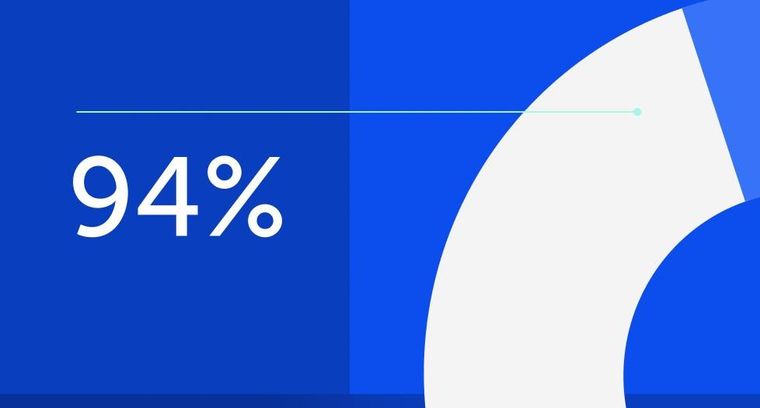
94% of researchers rate our articles as excellent or good
Learn more about the work of our research integrity team to safeguard the quality of each article we publish.
Find out more
ORIGINAL RESEARCH article
Front. Neurol., 29 June 2022
Sec. Neuromuscular Disorders and Peripheral Neuropathies
Volume 13 - 2022 | https://doi.org/10.3389/fneur.2022.902384
Myeloid-derived suppressor cells (MDSCs) are a population of myeloid progenitor cells with immunoregulatory functions and their role in myasthenia gravis (MG) was unknown. In this study, we investigated the phenotypic and functional alterations of MDSCs in MG before and after immunotherapy. The frequency of MDSCs significantly increased and negatively correlated to that of Th1 or Th17 cells after immunotherapy. MDSCs from untreated patients with MG showed an impaired suppression of IFN-γ production in T-cells and improved immunosuppressive function was identified after immunotherapy. The MFI of Arg-1 in MDSCs also increased after immunotherapy. These findings suggested the functional difference in MDSCs before and after immunotherapy, and MDSCs might play a role in disease remission.
Myasthenia gravis (MG), characterized by T-cell involvement and complement dependence, is a group of autoimmune diseases manifested as fluctuating muscle weakness, typically the best in the morning and more pronounced at the end of the day (1). It is caused by antibodies against the components of the post-synaptic muscle endplate at the neuromuscular junction. Eighty percent of patients with MG have detectable antibodies against muscle nicotinic acetylcholine receptors (AChR) and a minority have autoantibody against muscle-specific tyrosine kinase (MuSK) or low-density lipoprotein receptor-related protein 4 (LRP4) (2).
Immune regulatory cells, a small set of immune cells, play an essential role in curbing excessive immune activation and maintaining immune homeostasis. Various immune cells have been found to possess a regulatory capacity, mainly including regulatory T-cells (Tregs), regulatory B-cells (Bregs), macrophages, myeloid-derived suppressor cells (MDSCs), dendritic cells (DCs), and mesenchymal stromal cells (MSCs) (3). Autoimmune disorders may arise when there are defects in the number and function of those cells. In MG, decreased frequency of CD45RA+ FOXP3+ Tregs and reduced suppressive activity of CD4+ CD25high CD127low Tregs have been found (4, 5). Unlike Tregs, Bregs are not well defined due to lacking a specific transcription factor. Both CD1d+CD5+ and CD24high CD38high B cells, which were described to contain an enriched population of Bregs, showed impaired production of IL-10 and suppression of T-helper polarization in MG (6).
Myeloid-derived suppressor cells are a morphologically and functionally heterogeneous population of myeloid progenitor cells with immunoregulatory functions. In humans, MDSCs were identified by HLA-DR−−/low CD11b+CD33+ (HLA-DR, human leukocyte antigen–D-related) (7). Human MDSCs comprise two major subsets classified as CD33+CD11b+ HLA-DR−/low CD14+CD15− monocytic MDSCs (mMDSCs) and CD33+ CD11b+HLA-DR−/lowCD14−CD15+ granulocytic MDSCs (gMDSCs) (8). MDSCs suppress immune responses by several distinct mechanisms, including (1) production of Arginase-1 (Arg-1), inducible nitric oxide synthase (iNOS), and reactive oxygen species (ROS) to suppress T-cell responses and induce T-cell apoptosis; (7) (2) facilitating Tregs induction by releasing IL-10 and transforming growth factor–β (TGF-β) (7, 9); and (3) impairing T-cell function by expressing PD-1/PD-L1 and indoleamine 2,3-dioxygenase (IDO) (10, 11).
Since MDSCs are posited to play a deleterious role in malignancies, they may be functionally beneficial and protective in autoimmunity (12). However, the role of MDSCs in patients with autoimmune diseases is still controversial, and few studies have characterized the role of MDSCs in MG. We hypothesized that MDSCs might have a quantitative or functional defect in patients with MG. In this study, we analyzed the frequencies of circulating MDSCs, their inhibiting capacity on IFN-γ production in T-cells, and their expression of Arg-1 in patients with MG before and after immunotherapy, trying to find the functional alterations of MDSC in MG during different disease stages.
Generalized MG patients with anti-AChR antibodies and healthy controls (HC) were recruited at Neurology Department, Huashan Hospital, Fudan University from 2019 to 2020. Patients who had other autoimmune diseases and received immunotherapy before enrollment were excluded. All patients and HCs in this study had no malignancies, acute or chronic infections, or other autoimmune diseases. All the patients were followed for 6–12 months after steroid immunotherapy with or without immunosuppressants. The study was approved by the Institutional Review Board of Huashan Hospital, Fudan University, and written informed consent was granted from each participant.
Clinical severity, including Myasthenia Gravis Foundation of America (MGFA) classification, quantitative MG (QMG) score (13), and Myasthenia Gravis Activities of Daily Living (MG-ADL) (14) was evaluated at baseline and 6–12 months after immunotherapy, respectively. At the same time, peripheral blood samples were collected and serum anti-AChR antibody level (cutoff:0.45 nmol/L) was detected by radioimmunoassay (Kindstar Global, China).
Peripheral blood mononuclear cells (PBMCs) from healthy controls and patients were freshly isolated by Ficoll-Paque Plus gradient centrifugation (Amersham Biosciences, Uppsala, Sweden).
For analysis of MDSCs, Bregs, and Treg, PBMCs were stained with the following mAbs: anti-human CD14 PE-Cy7, anti-human CD33 PE, anti-human CD11b FITC, anti-human HLA-DR PerCP-Cy5.5, anti-human CD15-APC, anti-human CD127 APC (BD Biosciences, USA), anti-human CD19 PE-Cy7, anti-human CD5 FITC, anti-human CD1d PE, anti-human CD24-PE, anti-human CD38-APC, anti-human CD3 FITC, anti-human CD4 PerCP-Cy5.5, and anti-human CD25-PE (eBioscience, USA).
For analysis of Th1 and Th17 cells, PBMCs were stimulated with 50 ng/ml PMA (Sigma-Aldrich, USA) and 1 μg/ml ionomycin (Sigma-Aldrich) for 6 h. GolgiStop (BD Biosciences) was added 1 h after stimulation. After the washing step, the stimulated cells were stained with anti-human CD4 APC-H7, anti-human CD3 PerCp-Cy5.5, and anti-human CD8 FITC mAbs (BD Biosciences, USA), permeabilized using a Cytofix/Cytoperm Plus kit (BD Biosciences) and intracellularly stained with anti-human IFN-γ PE and anti-human IL-17 APC mAbs (BD Biosciences, USA).
For intracellular staining of human Arg-1, PBMCs were cryopreserved and thawed according to a standardized protocol. Cells then were stained with anti-human CD14, anti-human CD33, anti-human CD11b, anti-human HLA-DR, anti-human CD19, and anti-human CD3 mentioned above as well as anti-human CD56-APC, anti-human CD16-PE (eBioscience, USA), permeabilized using Cytofix/Cytoperm Plus kit (BD Biosciences, USA), and intracellular stained with anti-human Arg1-Alexa Fluor 405 (R&D, USA). Then the mean fluorescence intensities (MFI) of Arg-1 in MDSCs, NK cells, monocytes, T and B-cells were detected.
Flow cytometry data were obtained using a flow cytometer (BD FACS Canto and BD FACS Aria II, USA) and analyzed with Flowjo (Tree Star, USA).
For the function assay associated with MDSC, inhibition of T-cells is the gold standard. Non-specific inhibition of anti-CD3/CD28 induced IFN-γ production in T-cells by intracellular staining is one of the most used methods (8). Considering the difficulty obtaining sufficient MDSCs from PBMCs in patients with MG, we evaluated the impact of MDSCs on IFN-γ production in T-cells using MDSC depleted PBMCs by flow cytometry sorting.
First, MDSCs were depleted by FACS Aria II cell sorter (Becton Dickinson, Franklin Lakes, USA) based on their expression of HLA-DR, CD11b, and CD33 (BD Biosciences, USA). Then, control PBMCs and MDSC-depleted PBMCs were stimulated with.5 μg/ml purified plate-bound anti-CD3 (BD Biosciences, USA) mAb and soluble anti-CD28 (BD Biosciences, USA) mAb for 3 days in 96-well plates (Nunc, Germany), the staining for CD3 and intracellular IFN-γ detection were performed as described above.
Total RNA was extracted from isolated PBMC by TRIzol (Invitrogen, USA) and reverse-transcribed into complementary DNA (cDNA) using a PrimerScript RT Reagent Kit (Takara, China). Relative expressions of Arg-1, iNOS, IL-10, PD-L1, p47phox, gp91phox, and IDO were determined by normalizing the expression of each target gene to β-actin (11). Gene-specific primers are listed in Table S1. 2−(ΔΔCt) was used to calculate the relative fold gene expression in samples after immunotherapy.
Paired (before and after immunotherapy). and unpaired (HCs and patients with MG) samples were tested for statistical significance by the non-parametric Mann-Whitney U-test and parametric Student's t-test, respectively. The correlation coefficient was calculated using Spearman's test for non-parametric data. Numeric values are expressed as mean ± standard deviation, and p < 0.05 was considered statistically significant. All statistical analyses were performed using GraphPad 8 (La Jolla, USA).
We enrolled 30 generalized MG patients with anti-AChR antibodies and 30 HCs. The clinical characteristics of all patients and HCs are summarized in Table 1. The MGFA classification, QMG score, MG-ADL, and the titer of AChR antibody before and after immunotherapy and medication use are shown in Table S2. All the patients were given oral steroids with an initial dose of 20 mg of prednisone every day. The dose was increased by increments of 10 mg every 2 weeks without exceeding.75 mg/kg/d of body weight. The dose was maintained for 1–2 months when showing a ≥2-point reduction in ADL-MG and then reduced by 5 mg every 1–2 months. Intravenous methylprednisolone was administrated in two patients during hospitalization. Non-steroid immunosuppressants were added for patients who did not respond to steroids or refused to take a higher dose of oral steroids. All patients showed clinical improvement, 29/30 patients showed a ≥2-point reduction from baseline in MG-ADL, and only 1/30 showed a 1-point reduction.
To investigate the dynamic change of immune regulatory cells in patients with MG, we compared the frequencies of Tregs and Bregs as well as MDSCs by flow cytometry in patients with MG (n = 13) before (pre-treated) and after immunotherapy (post-treated). We observed a significantly higher frequency of HLA-DR−/low CD11b+CD33+MDSCs in PBMCs in patients after immunotherapy compared to baseline (p = 0.021. Figures 1A–C). While the frequencies of CD24 high CD38 high and CD1d+CD5+ Bregs in CD19+ lymphocytes decreased (p = 0.0032 and 0.019, respectively, Figures 1B,D,E). No significant change was observed (p = 0.24) in the frequency of CD25+CD127low Tregs in CD3+CD4+ lymphocytes (Figures 1B, 1F). So, we focused on the phenotypic and functional changes of MDSCs before and after immunotherapy.
Figure 1. Alternation of immune regulatory cells after immunotherapy. (A) Sequential gating strategy for myeloid-derived suppressor cells (MDSCs) identification (HLA-DR−/lowCD33+CD11b+) from peripheral blood mononuclear cell (PBMCs). (B) Representative data plots of MDSCs, Bregs, and Tregs from patients with MG before (Pre-treated) and after immunotherapy (post-treated). The frequency of MDSCs in PBMCs increased (C), the frequencies of CD19+CD24high CD38high Bregs (D) and CD19+CD5+CD1d+ Bregs (E) in CD19+ B-cells decreased, while the frequency of CD4+CD25+CD27low Tregs in CD3+CD4+ T-cells (F) showed no significant change. Horizontal lines and error bars represent mean ± SD, n = 13, *p < 0.05, **p < 0.01.
Representative data plots of MDSCs, mMDSCs (CD14+CD15−) and gMDSCs (CD14−CD15+) from HC, patients before and after immunotherapy were shown in Figure 2A. The frequencies of MDSC and CD14+CD15− mMDSCs in PBMC from 30 untreated patients with MG did not significantly change compared to those in 30 HCs (1.5 ± 1.1% vs. 1.4 ± 0.5%, p = 0.061, Figure 2B and 0.21 ± 0.26% vs. 0.2 ± 0.094, p = 0.85, Figure 2C, respectively). However, the frequency of CD14−CD15+ gMDSCs increased (0.73 ± 0.88% vs. 0.38 ± 0.26%, p = 0.04, Figure 2D) comparing with that in HCs.
Figure 2. Expansion of MDSCs in patients with myasthenia gravis (MG) after immunotherapy. (A) Representative data plots of MDSCs, mMDSCs (HLA-DR−/lowCD33+CD11b+CD14+) and gMDSCs (HLA-DR−/lowCD33+CD11b+CD15+) from healthy controls (HC), patients with MG before (pre-treated) and after immunotherapy (post-treated). No difference of frequencies of MDSC (B) and mMDSCs (C) in PBMC between untreated patients with MG and HCs, but an increased frequency of gMDSC (D) in untreated patients with MG. The frequency of MDSCs (B), both mMDSCs (C) and gMDSCs (D) was significantly increased after immunotherapy. Horizontal lines and error bars represent mean ± SD, n = 30, *p < 0.05, **p < 0.01.
Then, we compared the frequency of MDSCs in patients with MG before and after immunotherapy. The frequency of MDSCs (1.5 ± 1.1% vs. 3.6 ± 3.1%, p = 0.0009, Figure 2B), both CD14+ mMDSCs (0.21 ± 0.26% vs.0.75 ± 1.1%, p = 0.017, Figure 2C) and CD15+ gMDSCs (0.73 ± 0.88% vs. 2 ± 1.8%, p = 0.0011, Figure 2D) was significantly increased after immunotherapy. The result demonstrated that MDSCs and their subsets are all expanded in patients with MG after immunotherapy.
As shown in Figure 3, we observed a negative correlation between the frequency of MDSC and that of Th1 (r = −0.67, p = 0.012, Figure 3A) or Th17 (r = −0.69, p = 0.009, Figure 3B) cells in patients with MG after immunotherapy but not in untreated patients (p > 0.05). We did not find correlations between the frequency of MDSC and that of Tregs or Bregs (P > 0.05).
Figure 3. Correlation of frequency of MDSC in PBMCs with that of Th17 and Th1 cells after immunotherapy. Frequency of MDSCs in PBMCs negatively correlated with that of Th1 cells (A) or Th17 cells (B) in PBMCs from 13 patients after immunotherapy (n = 13).
As shown in Figure 4A, we observed a significant increase in the frequency of CD3+ IFN-γ+ T cells in MDSC depleted PBMCs compared with undepleted PBMCs from both HCs (n = 5, p = 0.0099, Figure 4B) and from patients with MG after immunotherapy (n = 6, p = 0.033, Figure 4D). However, the depletion of MDSCs from the PBMCs in untreated patients with MG did not lead to an increase in the frequency of CD3+IFNγ+T cells in PBMCs (n = 6, p = 0.695, Figure 4C). These findings suggested an impaired suppressive function in untreated patients with MG, and this defect could be ameliorated after immunotherapy.
Figure 4. Effect of MDSCs on IFN-γ production in CD3+ T-cell from HCs, patients with MG before and after immunotherapy. (A) Representative flow cytometry plots of IFN-γ+CD3+T cells in MDSC depleted PBMCs compared with undepleted PBMCs from HCs, pre-treated, and post-treated patients. A significant increase in the frequency of CD3+ IFN-γ+ T cells in MDSC depleted PBMCs compared with undepleted PBMCs from both HCs (n = 5, B) and from patients with MG after immunotherapy (n = 6, D), but no significant change in untreated patients with MG (n = 6, C). Error bars represent mean ± SD. *p < 0.05, **p < 0.01.
Several factors including Arg-1, iNOS, IL-10, PD-1, PD-L1, IL-10, TGF-β, p47phox, gp91phox, and IDO have been implicated in mMDSCs-mediated immunosuppression (9–11, 15–17).
To investigate the potential mechanism by which MDSCs suppress T-cell responses in MG, we measured the intracellular mRNA levels of those factors by quantitative real-time PCR. The expression of TGF-β, iNOS, IL-10, PD-L1, PD-1, and p47phox was upregulated after immunotherapy compared to baseline (P < 0.05, Figure 5A) while that of IDO and gp91phox showed no significant difference.
Figure 5. Arginase-1 expression in patients with MG before and after immunotherapy. (A) The expression of MDSC-related molecules was measured by qPCR (n = 9). *p < 0.05, **p < 0.01. (B) Representative flow cytometry analysis of Arg-1 MFI in PBMC subpopulations from pre-treated and post-treated patients. Arg-1 MFI in MDSCs increased after immunotherapy (n = 6, C). *p < 0.05.
Since the increase of Arg-1 expression identified by qPCR after immunotherapy was most significant (p < 0.01, Figure 5A), we next evaluated Arg-1 expression in various cell types by intracellular staining in six patients before and after immunotherapy. As shown in Figure 5B, the intracellular MFI of Arg-1 expression in MDSC was significantly increased after immunotherapy (p = 0.031, Figure 5C) while that in T-cells, B-cells, NK-cells, and monocytes showed no statistical differences (p > 0.05, Figure S1). These results suggested that the increased expression of Arg-1 identified by qPCR was MDSCs derived.
Myeloid-derived suppressor cells, first identified as immunosuppressive cells, expand in tumor tissues and peripheral blood in patients with malignancies. Considering the role of MDSCs in promoting tumor progression by stimulating tumor cell invasion, angiogenesis, and premetastatic niche development (18), we hypothesized that MDSCs might also play a role in autoimmune diseases and the expansion of MDSCs might alleviate disease severity. However, current knowledge on the role of MDSCs in different human autoimmune diseases is limited and conflicting, possibly due to the instability of MDSCs in vivo. In this study, we investigated the phenotypic and functional alterations of MDSC in MG before and after immunotherapy.
Myeloid-derived suppressor cell accumulation has been described in various autoimmune diseases, including rheumatoid arthritis, systemic lupus erythematosus (SLE), inflammatory bowel disease, and multiple sclerosis (19). However, we only identified an increased frequency of gMDSCs but no change of frequencies of MDSCs from untreated patients with MG compared to that from HCs. In agreement with our study, a recent study in relapsing-remitting multiple sclerosis (RR-MS) patients during relapse revealed similar results (20). These results might indicate a different role of mMDSC and gMDSC in autoimmune diseases.
Since there was no quantitative difference between HCs and patients with MG, we suspected function alteration in MDSCs from untreated patients with MG. In a previous study, MDSCs-treated EAMG mice showed significantly suppressed AChR-specific Th1 response compared with control, as measured by IFN-γ production (21). Here, we also explored the effect of MDSCs on T-cell function by evaluating their inhibiting capacity of IFN-γ production in T-cells. Depleting MDSCs in PBMC led to a significant increase of IFN-γ production in T-cells from HCs, but not from untreated patients with MG, which indicated a defect in the suppressive function of MDSCs from untreated MG.
The dynamic quantitive and functional change of MDSCs in different stages of autoimmune diseases has been previously reported. The circulating frequency of CD14+HLA-DR−/low MDSCs was significantly increased in newly diagnosed patients with SLE and decreased after immunotherapy (22). RR-MS patients with stable disease stage showed decreased frequencies of CD14+HLA-DRlow monocytic MDSCs and CD15+CD11b+HLA-DRlow granulocytic MDSCs compared to those during relapse (23). However, our study revealed a significant expansion of MDSCs, including both mMDSCs and gMDSCs after immunotherapy, and the expansion was in line with clinical improvement. We found that seven patients showed no increase in mMDSC or gMDSCs after immunotherapy, and the MG-ADL of these patients was zero or one. There might be a difference between patients who began to improve and those who reached the status of minimal symptom expression. Also, the depletion of MDSCs in PBMC from patients with MG after immunotherapy led to a significant increase in IFN-γ production in T-cells, suggesting an improved inhibiting capacity of MDSCs after immunotherapy.
Oral prednisone and prednisolone are first-line immunotherapy drugs for MG owing to the rapid effect. All the patients were treated with prednisone with a maximum dosage ranging from 20–80 mg or combined with non-steroid immunosuppressants in our cohort. Numerous clinical and experimental data have demonstrated that steroids can enhance the expansion and immunosuppressive function of MDSCs through several mechanisms, including inhibition of HIF1α-dependent glycolysis (24), upregulating the glucocorticoid receptor signaling (25), elevated expression of S100A8/9 (20) and transcription factor Ets1 (26). Furthermore, non-steroid immunosuppressants, tacrolimus, could also modulate the expansion and function of MDSCs (27, 28). The expansion and improved immunosuppressive function of MDSCs in MG after immunotherapy might be due to the effect of steroids and other immunosuppressants. Further longitudinal studies with longer follow-up and in vitro experiments are needed to clarify this phenomenon.
Increasing studies have analyzed the association of MDSCs and proinflammatory and regulatory T-cells in human autoimmune diseases. Mainly from studies of SLE and RA, two perspectives exist. Some studies have shown that MDSCs are highly potent in promoting Th17 cell differentiation and disease progression. In contrast, some evidence indicates the opposite effects of MDSCs on decreasing Th17 and Th1 differentiation as well as inducing Foxp3+ regulatory T-cell differentiation, which is correlated with a relative improvement in the disease (29–33). MG is a B-cell-mediated, T-cell-dependent autoimmune disease (34). The secretion of IFN-γ and IL-17 in AChR peptide-stimulated CD4+ T cells demonstrated that Th1 and Th17 cells are involved in the pathogenesis of MG (35, 36). The association between MDSCs and proinflammatory T cell populations has not been investigated. We observed a negative correlation between the frequency of MDSC and that of Th1 or Th17 cells in patients with MG after immunotherapy but not in untreated patients. The results might arise from the heterogeneous of MDSCs before and after immunotherapy, which had different effects on T-cell proliferation and differentiation. MDSCs could also induce expansion of regulatory B-cells in a murine model of SLE and collagen-induced arthritis (37, 38), but in our study of patients with MG, we found no correlations between the frequency of MDSC and that of Bregs. Accurate elucidation still needs to be further explored in vitro experiments and animal models.
It is well known that the depletion of arginine through the upregulation of Arg-1 was one of the first and foremost T-cell suppressive mechanisms described in MDSCs. Arg-1 reduces the nutrient of T-lymphocytes and produces ornithine and polyamines (7, 39). Low levels of L-arginine reduce the zeta chain expression of the TCR-CD3 on T cells, dampen the cell cycling in the G0/G1 phase (inhibiting T cell proliferation) and decrease proinflammatory cytokine production (40, 41). Plasma concentration of Arg-1 was also negatively correlated to the frequency of Th17 cells in RA (32) and disease activity in Sjögren syndrome (42). However, MDSCs from SLE patients exhibited significantly elevated Arg-1 production and increased potential to promote Th17 differentiation in vitro in an Arg-1–dependent manner. These studies suggest that the role of Arg-1 in the pathogenesis of autoimmune diseases is not univocal. In MG, our study showed that the expression of MDSC-derived Arg-1 was significantly upregulated after immunotherapy, and the expression of Arg-1 in MDSCs was negatively correlated with disease severity measured by MG-ADL. Further studies are required to explore the subtle role of Arg-1 for T cell proliferation and Th17 differentiation in MG.
There are some limitations in our study: (1) The patients were followed only for 6–12 months, and the long-term phenotypic and quantitive changes of MDSCs are still unknown; (2) Lacking the direct study of a suppressive effect of MDSCs on T-cell proliferation (3) Further in vitro and in vivo studies are needed to elucidate the role of MDSC derived Arg-1 in T-cell suppression and differentiation; (4) Since difficulties obtaining sufficient blood samples from all patients with MG, a limited sample size for correlated analysis between frequency of MDSCs and Th17/Th1, semi-quantity analysis of Arg-1 expression and IFN-γ production in T cells with and without MDSCs.
Although no frequency difference compared to HCs, MDSCs significantly expanded, and the frequency of MDSCs negatively correlated to that of Th1 or Th17 cells after immunotherapy. MDSCs from untreated patients with MG showed an impaired suppression of IFN-γ production in T cells and improved immunosuppressive function was identified after immunotherapy. The MFI of Arg-1 in MDSCs also increased after immunotherapy. These findings suggested the functional difference in MDSCs before and after immunotherapy, and MDSCs might play a role in disease remission.
The raw data supporting the conclusions of this article will be made available by the authors, without undue reservation.
The studies involving human participants were reviewed and approved by the Institutional Review Board of Huashan Hospital, Fudan University. The patients/participants provided their written informed consent to participate in this study.
YaW designed the study, generated and analyzed the experimental data, and drafted the original manuscript. CY reviewed, edited, and approved the final version of the manuscript. CS, YiW, SL, and JL collected clinical data. CZ critically reviewed, edited, and approved the final version of the manuscript. GZ assisted in conceptualizing and designing the study, interpreted the experimental data, and drafted part of the original manuscript. JX conceptualized the study, interpreted the experimental and clinical data comprehensively, critically reviewed, edited, and approved the final version of the manuscript. All authors contributed to the article and approved the submitted version.
This research was supported by the National Key Research and Development Program of China (No.2017YFD0502301-2) and the National Natural Science Foundation of China (No. 81901279 and 81803542).
The authors declare that the research was conducted in the absence of any commercial or financial relationships that could be construed as a potential conflict of interest.
All claims expressed in this article are solely those of the authors and do not necessarily represent those of their affiliated organizations, or those of the publisher, the editors and the reviewers. Any product that may be evaluated in this article, or claim that may be made by its manufacturer, is not guaranteed or endorsed by the publisher.
We thank Ting Li from BD Medical Devices Company, Ltd. Shanghai, China for technical support for the FACS sorter application.
The Supplementary Material for this article can be found online at: https://www.frontiersin.org/articles/10.3389/fneur.2022.902384/full#supplementary-material
AChR, acetylcholine receptor; Arg-1, Arginase-1; gMDSC, granulocytic MDSC; HC, healthy controls; IFN-γ, interferon γ; IL, interleukin; IDO, indoleamine-2 3-dioxynase; iNOS, inducible nitric oxide synthase; mMDSC, monocytic MDSC; MDSC, myeloid-derived suppressor cell; MG, myasthenia gravis; PBMC, peripheral blood mononuclear cell; PE, phycoerythrin; PerCP, peridinin chlorophyll protein; PMA, phorbol 12-myristate 13-acetate; ROS, reactive oxygen species; TGF-β, transforming growth factor-β.
1. Gilhus NE, Tzartos S, Evoli A, Palace J, Burns TM, Verschuuren J. Myasthenia gravis. Nat Rev Dis Primers. (2019) 5:30. doi: 10.1038/s41572-019-0079-y
2. Gilhus NE, Verschuuren JJ. Myasthenia gravis: subgroup classification and therapeutic strategies. Lancet Neurol. (2015) 14:1023–36. doi: 10.1016/S1474-4422(15)00145-3
3. Papp G, Boros P, Nakken B, Szodoray P, Zeher M. Regulatory Immune cells and functions in autoimmunity and transplantation immunology. Autoimmun Rev. (2017) 16:435–44. doi: 10.1016/j.autrev.2017.03.011
4. Kohler S, Keil TOP, Hoffmann S, Swierzy M, Ismail M, Ruckert JC, et al. Cd4(+) Foxp3(+) T regulatory cell subsets in myasthenia gravis patients. Clin Immunol. (2017) 179:40–6. doi: 10.1016/j.clim.2017.03.003
5. Thiruppathi M, Rowin J, Ganesh B, Sheng JR, Prabhakar BS, Meriggioli MN. Impaired regulatory function in circulating Cd4(+)Cd25(High)Cd127(Low/-) T-cells in patients with myasthenia gravis. Clin Immunol. (2012) 145:209–23. doi: 10.1016/j.clim.2012.09.012
6. Sheng JR, Rezania K, Soliven B. Impaired regulatory B-cells in myasthenia gravis. J Neuroimmunol. (2016) 297:38–45. doi: 10.1016/j.jneuroim.2016.05.004
7. Gabrilovich DI, Nagaraj S. Myeloid-derived suppressor cells as regulators of the immune system. Nat Rev Immunol. (2009) 9:162–74. doi: 10.1038/nri2506
8. Bronte V, Brandau S, Chen SH, Colombo MP, Frey AB, Greten TF, et al. Recommendations for myeloid-derived suppressor cell nomenclature and characterization standards. Nat Commun. (2016) 7:12150. doi: 10.1038/ncomms12150
9. Huang A, Zhang B, Yan W, Wang B, Wei H, Zhang F, et al. Myeloid-derived suppressor cells regulate immune response in patients with chronic hepatitis B virus infection through Pd-1-induced Il-10. J Immunol. (2014) 193:5461–9. doi: 10.4049/jimmunol.1400849
10. Nam S, Lee A, Lim J, Lim JS. Analysis of the expression and regulation of Pd-1 protein on the surface of Myeloid-derived suppressor cells (Mdscs). Biomol Ther. (2019) 27:63–70. doi: 10.4062/biomolther.2018.201
11. Yang F, Yu X, Zhou C, Mao R, Zhu M, Zhu H, et al. Hepatitis B E antigen induces the expansion of monocytic myeloid-derived suppressor cells to dampen T-cell function in chronic hepatitis B virus infection. PLoS Pathog. (2019) 15:e1007690. doi: 10.1371/journal.ppat.1007690
12. Boros P, Ochando J, Zeher M. Myeloid derived suppressor cells and autoimmunity. Hum Immunol. (2016) 77:631–6. doi: 10.1016/j.humimm.2016.05.024
13. Barohn RJ, McIntire D, Herbelin L, Wolfe GI, Nations S, Bryan WW. reliability testing of the quantitative myasthenia gravis score. Ann N Y Acad Sci. (1998) 841:769–72. doi: 10.1111/j.1749-6632.1998.tb11015.x
14. Wolfe GI, Herbelin L, Nations SP, Foster B, Bryan WW, Barohn RJ. Myasthenia gravis activities of daily living profile. Neurology. (1999) 52:1487–9. doi: 10.1212/WNL.52.7.1487
15. Jitschin R, Braun M, Buttner M, Dettmer-Wilde K, Bricks J, Berger J, et al. Cll-cells induce idohi Cd14+Hla-Drlo myeloid-derived suppressor cells that inhibit T-cell Responses and Promote Tregs. Blood. (2014) 124:750–60. doi: 10.1182/blood-2013-12-546416
16. Corzo CA, Cotter MJ, Cheng P, Cheng F, Kusmartsev S, Sotomayor E, et al. Mechanism regulating reactive oxygen species in tumor-induced myeloid-derived suppressor cells. J Immunol. (2009) 182:5693–701. doi: 10.4049/jimmunol.0900092
17. Wu Y, Chang YM, Lawson BS, Galban EM, Mittelman NS, Benedicenti L, et al. Myeloid-derived suppressor cell and regulatory T-cell frequencies in canine myasthenia gravis: a pilot study. Vet J. (2021) 267:105581. doi: 10.1016/j.tvjl.2020.105581
18. Ortiz ML, Lu L, Ramachandran I, Gabrilovich DI. Myeloid-derived suppressor cells in the development of lung cancer. Cancer Immunol Res. (2014) 2:50–8. doi: 10.1158/2326-6066.CIR-13-0129
19. Veglia F, Sanseviero E, Gabrilovich DI. Myeloid-derived suppressor cells in the era of increasing myeloid cell diversity. Nat Rev Immunol. (2021). doi: 10.1038/s41577-020-00490-y
20. Wang Z, Zheng G, Li G, Wang M, Ma Z, Li H, et al. Methylprednisolone alleviates multiple sclerosis by expanding myeloid-derived suppressor cells via glucocorticoid receptor beta and S100a8/9 up-regulation. J Cell Mol Med. (2020) 24:13703–14. doi: 10.1111/jcmm.15928
21. Li Y, Tu Z, Qian S, Fung JJ, Markowitz SD, Kusner LL, et al. Myeloid-derived suppressor cells as a potential therapy for experimental autoimmune myasthenia gravis. J Immunol. (2014) 193:2127–34. doi: 10.4049/jimmunol.1400857
22. Florez-Pollack S, Tseng LC, Kobayashi M, Hosler GA, Ariizumi K, Chong BF. Expansion of Myeloid-derived suppressor cells in the peripheral blood and lesional skin of cutaneous lupus patients. J Invest Dermatol. (2019) 139:478–81. doi: 10.1016/j.jid.2018.08.023
23. Iacobaeus E, Douagi I, Jitschin R, Marcusson-Stahl M, Andren AT, Gavin C, et al. Phenotypic and functional alterations of myeloid-derived suppressor cells during the disease course of multiple sclerosis. Immunol Cell Biol. (2018) 96:820–30. doi: 10.1111/imcb.12042
24. Lu Y, Liu H, Bi Y, Yang H, Li Y, Wang J, et al. Glucocorticoid receptor promotes the function of myeloid-derived suppressor cells by suppressing Hif1alpha-dependent glycolysis. cell mol immunol. (2018) 15:618–29. doi: 10.1038/cmi.2017.5
25. Zhao Y, Shen XF, Cao K, Ding J, Kang X, Guan WX, et al. Dexamethasone-induced myeloid-derived suppressor cells prolong allo cardiac graft survival through Inos- and glucocorticoid receptor-dependent mechanism. Front Immunol. (2018) 9:282. doi: 10.3389/fimmu.2018.00282
26. Hou Y, Feng Q, Xu M, Li GS, Liu XN, Sheng Z, et al. High-dose dexamethasone corrects impaired myeloid-derived suppressor cell function via Ets1 in immune thrombocytopenia. Blood. (2016) 127:1587–97. doi: 10.1182/blood-2015-10-674531
27. Heigl T, Singh A, Saez-Gimenez B, Kaes J, Van Herck A, Sacreas A, et al. Myeloid-derived suppressor cells in lung transplantation. Front Immunol. (2019) 10:900. doi: 10.3389/fimmu.2019.00900
28. Iglesias-Escudero M, Sansegundo-Arribas D, Riquelme P, Merino-Fernandez D, Guiral-Foz S, Perez C, et al. Myeloid-derived suppressor cells in kidney transplant recipients and the effect of maintenance immunotherapy. Front Immunol. (2020) 11:643. doi: 10.3389/fimmu.2020.00643
29. Wang Z, Zhu F, Wang J, Tao Q, Xu X, Wang H, et al. Increased Cd14(+)Hla-Dr(-/Low) Myeloid-derived suppressor cells correlate with disease severity in systemic lupus erythematosus patients in an Inos-Dependent Manner. Front Immunol. (2019) 10:1202. doi: 10.3389/fimmu.2019.01202
30. Rajabinejad M, Salari F, Gorgin Karaji A, Rezaiemanesh A. The role of myeloid-derived suppressor cells in the pathogenesis of rheumatoid arthritis; anti- or pro-inflammatory cells? Artif Cells Nanomed Biotechnol. (2019) 47:4149–58. doi: 10.1080/21691401.2019.1687504
31. Zhang H, Wang S, Huang Y, Wang H, Zhao J, Gaskin F, et al. Myeloid-derived suppressor cells are proinflammatory and regulate collagen-induced arthritis through manipulating Th17 Cell differentiation. Clin Immunol. (2015) 157:175–86. doi: 10.1016/j.clim.2015.02.001
32. Jiao Z, Hua S, Wang W, Wang H, Gao J, Wang X. Increased circulating myeloid-derived suppressor cells correlated negatively with Th17 cells in patients with rheumatoid arthritis. Scand J Rheumatol. (2013) 42:85–90. doi: 10.3109/03009742.2012.716450
33. Hoechst B, Ormandy LA, Ballmaier M, Lehner F, Kruger C, Manns MP, et al. A new population of myeloid-derived suppressor cells in hepatocellular carcinoma patients induces Cd4(+)Cd25(+)Foxp3(+) T Cells. Gastroenterology. (2008) 135:234–43. doi: 10.1053/j.gastro.2008.03.020
34. Conti-Fine BM, Milani M, Kaminski HJ. Myasthenia gravis: past, present, and future. J Clin Invest. (2006) 116:2843–54. doi: 10.1172/JCI29894
35. Cao Y, Amezquita RA, Kleinstein SH, Stathopoulos P, Nowak RJ, O'Connor KC. Autoreactive T Cells from Patients with Myasthenia Gravis Are Characterized by Elevated Il-17, Ifn-Gamma, and Gm-Csf and Diminished Il-10 Production. J Immunol. (2016) 196:2075–84. doi: 10.4049/jimmunol.1501339
36. Cebi M, Durmus H, Aysal F, Ozkan B, Gul GE, Cakar A, et al. Cd4(+) T-cells of myasthenia gravis patients are characterized by increased Il-21, Il-4, and Il-17a productions and higher presence of Pd-1 and Icos. Front Immunol. (2020) 11:809. doi: 10.3389/fimmu.2020.00809
37. Wu X, Zhu D, Tian J, Tang X, Guo H, Ma J, et al. Granulocytic myeloid-derived suppressor cell exosomal prostaglandin E2 Ameliorates Collagen-Induced Arthritis by Enhancing IL-10+ B Cells. Front Immunol. (2020) 11:588500. doi: 10.3389/fimmu.2020.588500
38. Park MJ, Lee SH, Kim EK, Lee EJ, Park SH, Kwok SK, et al. Myeloid-derived suppressor cells induce the expansion of regulatory B-cells and ameliorate autoimmunity in the sanroque mouse model of systemic lupus erythematosus. Arthritis Rheumatol. (2016) 68:2717–27. doi: 10.1002/art.39767
39. Sica A, Bronte V. Altered macrophage differentiation and immune dysfunction in tumor development. J Clin Invest. (2007) 117:1155–66. doi: 10.1172/JCI31422
40. Rodriguez PC, Quiceno DG, Ochoa AC. L-arginine availability regulates T-lymphocyte cell-cycle progression. Blood. (2007) 109:1568–73. doi: 10.1182/blood-2006-06-031856
41. Liu CY, Wang YM, Wang CL, Feng PH, Ko HW, Liu YH, et al. Population alterations of L-arginase- and inducible nitric oxide synthase-expressed Cd11b+/Cd14(-)/Cd15+/Cd33+ myeloid-derived suppressor cells and Cd8+ t lymphocytes in patients with advanced-stage non-small cell lung cancer. J Cancer Res Clin Oncol. (2010) 136:35–45. doi: 10.1007/s00432-009-0634-0
Keywords: myeloid derived suppressor cell (MDSC), myasthenia gravis (MG), Arginase-1, interferon γ (IFN- γ), immunoregulatory
Citation: Wang Y, Yan C, Su C, Wang Y, Luo S, Lu J, Zhao C, Zhao G and Xi J (2022) Increased Frequency of Myeloid-Derived Suppressor Cells in Myasthenia Gravis After Immunotherapy. Front. Neurol. 13:902384. doi: 10.3389/fneur.2022.902384
Received: 23 March 2022; Accepted: 03 May 2022;
Published: 29 June 2022.
Edited by:
Jens Schmidt, University Medical Center Göttingen, GermanyReviewed by:
Robert Adam Harris, Karolinska Institutet (KI), SwedenCopyright © 2022 Wang, Yan, Su, Wang, Luo, Lu, Zhao, Zhao and Xi. This is an open-access article distributed under the terms of the Creative Commons Attribution License (CC BY). The use, distribution or reproduction in other forums is permitted, provided the original author(s) and the copyright owner(s) are credited and that the original publication in this journal is cited, in accordance with accepted academic practice. No use, distribution or reproduction is permitted which does not comply with these terms.
*Correspondence: Gan Zhao, emhhb2dAZnVkYW4uZWR1LmNu; Jianying Xi, eGlqaWFueWluZ0BmdWRhbi5lZHUuY24=
Disclaimer: All claims expressed in this article are solely those of the authors and do not necessarily represent those of their affiliated organizations, or those of the publisher, the editors and the reviewers. Any product that may be evaluated in this article or claim that may be made by its manufacturer is not guaranteed or endorsed by the publisher.
Research integrity at Frontiers
Learn more about the work of our research integrity team to safeguard the quality of each article we publish.