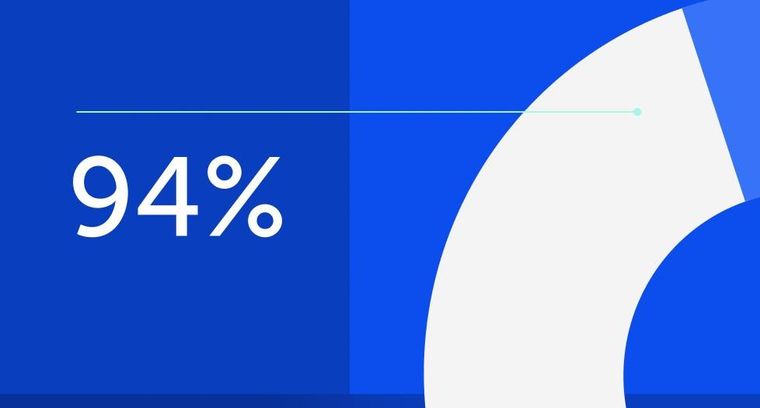
94% of researchers rate our articles as excellent or good
Learn more about the work of our research integrity team to safeguard the quality of each article we publish.
Find out more
ORIGINAL RESEARCH article
Front. Neurol., 19 July 2022
Sec. Neurotrauma
Volume 13 - 2022 | https://doi.org/10.3389/fneur.2022.904593
This article is part of the Research TopicVestibular Issues Following Traumatic Brain InjuryView all 9 articles
Background: Impairments to oculomotor (OM) and vestibulo-ocular reflex (VOR) function following pediatric mTBI have been demonstrated but are poorly understood. Such impairments can be associated with more negative prognosis, affecting physical and mental wellbeing, emphasizing the need to more fully understand how these evolve.
Objectives: to determine i) the extent to which performance on clinical and computerized tests of OM and VOR function varies over time in children and adolescents at 21 days, 3-, and 6-months post-mTBI; ii) the proportion of children and adolescents with mTBI presenting with abnormal scores on these tests at each timepoint.
Design: Prospective longitudinal design.
Setting: Tertiary care pediatric hospital.
Participants: 36 participants with mTBI aged 6 to18.
Procedures: Participants were assessed on a battery of OM and VOR tests within 21 days, at 3- and 6-months post injury.
Outcome measures: Clinical measures: Vestibular/ocular motor screening tool (VOMS) (symptom provocation and performance); Computerized measures: reflexive saccade test (response latency), video head impulse test (VOR gain), and dynamic visual acuity test (LogMAR change).
Analysis: Generalized estimating equations (parameter estimates and odd ratios) estimated the effect of time. Proportions above and below normal cut-off values were determined.
Results: Our sample consisted of 52.8% females [mean age 13.98 (2.4) years, assessed on average 19.07 (8–33) days post-injury]. Older children performed better on visual motion sensitivity (OR 1.43, p = 0.03) and female participants worse on near point of convergence (OR 0.19, p = 0.03). Change over time (toward recovery) was demonstrated by VOMS overall symptom provocation (OR 9.90, p = 0.012), vertical smooth pursuit (OR 4.04, p = 0.03), voluntary saccade performance (OR 6.06, p = 0.005) and right VOR gain (0.068, p = 0.013). Version performance and VOR symptom provocation showed high abnormal proportions at initial assessment.
Discussion: Results indicate impairments to the VOR pathway may be present and driving symptom provocation. Vertical smooth pursuit and saccade findings underline the need to include these tasks in test batteries to comprehensively assess the integrity of OM and vestibular systems post-mTBI.
Implications: Findings demonstrate 1) added value in including symptom and performance-based measures in when OM and VOR assessments; 2) the relative stability of constructs measured beyond 3 months post mTBI.
Children and adolescents are particularly susceptible, to mTBI/concussion (1, 2), when compared with most adult populations, with rates of pediatric mTBI estimated between 1.1 and 1.9 million each year in the USA (3), and the highest rates demonstrated among adolescents 12–17 year old (4). This may be due to anatomical, physiologic and developmental factors such as continuing maturation (5), incomplete myelination of the brain and a more flexible skeletal structure (6, 7). Environmental factors and activities of daily life in this age group can also contribute to their overall exposure to potentially high-risk situations (i.e., chaotic settings in sports/recreation participation, school yards and gym class). While most recover within 4 weeks, approximately one third of youth can suffer persistent symptoms 3 months post-mTBI (8) and 12–14% will present symptoms for more than 1 year post-mTBI (9, 10). Persisting symptoms can put children and adolescents at risk of negative physical and/or psychosocial repercussions resulting from a longer recovery process (11, 12).
While mTBI can lead to a wide range of disturbances, the impact on oculomotor (OM) and vestibulo-ocular reflex (VOR) function in pediatric mTBI has recently attracted a large amount of interest. Relevant literature has outlined high rates of reported abnormalities and/or impairments in eye movements controlled by the OM system (24–73%) (13–16) and in VOR function (43–69%) in children and adolescents (17–20). Such a high prevalence of abnormalities and/or impairments can have a significant impact on one's ability to navigate their environment and participate in recreational activities. In children and adolescents this may consequently affect their overall mental, physical, emotional and psychosocial health and wellbeing.
Abnormalities and/or impairments discussed can be quite impactful as uncompromised functioning of the OM system enables the eyes to move to allow for clear, binocular vision, as well as maintain un-impaired tracking and smooth movements of the eyes (21). The VOR and the ability to suppress this reflex allows one to maintain gaze stability on static and dynamic targets, respectively, while the head is in motion.
Impairments and/or symptoms related to OM and VOR function present in the acute phase of recovery following pediatric mTBI are predictors of prolonged recovery (18, 22). However, their evolution over time is poorly understood. Such questions have prompted recommendations for studies to measure OM and VOR function over time beyond the acute and subacute phase (23, 24) to better understand their overall recovery patterns. While a small number of studies have started to address this recommendation in pediatric mTBI populations, they almost exclusively end follow-up assessments at time of medical clearance and/or return to play (18, 19, 22, 25–27), and their focus has mostly been limited to sport-related concussion (19, 22, 25–28). In addition, these studies are heterogeneous with regards to the time from injury to initial evaluation, including samples with initial assessment in the acute time period (<10 days) (26, 27), the sub-acute time period (<4 weeks) (18, 19, 22, 25) and even portions of samples in the chronic period (>1 month) (19, 28). These limitations should be addressed to: (1) understand the evolution of OM and VOR function post mTBI during and beyond the acute/sub-acute phase in order to determine further characteristics of impairments that may persist; and (2) confirm that OM and VOR functions indeed remain uncompromised at the time of one's return to sport and daily life.
While OM and VOR studies in pediatric mTBI often include only two timepoints of interest in their results (frequently initial clinical presentation and time to recovery) and are performed through retrospective chart reviews (18, 19, 22, 25), two recent studies included data from multiple timepoints. Such studies evaluating injured individuals beyond time of initial injury and recovery are imperative as they allow recovery progress (or lack thereof) to be monitored and evaluated. Moreover, these studies provide information to ensure the recovery is complete and remains stable when the individual returns to their regular activities of daily life as this can trigger the re-emergence of certain symptoms.
The first study referenced, by Sinnott et al. (26), assessed OM and VOR-related symptom provocation initially within 10 days, at 11–21 days and followed 63 adolescent athletes with concussion to medical recovery (time to recovery of 3 groups ranged from 22.95 to 34.94 days). Assessments were conducted in the acute, subacute and prolonged/persistent phases. However, generalizability was limited (athletic populations and sport-related concussion) and the findings only speak to the evolution of these functions up to the time of medical clearance for return to activities. The second, by Zaslow et al. (28), included 3 timepoints: initial evaluation, return to play clearance and 1 month following return to play (RTP) clearance. This study produced findings demonstrating stable OM function beyond RTP clearance. However, VOR variables were limited and the sample size was small (13 adolescents) and exclusive to athletes (28).
Overall, there are very few studies examining OM and VOR function over time in youth post-mTBI (29, 30), particularly in primary school age children (6–12 years) and non-athletes (31). As the mechanism of injury influences the injury response and pathophysiological mechanisms involved (32, 33), recruiting participants with mTBI resulting from all mechanisms is of importance to allow progress to be made in understanding the heterogeneity of mTBI-response. More fully understanding the specific impairments that may compromise OM and VOR function following mTBI, the rate at which such impairments present, as well as characterizing how they resolve, will help guide treatments delivered to ultimately hasten the return to activities of daily living in these youths.
The primary objective of this study was to determine the extent to which performance on clinical and computerized tests of OM and VOR function varies over time in children and adolescents at 21 days, 3, and 6 months after a mild TBI. The secondary objective was to determine the proportion of children and adolescents with mTBI presenting with abnormal scores on OM and VOR tests at each timepoint when compared to cut-off scores pre-determined from published literature. Finally, a tertiary objective was to identify covariates that may contribute to observed changes over time.
We hypothesized that (1) performance would vary over time in tests for which higher abnormal rates of performance were recorded at initial assessment and (2) proportions with abnormal scores would decrease from initial assessment to 3 months and remain stable at 6 months demonstrating a trend toward recovery. We hypothesize that certain covariates known to influence injury response in the context of mTBI will demonstrate a significant effect on test performance.
This study used a prospective longitudinal design to characterize the evolution of children and adolescents' performance in OM and VOR function within 21 days of injury, as well as 3 months and 6 months after a mTBI. These timepoints were selected as they represent (1) the acute-subacute period following injury when injuries are likely to recover spontaneously, (2) a time where most deficits are resolved following mTBI (3 months), and (3) a timepoint corresponding to long-term deficits (6 months). A consecutive non probabilistic convenience sample of participants were recruited from the Emergency Department and mTBI follow-up program of a tertiary care pediatric trauma center, the Montréal Children's Hospital (McGill University Health Center) as well as at the University of Calgary Sport Medicine Centre or the Acute Sport Concussion Clinic (University of Calgary). The study was approved by the Pediatric panel of the McGill University Health Center Research Ethics Board and by the Conjoint Health Research Ethics Board at the University of Calgary.
This study was a sub-study of a larger multi-national project (the SiMPLy Rehab initiative). Participants aged 6 to 18 and assessed within 3 weeks of sustaining a physician-diagnosed mTBI (34) were included in the SimplyRehab study. Diagnosis of mTBI (as referenced) was aligned with current best practice in the Quebec trauma system. The participants included in this sub-study were those who had completed the 3 planned evaluations during the follow-up period of this larger project. Participants were excluded if any of the following were present: (i) history of a previous TBI in the preceding 6 months or any previous TBI with unresolved symptoms/impairments; (ii) presence of comorbidities that would restrict, negatively influence or prevent the participant's ability to complete the study protocol (i.e., spinal cord injury, orthopedic or neurological condition, severe visual, vestibular, or auditory deficit); (iii) medications which affect the vestibular system; (iv) participants who consented but withdrew prior to initial assessment. Prior to enrolling in the study all participants received standard acute concussion assessment/initial management from either the emergency department, a walk-in medical clinic, a pediatrician or a family physician. For the majority of participants (Montréal-based), care was guided by the Montréal Children's Hospital Concussion KiT (35), providing a plan for general activity management, return to learn and return to physical activity/sports.
All assessments took place at the mTBI/concussion program within the Montreal Children's Hospital (Montréal participants) or at the Concussion Lab at the University of Calgary (Calgary participants). Participants were approached, screened for inclusion and consented to participate. They then completed three evaluations over a period of 6 months. Initial assessment within 21 days, 3 month assessment within 3 and 6 month assessment within 6 months of injury. Prior to scheduled assessments at each time point, participants completed patient-reported outcome measures online. In-person evaluation consisted of clinical and computerized measures of OM and VOR function (outlined below) as well as of additional assessments of balance and gait included to better describe our sample. Sessions lasted approximately 75 min.
There is currently no gold standard measure to assess OM and VOR function post-mTBI. As such, a battery of tests was included to assess OM function, VOR response, gaze stability, as well as VOR suppression. Computerized and standard clinical assessments were included and are described below. The outcome measures included measure a wide range of unique variables. Table 1 outlines and defines the study variables, providing cut-off values for normal vs. abnormal findings in each outcome.
Table 1. Variables analyzed to determine changes over time in subcomponents contributing to OM and VOR function.
The VOMS (30, 36) was used as a clinical outcome measure for OM/VOR function. The VOMS is a clinical screening tool of the vestibulo-ocular and OM function that was developed specifically to assess symptom provocation (headache, dizziness, nausea, and fogginess) induced by common VOR and OM tasks in individuals who have sustained a concussion (30). The VOMS includes 7 tasks covering OM function (smooth pursuit, horizontal saccades, vertical saccades, and near point of convergence), VOR function (horizontal VOR and vertical VOR) as well as visual motion sensitivity (VMS, measuring VOR suppression). As per test protocol, prior to beginning the VOMS, each participant rated their current symptoms using a 0 (no symptom) to 10 (severe symptoms) point scale for four commonly reported symptoms: headache, nausea, dizziness, and fogginess. They then rated their symptoms following each task, and change from initial symptom score on the four symptom scales was summed to yield a total change score for each VOMS task. As previous literature has demonstrated cutoff scores of ≥ 2 total symptoms after any VOMS item to have a high rates (96%) of identifying concussion (30), and the presence of symptom provocation on at least 1 VOMS task to have negative effects on recovery (22), a variable of overall symptom provocation was used in this study. This variable was defined as an increase of 2 or more points on any task in the VOMS according to optimal change score cut-off scores recently published in Elbin et al. (37).
Clinical performance: In addition to noting the symptom provocation induced by each task, assessors also noted performance-based observations (normal-abnormal, qualitative descriptors) for each task included in the VOMS: smooth pursuit (vertical and horizontal), voluntary saccades (vertical and horizontal), convergence, VOR (vertical and horizontal) and VOR suppression (VMS task), as well as measured near point of convergence (NPC, cm). Symptom change score reported for each subcomponent was included to fulfill our secondary objective and thus described using proportions and means.
Additional clinical outcome measures to comprehensively characterize our sample at each timepoint include patient-reported outcome measures, cervical measures and balance measures (full descriptions can be found in Supplementary Table 1).
This allowed for computerized testing of reflexive saccades performed using the ICS Impulse software (39) (ICS® Impulse software, Natus®). This product's hardware is composed of accelerometers and a camera. It is combined with rapid computerized pupil tracking software. In this test the patient is required to wear goggles that project visual horizontal saccade stimuli (laser dots appearing horizontally) onto a flat surface while eye position data is captured. The variable of interest for this test was latency (38).
This test was selected to quantify VOR gain, assessing the corresponding horizontal semicircular canals. In this test, the patient is sitting, facing the wall, maintaining their gaze on a fixation dot, while the tester rotates the patient's head horizontally 10–20 degrees in short abrupt unpredictable movements left and right (39).
The NeuroCom InVision System (40) was selected to provide a behavioral measure of VOR. To perform this test, the patient is seated 10 feet from the screen. The software first determines the static visual acuity by having a participant respond to a series of tumbling E displays of varying sizes (stating the direction in which the E is facing: up, down, left, right) determined by an algorithm. The test is then performed dynamically (DVA test) with fixed velocity head movements at 120 deg/sec. For the duration of the DVA test, a head tracker with built in accelerometers is worn by the patient in order to precisely quantify the head velocity at which their responses occur.
At <21 days, 3 and 6 months (study time points), participant performance was reported as means and standard deviation for continuous variables and proportions and percentages for categorical variables (study timepoints consisted of each time of assessment and thus were categorical). To address our primary objective, generalized estimating equations (GEE) taking account of within-patient variation were used to estimate the effect of time on VOMS overall symptom provocation, performance on each VOMS task (smooth pursuit, horizontal and vertical saccades, convergence, horizontal and vertical VOR and VOR suppression), NPC, reflexive saccades (ICS Impulse), VOR gain (ICS Impulse) and DVA (InVision). Models included the fixed effect of time with adjustment for covariates including age, sex and time since injury at the time of initial evaluation. Odds ratios (OR) for dichotomized outcome variables and differences in continuous outcome variables were estimated with 95% confidence intervals with the significance level set at 0.05. With regards to the link functions, in the GEE logistic regression, the “logit” link function was used while in GEE linear regression the “Gaussian” link was used.
To address our secondary objective, proportions above and below previously published normal cut-off values were determined for outcome variables at each evaluation time point.
Sample descriptive characteristics are presented in Table 2. The sample included 52.8% females and 47.2% males, with a mean age of 13.98 (SD: 2.40, 7–17 years) and among which the majority were right-handed (89%). The average time from injury to initial assessment was 19.07 days (SD: 5.93, 8–33 days). Two participants slightly outside our permissible range for initial assessment (>21+7 days, participants remained in the subacute stage of recovery) remained in the analysis as their values did not differ significantly from the rest of the sample (see limitations). Injuries in our sample occurred from sport (80.6%) or recreation/other (19.4%) with the most common location of injury being the frontal (20.6%), left temporal (23.5%) and occipital region (20.6%). In our sample, 50% of participants did not have a history of previous mTBI, 25% had suffered one, and 25% had suffered two or three previous mTBI. To further characterize the sample, Table 3 contains scores for post-concussion symptoms, cervical examination, balance/vestibulospinal measures, and global outcome at each timepoint.
Table 3. Post-concussion symptoms, cervical, balance, functional and global outcome measures over time (see Supplementary Table 1, for explanation of outcome measures).
Table 4 presents VOMS change scores, proportions of participants with symptom provocation (≥2 point increase) and clinician-rated abnormal performance on each of the VOMS tasks, as well as mean performance on computerized outcome measures.
Table 4. Mean symptom change, proportions above symptom cut-offs and proportions demonstrating abnormal performance on oculomotor and vestibulo-ocular tasks.
For our primary objective, our GEE model adjusted change estimates and OR showed no effect of time since injury on any of the outcomes analyzed. Time since injury was therefore not considered further in the GEE models for this objective.
Outcomes showing significant change from initial assessment to 3-month assessment (indicating higher odds of demonstrating normal performance by 3-month assessment as compared to initial assessment) include VOMS overall symptom provocation (OR 9.90, p = 0.01), vertical smooth pursuit performance (OR 4.04, p = 0.03), vertical voluntary saccades performance (OR 6.06, p = 0.01), and VOR gain to the right (0.07, p = 0.01, 95% CI: 0.01–0.12). Outcomes showing significant changes from initial assessment to 6-month assessment (indicating higher odds of demonstrating normal performance by 6-month assessment as compared to initial assessment) include vertical smooth pursuit performance (OR 3.12, p = 0.04) and vertical voluntary saccade performance (OR 5.91, p = 0.01). See Table 5 for detailed results related to the GEE analysis.
For our secondary objective, proportions above and below cut-off values for all outcomes can be found in Table 4 and Figure 1 (VOMS symptoms only). Omitting performance on the DVA test, the saccade and smooth pursuit tasks on the VOMS had the highest proportion of observed abnormal performance, while the VOMS VOR tasks had the highest proportion of abnormal symptom provocation. Unusually high proportions of abnormal performance on the DVA InVision test were found across all three timepoints. These results will be discussed below.
Figure 1. Abnormal performance and symptom provocation proportions over time. This figure outlines the proportions of our sample demonstrating symptom provocation of 2 or more (top) and abnormal performance (bottom) on each VOMS component. VOR, vestibulo-ocular reflex; VMS, visual motion sensitivity; T, timepoint.
For our tertiary objective, an age effect was identified on performance for the VOR suppression task on the VOMS (OR 1.43, p = 0.03) demonstrating that the odds of having normal VOR suppression performance increases with age. Finally, female sex increased the odds of having abnormal NPC (OR 0.19, p = 0.03).
Identifying impairments and understanding the mechanisms that contribute to persistent symptoms following pediatric mTBI can be challenging. In the past, symptom report measures have typically been used given they are simple to administer and quick and easy to interpret. However, they are not precise or objective. Moreover, in pediatric populations, concussion-related symptom reporting has been found to be inconsistent (41, 42). Specific to OM and VOR symptom provocation, 3–21% of young athletes reported symptom improvement (rather than provocation) in the VOMS while completing testing (27) reinforcing certain challenges with such measures within this age group.
This study included more targeted evaluations of OM and VOR function to elucidate whether changes or compromises to specific subcomponents of these functions may persist over time beyond symptom resolution. As such, the study included symptom-based measures and objective measures of OM eye movements and VOR responses contributing to overall OM and VOR function. Outcomes were analyzed by subcomponents in order to determine the effect of mTBI on each in this pediatric sample.
The primary objective of this study was to determine the extent to which performance on clinical and computerized tests of OM and VOR function varies over time in children and adolescents at 21 days, 3, and 6 months after a mTBI. The results demonstrated statistically significant change over time from initial assessment to follow-up assessment(s) in the specific outcomes outlined and discussed below.
The secondary objective of this study was to determine the proportion of children with mTBI presenting with abnormal scores on OM and VOR tests at each timepoint. In terms of performance, our findings demonstrated that vertical smooth pursuits and vertical voluntary saccade performance displayed the greatest abnormal proportions. In terms of symptom provocation induced, when considering both the mean symptom change and the proportions with a symptom increase ≥ 2, horizontal VOR, vertical VOR and VMS symptom provocation displayed the highest means and proportions at initial assessment. Proportions then stabilized by 3 and 6 month assessments with the percentage of abnormal proportions at these timepoints (0–11.11% across all VOMS tasks) remaining within the normative values reported in previous literature (36, 43).
The tertiary objective of this study was to identify covariates that may contribute to observed changes over time. Age and sex were found to have an effect on VMS and NPC, respectively.
VOMS overall symptom provocation demonstrated significant change over time (p = 0.01). The odds of reporting symptom provocation on the VOMS assessment decreased from time of initial assessment to follow-up assessment. More specifically, the horizontal VOR, vertical VOR and VMS components of the VOMS contributed most to overall symptom provocation at initial assessment with decreasing contributions (seen through VOMS symptom change values) by 3 and 6 month assessments (Table 4). These findings are consistent with previous pediatric mTBI literature that has demonstrated a high prevalence of vestibulo-ocular dysfunctions (28.6–62.5%) (19, 44) and that has demonstrated scores on the VMS and VOR components of the VOMS to be predictive of concussion diagnosis (30).
As the VOR task targeted gaze stabilization (with active head motion) and the VMS task targeted VOR suppression through gaze pursuits while performing full-body rotations (active standing position), the symptom provocation induced may be representative of a sensory conflict (45) between the visual and vestibular systems during the tasks. The present study's results reinforce this possibility as the VOR and VMS components of the VOMS induced symptoms at initial assessment, while performance on an objective measure of VOR function (the vHIT) and clinician observed performance of VOR and VMS VOMS tasks at this same assessment were largely normal.
This sensory conflict may stem from an impairment at some point along the vestibular pathways mediating (1) the VOR response to stabilize gaze [to maintain a stable gaze it is crucial to have a fully functional VOR and intact VOR pathway (46)] and/or (2) the VOR inhibition required to generate the appropriate gaze pursuit response [in this instance to allow for gaze pursuit, neurons involved in the VOR's motor response must be attenuated or inhibited (46–48). Predictive pursuit commands contributing to VOR suppression may also play a role (49, 50)]. While outlining the specific neural pathways involved with the VOR and VOR suppression is beyond the scope of this paper, an in-depth understanding can be gained in Cullen and Roy (46), Cullen (47), Roy and Cullen (48), and Belton and McCrea (49). Future research with more precise measures could explore these findings to understand the physiological mechanisms that underly the symptoms induced. As impairments to the VOR seem to be quite responsive to targeted vestibular rehabilitation (51), understanding the mechanisms at play will allow these targeted approaches to be further refined.
A significant change over time was observed in right VOR gain (p = 0.01) as measured by the vHIT. While not significant, a similar trend was also identified in right DVA LogMAR change values (p = 0.06). The sample size and type of outcome measures used do not allow us to draw conclusions as to why this may be. Moreover, as previous literature found no differences in vHIT gain findings in their sample when comparing an mTBI group to a control group (52), further research is warranted to investigate the meaning as well as reproducibility (or not) of findings in the present study.
Nevertheless, previous studies outlining hemispheric asymmetries and lateralized activation patterns influenced by handedness could provide potential explanations as this study's sample contained mostly right-handed individuals (89%).
The role of handedness has been linked to different activation patterns in the brain (53), with right-handed individuals demonstrating pronounced contributions from the right hemisphere when undergoing vestibular stimulation (54). This phenomenon highlights a preference to the non-dominant hemisphere when considering vestibular function (55), consistent with findings by Bronstein et al. (56) and Arshad et al. (57) who demonstrated VOR-specific modulation dependent on their subject's handedness.
While discussing the potential role of handedness in the context of this study's findings is exploratory, future work to understand the specific role of handedness in relation to the reflexive control of eye movements could bring additional insight on this topic. Investigating the control of the VOR specifically at the brainstem and cerebellar vs. the cortical and subcortical levels would be informative as these regions are responsible for the reflexive control of gaze/head/body and self-motion/voluntary movement/balance, respectively, (58).
Our findings show significant change over time in performance on vertical saccade and SP tasks indicating higher odds of demonstrating normal performance by 3-month assessment as compared to initial assessment and by 6-month assessment as compared to initial assessment. These findings indicate the potential value of adding performance quantifiers when using the VOMS to assess the functioning of OM and VOR subcomponents. At the present time, there are few reliable and validated clinical measures that demonstrate the ability to quantify OM and VOR performance improvements over time following mTBI. While a previous study by Anzalone et al. (22) included additional performance quantifiers to the VOMS, it did not separate abnormal symptom provocation from abnormal clinical-observation. In our study, having both symptom provocation and clinician observation allowed the significant changes over time in performance on the vertical SP task (p = 0.03, 3-month assessment and 0.04, 6 month assessment) and performance on the vertical voluntary saccade task (p = < 0.01, 3-month assessment and 0.01, 6 month assessment) in the VOMS to be detected while also allowing us to identify the VOR contributions (previously outlined) contributing to symptom provocation. Had performance quantifiers not been included, the changes in performance on version tasks would have been overlooked. Further, it is of note that the findings of abnormal performance (clinician-observed) on vertical saccades and smooth pursuits tasks of the VOMS did not seem to translate to symptom provocation induced by these same tasks. As many would intuit observed abnormalities in performance to translate to symptom provocation, this mismatch could be explored in future research.
With regards to the directionality of the findings (vertical), this is of interest as neurophysiological contributions to saccades and smooth pursuits in the horizontal vs. vertical directions are not the same. When considering saccades, directional differences were extensively explored in the work of Irving and Lillakas (59). Four important differences were outlined: (i) the pulse innervation from the excitatory burst neurons for horizontal saccades originates from the paramedian pontine reticular formation for the horizontal direction, while this innervation originates in the rostral interstitial nucleus of the medial longitudinal fasciculus for saccades in the vertical direction (60); (ii) the neural integrator differs when considering the horizontal and vertical directions; (iii) while only two extraocular muscles are responsible for moving the eyes horizontally, four extraocular muscles must work in an integrated manner to produce vertical eye movements; and (iv) greater horizontal saccade use was demonstrated by Foulsham et al. (2011) when navigating our environments, outlining that it would be plausible to infer increased horizontal saccade pathway efficiency (59).
With regards to smooth pursuits, while the pathways share similarities, the pathway for vertical SP includes the rostral nucleus reticularis tegmenti pontis (rather than the dorsolateral pontine nuclei in the horizontal direction), involves the y-group nucleus (rather than the medial vestibular nucleus) and involves the dentate nucleus (61). Moreover, in a study by Ingster-Moati et al. (62) maturational differences were demonstrated, highlighting later maturation of the brain networks associated with vertical smooth pursuits (11 years old compared to 8 years old in the horizontal direction).
Overall, such studies along with findings of this present study underline that directional differences observed when measuring OM eye movements are important to consider when interpreting results as positive findings could hold different meanings depending on directionality.
All models in this study included sex, age and time since injury as potential covariates. The findings demonstrate increased odds of normal VMS with increasing age in a pediatric mTBI population. This would suggest improvements in VMS as one matures through adolescence and approaches adulthood. Such findings align with literature indicating higher rates of motion sensitivity in children, with peak incidence rates at pre-adolescence (63), and then decreasing into adulthood (64).
With regards to sex, our findings indicate increased odds of abnormal NPC in female children and adolescents with mTBI, and support similar findings by Gray et al. (20). Further research could explore potential associations between the neural pathways associated with convergence and the structural and functional differences between male and female brains highlighted in the context of concussion by Solomito et al. (65).
Of note, in this study, the high abnormal proportions in the DVA variables underline a need to further investigate: (1) if impairments to associated pathways may be present and causing these distinctively unique clinical findings, thus speaking to potential pathophysiological mechanisms at play in pediatric mTBI; (2) the reliability of the InVision DVA test when used in pediatric mTBI populations as, in addition to our findings, there are varied opinions on the reliability of the DVA InVision test (66–68) and/or; (3) the cut-off values used (this study used a cut-off value of 0.3 LogMAR). Expanding on the latter, while cut-off values of 0.2 and 0.3 are commonly used in clinical DVA assessments, these may not be adequate in the cDVA test. One particular study highlights this with a sample mean cDVA LogMAR change as high as 0.23 (0.13) when tested at 150 deg/s. in healthy NCAA division 1 athletes (69). As such a population would be expected to have superior performances to most, these findings are perplexing. A more reliable approach to cut-off scores when administering the cDVA test may be that used by Goebel et al. (70) who determined a cut-off of 0.33 LogMAR based on 2 SD from the mean of healthy control values obtained in this same study.
Overall, a limitation of this study is the lack of control group to provide comparative values and thus a more rigorous characterization of the change over time observed in this mTBI sample. Additionally, as with all assessments relying on clinician rating, some variability in the interpretation of participants' performance could have occurred.
Individuals recruited from the concussion clinic may have represented a population experiencing more complicated recovery. As the sample consisted of ~80% sport-related mTBI the generalizability of this study would be most suitable to similar populations. Moreover, six individuals included in this study were seen by a physiotherapist prior to their initial evaluation. While their values do not demonstrate obvious differences from the sample, this could lead to overestimating the effect of time on recovery. Finally, a small protocol deviation occurred with two individuals included in this study assessed at a time slightly beyond the permissible range due to a scheduling error. Values for these individuals did not demonstrate obvious differences from the rest of the sample and as such were included in the analyses.
With regards to our data, three participants, two at initial assessment and one at 3 month assessment demonstrated symptom improvement during the VOMS assessment. These values were removed from symptom provocation calculations as there is no physiological explanation for negative symptom reports (27) thus these would be considered to be inconsistent and unreliable (41). Further, three individuals demonstrated elevated perception times thus, according to software specifications, the validity of their LogMAR values is questionable. Lastly, as this study had a modest sample size, a type I error may have occurred where a difference was identified but may have been due to chance. Further literature is needed to confirm our findings.
With increasing literature focused on OM and VOR function following pediatric mTBI, it is becoming evident that impairments to OM and VOR function may often present in a portion of this population and could influence the recovery process. This is supported by the clinical profile perspective of Kontos et al. (71), which has identified the vestibular and ocular profiles as two of the five distinct clinical trajectories following sport-related concussion. While impairments found within these profiles now often guide physical therapy interventions in mTBI settings, the evolution and precise mechanisms underlying these impairments are poorly understood. It is thus challenging to speak conclusively to the pathophysiology associated with negative prognosis. Continuing to adapt clinical assessments to include more objective rather than uniquely symptom-based measures will help to address this. Ensuring such assessments beyond medical clearance in order to monitor OM and VOR function will be equally important in order to understand whether recovery of these functions is maintained.
This study identified significant changes over time in VOMS overall symptom provocation (driven by the VOR components), vertical voluntary saccade performance, vertical smooth pursuit performance and right VOR gain. The subcomponents with the largest proportions of abnormal performance-based results were vertical voluntary saccade and vertical smooth pursuit performance. The subcomponents with the largest proportions of abnormal symptom-based results were the VOR, VOR cancellation and convergence subcomponents. Furthermore, males had higher odds of having normal NPC, and older children and adolescents had higher odds of having normal VMS performance.
The findings according to subcomponents of OM and VOR function highlight certain important observations. With regards to the VOMS, findings demonstrate: (i) the potential value of further exploring the underlying mechanisms of the VOR tasks that seem to drive overall symptom provocation on the VOMS; (ii) the value of including objective performance quantifiers to the VOMS in order to capture functional abnormalities that may be overlooked if solely relying on symptom provocation; and (iii) the value of including vertical and horizontal components when assessing SPs and saccades. With regards to the asymmetrical change in VOR gain, findings encourage exploring the potential contribution of handedness on the reflexive control of eye movements.
Research exploring specific variables within each subcomponent outlined in this study, using computerized measures to increase the granularity of findings, and with a much larger pediatric mTBI sample would bring valuable insight to help understand the mechanisms underlying impairments to OM and VOR function in this population. Precise findings in specific variables may then encourage future studies to draw links with associated brain regions and neural circuits.
The raw data supporting the conclusions of this article will be made available by the authors, without undue reservation.
The studies involving human participants were reviewed and approved by Pediatric Panel of the McGill University Health Center Research Ethics Board and the Conjoint Health Research Ethics Board at the University of Calgary. Written informed consent to participate in this study was provided by the participants' legal guardian/next of kin.
AC and IG developed the study design and methodology. AC performed the recruitment, statistical analyses, supporting statistician, and data collection. KS, LG, MC, MK-L, MB, CD, and IG supported the laboratory activities. KS, LG, MC, MK-L, MB, and CD contributed insights to discussion sections and interpretations of data analyses. IG provided extensive guidance throughout manuscript development. AC, KS, LG, MC, MK-L, MB, CD, and IG contributed to conclusion and overall manuscript content. All authors contributed to the article and approved the submitted version.
This work was funded by the Canadian Institute of Health Research under grant number EIN 150763 and the Fonds de recherche du Québec under grant number 3679 as part of an ERA-NET NEURON JTC Cofund Program.
Beyond the authorship team, this work acknowledges the contributions of the research coordinators and clinicians at the Montreal Children's Hospital Concussion Clinic and statistician Mr. Xun Zhang. The contents of this manuscript have previously appeared online in author AC's thesis (72).
The authors declare that the research was conducted in the absence of any commercial or financial relationships that could be construed as a potential conflict of interest.
All claims expressed in this article are solely those of the authors and do not necessarily represent those of their affiliated organizations, or those of the publisher, the editors and the reviewers. Any product that may be evaluated in this article, or claim that may be made by its manufacturer, is not guaranteed or endorsed by the publisher.
The Supplementary Material for this article can be found online at: https://www.frontiersin.org/articles/10.3389/fneur.2022.904593/full#supplementary-material
1. Karlin AM. Concussion in the pediatric and adolescent population:“different population, different concerns”. PM R. (2011) 3:S369–79. doi: 10.1016/j.pmrj.2011.07.015
2. Meehan WP, Taylor AM, Proctor M. The pediatric athlete: younger athletes with sport-related concussion. J Clin Sports Med. (2011) 30:133–44. doi: 10.1016/j.csm.2010.08.004
3. Bryan MA, Rowhani-Rahbar A, Comstock RD, Rivara F, Seattle sports concussion research C. sports- and recreation-related concussions in US youth. Pediatrics. (2016) 138:e20154635. doi: 10.1542/peds.2015-4635
4. Zonfrillo MR, Kim KH, Arbogast KB. Emergency department visits and head computed tomography utilization for concussion patients from 2006 to 2011. Acad Em Med. (2015) 22:872–7. doi: 10.1111/acem.12696
5. Buzzini SR, Guskiewicz KM. Sport-related concussion in the young athlete. Curr Opin Pediatr. (2006) 18:376–82. doi: 10.1097/01.mop.0000236385.26284.ec
6. Ommaya AK, Goldsmith W, Thibault L. Biomechanics and neuropathology of adult and paediatric head injury. Br J Neurosurg. (2002) 16:220–42. doi: 10.1080/02688690220148824
7. Cook RS, Schweer L, Shebesta KF, Hartjes K, Falcone RA. Mild traumatic brain injury in children: just another bump on the head? J Trauma Nurs. (2006) 13:58–65. doi: 10.1097/00043860-200604000-00007
8. Lannsjo M, af Geijerstam JL, Johansson U, Bring J, Borg J. Prevalence and structure of symptoms at 3 months after mild traumatic brain injury in a national cohort. Brain Inj. (2009) 23:213–9. doi: 10.1080/02699050902748356
9. Rutherford WH, Merrett JD, McDonald JR. Symptoms at one year following concussion from minor head injuries. Injury. (1979) 10:225–30. doi: 10.1016/0020-1383(79)90015-9
10. Oldenburg C, Lundin A, Edman G, Deboussard CN, Bartfai A. Emotional reserve and prolonged post-concussive symptoms and disability: a swedish prospective 1-Year mild traumatic brain injury cohort study. BMJ Open. (2018) 8:e020884. doi: 10.1136/bmjopen-2017-020884
11. Iadevaia C, Roiger T, Zwart MB. Qualitative examination of adolescent health-related quality of life at 1 year postconcussion. J Athl Train. (2015) 50:1182–9. doi: 10.4085/1062-6050-50.11.02
12. Valovich McLeod TC, Wagner AJ, Bacon CEW. Lived experiences of adolescent athletes following sport-related concussion. Orthop J Sports Med. (2017) 5:2325967117745033. doi: 10.1177/2325967117745033
13. Master CL, Scheiman M, Gallaway M, Goodman A, Robinson RL, Master SR, et al. Vision diagnoses are common after concussion in adolescents. Clin Pediatr. (2016) 55:260–7. doi: 10.1177/0009922815594367
14. Storey EP, Master SR, Lockyer JE, Podolak OE, Grady MF, Master CL. Near point of convergence after concussion in children. Optom Vision Science. (2017) 94:96–100. doi: 10.1097/OPX.0000000000000910
15. Cross E, Podolak OE, Lockyer J, Corwin DJ, Storey EP, Grady MF, et al. Prevalence of vestibular and vision problems following concussion in children under 12. Pediatrics. (2018) 141:201. doi: 10.1542/peds.141.1_MeetingAbstract.201
16. Reneker JC, Cheruvu VK, Yang J, James MA, Cook CE. Physical examination of dizziness in athletes after a concussion: a descriptive study. Musculoskelet Sci Pract. (2018) 34:8–13. doi: 10.1016/j.msksp.2017.11.012
17. Corwin DJ, Wiebe DJ, Zonfrillo MR, Grady MF, Robinson RL, Goodman AM, et al. Vestibular deficits following youth concussion. J Pediatr. (2015) 166:1221–5. doi: 10.1016/j.jpeds.2015.01.039
18. Master CL, Master SR, Wiebe DJ, Storey EP, Lockyer JE, Podolak OE, et al. Vision and vestibular system dysfunction predicts prolonged concussion recovery in children. Clin J Sport Med. (2018) 28:139–45. doi: 10.1097/JSM.0000000000000507
19. Ellis MJ, Cordingley D, Vis S, Reimer K, Leiter J, Russell K. Vestibulo-ocular dysfunction in pediatric sports-related concussion. J Neurosurg Pediatr. (2015) 16:248–55. doi: 10.3171/2015.1.PEDS14524
20. Gray M, Wilson JC, Potter M, Provance AJ, Howell DR. Female adolescents demonstrate greater oculomotor and vestibular dysfunction than male adolescents following concussion. Phys Ther Sport. (2020) 42:68–74. doi: 10.1016/j.ptsp.2020.01.001
21. Pillai C, Gittinger JWJr. Vision testing in the evaluation of concussion. Semin Ophthalmol. (2017) 32:144–52. doi: 10.1080/08820538.2016.1228412
22. Anzalone AJ, Blueitt D, Case T, McGuffin T, Pollard K, Garrison JC, et al. A positive vestibular/ocular motor screening (VOMS) is associated with increased recovery time after sports-related concussion in youth and adolescent athletes. Am J Sports Med. (2017) 45:474–9. doi: 10.1177/0363546516668624
23. Hoffer ME, Balaban C, Szczupak M, Buskirk J, Snapp H, Crawford J, et al. The use of oculomotor, vestibular, and reaction time tests to assess mild traumatic brain injury (mTBI) over time. Laryngoscope Investig Otolaryngol. (2017) 2:157–65. doi: 10.1002/lio2.74
24. Cheever KM, McDevitt J, Tierney R, Wright WG. Concussion recovery phase affects vestibular and oculomotor symptom provocation. Int J Sports Med. (2018) 39:141–7. doi: 10.1055/s-0043-118339
25. Desai N, Wiebe DJ, Corwin DJ, Lockyer JE, Grady MF, Master CL. Factors affecting recovery trajectories in pediatric female concussion. Clin J Sport Med. (2019) 29:361–7. doi: 10.1097/JSM.0000000000000646
26. Sinnott AM, Elbin RJ, Collins MW, Reeves VL, Holl CL, Kontos AP. Persistent vestibular-ocular impairment following concussion in adolescents. J Sci Med Sport. (2019) 22:1292–7. doi: 10.1016/j.jsams.2019.08.004
27. Elbin RJ, Sufrinko A, Anderson MN, Mohler S, Schatz P, Covassin T, et al. Prospective changes in vestibular and ocular motor impairment after concussion. J Neurol Phys Ther. (2018) 42:142–8. doi: 10.1097/NPT.0000000000000230
28. Zaslow T, Mueske NM, Yu GJ, Conrad-Forrest A, Edison B, Wren TAL. Oculomotor function in adolescent athletes following concussion. Orthopaed J Sports Med. (2020) 8:2325967120S0015. doi: 10.1177/2325967120S00150
29. Alsalaheen BA, Mucha A, Morris LO, Whitney SL, Furman JM, Camiolo-Reddy CE, et al. Vestibular rehabilitation for dizziness and balance disorders after concussion. J Neurol Phys Ther. (2010) 34:87–93. doi: 10.1097/NPT.0b013e3181dde568
30. Mucha A, Collins MW, Elbin RJ, Furman JM, Troutman-Enseki C, DeWolf RM, et al. A brief vestibular/ocular motor screening (VOMS) assessment to evaluate concussions: preliminary findings. Am J Sports Med. (2014) 42:2479–86. doi: 10.1177/0363546514543775
31. Graham R Rivara FP Ford MA Spicer CM National Research Council. Committee on sports-related concussions in youth. Sports-Related Concussions in Youth: Improving the Science, Changing the Culture. Washington, DC.: National Academies Press (2014).
32. Saatman KE, Duhaime AC, Bullock R, Maas AI, Valadka A, Manley GT, et al. Classification of traumatic brain injury for targeted therapies. J Neurotrauma. (2008) 25:719–38. doi: 10.1089/neu.2008.0586
33. Pavlovic D, Pekic S, Stojanovic M, Popovic V. Traumatic brain injury: neuropathological, neurocognitive and neurobehavioral sequelae. Pituitary. (2019) 22:270–82. doi: 10.1007/s11102-019-00957-9
34. Carroll L, Cassidy JD, Holm L, Kraus J, Coronado V. Methodological issues and research recommendations for mild traumatic brain injury: the WHO collaborating centre task force on mild traumatic brain injury. J Rehabil Med. (2004) 36:113–25. doi: 10.1080/16501960410023877
35. Montreal Children's Hospital: Trauma. Understanding and Managing Concussion in Youth 2018. Montreal, QC: MCH Trauma. 3.
36. Kontos AP, Sufrinko A, Elbin RJ, Puskar A, Collins MW. Reliability and associated risk factors for performance on the vestibular/ocular motor screening (VOMS) tool in healthy collegiate athletes. Am J Sports Med. (2016) 44:1400–6. doi: 10.1177/0363546516632754
37. Elbin RJ, Eagle SR, Marchetti GF, Anderson M, Schatz P, Womble MN, et al. Using change scores on the vestibular ocular motor screening (Voms) tool to identify concussion in adolescents. Appl Neuropsychol Child. (2021) 24:1–7. doi: 10.1080/21622965.2021.1911806
39. McGarvie LA, MacDougall HG, Halmagyi GM, Burgess AM, Weber KP, Curthoys IS. The video head impulse test (VHIT) of semicircular canal function - age-dependent normative values of VOR gain in healthy subjects. Front Neurol. (2015) 6:154. doi: 10.3389/fneur.2015.00154
40. NeuroCom International I. Clinical Integration Manual- Invision System. Clackamas, OR: NeuroCom International, Inc (2008).
41. Iverson GL, Silverberg ND, Mannix R, Maxwell BA, Atkins JE, Zafonte R, et al. Factors associated with concussion-like symptom reporting in high school athletes. JAMA Pediatr. (2015) 169:1132–40. doi: 10.1001/jamapediatrics.2015.2374
42. Asken BM, Snyder AR, Smith MS, Zaremski JL, Bauer RM. Concussion-like symptom reporting in non-concussed adolescent athletes. Clin Neuropsychol. (2017) 31:138–53. doi: 10.1080/13854046.2016.1246672
43. Moran RN, Covassin T, Elbin R, Gould D. Nogle SJTAjosm. Reliability and normative reference values for the vestibular/ocular motor screening (Voms) tool in youth athletes. Am J Sports Med. (2018) 46:1475–80. doi: 10.1177/0363546518756979
44. Ellis MJ, Cordingley DM, Vis S, Reimer KM, Leiter J, Russell K. Clinical predictors of vestibulo-ocular dysfunction in pediatric sports-related concussion. J Neurosurg: Pediatrics. (2017) 19:38–45. doi: 10.3171/2016.7.PEDS16310
45. Zhang LL, Wang JQ, Qi RR, Pan LL, Li M, Cai YL. Motion sickness: current knowledge and recent advance. CNS Neurosci Ther. (2016) 22:15–24. doi: 10.1111/cns.12468
46. Cullen KE, Roy JE. Signal processing in the vestibular system during active versus passive head movements. J Neurophysiol. (2004) 91:1919–33. doi: 10.1152/jn.00988.2003
47. Cullen KE. The vestibular system: multimodal integration and encoding of self-motion for motor control. Trends Neurosci. (2012) 35:185–96. doi: 10.1016/j.tins.2011.12.001
48. Roy JE, Cullen KE. Vestibuloocular reflex signal modulation during voluntary and passive head movement. J Neurophysiol. (2002) 87:2337–57. doi: 10.1152/jn.2002.87.5.2337
49. Belton T, McCrea RA. Role of the cerebellar flocculus region in cancellation of the VOR during passive whole body rotation. J Neurophysiol. (2000) 84:1599–613. doi: 10.1152/jn.2000.84.3.1599
50. Barnes GR. Visual-vestibular interaction in the control of head and eye movement: the role of visual feedback and predictive mechanisms. Prog Neurobiol. (1993) 41:435–72. doi: 10.1016/0301-0082(93)90026-O
51. Kontos AP, Eagle SR, Mucha A, Kochick V, Reichard J, Moldolvan C, et al. A randomized controlled trial of precision vestibular rehabilitation in adolescents following concussion: preliminary findings. J Ped. (2021) 239:193–9. doi: 10.1016/j.jpeds.2021.08.032
52. Alkathiry AA, Kontos AP, Furman JM, Whitney SL, Anson ER, Sparto PJ. Vestibulo-ocular reflex function in adolescents with sport-related concussion: Preliminary results. Sports Health. (2019) 11:479–85. doi: 10.1177/1941738119865262
53. Li M, Chen H, Wang J, Liu F, Long Z, Wang Y, et al. Handedness- and hemisphere-related differences in small-world brain networks: a diffusion tensor imaging tractography study. Brain Connect. (2014) 4:145–56. doi: 10.1089/brain.2013.0211
54. Dieterich M, Bense S, Lutz S, Drzezga A, Stephan T, Bartenstein P, et al. Dominance for vestibular cortical function in the non-dominant hemisphere. Cerebral Cortex. (2003) 13:994–1007. doi: 10.1093/cercor/13.9.994
55. Arshad Q, Siddiqui S, Ramachandran S, Goga U, Bonsu A, Patel M, et al. Right hemisphere dominance directly predicts both baseline V1 cortical excitability and the degree of top-down modulation exerted over low-level brain structures. Neuroscience. (2015) 311:484–9. doi: 10.1016/j.neuroscience.2015.10.045
56. Bronstein AM, Patel M, Arshad Q. A brief review of the clinical anatomy of the vestibular-ocular connections-how much do we know? Eye. (2015) 29:163–70. doi: 10.1038/eye.2014.262
57. Arshad Q, Nigmatullina Y, Bronstein AM. Handedness-related cortical modulation of the vestibular-ocular reflex. J Neurosci. (2013) 33:3221–7. doi: 10.1523/JNEUROSCI.2054-12.2013
58. Dieterich M, Kirsch V, Brandt T. Right-sided dominance of the bilateral vestibular system in the upper brainstem and thalamus. J Neurol. (2017) 264:55–62. doi: 10.1007/s00415-017-8453-8
59. Irving EL, Lillakas L. Difference between vertical and horizontal saccades across the human lifespan. Exp Eye Res. (2019) 183:38–45. doi: 10.1016/j.exer.2018.08.020
60. Hunfalvay M, Roberts CM, Murray NP, Tyagi A, Kelly H, Bolte T. Horizontal and vertical self-paced saccades as a diagnostic marker of traumatic brain injury. Concussion. (2019) 4:CNC60. doi: 10.2217/cnc-2019-0001
61. Wong AMF. Eye Movement Disorders. Oxford: Oxford University Press (2008). doi: 10.1093/oso/9780195324266.001.0001
62. Ingster-Moati I, Vaivre-Douret L, Bui Quoc E, Albuisson E, Dufier JL, Golse B. Vertical and horizontal smooth pursuit eye movements in children: a neuro-developmental study. Eur J Paediatr Neurol. (2009) 13:362–6. doi: 10.1016/j.ejpn.2008.07.003
63. Graudins L. Preventing motion sickness in children. Aust Prescr. (2009) 32:61–3. doi: 10.18773/austprescr.2009.032
64. Huppert D, Grill E, Brandt T. Survey of motion sickness susceptibility in children and adolescents aged 3 months to 18 Years. J Neurol. (2019) 266:65–73. doi: 10.1007/s00415-019-09333-w
65. Solomito MJ, Reuman H, Wang DH. Sex differences in concussion: a review of brain anatomy, function, and biomechanical response to impact. Brain Inj. (2019) 33:105–10. doi: 10.1080/02699052.2018.1542507
66. Riska KM, Hall CD. Reliability and normative data for the dynamic visual acuity test for vestibular screening. J Otol Neurotol. (2016) 37:545–52. doi: 10.1097/MAO.0000000000001014
67. Mohammad MT, Whitney SL, Marchetti G, Sparto PJ, Ward B, Furman JM. The reliability and response stability of dynamic testing of the vestibulo-ocular reflex in patients with vestibular disease. J Vestib Res. (2011) 21:277–88. doi: 10.3233/VES-2011-0430
68. Kaufman DR, Puckett MJ, Smith MJ, Wilson KS, Cheema R, Landers MR. Test–retest reliability and responsiveness of gaze stability and dynamic visual acuity in high school and college football players. Phys Ther in Sport. (2014) 15:181–8. doi: 10.1016/j.ptsp.2013.10.002
69. Marquez C, Lininger M, Raab S. Establishing normative change values in visual acuity loss during the dynamic visual acuity test. Int J Sports Phys Ther. (2017) 12:227–32.
70. Goebel JA, Tungsiripat N, Sinks B, Carmody J. Gaze stabilization test: a new clinical test of unilateral vestibular dysfunction. Otol Neurotol. (2007) 28:68–73. doi: 10.1097/01.mao.0000244351.42201.a7
71. Kontos A, Sufrinko A, Sandel N, Emami K, Collins MW. Sport related concussion clinical profiles: clinical characteristics, targeted treatments, and preliminary evidence. Curr Sports Med Rep. (2019) 18:82–92. doi: 10.1249/JSR.0000000000000573
Keywords: mild traumatic brain injuries, pediatric, vestibulo-ocular reflex (VOR), assessment, oculomotor
Citation: Crampton A, Schneider KJ, Grilli L, Chevignard M, Katz-Leurer M, Beauchamp MH, Debert C and Gagnon IJ (2022) Characterizing the evolution of oculomotor and vestibulo-ocular function over time in children and adolescents after a mild traumatic brain injury. Front. Neurol. 13:904593. doi: 10.3389/fneur.2022.904593
Received: 25 March 2022; Accepted: 28 June 2022;
Published: 19 July 2022.
Edited by:
Nicholas Gerald Murray, University of Nevada, Reno, United StatesReviewed by:
Kody Campbell, Oregon Health and Science University, United StatesCopyright © 2022 Crampton, Schneider, Grilli, Chevignard, Katz-Leurer, Beauchamp, Debert and Gagnon. This is an open-access article distributed under the terms of the Creative Commons Attribution License (CC BY). The use, distribution or reproduction in other forums is permitted, provided the original author(s) and the copyright owner(s) are credited and that the original publication in this journal is cited, in accordance with accepted academic practice. No use, distribution or reproduction is permitted which does not comply with these terms.
*Correspondence: Adrienne Crampton, YWRyaWVubmUuY3JhbXB0b25AbWFpbC5tY2dpbGwuY2E=
Disclaimer: All claims expressed in this article are solely those of the authors and do not necessarily represent those of their affiliated organizations, or those of the publisher, the editors and the reviewers. Any product that may be evaluated in this article or claim that may be made by its manufacturer is not guaranteed or endorsed by the publisher.
Research integrity at Frontiers
Learn more about the work of our research integrity team to safeguard the quality of each article we publish.