- 1Convergent Medical Technologies, Inc., Oyster Bay, NY, United States
- 2Department of Biomedical Engineering, The City College of New York, New York, NY, United States
- 3Department of Neurology, NYU Grossman School of Medicine, New York, NY, United States
- 4Division of Gastroenterology and Hepatology, University of Michigan School of Medicine, Ann Arbor, MI, United States
- 5NeuronOff, Inc., Cleveland, OH, United States
- 6Division of Cardiology, Duke Clinical Research Institute, Duke University, Durham, NC, United States
- 7Neurovations, Napa, CA, United States
- 8Cala Health, Burlingame, CA, United States
- 9Departments of Surgery and Science Education, Zucker School of Medicine at Hofstra/Northwell, Institute of Bioelectronic Medicine, Feinstein Institutes for Medical Research, Manhasset, NY, United States
- 10Spark Biomedical, Inc., Dallas, TX, United States
- 11Pacific Pain Treatment Center, Napa, CA, United States
- 12ElectroCore, Inc., Basking Ridge, NJ, United States
- 13National Spine and Pain, ElectroCore, Inc., Jacksonville, FL, United States
- 14Department of Neurology, Ghent University Hospital, Ghent, Belgium
Since the outbreak of the COVID-19 pandemic, races across academia and industry have been initiated to identify and develop disease modifying or preventative therapeutic strategies has been initiated. The primary focus has been on pharmacological treatment of the immune and respiratory system and the development of a vaccine. The hyperinflammatory state (“cytokine storm”) observed in many cases of COVID-19 indicates a prognostically negative disease progression that may lead to respiratory distress, multiple organ failure, shock, and death. Many critically ill patients continue to be at risk for significant, long-lasting morbidity or mortality. The human immune and respiratory systems are heavily regulated by the central nervous system, and intervention in the signaling of these neural pathways may permit targeted therapeutic control of excessive inflammation and pulmonary bronchoconstriction. Several technologies, both invasive and non-invasive, are available and approved for clinical use, but have not been extensively studied in treatment of the cytokine storm in COVID-19 patients. This manuscript provides an overview of the role of the nervous system in inflammation and respiration, the current understanding of neuromodulatory techniques from preclinical and clinical studies and provides a rationale for testing non-invasive neuromodulation to modulate acute systemic inflammation and respiratory dysfunction caused by SARS-CoV-2 and potentially other pathogens. The authors of this manuscript have co-founded the International Consortium on Neuromodulation for COVID-19 to advocate for and support studies of these technologies in the current coronavirus pandemic.
Introduction
Preventative strategies to reduce infections of the coronavirus, SARS-CoV-2, including wearing masks (N95 most effective) and face shields, avoiding prolonged exposure to infected individuals, avoiding confined indoor spaces, quarantines, and vaccines and boosters, have proven successful. While disease-modifying therapeutic strategies for coronavirus-induced disease-2019 (COVID-19), including the antiviral remdesivir (1), are emerging, the contagiousness of the virus and the morbidity and mortality associated with COVID-19 continue to place an urgent demand on the development of new therapies. Presently worldwide, nearly 450 million persons have been infected worldwide, 6 million of whom have succumbed to the disease (https://coronavirus.jhu.edu/map.html). Severe cases of COIVD-19 are often associated with a “cytokine storm,” which may cause dysfunction of the lungs and other organs. Studies investigating pharmacological therapies targeting the immune and respiratory systems are ongoing.
The human immune and respiratory systems are regulated by the central nervous system (CNS), and therapeutic targeting of these neural pathways is potentially one approach to regulate important aspects of the pathologic sequelae of COVID-19. Neuromodulation directly targets the nervous system through the application of electric or magnetic fields to neuronal tissue, including peripheral nerve fibers and directly to the brain. Neuromodulation may therefore provide alternative therapeutic strategies for the neurobiological systems involved in the pathophysiology of SARS-CoV-2 infections.
This review provides an overview of the role of the nervous system in immune and respiratory control, current understanding of how neuromodulatory techniques may be effective in immune or pulmonary disorders, and provides a future perspective of its role in the treatment of COVID-19. The authors of this review formed the International Consortium on Neuromodulation for COVID-19 (www.covidneuromod.org) in April, 2020, in response to the pandemic with the understanding that neuromodulation, particularly vagus nerve stimulation (VNS) or sacral nerve modulation (SNM), may be able to block infection-induced inflammation through the cholinergic antiinflammatory pathway (CAP). Now including 30 members on three continents, this group was formed to advocate for and support the scientific study of neuromodulation technologies for treatment of excessive inflammation and pulmonary dysfunction in this and future pandemics when appropriate. The authors provide recommendations on clinical trial endpoints, as well as recommended publication guidelines in an effort to standardize technology characterizations.
The Hyperinflammatory State in COVID-19
In up to 30% of mortality caused by SARS-CoV-2, a severe systemic inflammatory response known as a “cytokine storm” is implicated in multi-system organ failure (2, 3). These cases are characterized by high concentrations of circulating proinflammatory cytokines, including TNF, IL-6, IL-7; the inflammatory chemokines CCL2, CCL3 and CXCL10 (4); and acute-phase proteins including C-reactive protein and ferritin are also associated with disease severity (4, 5). Clinically, the most typical phenotype of severe COVID-19 is acute respiratory distress syndrome (ARDS), which affects 3.4% of infected patients overall and 15.6–17.0% of severely infected patients (6). The COVID-19 hyperinflammatory response may induce disseminated intravascular coagulation through both endothelial cell activation and impairment of endogenous anticoagulant pathways (7–12). This disease pathophysiology may pose specific problems for individuals with preexisting cardiopulmonary conditions, including hypertension and chronic obstructive pulmonary disease.
Role of the Nervous System in Immune and Respiratory Control
The mammalian nervous and immune systems evolved to maintain homeostasis and protect the host against tissue injury and infection (13). Autonomic nerves monitor infection and inflammation and form homeostatic reflexes to regulate host responses (14, 15). An important neural conduit for monitoring and regulating immune responses is the vagus nerve (VN), the body's longest cranial nerve. Peripheral vagal fibers sense local cytokines and damage- and pathogen-associated molecular patterns (DAMPs, PAMPs), which activate neural regulatory reflexes (16, 17). The brain can respond to active inflammation via glucocorticoid release from the hypothalamic-pituitary-adrenal (HPA) axis, or through activation of the CAP (18). The CAP comprises efferent VN fibers innervating the spleen via the abdominal celiac ganglion and splenic nerve. The role of sympathetic nerves in regulating inflammation and pulmonary function are reviewed elsewhere (15, 19–21).
Electrical VNS was first shown to protect against excessive inflammation in 2000, when Borovikova et al. reported that VNS reduces systemic proinflammatory cytokine production and maintains blood pressure in a rodent model of endotoxic shock (22). Electrically or pharmacologically stimulating the CAP protects against excessive systemic inflammation, maintains tissue perfusion, and preserves vital organ function in models of arthritis, burns, colitis, hemorrhagic shock, polymicrobial intraabdominal sepsis, and stroke (23–34). Importantly, disruption of CAP signaling delays resolution of inflammation and bacterial clearance in experimental peritonitis (35). Specific CAP components also play key roles in regulation of circulation, microvascular contractility, and viral infection clearance in experimental models (36, 37).
Molecular and functional mapping of the CAP have revealed that VNS inhibits release of pro-inflammatory mediators via acetylcholine-releasing T lymphocytes expressing choline acetyltransferase (ChAT+) (38). Local acetylcholine secretion in spleen inactivates cytokine-producing macrophages through surface α7 nicotinic acetylcholine receptor subunits (37). Pro-inflammatory cytokine production in spleen contributes significantly to overall systemic inflammation (39). Elimination of these cellular or molecular components via genetic deletion or surgical disruption abolishes CAP activity and results in significantly elevated serum cytokine levels (40).
In addition to providing neural regulation of inflammation, the VN also provides the dominant autonomic innervation of the airways, carrying fibers that are responsible for both bronchoconstriction and relaxation through distinct sets of pulmonary nerve branches (41–43). Low-intensity VNS relaxes airway smooth muscle in animal models of bronchoconstriction by activating the HPA axis to release catecholamines from the adrenal cortex (41, 44, 45). These in turn bind to β-receptors on bronchial smooth muscle causing relaxation. Measured concentrations of epinephrine and norepinephrine were sufficient to relax smooth muscle, but insufficient to cause significant cardiac effects (46, 47).
The sacral nerve (SN) is part of the parasympathetic nervous system that also holds therapeutic potential for pathological inflammatory processes. Both afferent and efferent SN fibers innervate the pelvic organs including colon, rectum, urinary bladder and genital organs. Sacral nerve modulation (SNM) has been used to treat diseases of the pelvic organs, including pain, urinary retention, overactive bladder and fecal incontinence (48, 49). The mechanisms of action of SNM are not completely understood. Limited findings suggest that SNM ameliorates overactive bladder by blocking C fiber activities or overactivity (50). In treating urinary retention, SNM seems to stimulate relaxation of pelvic floor muscles and the urethra, facilitating micturition (51, 52). The mechanism involved in SNM-induced improvement in fecal incontinence is also poorly understood, with most research findings suggesting that SNM stimulates afferent fibers from the rectum, pelvic floor and anal sphincter, resulting in reduced activation of C fibers during rectal filling and inhibiting inputs from the rectum to the pontine center (53). SNM in patients with fecal incontinence may activate somatic afferent fibers to inhibit colonic motility and enhance internal anal sphincter pressure (54).
Recently, SNM has been shown to reduce intestinal inflammation in rodent models of inflammatory bowel diseases, suppress a number of proinflammatory cytokines including TNF, and enhance anti-inflammatory cytokines such as IL-10 in both intestinal tissues and in circulation (55, 56). The anti-inflammatory effect of SNM on the colon is mediated via the spinal afferent and vagal efferent pathways evidenced by the following factors (57): (1) SNM activates neurons in the nucleus tractus solitaries (57, 58); (2) SNM increases vagal efferent activity as assessed by the spectral analysis of heart rate variability and plasma level of pancreatic polypeptide (55); (3) bilateral subdiaphragmatic vagotomy almost completely abolishes the anti-inflammatory effect of SNM on the colon (57); and (4) SNM induces the release of acetylcholine in the colon (55). These findings suggest that SNM shares a similar vagal efferent pathway as VNS. Indeed, a comparative study has shown that the anti-inflammatory effect of SNM was similar to that of VNS and the combination of SNM and VNS did not show a synergistic effect in comparison with SNM or VNS alone (59, 60).
Together, these findings suggest that VNS or SNM enhance vagal efferent activity and have systemic anti-inflammatory effects. Several VNS technologies are being investigated, some for use in COVID-19, as summarized below.
Vagus Nerve Modulation in Clinical Practice and Research
Electrical signaling between the CNS and individual organ systems is essential to organ function. Homeostasis is continually monitored and regulated through a bi-directional feedback loop that senses the physiological status of organ function, central nervous system processing of the sensory information, and appropriate feed-back to the organs. Therapeutic neuromodulation aims to restore a diseased organ system by interfering with or augmenting its neural control mechanisms via targeted application of external energy sources, typically electrical or magnetic. Neuromodulation technologies have been developed to treat neuropsychiatric disorders and conditions where the nervous system is known to play an important role, including epilepsy, depression and pain (61, 62). Of note, VNS utilizing a surgically implanted electrical pulse generator is an established clinical therapy for the treatment of medically refractory epilepsy and depression. Since 1988, more than 100,000 patients have received this FDA-approved and CE-marked therapy, and long-term follow-up studies demonstrate overall safety of the approach (63).
Functional mapping of the CAP has provided a foundation for clinical trials to evaluate the efficacy of VNS to treat inflammatory diseases. Several studies, albeit with a limited number of patients included, show encouraging data on use of electrical VNS for the treatment of chronic inflammation, e.g., rheumatoid arthritis and Crohn's disease (64–66). VNS significantly reduced whole blood TNF, IL-6, and IL-1β concentrations, and decreased scores on a validated disease activity composite score (DAS28-CRP score) (65). Likewise, non-invasive cervical VNS was tested in two clinical trials for treating acute asthma patients presenting to the emergency department who were unresponsive to 1 h of standard of care therapy (67–69). In both studies, VNS significantly improved two measures of airway patency, FEV1 and perceived work of breathing, after 15, 30 and 60 min (compared with a matched control group), with no reported serious adverse events. These data suggest a possible, novel treatment for COVID-19 patients suffering from acute respiratory dysfunction. However, care must be exercised in choosing stimulation parameters because high intensity cervical VNS, such as is used to treat epilepsy and depression, is capable of inducing bronchoconstriction, which is reversed upon cessation of stimulation (70).
Neuromodulation Technologies for COVID-19
In light of the available mechanistic experimental data on the capacity of neuromodulation techniques to attenuate excessive acute and chronic inflammation, and induce bronchodilation; the clinical safety record of electrical VNS; and the encouraging reports on reduced inflammation with chronic implantation of an electrical vagus nerve stimulator in clinical trials, it is reasonable to consider whether neuromodulation devices may be useful tools to reduce the morbidity and mortality of COVID-19 patients (71–79). A significant challenge for applications in acute-onset excessive inflammation is the delivery method of VNS, as it is currently impractical to implant highly symptomatic COVID-19 patients with an active device that is only needed for acute treatment. However, less invasive neuromodulation techniques, either percutaneous or transcutaneous, may be utilized (Tables 1–4). These methods may be technically suitable for acute use, but their clinical feasibility and efficacy in COVID-19 patients are just beginning to be evaluated. Results from two recent COVID-19 studies have been published, results are pending from several ongoing clinical trials, and a number have been discontinued (discussed below).
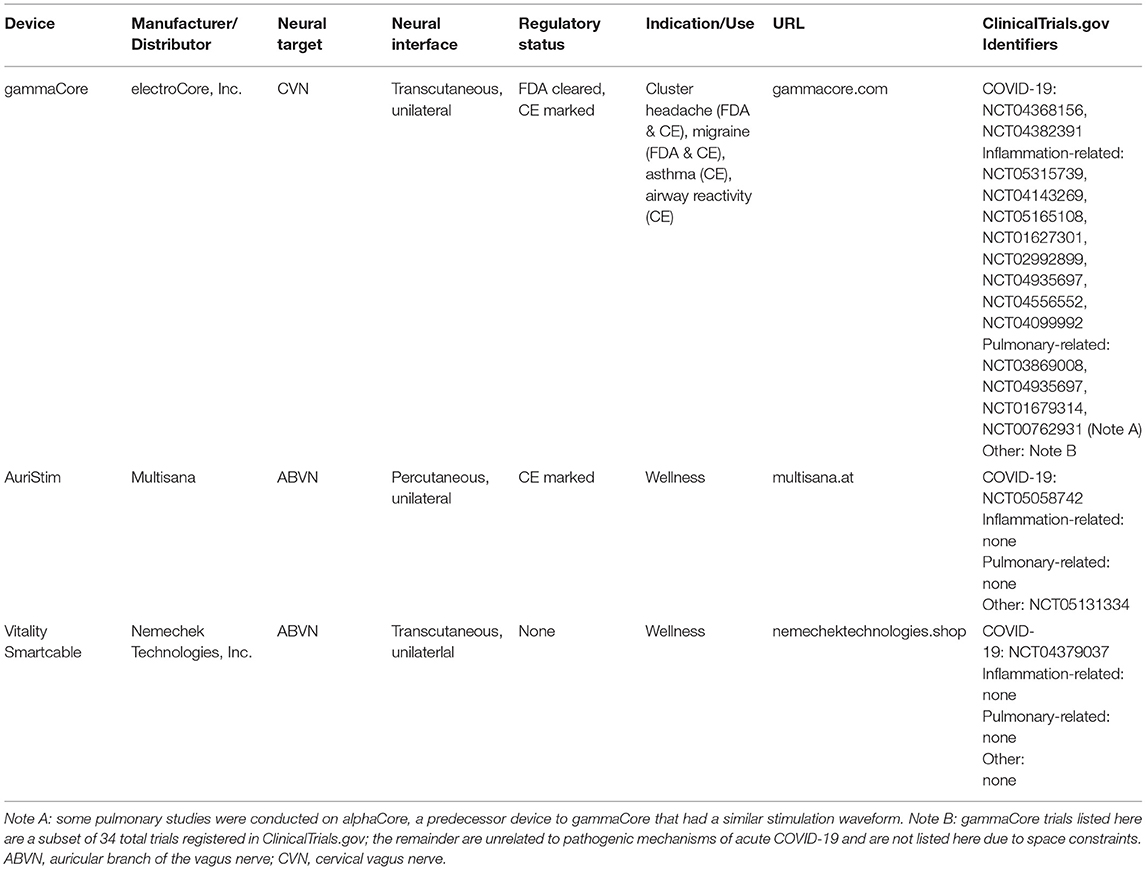
Table 1. Examples of commercially available devices that are currently being tested or have recently been tested in COVID-19 trials.
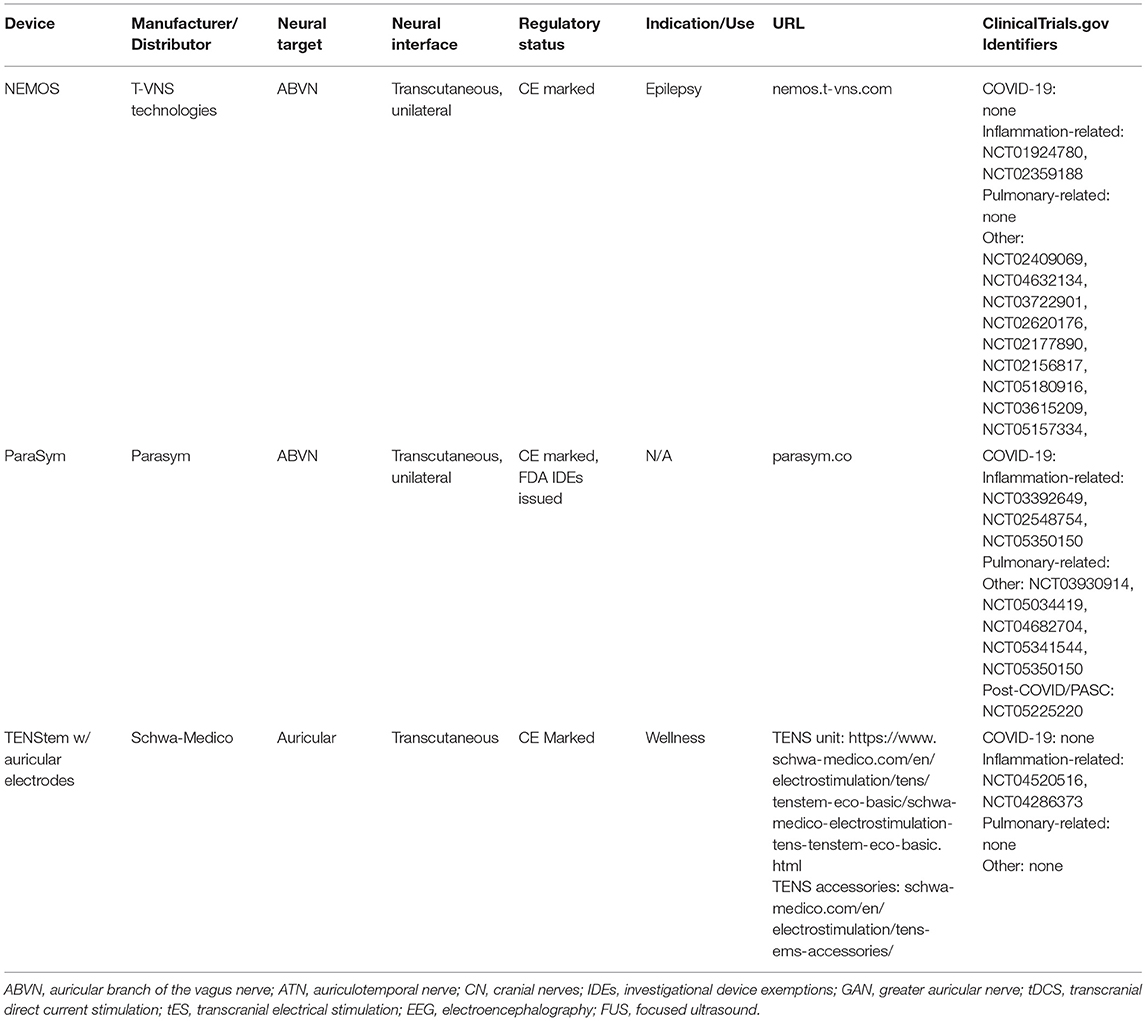
Table 2. Examples of commercially available devices that are currently being tested in non-COVID-19 trials, but are assessing endpoints related to pathogenic mechanisms of COVID-19.
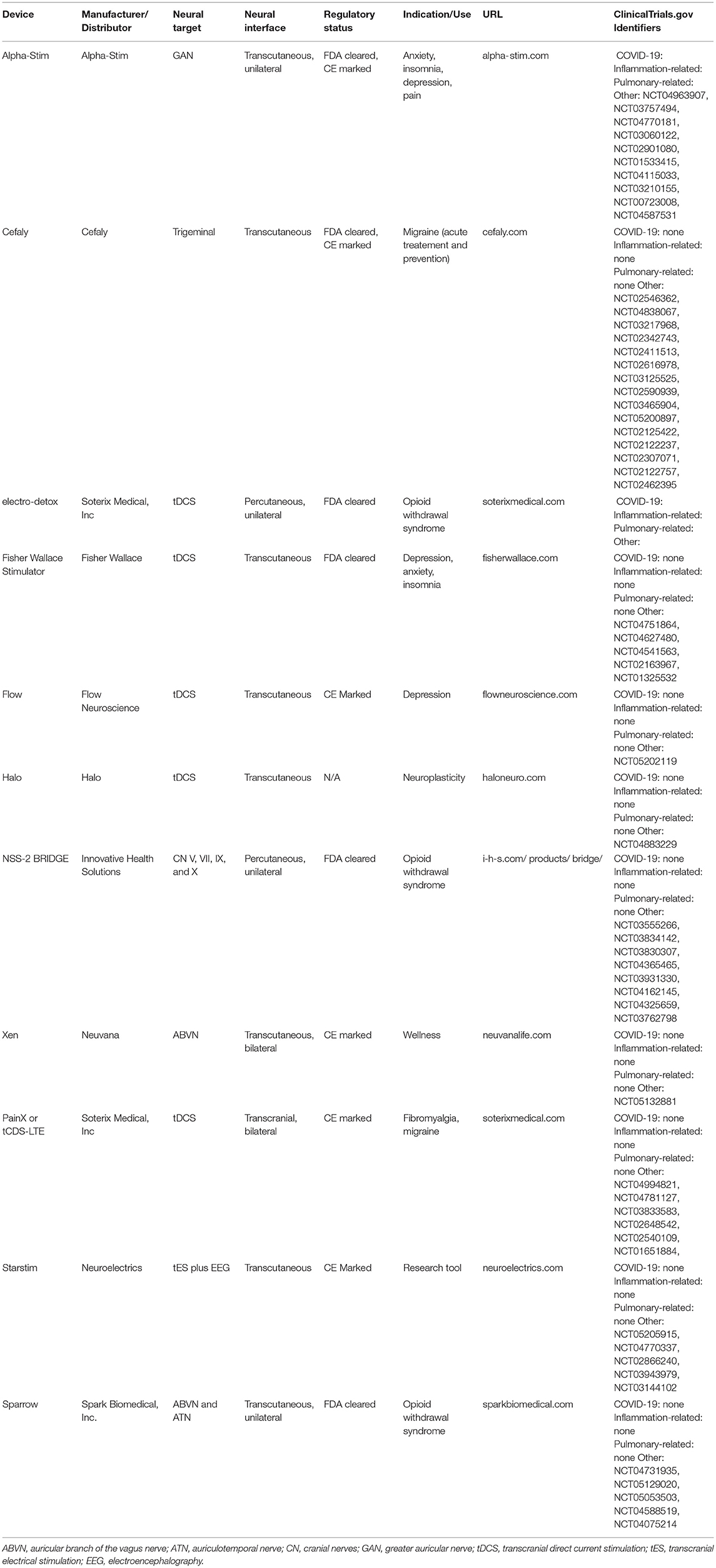
Table 3. Examples of commercially available devices that are currently being tested in trials unrelated to COVID-19, but which have neural mechanisms of action that could be related to the pathological sequelae of COVID-19.
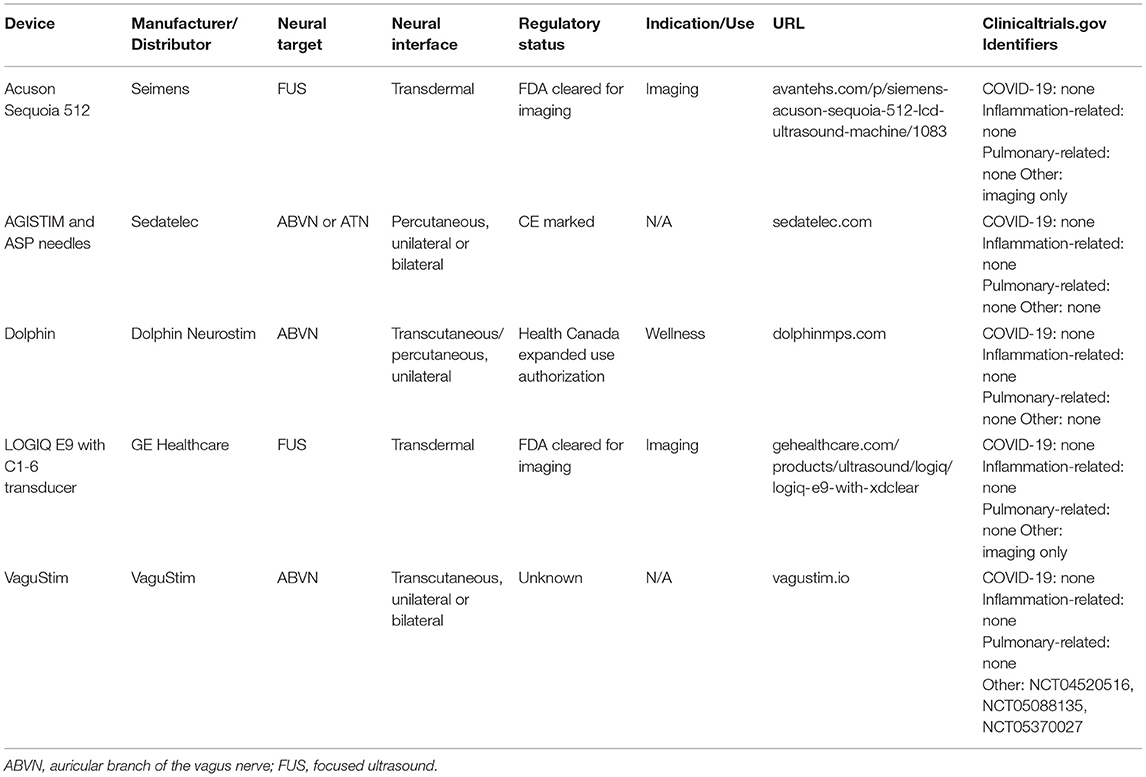
Table 4. Examples of devices with neural mechanisms that may be related to modulating the pathological sequelae of COVID-19, but which have not yet been assessed for relevant endpoints.
Transcutaneous Cervical VNS
gammaCore (electroCore, Inc., Rockaway, NJ USA) is a handheld, transcutaneous cervical vagus nerve stimulator that has been FDA approved and CE marked for cluster headache and migraine. Functional magnetic resonance imaging has shown that gammaCore specifically modulates various brain structures, including the locus coeruleus (LC) and the NTS, and that these activation patters are similar between invasive VNS, non-invasive cervical VNS, and transcutaneous auricular VNS (described further below) (80–82). In off-label studies, gammaCore reduced expression of interleukin [IL]-1β, IL-6, IL-8, tumor necrosis factor [TNF]), macrophage inflammatory protein [MIP]-1α, and monocyte chemoattractant protein [MCP]-1, and increased cardiac vagal tone (83–88). gammaCore also improved lung function in treatment-refractory emergent asthma (69). Together, these observations suggest that gammaCore may have therapeutic utility in COVID-19. Indeed, the FDA issued an Emergency Use Authorization for asthma patients experiencing exacerbation of symptoms when infected with the novel coronavirus (89).
Transcutaneous Auricular VNS
Recently, several studies examined the function of the auricular branch of the vagus nerve (ABVN) as a potential non-invasive method to activate the NTS and associated structures. Functional magnetic resonance imaging has shown specific modulation of various brain structures following transcutaneous auricular neurostimulation (tAN), including the NTS and nucleus spinalis of the trigeminal nerve (90, 91). Thus, the ABVN serves as a non-invasive access point to the CNS to deliver information, as similar to cervical VNS. Stimulation of the ABVN downregulated expression of IL-6, IL-1β, IP-10, MIP-1α, TNF, and C-reactive protein (CRP); and increased expression of the anti-inflammatory cytokine IL-10 (92–94). These stimulation paradigms also reduced incidence of pneumonia and hospital length of stay following lung lobectomy; reduced atrial fibrillation burden; and improved disease activity scores (DAS28-CRP) in rheumatoid arthritis (92–94). Electrical stimulation parameters that have typically shown results have focused on the left ear, using stimulation pulses of ~200 ms, delivered at 20 Hz, with current at or somewhat below a level that causes discomfort; and treatment paradigms have delivered as little as 1 h of stimulation daily for 6 months or less (93). In March of 2021, Health Canada issued an expanded use authorization to Dolphin Neurostim for the acute treatment of adult patients with known or suspected COVID-19 who are experiencing exacerbation of asthma-related dyspnea and reduced airflow (95).
Electrical and Magnetic Transcranial Approaches
In addition to modulation of neural pathways that directly modify end-organ function, top-down neuromodulation technologies target central neural structures to achieve similar effects. Low-intensity transcranial electrical stimulation (tES) approaches include transcranial Direct Current Stimulation (tDCS) (96) and transcranial Alternating Current Stimulation (tACS) (97). Transcranial Magnetic Stimulation (TMS) applies varied pulse waveforms (98). Transcranial approaches share the goal of directly activating intra-cranial brain structures (99), though ancillary peripheral [e.g., cranial nerve (100)] stimulation cannot be excluded. The rational for tES, and especially tDCS, for COVID-19 brain disorders have been reviewed (77, 101, 102). Several early trials and case series suggest efficacy of tDCS of post-acute sequelae SARS-CoV-2 infection (PASC)/Long-COVID (103–105).
Noninvasive brain stimulation has the broad potential to manage some of the complications of COVID-19 by stimulating the regions involved in the regulation of systemic anti-inflammatory responses and recovery of respiration. There is a bidirectional influence between the brain and immune response (106). Deep brainstem and forebrain regions mediate the immune response throughout the body and can be potential targets for noninvasive neuromodulatory approaches such as tES/TMS. While it is impractical to activate deep regions selectivity (e.g., without activating superficial cortex) by tES or TMS, deep brain regions can certainly be reached by tES (107–110) current and through connectivity to cortical activated areas (111, 112). Cortical regions are conventionally targeted with tES/TMS, including frontal (113) and temporal regions (114) that can be used to influence the systemic immune response and prevent neuroinflammation (106). Furthermore, tES/TMS may be investigated for application in the restoration of respiratory function in recovery (115). There are thus multiple pathways by which tES/TMS can address immediate and long-term COVID-19 morbidity, but subject to direct experimental testing in COVID-19 patients these links remain indirect.
Ultrasound-Guided Percutaneous Electrodes
One means of direct, percutaneous stimulation was recently demonstrated in mice, using low intensity “imaging” ultrasound to guide a percutaneous needle electrode within proximity to the cervical vagus nerve, but can be extrapolated to other targets as well. Using relatively low energy electrical stimulation, biomarkers of vagus engagement, including bradycardia and brainstem c-FOS activation was confirmed. Percutaneous VNS modulated endotoxin-induced TNF production, CNS inflammation, as well as cognitive dysfunction (116). Coupled with the established long-duration of anti-inflammatory effects of VNS after a single treatment (117, 118), this data demonstrates the feasibility of using ultrasound-guided percutaneous electrical stimulation to modify cytokine storm. Translation of this technique to humans should be trivial. While minimally invasive, this procedure would require a trained health care provider to perform and would be limited to in-clinic use. This technique was used to stimulate the vagus nerve in the Miner clinical study of VNS on bronchoconstriction patients (67).
Focused Ultrasound
A promising non-invasive technology is focused ultrasound, which uses acoustic pressure waves to transmit mechanical and heat energy to neural tissues, rather than electrical energy. The long history of focused ultrasound to therapeutically modulate neural tissue since the 1950's, in both central and peripheral systems, has been reviewed elsewhere (119). These established techniques can be used for destructive targeted ablation of tissues, to activate neurons, or to suppress the activation of neural circuits. The elucidation of neural circuits that control inflammation have generated a number of intervention points that may be targeted by focused ultrasound, including deep brain structures, cranial nerves, peripheral nerves, and end organs.
The spleen and its attendant nerves are a major interaction nexus between the neural and immune systems (38, 39, 120), and has recently been explored as a target for focused ultrasound intervention in rodents by several groups (121–124). Focused ultrasound targeting the spleen reduced endotoxin-induced TNF (123), retention of renal form and function following ischemia reperfusion injury, including reduction in renal accumulation of neutrophils and dendritic cells (121, 122), reduction in plasma creatinine following cecal ligation and puncture (122), and attenuation of disease manifestation in a serum transfer model of inflammatory arthritis (124). Demonstrating the potential to tailor a specific outcome by modulating a specifically targeted tissue, focused ultrasound on glucose sensory neurons in the liver prevented hyperglycemia following endotoxin exposure (123). While extremely promising as a non-invasive technique to modulate inflammation and its sequelae, these studies have demonstrated that both the dose delivered and the sub-organ location targeted have a large impact on the physiological outcome, relegating its use to a trained health care provider in-clinic. Focused ultrasound as a modulator of inflammation still requires demonstration of translation to humans.
Alternative Mechanisms
The exact mechanisms of VNS are not fully understood. In addition to activating efferent CAP fibers to the spleen, VNS is believed to proportionally activate afferent fibers (40, 125). Vagal afferents carry sensory information to the dorsal medullary complex, in particular to the nucleus of the solitary tract (NTS). Activation of the NTS plays an important role in modulating cardiopulmonary function (126–128). Therapeutic mechanisms in epilepsy and depression may involve regulating the serotonergic and/or noradrenergic projections from the raphe and LC, respectively, which in turn modulates activity in the hypothalamus, thalamus, insular cortex, and cerebellum, hippocampus, amygdala, and posterior cingulate gyrus (129, 130). These structures are associated with the regulation of mood and emotion, seizure activity, anxiety, intestinal activity, satiety, and pain perception (131–134). Other potential, centrally mediated mechanisms for VNS include elevation of gamma aminobutyric acid (GABA) levels in the brain stem (135), desynchronization of cortical and thalamocortical network activity (125), suppression of cortical spreading depression (136), altering expression of brain-derived neurotrophic factor and other growth factors and increasing norepinephrine in prefrontal cortex (137), and altering brain waste clearance dynamics (138).
Recent COVID-19 Trials
There are currently six studies listed on ClinicalTrials.gov assessing the effects of VNS on immune and/or pulmonary function in COVID-19 (Table 5). Two of the trials used gammaCore, and thus targeted the cervical vagus nerve transcutaneously. Four trials employed several different auricular vagus nerve stimulation devices. Two studies are completed, three are open to enrollment or active but not recruiting, and one terminated early due to low recruitment rates.
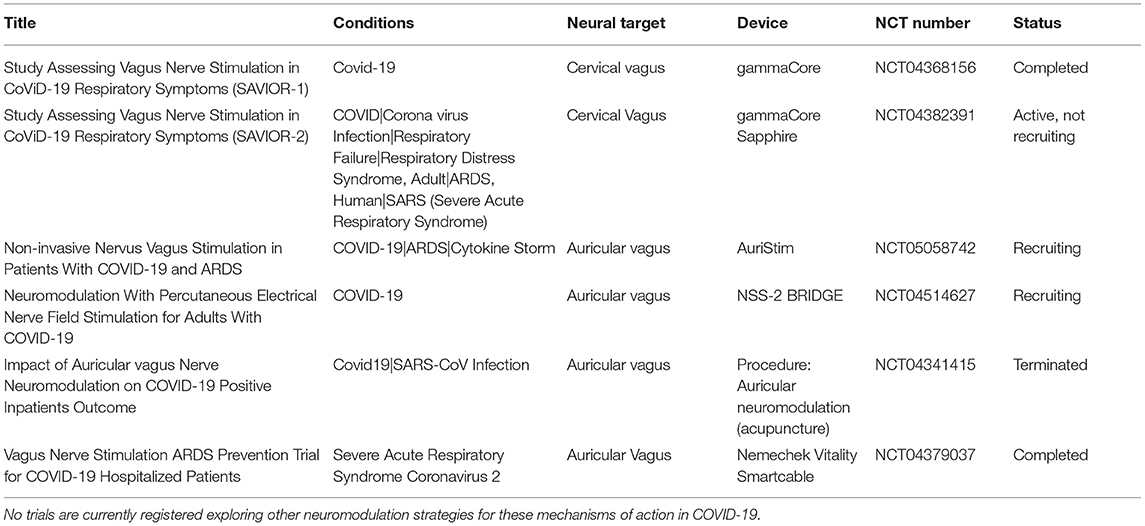
Table 5. Clinical trials of vagus nerve stimulation in COVID-19 that are registered at ClinicalTrials.gov and that include proposed mechanisms of action related to immune and/or pulmonary function.
The use of cervical non-invasive VNS in COVID-19 was first published in a case report of two COVID-19-positive subjects, which provided preliminary observations that transcutaneous cervical VNS reduced the use of opioid and cough suppressant medications and relieved symptoms of chest tightness and shortness of breath (139). Two randomized control trials of gammaCore in inpatients with COVID-19 and respiratory distress have since been initiated: SAVIOR-1, performed in Valencia, Spain, was a prospective, randomized controlled trial (RCT) of VNS vs. standard of care, which evaluated a total of 90 patients, 45 in each arm. Improvement in respiratory function and proinflammatory cytokine levels were measured following VNS (NCT04368156) (140). SAVIOR-2, performed at Allegheny Health Network, was scheduled to study 60 patients and also followed respiratory parameters and cytokines (NCT04382391). This study closed to enrollment after 21 subjects due to low case burdens resulting in low recruitment rates.
The remaining studies employed devices targeting the auricular branch of the vagus nerve either in the cymba concha or the tragus. The results of the first study suggest that auricular VNS results in infrequent mechanical ventilation and a high rate of survival, though it should be noted that this trial in 51 subjects was a single-arm, uncontrolled, open-label, observational trial (141). Studies of two additional auricular VNS devices are actively recruiting, and results are not yet available. A French study is assessing auricular nerve stimulation via four percutaneously placed leads on each tragus and is being compared to sham (no needle placement; NCT04341415). Enrollment has been halted due to a low case load (142).
Future Perspectives
COVID-19 is a deadly disease, the most serious effects of which appear to be hyperactivation of an acute inflammatory response (“cytokine storm”) and compromised lung function. To date, pharmacological strategies have not been generally successful in modulating the cytokine response and clinical sequellae to SARS-CoV-2, with the exception of dexamethasone, a non-specific anti-inflammatory corticosteroid that reduces mortality by one-third in severe COVID patients with acute respiratory distress (143). As described here, there is substantial preclinical and clinical evidence that neuromodulation strategies may have a salutary impact on the host responses to the virus, and clinical trials to assess these strategies are warranted. Given the unabated SARS-CoV-2 pandemic, speed to execute these trials and deliver rigorous results is of essence.
The authors of this paper have formed the International Consortium on Neuromodulation for COVID-19 (ICNC; www.covidneuromod.org) with the mission to provide global leadership for the rapid advancement and clinical adoption of neuromodulation technologies to treat emerging infectious diseases. The ICNC envisions a global infrastructure capable of the rapid deployment and clinical validation of neuromodulation technologies for pandemics, epidemics, and other emerging public health crises for which they may be efficacious. Further, beyond applications for immunological and respiratory control in response to infection, neuromodulation has multiple potential therapeutic applications for the post-acute recovery phase. For example, a recent report has indicated that >87% of COVID-19 patients continue to experience at least one symptom following acute recovery (144), the most frequent of which was fatigue. Noninvasive brain stimulation approaches have reliable benefit for fatigue (145), as well as for psychological symptoms such as depression and anxiety that are common in the recovery period (146–148).
ICNC members have developed recommended endpoints for neuromodulation clinical in COVID-19, as well as recommended publishing guidelines to describe the technology tested.
While prospective, RCTs are the gold standard for such clinical trials and sham control arms are desirable, they might not always be necessary if objective endpoints are used to measure outcomes (149). Such endpoints will vary based on hypothesized mechanism of action of the technology, as well as the patient population being studied: wearable and handheld neuromodulation technologies can be trialed in hospitalized patients (non-ICU or ICU), as well as ambulatory outpatients pre- or post-hospitalization. Nevertheless, some key data elements and outcome measures are recommended, in the anticipation that post-study meta-analyses can illuminate overarching trends not apparent in individual studies (Table 6). Upon publication of study results, the ICNC members recommend careful descriptions of the neuromodulation technology (150).
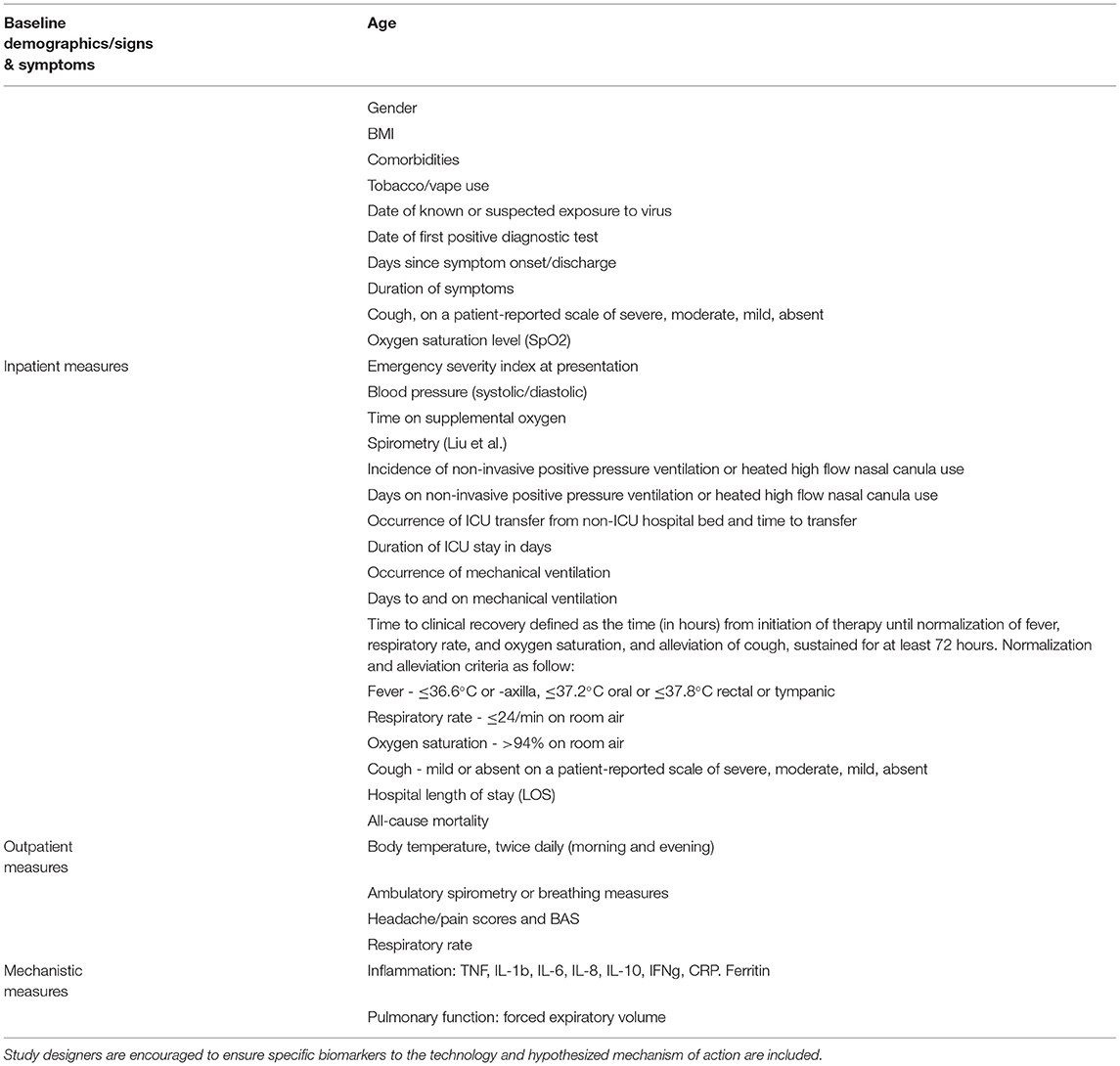
Table 6. Recommended clinical trial endpoints for studies of neuromodulation technologies in COVID-19.
We strongly believe that multi-national and academic-industry collaborative and coordinated efforts to tackle COVID-19 are necessary to advance our knowledge and provide the urgently needed therapeutic options. Our present collaboration and this review on neuromodulation will hopefully serve as an example/roadmap for other scientific areas.
Author Contributions
All authors wrote sections of the manuscript, contributed to manuscript revision, and read and approved the submitted version.
Conflict of Interest
CC is an equity holder of Convergent Medical Technologies, Inc., reports personal fees from electroCore Inc., and Spark Biomedical, and has issued and pending patents related to vagus nerve stimulation to control bleeding and inflammation. MB reports personal fees from Soterix Medical, grants from Google X, personal fees from Halo Neuroscience, outside the submitted work; in addition, he has a patent Brain Stimulation issued. MFr reports pending patent applications describing injectable electrode structures for Neuronoff, Inc., and is an employee and equity holder of Neuronoff, Inc. He further reports issued patents for selective Vagus nerve stimulation and selective electrical vagal block for regulation of autonomic functions such as heart rate and blood pressure for Boston Scientific. MFu reports personal fees from Axon Therapies, CVRx, Daxor, Edwards Life Sciences, Galvani, and Respicardia, outside the submitted work. SH is an employee and equity holder of Cala Health. NK reports a patent devices and methods for reducing inflammation using electrical stimulation pending. BS reports personal fees from electroCore, and reports patents issued and pending related to transcutaneous cervical vagus nerve stimulation. PS is an employee and equity holder of electroCore, and reports patents issued and pending related to transcutaneous cervical vagus nerve stimulation.
The remaining authors declare that the research was conducted in the absence of any commercial or financial relationships that could be construed as a potential conflict of interest.
Publisher's Note
All claims expressed in this article are solely those of the authors and do not necessarily represent those of their affiliated organizations, or those of the publisher, the editors and the reviewers. Any product that may be evaluated in this article, or claim that may be made by its manufacturer, is not guaranteed or endorsed by the publisher.
Acknowledgments
The authors wish to thank additional members of the ICNC for their discussions and advice on this manuscript and other ICNC matters: Abrahão Fontes Baptista, Lecio Figueira, Eugenijus Kaniusas, Mark Lambert, Hubert Lim, Alexandre OKano, Fivos Panetsos, JoJo Platt, Lawrence Poree, Daniel Powell, Christopher Puleo, Claire-Marie Rangon, Carlos Tornero, and Ricardo Vallejo.
References
1. Ader F, Bouscambert-Duchamp M, Hites M, Peiffer-Smadja N, Poissy J, Belhadi D, et al. Remdesivir plus standard of care versus standard of care alone for the treatment of patients admitted to hospital with COVID-19 (DisCoVeRy): a phase 3, randomised, controlled, open-label trial. Lancet Infect Dis. (2022) 22:209–21. doi: 10.1016/S1473-3099(21)00485-0
2. Tay MZ, Poh CM, Rénia L, MacAry PA, Ng LFP. The trinity of COVID-19: immunity, inflammation and intervention. Nat Rev Immunol. (2020) 20:363–74. doi: 10.1038/s41577-020-0311-8
3. Gustine JN, Jones D. Immunopathology of Hyperinflammation in COVID-19. Am J Pathol. (2020). doi: 10.1016/j.ajpath.2020.08.009
4. Merad M, Martin JC. Pathological inflammation in patients with COVID-19: a key role for monocytes and macrophages. Nat Rev Immunol Nat Res. (2020) 20:355–62. doi: 10.1038/s41577-020-0331-4
5. Del Valle DM, Kim-Schulze S, Huang HH, Beckmann ND, Nirenberg S, Wang B, et al. An inflammatory cytokine signature predicts COVID-19 severity and survival. Nat Med. (2020) 26:1636–43. doi: 10.1038/s41591-020-1051-9
6. Kim IC, Kim HA, Park JS, Nam CW. Updates of cardiovascular manifestations in COVID-19: Korean experience to broaden worldwide perspectives. Korean Circ J. (2020) 50:543. doi: 10.4070/kcj.2020.0205
7. Tang N, Li D, Wang X, Sun Z. Abnormal coagulation parameters are associated with poor prognosis in patients with novel coronavirus pneumonia. J Thromb Haemost. (2020) 18:844–7. doi: 10.1111/jth.14768
8. Oxley TJ, Mocco J, Majidi S, Kellner CP, Shoirah H, Singh IP, et al. Large-vessel stroke as a presenting feature of covid-19 in the young. N Engl J Med. (2020) 382:e60. doi: 10.1056/NEJMc2009787
9. Liu PP, Blet A, Smyth D, Li H. The Science underlying COVID-19: implications for the cardiovascular system. Circulation. (2020) 142:68–78. doi: 10.1161/CIRCULATIONAHA.120.047549
10. Zhang Y, Xiao M, Zhang S, Xia P, Cao W, Jiang W, et al. Coagulopathy and antiphospholipid antibodies in patients with covid-19. N Engl J Med. (2020) 382:E38. doi: 10.1056/NEJMc2007575
11. Levi M, Van Der Poll T. Coagulation in sepsis: all bugs bite equally. Crit Care. (2004) 8:99–100. doi: 10.1186/cc2595
12. Van Der Poll T, Van De Veerdonk FL, Scicluna BP, Netea MG. The immunopathology of sepsis and potential therapeutic targets. Nat Rev Immunol. (2017) 17:407–20. doi: 10.1038/nri.2017.36
13. Chiu IM, Von Hehn CA, Woolf CJ. Neurogenic inflammation and the peripheral nervous system in host defense and immunopathology. Nat Neurosci. (2012) 15:1063–7. doi: 10.1038/nn.3144
14. Chu C, Artis D, Chiu IM. Neuro-immune Interactions in the Tissues. Immunity. (2020) 52:464–74. doi: 10.1016/j.immuni.2020.02.017
15. Bellocchi C, Carandina A, Montinaro B, Targetti E, Furlan L, Rodrigues GD, et al. The interplay between autonomic nervous system and inflammation across systemic autoimmune diseases. Int J Mol Sci. (2022) 23:2449. doi: 10.3390/ijms23052449
16. Caravaca AS, Tsaava T, Goldman L, Silverman H, Riggott G, Chavan SS, et al. A novel flexible cuff-like microelectrode for dual purpose, acute and chronic electrical interfacing with the mouse cervical vagus nerve. J Neural Eng. (2017) 14:066005. doi: 10.1088/1741-2552/aa7a42
17. Zanos TP, Silverman HA, Levy T, Tsaava T, Battinelli E, Lorraine PW, et al. Identification of cytokine-specific sensory neural signals by decoding murine vagus nerve activity. Proc Natl Acad Sci U S A. (2018) 115:E4843–52. doi: 10.1073/pnas.1719083115
18. Andersson U, Tracey KJ. Reflex principles of immunological homeostasis. Annu Rev Immunol. (2012) 30:313–35. doi: 10.1146/annurev-immunol-020711-075015
19. Vaillancourt M, Chia P, J Sarji S, Nguyen Hoftman N, Ruffenach G, et al. Autonomic nervous system involvement in pulmonary arterial hypertension. Respir Res. (2017) 18:201. doi: 10.1186/s12931-017-0679-6
20. Al-kuraishy HM, Al-Gareeb AI, Mostafa-Hedeab G, Kasozi KI, Zirintunda G, Aslam A, et al. Effects of β-Blockers on the Sympathetic and Cytokines Storms in Covid-19. Front Immunol. (2021) 12:749291. doi: 10.3389/fimmu.2021.749291
21. McAllen RM, McKinley MJ, Martelli D. Reflex regulation of systemic inflammation by the autonomic nervous system. Auton Neurosci. (2022) 237:102926. doi: 10.1016/j.autneu.2021.102926
22. Borovikova L V, Ivanova S, Zhang M, Yang H, Botchkina GI, Watkins LR, et al. Vagus nerve stimulation attenuates the systemic inflammatory response to endotoxin. Nature. (2000) 405:458–62. doi: 10.1038/35013070
23. Steinberg BE, Sundman E, Terrando N, Eriksson LI, Olofsson PS. Neural control of inflammation. Anesthesiology. (2016) 124:1174–89. doi: 10.1097/ALN.0000000000001083
24. Li L, Wang D, Pan H, Huang L, Sun X, He C, et al. Non-invasive Vagus nerve stimulation in cerebral stroke: current status and future perspectives. Front Neurosci. (2022) 16:820665. doi: 10.3389/fnins.2022.820665
25. Du L, Yang Z, Sheng H, Liu M, Sun Q. Effects of Long-term vagus nerve electrical stimulation therapy on acute cerebral infarction and neurological function recovery in post MCAO mice. Oxid Med Cell Longev. (2022) 2022:1–9. doi: 10.1155/2022/8131391
26. Azabou E, Bao G, Costantino F, Jacota M, Lazizi C, Nkam L, et al. Randomized cross over study assessing the efficacy of non-invasive stimulation of the vagus nerve in patients with axial spondyloarthritis resistant to biotherapies: the ESNV-SPA study protocol. Front Hum Neurosci. (2021) 15:679775. doi: 10.3389/fnhum.2021.679775
27. Powell K, Shah K, Hao C, Wu YC, John A, Narayan RK, et al. Neuromodulation as a new avenue for resuscitation in hemorrhagic shock. Bioelectronic Medicine. (2019) 5:17. doi: 10.1186/s42234-019-0033-z
28. Wu J, Yin Y, Qin M, Li K, Liu F, Zhou X, et al. Vagus nerve stimulation protects enterocyte Glycocalyx after hemorrhagic shock via the cholinergic anti-inflammatory pathway. Shock. (2021) 56:832–9. doi: 10.1097/SHK.0000000000001791
29. Bonaz B, Sinniger V, Pellissier S. Therapeutic potential of vagus nerve stimulation for inflammatory bowel diseases. Front Neurosci. (2021) 15:650971. doi: 10.3389/fnins.2021.650971
30. Caravaca AS, Levine YA, Drake A, Eberhardson M, Olofsson PS. Vagus nerve stimulation reduces indomethacin-induced small bowel inflammation. Front Neurosci. (2022) 15:730407. doi: 10.3389/fnins.2021.730407
31. Hoover DB. Cholinergic modulation of the immune system presents new approaches for treating inflammation. Pharmacol Ther. (2017) 179:1–16. doi: 10.1016/j.pharmthera.2017.05.002
32. Costantini TW, Coimbra R, Weaver JL, Eliceiri BP. Precision targeting of the vagal anti-inflammatory pathway attenuates the systemic inflammatory response to burn injury. J Trauma Acute Care Surg. (2022) 92:323–9. doi: 10.1097/TA.0000000000003470
33. Courties A, Berenbaum F, Sellam J. Vagus nerve stimulation in musculoskeletal diseases. Joint Bone Spine. (2021) 88:105149. doi: 10.1016/j.jbspin.2021.105149
34. Brock C, Rasmussen SE, Drewes AM, Møller HJ, Brock B, Deleuran B, et al. Vagal nerve stimulation-modulation of the anti-inflammatory response and clinical outcome in psoriatic arthritis or ankylosing spondylitis. Mediators Inflamm. (2021) 2021:1–9. doi: 10.1155/2021/9933532
35. Dalli J, Colas RA, Arnardottir H, Serhan CN. Vagal regulation of group 3 innate lymphoid cells and the immunoresolvent PCTR1 controls infection resolution. Immunity. (2017) 46:92–105. doi: 10.1016/j.immuni.2016.12.009
36. Cox MA, Duncan GS, Lin GHY, Steinberg BE, Yu LX, Brenner D, et al. Choline acetyltransferase–expressing T cells are required to control chronic viral infection. Science. (2019) 8:363. doi: 10.1126/science.aau9072
37. Malin SG, Shavva VS, Tarnawski L, Olofsson PS. Functions of acetylcholine-producing lymphocytes in immunobiology. Curr Opin Neurobiol. (2020) 62:115–21. doi: 10.1016/j.conb.2020.01.017
38. Rosas-Ballina M, Olofsson PS, Ochani M, Valdés-Ferrer SI, Levine YA, Reardon C, et al. Acetylcholine-synthesizing T cells relay neural signals in a vagus nerve circuit. Science. (2011) 334:98–101. doi: 10.1126/science.1209985
39. Huston JM, Ochani M, Rosas-Ballina M, Liao H, Ochani K, Pavlov VA, et al. Splenectomy inactivates the cholinergic antiinflammatory pathway during lethal endotoxemia and polymicrobial sepsis. J Exp Med. (2006) 203:1623–9. doi: 10.1084/jem.20052362
40. Olofsson PS, Rosas-Ballina M, Levine YA, Tracey KJ. Rethinking inflammation: Neural circuits in the regulation of immunity. Immunol Rev. (2012) 248:188–204. doi: 10.1111/j.1600-065X.2012.01138.x
41. Hoffmann TJ, Mendez S, Staats P, Emala CW, Guo P. Inhibition of histamine-induced bronchoconstriction in guinea pig and swine by pulsed electrical vagus nerve stimulation. Neuromodulation. (2009) 12:261–9. doi: 10.1111/j.1525-1403.2009.00234.x
42. Hoffmann TJ, Simon BJ, Zhang Y, Emala CW. Low voltage vagal nerve stimulation reduces bronchoconstriction in guinea pigs through catecholamine release. Neuromodulation. (2012) 15:527–36. doi: 10.1111/j.1525-1403.2012.00454.x
43. Ricciardolo FLM, Trevisani M, Geppetti P, Nadel JA, Amadesi S, Bertrand C. Role of nitric oxide and septide-insensitive NK1 receptors in bronchoconstriction induced by aerosolised neurokinin A in guinea-pigs. Br J Pharmacol. (2000) 129:915–20. doi: 10.1038/sj.bjp.0703135
44. Sepulveda P, Bohill G, Hoffmann TJ. Treatment of asthmatic bronchoconstriction by percutaneous low voltage vagal nerve stimulation: case report. Internet J Asthma Allergy Immunol. (2010) 7:2. doi: 10.5580/4a7
45. Pavlov VA, Chavan SS, Tracey KJ. Molecular and Functional Neuroscience in Immunity. Annu Rev Immunol. (2018) 36:783–812. doi: 10.1146/annurev-immunol-042617-053158
46. Hosoi T, Okuma Y, Nomura Y. Electrical stimulation of afferent vagus nerve induces IL-1β expression in the brain and activates HPA axis. Am J Physiol Regul Integr Comp Physiol. (2000) 279:R141–7. doi: 10.1152/ajpregu.2000.279.1.R141
47. Knox AJ, Campos-Gongora H, Wisniewski A, MacDonald IA, Tattersfield AE. Modification of bronchial reactivity by physiological concentrations of plasma epinephrine. J Appl Physiol. (1992) 73:1004–7. doi: 10.1152/jappl.1992.73.3.1004
48. Simillis C, Lal N, Qiu S, Kontovounisios C, Rasheed S, Tan E, et al. Sacral nerve stimulation versus percutaneous tibial nerve stimulation for faecal incontinence: a systematic review and meta-analysis. Int J Colorectal Dis. (2018) 33:645–8. doi: 10.1007/s00384-018-2976-z
49. Siddiqui NY, Wu JM, Amundsen CL. Efficacy and adverse events of sacral nerve stimulation for overactive bladder: a systematic review. Neurourol Urodyn. (2010) 29 Suppl 1: S18–23. doi: 10.1002/nau.20786
50. Wang Y, Hassouna MM. Neuromodulation reduces c-fos gene expression in spinalized rats: a double-blind randomized study. J Urol. (2000) 163:1966–70. doi: 10.1097/00005392-200006000-00103
51. Elkelini MS, Abuzgaya A, Hassouna MM. Mechanisms of action of sacral neuromodulation. Int Urogynecol J. (2010) 21:439–46. doi: 10.1007/s00192-010-1273-3
52. Aboseif S, Tamaddon K, Chalfin S, Freedman S, Mourad MS, Chang JH, et al. Sacral neuromodulation in functional urinary retention: an effective way to restore voiding. BJU Int. (2002) 90:662–5. doi: 10.1046/j.1464-410X.2002.02989.x
53. Janssen PTJ, Komen N, Melenhorst J, Bouvy ND, Jahanshahi A, Temel Y, et al. Sacral neuromodulation for fecal incontinence. J Clin Gastroenterol. (2017) 51:669–76. doi: 10.1097/MCG.0000000000000850
54. Gourcerol G, Vitton V, Leroi AM, Michot F, Abysique A, Bouvier M. How sacral nerve stimulation works in patients with faecal incontinence. Colorectal Dis. (2011) 13:e203–11. doi: 10.1111/j.1463-1318.2011.02623.x
55. Zhang N, Zhang H, Jiang L, Zhang S, Yin J, Schramm L, et al. A novel method of sacral nerve stimulation for colonic inflammation. Neurogastroenterol Motil. (2020) 1:32. doi: 10.1111/nmo.13825
56. Pasricha TS, Zhang H, Zhang N, Chen JDZ. Sacral nerve stimulation prompts vagally-mediated amelioration of rodent colitis. Physiol Rep. (2020) 1:8. doi: 10.14814/phy2.14294
57. Tu L, Gharibani P, Zhang N, Yin J, Chen JD. Anti-inflammatory effects of sacral nerve stimulation: a novel spinal afferent and vagal efferent pathway. Am J Physiol. (2020) 318:G624–34. doi: 10.1152/ajpgi.00330.2019
58. Ye F, Liu Y, Li S, Zhang S, Foreman RD, Chen JD. Sacral nerve stimulation increases gastric accommodation in rats: a spinal afferent and vagal efferent pathway. Am J Physiol. (2020) 318:G574–81. doi: 10.1152/ajpgi.00255.2019
59. Guo J, Jin H, Shi Z, Yin J, Pasricha T, Chen JDZ. Sacral nerve stimulation improves colonic inflammation mediated by autonomic-inflammatory cytokine mechanism in rats. Neurogastroenterol Motil. (2019) 1:31. doi: 10.1111/nmo.13676
60. Wang X, Zhang S, Pasricha PJ, Chen JDZ. Ameliorating effects of sacral neuromodulation on gastric and small intestinal dysmotility mediated via a sacral afferent-vagal efferent pathway. Neurogastroenterol Motil. (2020) 1:32. doi: 10.1111/nmo.13837
61. Pancrazio J. Introduction. In: Krames ES, Peckham PH RA, editor. Neuromodulation: Comprehensive Textbook of Principles, Technologies, and Therapies. 2nd edn. London: Academic Press Inc. (2018). p. 69–70.
62. Vonck K, Boon P. Epilepsy: Closing the loop for patients with epilepsy. Nat Rev Neurol. (2015) 11:252–4. doi: 10.1038/nrneurol.2015.56
63. Mertens A, Raedt R, Gadeyne S, Carrette E, Boon P, Vonck K. Recent advances in devices for vagus nerve stimulation. Expert Rev Med Devices. (2018) 15:527–39. doi: 10.1080/17434440.2018.1507732
64. Koopman FA, Chavan SS, Miljko S, Grazio S, Sokolovic S, Schuurman PR, et al. Vagus nerve stimulation inhibits cytokine production and attenuates disease severity in Rheumatoid arthritis. Proc Natl Acad Sci U S A. (2016) 113:8284–9. doi: 10.1073/pnas.1605635113
65. Sinniger V, Pellissier S, Fauvelle F, Trocmé C, Hoffmann D, Vercueil L, et al. A 12-month pilot study outcomes of vagus nerve stimulation in Crohn's disease. Neurogastroenterol Motil. (2020). doi: 10.1111/nmo.13911
66. Eberhardson M, Tarnawski L, Centa M, Olofsson PS. Neural control of inflammation: bioelectronic medicine in treatment of chronic inflammatory disease. Cold Spring Harb Perspect Med. (2020) 1:10. doi: 10.1101/cshperspect.a034181
67. Miner JR, Lewis LM, Mosnaim GS, Varon J, Theodoro D, Hoffmann TJ. Feasibility of percutaneous vagus nerve stimulation for the treatment of acute asthma exacerbations. Acad Emerg Med. (2012) 19:421–9. doi: 10.1111/j.1553-2712.2012.01329.x
68. Steyn E, Mohamed Z, Husselman C. Non-invasive vagus nerve stimulation for the treatment of acute asthma exacerbations - Results from an initial case series. Int J Emerg Med. (2013) 6:7. doi: 10.1186/1865-1380-6-7
69. Staats P, Emala C, Simon B, Errico JP. Neuromodulation for Asthma. In: Krames ES, Peckham PH, Rezai AR, eds. Neuromodulation: Comprehensive Textbook of Principles, Technologies, and Therapies. 2nd ed. Academic Press/Elsevier (2018).
70. Bijwadia JS, Hoch RC, Dexter DD. Identification and treatment of bronchoconstriction induced by a vagus nerve stimulator employed for management of seizure disorder. Chest. (2005) 127:401–2. doi: 10.1378/chest.127.1.401
71. Fudim M, Qadri YJ, Ghadimi K, MacLeod DB, Molinger J, Piccini JP, et al. Implications for neuromodulation therapy to control inflammation and related organ dysfunction in COVID-19. J Cardiovasc Transl Res. (2020) 13:894–9. doi: 10.1007/s12265-020-10031-6
72. Kaniusas E, Szeles JC, Kampusch S, Alfageme-Lopez N, Yucuma-Conde D, Li X, et al. Non-invasive Auricular Vagus Nerve Stimulation as a Potential Treatment for Covid19-Originated Acute Respiratory Distress Syndrome. Front Physiol. (2020) 11:890. doi: 10.3389/fphys.2020.00890
73. Bonaz B, Sinniger V, Pellissier S. Targeting the cholinergic anti-inflammatory pathway with vagus nerve stimulation in patients with Covid-19? Bioelectron Med. (2020) 6:15. doi: 10.1186/s42234-020-00051-7
74. Azabou E, Bao G, Bounab R, Heming N, Annane D. Vagus nerve stimulation: a potential adjunct therapy for COVID-19. Front Med (Lausanne). (2021) 8:625836. doi: 10.3389/fmed.2021.625836
75. Mastitskaya S, Thompson N, Holder D. Selective vagus nerve stimulation as a therapeutic approach for the treatment of ARDS: a rationale for neuro-immunomodulation in COVID-19 disease. Front Neurosci. (2021) 15:667036. doi: 10.3389/fnins.2021.667036
76. Qin Z, Xiang K, Su DF, Sun Y, Liu X. Activation of the cholinergic anti-inflammatory pathway as a novel therapeutic strategy for COVID-19. Front Immunol. (2020) 11:595342. doi: 10.3389/fimmu.2020.595342
77. Baptista AF, Baltar A, Okano AH, Moreira A, Campos ACP, Fernandes AM, et al. Applications of non-invasive neuromodulation for the management of disorders related to COVID-19. Front Neurol. (2020) 11:573718. doi: 10.3389/fneur.2020.573718
78. Bara GA, de Ridder D, Maciaczyk J. Can neuromodulation support the fight against the COVID19 pandemic? Transcutaneous non-invasive vagal nerve stimulation as a potential targeted treatment of fulminant acute respiratory distress syndrome. Med Hypotheses. (2020) 143:110093. doi: 10.1016/j.mehy.2020.110093
79. Andersson U. The cholinergic anti-inflammatory pathway alleviates acute lung injury. Mol Med. (2020) 26:64. doi: 10.1186/s10020-020-00184-0
80. Frangos E, Ellrich J, Komisaruk BR. Non-invasive Access to the vagus nerve central projections via electrical stimulation of the external ear: fMRI evidence in humans. Brain Stimul. (2015) 8:624–36. doi: 10.1016/j.brs.2014.11.018
81. Frangos E, Komisaruk BR. Access to vagal projections via cutaneous electrical stimulation of the neck: fMRI evidence in healthy humans. Brain Stimul. (2017) 10:19–27. doi: 10.1016/j.brs.2016.10.008
82. Komisaruk BR, Frangos E. Vagus nerve afferent stimulation: projection into the brain, reflexive physiological, perceptual, and behavioral responses, and clinical relevance. Auton Neurosci. (2022) 237:102908. doi: 10.1016/j.autneu.2021.102908
83. Lerman I, Hauger R, Sorkin L, Proudfoot J, Davis B, Huang A, et al. Noninvasive transcutaneous vagus nerve stimulation decreases whole blood culture-derived cytokines and chemokines: a randomized, blinded, healthy control pilot trial. Neuromodulation. (2016) 19:283–91. doi: 10.1111/ner.12398
84. Brock C, Brock B, Aziz Q, Møller HJ, Pfeiffer Jensen M, Drewes AM, et al. Transcutaneous cervical vagal nerve stimulation modulates cardiac vagal tone and tumor necrosis factor-alpha. Neurogastroenterol Motil. (2017) 1:29. doi: 10.1111/nmo.12999
85. Tarn J, Legg S, Mitchell S, Simon B, Ng WF. The effects of noninvasive vagus nerve stimulation on fatigue and immune responses in patients with primary sjögren's syndrome. Neuromodulation. (2019) 22:580–5. doi: 10.1111/ner.12879
86. Wittbrodt MT, Gurel NZ, Nye JA, Ladd S, Shandhi MMH, Huang M, et al. Non-invasive vagal nerve stimulation decreases brain activity during trauma scripts. Brain Stimul. (2020) 13:1333–48. doi: 10.1016/j.brs.2020.07.002
87. Mondal B, Choudhury S, Banerjee R, Roy A, Chatterjee K, Basu P, et al. Non-invasive vagus nerve stimulation improves clinical and molecular biomarkers of Parkinson's disease in patients with freezing of gait. NPJ Parkinsons Dis. (2021) 7:46. doi: 10.1038/s41531-021-00190-x
88. Bremner JD, Wittbrodt MT, Gurel NZ, Shandhi MH, Gazi AH, Jiao Y, et al. Transcutaneous cervical vagal nerve stimulation in Patients with Posttraumatic Stress Disorder (PTSD): a pilot study of effects on PTSD symptoms and interleukin-6 response to stress. J Affect Disord Rep. (2021) 6: 100190. doi: 10.1016/j.jadr.2021.100190
89. U.S. Food and Drug Administration. electroCore EUA. Available online at: https://www.fda.gov/media/139967/download (accessed March 12, 2022).
90. Chae JH, Nahas Z, Lomarev M, Denslow S, Lorberbaum JP, Bohning DE, et al. review of functional neuroimaging studies of vagus nerve stimulation (VNS). J Psychiatr Res. (2003) 37:443–55. doi: 10.1016/S0022-3956(03)00074-8
91. Kraus T, Kiess O, Hösl K, Terekhin P, Kornhuber J, Forster C, et al. fMRI effects of sham-controlled transcutaneous electrical nerve stimulation in the left outer auditory canal - a pilot study. Brain Stimul. (2013) 6:798–804. doi: 10.1016/j.brs.2013.01.011
92. Salama M, Akan A, Mueller MR. Transcutaneous stimulation of auricular branch of the vagus nerve attenuates the acute inflammatory response after lung lobectomy. World J Surg. (2020) 1:44. doi: 10.1007/s00268-020-05543-w
93. Stavrakis S, Stoner JA, Humphrey MB, Morris L, Filiberti A, Reynolds JC, et al. TREAT AF (Transcutaneous Electrical Vagus Nerve Stimulation to Suppress Atrial Fibrillation): a randomized clinical trial. JACC Clin Electrophysiol. (2020) 6:282–91. doi: 10.1016/j.jacep.2019.11.008
94. Addorisio ME, Imperato GH, de Vos AF, Forti S, Goldstein RS, Pavlov VA, et al. Investigational treatment of rheumatoid arthritis with a vibrotactile device applied to the external ear. Bioelectron Med. (2019) 5:4. doi: 10.1186/s42234-019-0020-4
95. Health Canada. Dolphin Extended Use Authorization. Available online at: https://www.canada.ca/en/health-canada/services/drugs-health-products/covid19-industry/medical-devices/authorized/expanded-use.html (accessed March 13, 2022).
96. Woods AJ, Antal A, Bikson M, Boggio PS, Brunoni AR, Celnik P, et al. A technical guide to tDCS, and related non-invasive brain stimulation tools. Clin Neurophysiol. (2016) 127:1031–48. doi: 10.1016/j.clinph.2015.11.012
97. Antal A, Alekseichuk I, Bikson M, Brockmöller J, Brunoni AR, Chen R, et al. Low intensity transcranial electric stimulation: Safety, ethical, legal regulatory and application guidelines. Clin Neurophysiol. (2017) 128:1774–809. doi: 10.1016/j.clinph.2017.06.001
98. Huang YZ, Sommer M, Thickbroom G, Hamada M, Pascual-Leonne A, Paulus W, et al. Consensus: New methodologies for brain stimulation. Brain Stimul. (2009) 2:2–13. doi: 10.1016/j.brs.2008.09.007
99. Peterchev AV, Wagner TA, Miranda PC, Nitsche MA, Paulus W, Lisanby SH, et al. Fundamentals of transcranial electric and magnetic stimulation dose: definition, selection, and reporting practices. Brain Stimul. (2012) 5:435–53. doi: 10.1016/j.brs.2011.10.001
100. Adair D, Truong D, Esmaeilpour Z, Gebodh N, Borges H, Ho L, et al. Electrical stimulation of cranial nerves in cognition and disease. Brain Stimul. (2020) 13:717–50. doi: 10.1016/j.brs.2020.02.019
101. Pilloni G, Bikson M, Badran BW, George MS, Kautz SA, Okano AH, et al. Update on the Use of Transcranial Electrical Brain Stimulation to Manage Acute and Chronic COVID-19 Symptoms. Front Hum Neurosci. (2020) 14:595567. doi: 10.3389/fnhum.2020.595567
102. Silva Filho E, Moura S, Santos ADC, Brasileiro-Santos MDS, Albuquerque JA. Transcranial direct current stimulation as a strategy to manage COVID-19 pain and fatigue. Rev Assoc Med Bras. (2021) 67:26–8. doi: 10.1590/1806-9282.67.01.20200671
103. Eilam-Stock T, George A, Lustberg M, Wolintz R, Krupp LB, Charvet LE. Telehealth transcranial direct current stimulation for recovery from Post-Acute Sequelae of SARS-CoV-2 (PASC). Brain Stimul. (2021) 14:1520–2. doi: 10.1016/j.brs.2021.10.381
104. Gómez L, Vidal B, Cabrera Y, Hernández L, Rondón Y. Successful Treatment of Post-COVID Symptoms With Transcranial Direct Current Stimulation. Prim Care Companion CNS Disord. (2021) 2:23. doi: 10.4088/PCC.21cr03059
105. Rosen AC, Lavacot JA, Porter IM, Chao SZ, Bikson M, Kumar AA, et al. TDCS in a patient with dreadlocks: Improvements in COVID-19 related verbal fluency dysfunction. Brain Stimul. (2022) 15:254–6. doi: 10.1016/j.brs.2022.01.004
106. Dantzer R. Neuroimmune interactions: from the brain to the immune system and vice versa. Physiol Rev. (2018) 98:477–504. doi: 10.1152/physrev.00039.2016
107. Gomez-Tames J, Asai A, Hirata A. Significant group-level hotspots found in deep brain regions during transcranial direct current stimulation (tDCS): a computational analysis of electric fields. Clin Neurophysiol. (2020) 131:755–65. doi: 10.1016/j.clinph.2019.11.018
108. Dasilva AF, Mendonca ME, Zaghi S, Lopes M, Dossantos MF, Spierings EL, et al. TDCS-induced analgesia and electrical fields in pain-related neural networks in chronic migraine. Headache. (2012) 52:1283–95. doi: 10.1111/j.1526-4610.2012.02141.x
109. Huang Y, Datta A, Parra LC. Optimization of interferential stimulation of the human brain with electrode arrays. J Neural Eng. (2020) 17:036023. doi: 10.1088/1741-2552/ab92b3
110. Huang Y, Liu AA, Lafon B, Friedman D, Dayan M, Wang X, et al. Measurements and models of electric fields in the in vivo human brain during transcranial electric stimulation. Elife. (2017) 7:6. doi: 10.7554/eLife.18834
111. Bestmann S, Feredoes E. Combined neurostimulation and neuroimaging in cognitive neuroscience: past, present, and future. Ann N Y Acad Sci. (2013) 1296:11–30. doi: 10.1111/nyas.12110
112. Bestmann S, Baudewig J, Siebner HR, Rothwell JC, Frahm J. BOLD MRI responses to repetitive TMS over human dorsal premotor cortex. Neuroimage. (2005) 28:22–9. doi: 10.1016/j.neuroimage.2005.05.027
113. Iseger TA, van Bueren NER, Kenemans JL, Gevirtz R, Arns M. A frontal-vagal network theory for Major Depressive Disorder: Implications for optimizing neuromodulation techniques. Brain Stimul. (2020) 13:1–9. doi: 10.1016/j.brs.2019.10.006
114. Montenegro RA, Farinatti Pde T, Fontes EB, Soares PP, Cunha FA, Gurgel JL, et al. Transcranial direct current stimulation influences the cardiac autonomic nervous control. Neurosci Lett. (2011) 497:32–6. doi: 10.1016/j.neulet.2011.04.019
115. Vandermeeren Y, Jamart J, Ossemann M. Effect of tDCS with an extracephalic reference electrode on cardio-respiratory and autonomic functions. BMC Neurosci. (2010) 16:11. doi: 10.1186/1471-2202-11-38
116. Huffman WJ, Subramaniyan S, Rodriguiz RM, Wetsel WC, Grill WM, Terrando N. Modulation of neuroinflammation and memory dysfunction using percutaneous vagus nerve stimulation in mice. Brain Stimul. (2019) 12:19–29. doi: 10.1016/j.brs.2018.10.005
117. Huston JM, Gallowitsch-Puerta M, Ochani M, Ochani K, Yuan R, Rosas-Ballina M, et al. Transcutaneous vagus nerve stimulation reduces serum high mobility group box 1 levels and improves survival in murine sepsis. Crit Care Med. (2007) 35:2762–8. doi: 10.1097/00003246-200712000-00014
118. Levine YA, Faltys M, Chernoff D. Harnessing the inflammatory reflex for the treatment of inflammation-mediated diseases. Cold Spring Harb Perspect Med. (2020) 1:10. doi: 10.1101/cshperspect.a034330
119. Blackmore J, Shrivastava S, Sallet J, Butler CR, Cleveland RO. Ultrasound neuromodulation: a review of results, mechanisms and safety. Ultrasound in Medicine and Biology Elsevier USA. (2019) 45:1509–36. doi: 10.1016/j.ultrasmedbio.2018.12.015
120. Rosas-Ballina M, Ochani M, Parrish WR, Ochani K, Harris YT, Huston JM, et al. Splenic nerve is required for cholinergic antiinflammatory pathway control of TNF in endotoxemia. Proc Natl Acad Sci U S A. (2008) 105:11008–13. doi: 10.1073/pnas.0803237105
121. Gigliotti JC, Huang L, Ye H, Bajwa A, Chattrabhuti K, Lee S, et al. Ultrasound prevents renal ischemia-reperfusion injury by stimulating the splenic cholinergic anti-inflammatory pathway. J Am Soc Nephrol. (2013) 24:1451–60. doi: 10.1681/ASN.2013010084
122. Gigliotti JC, Huang L, Bajwa A, Ye H, Mace EH, Hossack JA, et al. Ultrasound modulates the splenic neuroimmune axis in attenuating AKI. J Am Soc Nephrol. (2015) 26:2470–81. doi: 10.1681/ASN.2014080769
123. Cotero V, Fan Y, Tsaava T, Kressel AM, Hancu I, Fitzgerald P, et al. Noninvasive sub-organ ultrasound stimulation for targeted neuromodulation. Nat Commun. (2019) 1:10. doi: 10.1038/s41467-019-08750-9
124. Zachs DP, Offutt SJ, Graham RS, Kim Y, Mueller J, Auger JL, et al. Noninvasive ultrasound stimulation of the spleen to treat inflammatory arthritis. Nat Commun. (2019) 1:10. doi: 10.1038/s41467-019-08721-0
125. Henry TR. Therapeutic mechanisms of vagus nerve stimulation. Neurology. (2002) 59(6 Suppl. 4): S3–14. doi: 10.1212/WNL.59.6_suppl_4.S3
126. Groves DA, Brown VJ. Vagal nerve stimulation: a review of its applications and potential mechanisms that mediate its clinical effects. Neurosci Biobehav Rev. (2005) 29:493–500. doi: 10.1016/j.neubiorev.2005.01.004
127. Sluka KA, Walsh D. Transcutaneous electrical nerve stimulation: basic science mechanisms and clinical effectiveness. J Pain. (2003) 4:109–21. doi: 10.1054/jpai.2003.434
128. Dietrich S, Smith J, Scherzinger C, Hofmann-Preiß K, Freitag T, Eisenkolb A, et al. A novel transcutaneous vagus nerve stimulation leads to brainstem and cerebral activations measured by functional MRI. Biomed Tech. (2008) 53:104–11. doi: 10.1515/BMT.2008.022
129. Henry TR, Bakay RAE, Votaw JR, Pennell PB, Epstein CM, Faber TL, et al. Brain blood flow alterations induced by therapeutic vagus nerve stimulation in partial epilepsy: I. Acute effects at high and low levels of stimulation. Epilepsia. (1998) 39:983–90. doi: 10.1111/j.1528-1157.1998.tb01448.x
130. Henry TR, Votaw JR, Pennell PB, Epstein CM, Bakay RAE, Faber TL, et al. Acute blood flow changes and efficacy of vagus nerve stimulation in partial epilepsy. Neurology. (1999) 52:1166–73. doi: 10.1212/WNL.52.6.1166
131. Berthoud HR, Neuhuber WL. Functional and chemical anatomy of the afferent vagal system. Auton Neurosci. (2000) 85:1–17. doi: 10.1016/S1566-0702(00)00215-0
132. George MS, Nahas Z, Bohning DE, Mu Q, Kozel FA, Borckhardt J, et al. Mechanisms of action of vagus nerve stimulation (VNS). Clin Neurosci Res. (2004) 4:71–9. doi: 10.1016/j.cnr.2004.06.006
133. George MS, Sackeim HA, Marangell LB, Husain MM, Nahas Z, Lisanby SH, et al. Vagus nerve stimulation: A potential therapy for resistant depression? Psychiatr Clin North Am. (2000) 23:757–83. doi: 10.1016/S0193-953X(05)70196-9
134. George MS, Sackeim HA, Rush AJ, Marangell LB, Nahas Z, Husain MM, et al. Vagus nerve stimulation: a new tool for brain research and therapy. Biol Psychiatry. (2000) 47:287–95. doi: 10.1016/S0006-3223(99)00308-X
135. Walker BR, Easton A, Gale K. Regulation of limbic motor seizures by GABA and glutamate transmission in nucleus tractus solitarius. Epilepsia. (1999) 40:1051–7. doi: 10.1111/j.1528-1157.1999.tb00818.x
136. Chen SP, Ay I, Lopes De Morais A, Qin T, Zheng Y, Sadeghian H, et al. Vagus nerve stimulation inhibits cortical spreading depression. Pain. (2016) 157:797–805. doi: 10.1097/j.pain.0000000000000437
137. Follesa P, Biggio F, Gorini G, Caria S, Talani G, Dazzi L, et al. Vagus nerve stimulation increases norepinephrine concentration and the gene expression of BDNF and bFGF in the rat brain. Brain Res. (2007) 1179:28–34. doi: 10.1016/j.brainres.2007.08.045
138. Cheng K, Brodnick S, Blanz S, Zeng W, Kegel J, Pisaniello J, et al. Is vagus nerve stimulation brain washing? bioRxiv. (2019) 13:733410. doi: 10.1101/733410
139. Staats P, Giannakopoulos G, Blake J, Liebler E, Levy RM. The use of non-invasive vagus nerve stimulation to treat respiratory symptoms associated with COVID-19: a theoretical hypothesis and early clinical experience. Neuromodulation. (2020) 23:784–8. doi: 10.1111/ner.13172
140. Tornero C, Pastor E, Garzando MDM, Orduña J, Forner MJ, Bocigas I, et al. Non-invasive Vagus Nerve Stimulation for COVID-19: Results From a Randomized Controlled Trial (SAVIOR I). Front Neurol. (2022) 8:13. doi: 10.3389/fneur.2022.820864
141. Nemechek P, Antonelli G, Braida A. Transcutaneous vagus nerve stimulation is associated with lower mechanical ventilation and mortality in COVID-19 patients. J Emerg Dis Virol. (2021) 6:1–5. doi: 10.16966/2473-1846.165
142. Rangon CM, Barruet R, Mazouni A, le Cossec C, Thevenin S, Guillaume J, et al. Auricular neuromodulation for mass vagus nerve stimulation: insights from SOS COVID-19 a multicentric, randomized, controlled, double-blind french pilot study. Front Physiol. (2021) 2:12. doi: 10.3389/fphys.2021.704599
143. Ortolani C, Pastorello EA. Hydroxychloroquine and dexamethasone in COVID-19: who won and who lost? Clin Mol Allergy. (2020) 18:17. doi: 10.1186/s12948-020-00132-7
144. Carfì A, Bernabei R, Landi F. persistent symptoms in patients after acute COVID-19. JAMA. (2020) 324:603–5. doi: 10.1001/jama.2020.12603
145. Lefaucheur JP, Chalah MA, Mhalla A, Palm U, Ayache SS, Mylius V. The treatment of fatigue by non-invasive brain stimulation. Neurophysiol Clin. (2017) 47:173–84. doi: 10.1016/j.neucli.2017.03.003
146. Brunoni AR, Sampaio-Junior B, Moffa AH, Aparício L V, Gordon P, Klein I, et al. Noninvasive brain stimulation in psychiatric disorders: a primer. Braz J Psychiatry. (2019) 41:70–81. doi: 10.1590/1516-4446-2017-0018
147. Vicario CM, Salehinejad MA, Felmingham K, Martino G, Nitsche MA. A systematic review on the therapeutic effectiveness of non-invasive brain stimulation for the treatment of anxiety disorders. Neurosci Biobehav Rev. (2019) 96:219–31. doi: 10.1016/j.neubiorev.2018.12.012
148. Guo ZP, Sörös P, Zhang ZQ, Yang MH, Liao D, Liu CH. Use of Transcutaneous auricular vagus nerve stimulation as an adjuvant therapy for the depressive symptoms of COVID-19: a literature review. Front Psychiatry. (2021) 15:12. doi: 10.3389/fpsyt.2021.765106
149. Fudim M, Ali-Ahmed F, Patel MR, Sobotka PA. Sham trials: benefits and risks for cardiovascular research and patients. Lancet. (2019) 393:2104–6. doi: 10.1016/S0140-6736(19)31120-1
150. Farmer AD, Strzelczyk A, Finisguerra A, Gourine Av, Gharabaghi A, Hasan A, et al. International consensus based review and recommendations for minimum reporting standards in research on transcutaneous vagus nerve stimulation (Version 2020). Front Hum Neurosci. (2020) 14:568051. doi: 10.3389/fnhum.2020.568051
Keywords: vagus nerve (VN) stimulation, sacral nerve electrical stimulation, COVID-19, cytokine storm, acute respiratory distress (ARDS), cranial nerve stimulation, non-invasive
Citation: Czura CJ, Bikson M, Charvet L, Chen JDZ, Franke M, Fudim M, Grigsby E, Hamner S, Huston JM, Khodaparast N, Krames E, Simon BJ, Staats P and Vonck K (2022) Neuromodulation Strategies to Reduce Inflammation and Improve Lung Complications in COVID-19 Patients. Front. Neurol. 13:897124. doi: 10.3389/fneur.2022.897124
Received: 15 March 2022; Accepted: 25 May 2022;
Published: 14 July 2022.
Edited by:
Eugene Golanov, Houston Methodist Hospital, United StatesReviewed by:
Brandt D. Pence, University of Memphis, United StatesBruno Bonaz, Centre Hospitalier Universitaire de Grenoble, France
Copyright © 2022 Czura, Bikson, Charvet, Chen, Franke, Fudim, Grigsby, Hamner, Huston, Khodaparast, Krames, Simon, Staats and Vonck. This is an open-access article distributed under the terms of the Creative Commons Attribution License (CC BY). The use, distribution or reproduction in other forums is permitted, provided the original author(s) and the copyright owner(s) are credited and that the original publication in this journal is cited, in accordance with accepted academic practice. No use, distribution or reproduction is permitted which does not comply with these terms.
*Correspondence: Christopher J. Czura, Y2hyaXNjenVyYUBtYWMuY29t
†ORCID: Christopher J. Czura orcid.org/0000-0002-1347-6986
Marom Bikson orcid.org/0000-0002-0493-8333
Leigh Charvet orcid.org/0000-0003-4429-9713
Manfred Franke orcid.org/0000-0001-6638-5909
Marat Fudim orcid.org/0000-0002-8671-7007
Sam Hamner orcid.org/0000-0002-0465-4285
Jared M. Huston orcid.org/0000-0002-1393-801X
Navid Khodaparast orcid.org/0000-0003-4621-1295
Elliot Krames orcid.org/0000-0001-5374-022X
Bruce J. Simon orcid.org/0000-0002-4356-6093
Peter Staats orcid.org/0000-0002-4079-5165
Kristl Vonck orcid.org/0000-0002-2671-2650