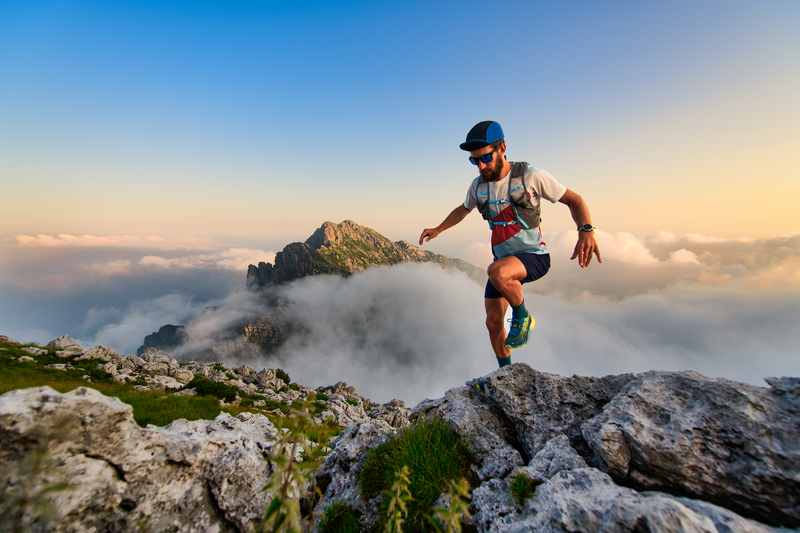
94% of researchers rate our articles as excellent or good
Learn more about the work of our research integrity team to safeguard the quality of each article we publish.
Find out more
REVIEW article
Front. Neurol. , 31 March 2022
Sec. Stroke
Volume 13 - 2022 | https://doi.org/10.3389/fneur.2022.842212
Gamma-delta (γδ) T cells are a small subset of T cells that are reported to have a proinflammatory role in the pathophysiology of cerebral ischemia stroke (CIS). Upon activation by interleukin-1 beta (IL-1β), IL-23 and IL-18, γδ T cells are stimulated to secrete various cytokines, such as IL-17a, IL-21, IL-22, and interferon-gamma (IFN-γ). In addition, they all play a pivotal role in the inflammatory and immune responses in ischemia. Nevertheless, the exact mechanisms responsible for γδ T cell proinflammatory functions remain poorly understood, and more effective therapies targeting at γδ T cells and cytokines they release remain to be explored, particularly in the context of CIS. CIS is the second most common cause of death and the major cause of permanent disability in adults worldwide. In this review, we focus on the neuroinflammatory and immune functions of γδ T cells and related cytokines, intending to understand their roles in CIS, which may be crucial for the development of novel effective clinical applications.
Stroke, a common disease, is the second leading cause of death and the third leading cause of disability in adults worldwide and places an increasing burden on families and communities (1). Stroke can be broadly divided into the ischemic and hemorrhagic stroke, and the former makes up ~87% of all stroke cases (2). Nowadays, clinical treatment of ischemic stroke is limited to interventions that restore blood flow through either pharmacological thrombolysis—tissue plasminogen activator (tPA), the only approved therapeutic agent—or mechanical thrombectomy. However, due to the moderate recanalization rate, limited time window, adverse effects, and some thrombolytic contraindications, only a small number of patients could receive and benefit from the therapies in time (3). Therefore, it is necessary to continue to explore the pathogenic mechanisms contributing to neurologic injury following a stroke to develop alternative therapeutic strategies.
Severe stenosis or occlusion of a cerebral artery, especially the middle cerebral artery, deprives nerve cells, including neurons and glial cells, of nutrition, such as oxygen, glucose, and lipids (4). Ischemia triggers a complex cascade of events that include energy failure (5), ion imbalance, excitotoxicity (6), oxidative stress (7), cell death, activation of microglia and complement system (8–10), and initiate the inflammation and immune responses. The cascades eventually lead to irreversible brain damage (11). After recanalizing successfully, restoring blood flowing to the ischemic brain causes secondary reperfusion injury. Reperfusion results in the production of reactive oxygen species (ROS) and the amplification of inflammation and immune responses, which subsequently cause undue neural death, impairment of the integrity of the blood-brain barrier (BBB), and activation of innate and adaptive immune systems, and eventually lead to brain damage even worse (12–15). In addition, the affected brain tissue also accumulates specific proinflammatory cells, especially neutrophils, macrophages, and T cells; Of which, T lymphocytes are one of the critical factors to the entire pathophysiology process of CIS (16, 17).
T lymphocytes develop from bone marrow-derived precursors and mature in the thymus after a complex developmental sequence associated with differentiation, selection, and proliferation episodes. They exert a central role in the adaptive immune system and are important in the crosstalk between innate and adaptive immune systems (18). T cells recognize antigens through T cell antigen receptor (TCR) composed of two distinct polypeptide chains (19), two possible pairs of which have been identified: TCRα and TCRβ, or TCRγ and TCRδ, defining αβ and γδ T cells, respectively. Certainly, all types of T cells are closely involved in inflammatory events in stroke (20). Notably, an interest in γδ T cells grows rapidly owing to their essential contributions to immunopathology in many diseases including CIS, therefore, γδ T cells are the focus of our review.
γδ T cells are divided according to the type of Vγ and Vδ chain they express at the TCRs. Of note, classification is one of the differences between human and mouse γδ T cells. In human, three main Vδ gene segments, Vδ1, Vδ2, and Vδ3, are most frequently used in the rearrangement of the δ chain, while seven functional Vγ gene segments, Vγ2, Vγ3, Vγ4, Vγ5, Vγ8, Vγ9, and Vγ11 are used for rearrangement of the γ chain (21). In human beings and Non-human primates along with a few other species, Vγ9Vδ2 T cells are the major γδ T cell subset and are the potential therapeutic target in many diseases (22, 23). While in mice γδ T cell subsets are named according to the Vγ chain used, which contains Vγ1, Vγ4, Vγ5, Vγ6, and Vγ7. Thus, the characteristics describing the γδ T cell subsets of one particular species cannot be applied to another species directly because each repertoire is unique (24). Hence, the experimental results obtained from murine studies need further validation before they are applied to clinical practices.
During fetal thymic development, γδ T cells are the first T cells to appear in the thymus. However, the relative proportion of γδ T cells decreases with the emergence and development of αβ T cells. In adult humans, γδ T cells make up 3–10% of T cells in the peripheral blood (25–27). Certain tissues, including lung, skin, thymus, lymph node, spleen, and breast, also find a similar frequency of γδ T cells (26, 28, 29). In comparison, γδ T cells can constitute up to 30% of all T cells in some compartments of the intestinal tract (30). While in adult mice, γδ T cells constitute 1–4% of total T cells in the blood, lymph node, liver, and spleen (31–33). Moreover, γδ T cells are also widespread within epithelial-rich tissues that form the inner and outer surfaces of the body, such as the reproductive tract, intestinal epithelial cells, and skin epidermis and dermis (34–38). They have the potential to be the source of γδ T cells infiltrating into the ischemic brain, which will be mentioned later.
Strikingly, a unique feature of murine γδ T cells is the preferential expression of different Vγ segments in different tissues. For example, Vγ5 + γδ T cells are present in the epidermis, Vγ7 + γδ T cells lie in the gut epithelia, Vγ6 + γδ T cells localize to the reproductive mucosa (39). Likewise, in humans, Vδ1 + T cells are a major subset in many tissues, such as thymus, spleen (40), breast (41), decidua (42), liver (43), lung, intestinal epithelia (44, 45) and skin dermis and epidermis (46), and Vγ9Vδ2 T cells are the major γδ T cell subset in the peripheral blood. Understanding of γδ T cell subtypes and their specific distribution fully and then developing therapies that target the specific cell subtype and their tissue specificity could increase effectiveness and reduce side effects of medicines or other treatments.
In the context of stroke, γδ T cells infiltrate into affected brain parenchyma and leptomeninges and participate in the inflammatory and immune responses in brain ischemic injury (39, 47). CC chemokine receptor 6 (CCR6) is required for the infiltration of IL-17-producing γδ T cells in experimental stroke. Genetic deficiency of CCR6 is associated with diminished infiltration of IL-17-producing γδ T cells and a significantly improved neurological outcome (48). In addition, dysbiosis in some tissues also influences the infiltration of γδ T cells. The article by Benakis et al. (47) made the point that intestinal dysbiosis altered immune homeostasis in the small intestine, d decreased the number of γδ T cells in the meninges, suppressed the function of effector IL-17-positive γδ T cells, and then affected ischemic stroke outcome. Inspired by the proximity, Brea et al. explored and found that nasal-associated lymphoid tissue (NALT) is not the source of stroke-associated IL-17a + γδ T cells (49). Whether γδ T cells can migrate from tissues with a high frequency of γδ T cells mentioned above, such as reproductive tract and skin dermis, remains to be further explored and confirmed. Moreover, the specific mechanisms leading to the migration of γδ T cells remain to be further elucidated. Recently, de Lima et al. (34) have found a high representation of γδ T cell receptor-expressing cells without expression of the conventional T cell coreceptors CD4 and CD8 in the dura mater. Of note, these cells can regulate anxiety-like behavior via IL-17a signaling. It is worth considering whether dura-associated γδ T cells are involved in the pathophysiology of CIS just like infiltrated γδ T cells; certainly, if they do, the mechanisms they work in CIS need to be explored.
γδ T cells express IL-23 receptor (IL-23R), IL-18R (50, 51). The receptors are combined with IL-23, IL-1β, and IL-18, which are produced from dendritic cells and infiltrated macrophages, rather than residential microglia, and then γδ T cells are stimulated to produce IL-17a, IL-21, IL-22, and IFN-γ, in the absence of TCR engagement (51–53). In addition, γδ T cells express Toll-like receptor (TLR) 7, TLR9, and the central TLR adapter molecular MyD88 intracellularly, and express TLR2 on the cell surface. However, upon TLR stimulation with TLR ligands, γδ T cells do not secret IL-17a (54). McCandless et al. (55) have reported that γδ T cells can secrete IL-1β in experimental autoimmune encephalomyelitis (EAE), and depletion of γδ T cells can decrease IL-1β levels. However, whether γδ T cells can secrete IL-1β in CIS has not been demonstrated.
Most of these molecules mentioned above are involved in the pathological process of ischemic brain injury. Besides, in the fields of anti-bacterial immunity and tumor immunity, γδ T cells can be activated by phosphoantigens (pAgs), which includes (E)-1-hydroxy-2-methyl-but-2enyl pyrophosphate (HMBPP), dimethylallyl pyrophosphate (DMAPP) and isopentyl pyrophosphate (IPP), through γδ TCR (56, 57). And butyrophilins (BTN)/butyrophilin-like molecules (BTNL) are core mediators of pAg sensing by γδ T cells (58). What's more, little research has focused on the signaling pathways that exist in γδ T cells intracellularly after activation through ligands combined with their receptors. The small amount, the difficulty to extract, and no cell line are characteristics of γδ T cells and may be why they are short of research. Noteworthy, these signaling pathways are worth studying to understand the pathogenic process in CIS more sufficiently and to develop more effective therapies.
γδ T cells exert an important effect on brain tissue injury in CIS not by themselves, but mainly by cytokines they release, including IL-17a, IL-21, IL-22, and IFN-γ. Each of them plays a unique role in the process of cerebral ischemia and reperfusion injury (Figure 1), including promoting BBB breakdown, neutrophil infiltration, neuronal cell apoptosis and autophagy, and so on, resulting in unreversible brain damage.
Figure 1. Activation and effects of γδ T cells in the pathophysiology process of CIS. Upon activated by IL-23, IL-1β and IL-18, infiltrated γδ T cells are stimulated to produce IL-17a, IL-21, IL-22, and IFN-γ. They participate in the pathophysiological process of CIS, involving promoting BBB breakdown, neutrophil infiltration, neuronal cell apoptosis and autophagy, and so on, leading to affected brain tissue damage and neurological deficit.
It has been reported that in both experimental models and patients, IL-17a is augmented in brain tissue and peripheral blood after CIS. γδ T cells are the main sources of IL-17a in the context of stroke (52). Other innate and adaptive immune cells, including Th17 cells, natural killer T (NKT) cells, natural killer (NK) cells, group 3 innate lymphoid cells (ILC3s), and neutrophils have been found to secrete IL-17a as well (59, 60). As previously reported, IL-17a-releasing γδ T cells peak on day 3 after the onset of ischemia, and IL-17a seems to have a crucial role in the maturation of brain infarction (59).
Firstly, IL-17a could promote neutrophil infiltration. By binding with IL-17R, which is expressed on glial cells and brain microvascular endothelial cells (BMECs) and is up-regulated after stroke in experimental models, IL-17a promotes glia and BMECs to secret and activate large amounts of CXCL1; CXCL1 is a neutrophil chemoattractant that could lead neutrophils to infiltrate into the affected cerebral parenchyma (61–65). Moreover, augmented infiltrating neutrophils can further destroy the integrity of the blood-brain barrier (BBB), and promote the lysis and apoptosis of nerve cells, as a result, to aggravate brain injury (18, 66, 67).
Secondly, IL-17a could destroy the structural integrity of BBB. Huppert et al. (68) have found that IL-17a could induce ROS production so that to down-regulate the expression of tight junction (TJ) molecule occluding. Ni et al. (69) have verified that IL-17a could elevate matrix metalloproteinase (MMP)-2, MMP-3, and MMP-9 in BMECs to hydrolyze TJs, leading to increased BBB permeability. Zhu et al. (70) have uncovered that IL-17a induced the production of von Willebrand factor by endothelial cells, and promoted endothelial cells apoptosis by activating caspase-3 and caspase-9 and up-regulating the ratio of Bcl-2-associated X protein (Bax)/B cell lymphoma/leukemia-2 (Bcl-2). However, the aforementioned damaging effect of IL-17a on BBB has only been confirmed in other models of brain inflammatory diseases, and the effect needs to be verified in CIS.
Thirdly, IL-17a promotes neuron death, including apoptosis and autophagy. IL-17a facilitates the expression of apoptosis-related proteins including caspase-3, caspase-9, and Bax, and increases the ratio of Bax/Bcl-2 after brain injury, thus resulting in neuron apoptosis (71). Liu et al. (72) have also found that IL-17a could mediate excessive autophagy to aggravate neuronal ischemic injuries via the Src-PP2B-mTOR pathway.
All the damaging effects above lead to larger infarction and worse outcomes. Likewise, IL-17a and γδ T cells have also been demonstrated to be implicated in human stroke. Infiltration of γδ T cells and secretion of IL-17a have been documented in ischemic human brain tissue and circulating IL-17a is elevated as well (53, 61, 73). Later, clinical research is needed to verify the role of the γδ T cell-IL-17a axis in patients suffering from ischemic stroke and potential mechanisms, so that to develop more effective therapies applied to clinical practice.
Noteworthy, IL-17a plays a dual role at different time points after ischemic stroke in mice. The article by Lin et al. makes the point that IL-17a showed two apparent peaks of expression in the ischemic hemisphere: one occurring within 3 days, which is secreted from γδ T cells and has detrimental roles in the pathogenesis of acute ischemic stroke as mentioned above, and the other on 28 d after stroke. And astrocytes are the major cellular source of the second peak of IL-17a that has a property in the maintenance and augment of survival and neuronal differentiation of subventricular zone (SVZ) neural precursor cells (NPCs), and subsequent synaptogenesis and functional recovery after ischemic stroke (74). Whether promoting IL-17a releasing or exogenous administration of IL-17a during convalescence improves stroke outcomes remains to be explored and verified.
In a mouse model of transient middle cerebral artery occlusion (tMCAO), IL-21 is robust up-regulated in the injured brain, and IL-21 exerts a pronounced effect on brain injury via up-regulating autophagy-related genes of neuronal cells which express IL-21R. In addition, in postmortem human brain tissue, IL-21 was also found in the area surrounding acute stroke lesions, suggesting that IL-21-mediated brain injury may be relevant to human stroke (75). IL-21 gene owns two polymorphisms, rs907715G/A and rs4833837A/G, and the former causes the augment of IL-21 mRNA and protein levels in peripheral blood mononuclear cells (PBMCs) and shows a positive correlation with brain ischemic injury; thus, IL-21 may be important in the development of the disease (76). Therefore, there is a potential for regulation at the protein level, even genetic level, to prevent and mitigate ischemic stroke in the brain.
Surprisingly, Weiner et al. (77) and Lee et al. (78) found that IL-21R exerted a neuroprotective effect via Janus tyrosine kinase (JAK)/signal transducer and activator of transcription (STAT) signaling pathways and upregulation of caspase-3, and neuronal cell death and infarct volume increased in IL-21R-deficient mice suffered from ischemia compared with the control group. Given that the protective effect of IL-21R was more evident in permanent middle cerebral artery occlusion (pMCAO) than in tMCAO, and T lymphocytes infiltration, which was the source of IL-21, was obvious in tMCAO while poor in pMCAO, the reason for the difference above may be the source of IL-21 and IL-21R, resident or infiltration, and maybe also related to a collateral-independent effect on cerebral injury (78). Therefore, the exact role of IL-21 in CIS requires more evidence to elucidate.
IL-22 exerts a protective effect against CIS. Dong et al. have found that injecting intraperitoneally with recombinant mouse IL-22 protein (rIL-22) into mice could reduce the expression of inflammatory cytokines, including IL-1β, monocyte chemotactic protein (MCP)-1 and tumor necrosis factor (TNF)-α, both in the serum and the ischemic cerebral cortex. In addition, IL-22 treatment also decreased oxidative stress and neuronal apoptosis in affected brain tissue. Moreover, treatment with IL-22 significantly increased JAK2 and STAT3 phosphorylation levels in mice and PC12 cells. The effects mentioned above lead to the reduction of infarct size, neurological deficits, and brain water content in mice subjected to CIS (79). There is a problem that IL-22 is administered exogenously, but not γδ T cell-derived. The research about the role of γδ T cell-derived IL-22 in brain ischemic stroke is rare, and the neuroprotective effect of IL-22 in stroke needs more evidence to verify.
In mice suffering from stroke, Th1 cells are the main source of IFN-γ, and a very few γδ T cells could produce IFN-γ (52). Similarly, using a fate-tracking system, Hirota et al. (80) have found that <20% of γδ T cells were shown to express IFN-γ in the central nervous system (CNS) in the context of EAE. The same as the IL-17a, IFN-γ-producing cells strongly accumulate by day 3 after ischemia in affected brain tissue and decrease thereafter (52). Certainly, IFN-γ participates in the pathophysiological process of CNS-related diseases. In some central nervous systems (CNS)-related diseases, for example, spinal cord injury (SCI), γδ T cells are detected at the lesion sites and express the inflammatory cytokine IFN-γ, which induces macrophages to transform into M1 phenotype with increased secretion of proinflammatory cytokines, such as TNF-α (81). Likewise, the article by Gelderblom et al. makes the point that IFN-γ produced by CD4+ T cells could induce TNF-α production from macrophages. And synergistic with TNF-α, IL-17a could enhance astrocytes to secrete CXCL1, leading to enhanced neutrophil infiltration in a mouse model of stroke (61). Blocking IFN-γ or inactivating Vγ4+ γδ T cells with antibodies has a beneficial effect and improves the functional recovery after SCI (81). Therefore, manipulation of γδ T cell and IFN-γ functions may be a promising approach for CIS treatment in the future.
On one hand, through combination with IL-18R expressed on γδ T cells, IL-1β are involved to stimulate IL-17a production by γδ T cells (51). On the other hand, IL-1β may be secreted by γδ T cells (55). As reported by Mccandless et al., in the rodent model of multiple sclerosis (MS), EAE, the inflammatory cytokine IL-1β, γδ T cells are one of the sources of which, participates in the pathogenic process through mediating pathologic relocation of CXCL12 and disruption of BBB (55). In addition, IL-1β is involved in perpetuating immune responses and contributing to disease severity in a variety of CNS diseases, such as neurodegenerative diseases, traumatic brain injury, and diabetic retinopathy (82). Moreover, it is beneficial to block IL-1β signaling in some autoimmune and autoinflammatory diseases, making IL-1β a potential therapeutic target in neuroinflammatory conditions, including CIS. However, the evidence that γδ T cells secrete IL-1β is particularly rare, and more research is needed to support this.
These five cytokines mentioned above that play a role in brain stroke are released partly from T lymphocytes, but not specifically from γδ T cells, thus, there remains a lot of research to be done to confirm the role of the specific γδ T cell-derived cytokines in cerebral ischemia and reperfusion injury to further improve the mechanisms.
γδ T cells and related cytokines have essential roles in ischemic brain injury. Treatments targeting γδ T cells and their cytokines could be good therapeutic targets for mitigating ischemic brain damage, given the truth that either genetic disruption or pharmacological blockade of γδ T cells, IL-17a or IL-21 shows a significant neuroprotective effect on ischemic brain damage in murine stroke (Table 1). Of note, in other neuroinflammatory diseases, blockade of IFN-γ and IL-1β signaling also have a neuroprotective effect, while their beneficial effect has not been verified in CIS. In addition, through modifying the composition of the gut microbiota, Benakis et al. found that intestinal dysbiosis could reduce ischemic brain injury. The mechanism was shown to be related to a reduction of IL-17-positive γδ T cells and an increase of regulatory T (Treg) cells through altering dendritic cell activity. And dysbiosis could suppress the trafficking of effector T cells from the gut to the leptomeninges after stroke. The findings uncovered a gut-brain axis, which was unrecognized previously, and an impact of the intestinal flora and meningeal IL-17+ γδ T cells on ischemic brain damage (47). Inspired by this article, there remains a possibility that diseases with altering immune homeostases, such as inflammatory bowel disease (IBD), Crohn's disease (CD), and systemic lupus erythematosus (SLE), could affect ischemic stroke outcomes by impacting the trafficking of γδ T cells into ischemic brain tissue.
Nevertheless, in human stroke, the evidence that treatments targeting γδ T cells and cytokines they release are beneficial to the outcomes is rare. Caccamo et al. (91) have found that in the peripheral blood and at the site of disease in children with bacterial meningitis, the percentage of IL-17+Vγ9Vδ2 T lymphocytes was increased, while this pattern was reversed after successful antibacterial therapy. This article indicated that IL-17+γδ T cells participated in the neurological disease, and γδ T cells might be the target of the antibiotic therapy. Moreover, blockade of the proinflammatory cytokine IL-17a with secukinumab, a fully human selective anti-IL-17a monoclonal antibody, is a valid therapeutic approach and may be useful in the treatment of psoriasis, Rheumatoid Arthritis (RA), and noninfectious uveitis (92). Whereupon, γδ T cells and cytokines they release are therapeutic targets with great potential and are worth studying.
However, there is controversy over the efficacy of therapies targeting γδ T cells and related cytokines. Adamski et al. (93) did not find associations of γδ T cell counts with lesion volume, stroke severity, and outcome in the clinical study. However, it does not mean that γδ T cells do not exert effects on human stroke. And a large patient sample is needed to verify their associations. Meanwhile, several anti-IL-17a drugs are in clinical trials for some inflammatory disorders, such as IBD and CD. Targeted inhibition of IL-17a by secukinumab is ineffective in patients with moderate to severe CD and adverse events are noted compared with placebo (94). A separate line of evidence also reports that IL-17a acts on intestinal epithelium to promote barrier function, whereas IL-17a or IL-17 receptor A (IL-17RA) inhibition promotes severe weakening of the barrier, culminating in increased colonic inflammation and accelerated mortality (95). Similar results obtained report that IL-17 regulates occludin protein that limits gut excessive permeability and maintains barrier integrity during an epithelial injury in a dextran sodium sulfate (DSS) model of IBD, while neutralizing IL-17 causes increased gut permeability (96), further confirming the deleterious effects of neutralizing IL-17a and IL-17RA.
The dichotomy in the efficacy of therapies targeting γδ T cells and related cytokines does exist. Since then, there is a long way to go to verify that γδ T cells and related cytokines are promising therapeutic targets in patients attacked by CIS.
CIS is an increasing threat to endanger health and safety all over the world. A better understanding of relations between brain tissue damage after ischemic stroke and inflammatory and immune responses remains to be improved to shed light on the development of more effective therapies in the future. γδ T cells, a small subset of T cells, regulate the inflammation process in many diseases, including CIS. After the onset of ischemic stroke, upon activation, γδ T cells can release IL-17a, IL-21, IL-22, and IFN-γ, and then participated in the pathogenic process, including secretion of pro-inflammatory factors, breakdown of the integrity of BBB, and recruitment of inflammatory cells into the affected tissues, and eventually cause irreversible brain injury. A variety of researches have demonstrated that suppressing the infiltration of γδ T cells and reduction of IL-17a and IL-21 levels via either pharmacological or genetic tools could improve the outcome of stroke. Noteworthy, our experiments aim to benefit patients attacked by stroke, hence more evidence is required to confirm whether Pre-clinical pathogenic mechanisms mentioned above are also appropriate to patients suffering from CIS. This is to provide a more sufficient basis for clinical application. In conclusion, γδ T cells and related cytokines play a vital role in the stroke and may be therapeutic targets with great potential for treatment.
LW and CY contributed to editing the manuscript and discussed the topic and structure of this article together. SY and YL provided administrative support. JC, YG, CW, YW, FW, YS, and MD helped with the manuscript editing and discussions. All authors contributed to the review and approved the submitted version.
This work was supported by the Major Technological Innovation Special Project of Hubei Province of China (2019ACA167) 2019 College-level Teaching Reform Research Project (02.03.2019.15-15).
The authors declare that the research was conducted in the absence of any commercial or financial relationships that could be construed as a potential conflict of interest.
All claims expressed in this article are solely those of the authors and do not necessarily represent those of their affiliated organizations, or those of the publisher, the editors and the reviewers. Any product that may be evaluated in this article, or claim that may be made by its manufacturer, is not guaranteed or endorsed by the publisher.
We wish to thank Ruijie Niu for her critical comments and encouragement.
1. Collaborators TG 2016 LR of S. Global, regional, and country-specific lifetime risks of stroke, 1990 and 2016. New Engl J Med. (2018) 379:2429–37. doi: 10.1056/NEJMoa1804492
2. Benjamin EJ, Virani SS, Callaway CW, Chamberlain AM, Chang AR, Cheng S, et al. Heart disease and stroke statistics-−2018 update. Circulation. (2018) 137:e67–e492. doi: 10.1161/CIR.0000000000000558
3. Yeo LLL, Paliwal P, Teoh HL, Seet RC, Chan BPL, Liang S, et al. Timing of recanalization after intravenous thrombolysis and functional outcomes after acute ischemic stroke. Jama Neurol. (2013) 70:353–8. doi: 10.1001/2013.jamaneurol.547
4. Shichita T, Sakaguchi R, Suzuki M, Yoshimura A. Post-ischemic inflammation in the brain. Front Immunol. (2012) 3:132. doi: 10.3389/fimmu.2012.00132
5. Karaszewski B, Wardlaw JM, Marshall I, Cvoro V, Wartolowska K, Haga K, et al. Early brain temperature elevation and anaerobic metabolism in human acute ischaemic stroke. Brain. (2009) 132:955–64. doi: 10.1093/brain/awp010
6. Castillo J, Loza MI, Mirelman D, Brea J, Blanco M, Sobrino T, et al. A novel mechanism of neuroprotection: blood glutamate grabber. J Cereb Blood Flow Metabolism. (2015) 36:292–301. doi: 10.1177/0271678X15606721
7. Chan PH. Reactive oxygen radicals in signaling and damage in the ischemic brain. J Cereb Blood Flow Metabolism. (2001) 21:2–14. doi: 10.1097/00004647-200101000-00002
8. Peerschke EI, Yin W, Ghebrehiwet B. Complement activation on platelets: implications for vascular inflammation and thrombosis. Mol Immunol. (2010) 47:2170–5. doi: 10.1016/j.molimm.2010.05.009
9. Mocco J, Mack WJ, Ducruet AF, Sosunov SA, Sughrue ME, Hassid BG, et al. Complement component C3 mediates inflammatory injury following focal cerebral ischemia. Circ Res. (2006) 99:209–17. doi: 10.1161/01.RES.0000232544.90675.42
10. Peerschke BEIB, Reid KBM, Ghebrehiwet B. Platelet activation by Clq results in the induction of C Ub//33 Integrins (GPIlb-rlla) and the expression of P-Selectin and procoagulant activity. J Exp Med. 178:579–87. doi: 10.1084/jem.178.2.579
11. Iadecola C, Anrather J. The immunology of stroke: from mechanisms to translation. Nat Med. (2011) 17:796–808. doi: 10.1038/nm.2399
12. Kevil CG, Oshima T, Alexander B, Coe LL, Alexander JS. H2O2-mediated permeability: role of MAPK and occludin. Am J Physiol-cell Ph. (2000) 279:C21–30. doi: 10.1152/ajpcell.2000.279.1.C21
13. Peters O, Back T, Lindauer U, Busch C, Megow D, Dreier J, et al. Increased formation of reactive oxygen species after permanent and reversible middle cerebral artery occlusion in the rat. J Cereb Blood Flow Metabolism. (1997) 18:196–205. doi: 10.1097/00004647-199802000-00011
14. Allen CL, Bayraktutan U. Oxidative stress and its role in the pathogenesis of ischaemic stroke. Int J Stroke. (2009) 4:461–70. doi: 10.1111/j.1747-4949.2009.00387.x
15. Kelly PJ, Morrow JD, Ning M, Koroshetz W, Lo EH, Terry E, et al. Oxidative stress and matrix metalloproteinase-9 in acute ischemic stroke. Stroke. (2008) 39:100–4. doi: 10.1161/STROKEAHA.107.488189
16. Gelderblom M, Leypoldt F, Steinbach K, Behrens D, Choe C-U, Siler DA, et al. Temporal and spatial dynamics of cerebral immune cell accumulation in stroke. Stroke. (2009) 40:1849–57. doi: 10.1161/STROKEAHA.108.534503
17. Yilmaz G, Arumugam TV, Stokes KY, Granger DN. Role of T lymphocytes and interferon-γ in ischemic stroke. Circulation. (2006) 113:2105–12. doi: 10.1161/CIRCULATIONAHA.105.593046
18. Gu L, Jian Z, Stary C, Xiong X. T Cells and cerebral ischemic stroke. Neurochem Res. (2015) 40:1786–91. doi: 10.1007/s11064-015-1676-0
19. Wucherpfennig KW, Gagnon E, Call MJ, Huseby ES, Call ME. Structural biology of the T-cell receptor: insights into receptor assembly, ligand recognition, and initiation of signaling. Csh Perspect Biol. (2010) 2:a005140. doi: 10.1101/cshperspect.a005140
20. Bravo-Alegria J, McCullough LD, Liu F. Sex differences in stroke across the lifespan: the role of T lymphocytes. Neurochem Int. (2017) 107:127–37. doi: 10.1016/j.neuint.2017.01.009
21. Adams EJ, Gu S, Luoma AM. Human gamma delta T cells: evolution and ligand recognition. Cell Immunol. (2015) 296:31–40. doi: 10.1016/j.cellimm.2015.04.008
22. Pauza CD, Liou M-L, Lahusen T, Xiao L, Lapidus RG, Cairo C, et l. Gamma delta T cell therapy for cancer: it is good to be local. Front Immunol. (2018) 9:1305. doi: 10.3389/fimmu.2018.01305
23. Karunakaran MM, Göbel TW, Starick L, Walter L, Herrmann T. Vγ9 and Vδ2 T cell antigen receptor genes and butyrophilin 3 (BTN3) emerged with placental mammals and are concomitantly preserved in selected species like alpaca (Vicugna pacos). Immunogenetics. (2014) 66:243–54. doi: 10.1007/s00251-014-0763-8
24. Shiromizu CM, Jancic CC. γδ T lymphocytes: an effector cell in autoimmunity and infection. Front Immunol. (2018) 9:2389. doi: 10.3389/fimmu.2018.02389
25. Lanier LL, Ruitenberg J, Bolhuls RLH, Borst J, Phillis JH, Testi R. Structural and serological heterogeneity of γ/δ T cell antigen receptor expression in thymus and peripherar blood. Eur J Immunol. (1988) 18:1985–92. doi: 10.1002/eji.1830181218
26. Groh V, Porcelli S, Fabbi M, Lanier LL, Picker LJ, Anderson T, et al. Human lymphocytes bearing T cell receptor gamma/delta are phenotypically diverse and evenly distributed throughout the lymphoid system. J Exp Medicine. (1989) 169:1277–94. doi: 10.1084/jem.169.4.1277
27. Esin S, Shigematsu M, Nagai S, Eklund A, Wigzell H, Grunewald J. Different percentages of peripheral blood γδ+ T cells in healthy individuals from different areas of the world. Scand J Immunol. (1996) 43:593–6. doi: 10.1046/j.1365-3083.1996.d01-79.x
28. Parker CM, Groh V, Band H, Porcelli SA, Morita C, Fabbi M, et al. Evidence for extrathymic changes in the T cell receptor gamma/delta repertoire. J Exp Medicine. (1990) 171:1597–612. doi: 10.1084/jem.171.5.1597
29. Papadopoulou M, Sanchez GS, Vermijlen D. Innate and adaptive γδ T cells: how, when, and why. Immunol Rev. (2020) 298:99–116. doi: 10.1111/imr.12926
30. Mayassi T, Jabri B. Human intraepithelial lymphocytes. Mucosal Immunol. (2018) 11:1281–9. doi: 10.1038/s41385-018-0016-5
31. Kohlgruber AC, Gal-Oz ST, LaMarche NM, Shimazaki M, Duquette D, Koay H-F, et al. γδ T cells producing interleukin-17A regulate adipose regulatory T cell homeostasis and thermogenesis. Nat Immunol. (2018) 19:464–74. doi: 10.1038/s41590-018-0094-2
32. Chen H, Eling N, Martinez-Jimenez CP, O'Brien LM, Carbonaro V, Marioni JC, et al. IL-7-dependent compositional changes within the γδ T cell pool in lymph nodes during ageing lead to an unbalanced anti-tumour response. Embo Rep. (2019) 20:e47379. doi: 10.15252/embr.201847379
33. Sandrock I, Zietara N, Łyszkiewicz M, Oberdörfer L, Witzlau K, Krueger A, et al. MicroRNA-181a/b-1 is not required for innate γδ NKT effector cell development. PLoS One. (2015) 10:e0145010. doi: 10.1371/journal.pone.0145010
34. Lima KA de, Rustenhoven J, Mesquita SD, Wall M, Salvador AF, Smirnov I, et al. Meningeal γδ T cells regulate anxiety-like behavior via IL-17a signaling in neurons. Nat Immunol. (2020) 21:1421–9. doi: 10.1038/s41590-020-0776-4
35. Itohara S, Farr AG, Lafaille JJ, Bonneville M, Takagaki Y, Haas W, et al. Homing of a γδ thymocyte subset with homogeneous T-cell receptors to mucosal epithelia. Nature. (1990) 343:754–7. doi: 10.1038/343754a0
36. Goodman T, Lefrancois L. Intraepithelial lymphocytes. Anatomical site, not T cell receptor form, dictates phenotype and function. J Exp Medicine. (1989) 170:1569–81. doi: 10.1084/jem.170.5.1569
37. Gray EE, Suzuki K, Cyster JG. Cutting edge: identification of a motile IL-17–producing γδ T cell population in the dermis. J Immunol. (2011) 186:6091–5. doi: 10.4049/jimmunol.1100427
38. Havran WL, Allison JP. Origin of Thy-1+ dendritic epidermal cells of adult mice from fetal thymic precursors. Nature. (1990) 344:68–70. doi: 10.1038/344068a0
39. Wo J, Zhang F, Li Z, Sun C, Zhang W, Sun G. The role of gamma-delta T cells in diseases of the central nervous system. Front Immunol. (2020) 11:580304. doi: 10.3389/fimmu.2020.580304
40. Inghirami G, Zhu BY, Chess L, Knowles DM. Flow cytometric and immunohistochemical characterization of the gamma/delta T-lymphocyte population in normal human lymphoid tissue and peripheral blood. Am J Pathology. (1990) 136:357–67.
41. Wu Y, Kyle-Cezar F, Woolf RT, Naceur-Lombardelli C, Owen J, Biswas D, et al. An innate-like Vδ1+ γδ T cell compartment in the human breast is associated with remission in triple-negative breast cancer. Sci Transl Med. (2019) 11:eaax9364. doi: 10.1126/scitranslmed.aax9364
42. Terzieva A, Dimitrova V, Djerov L, Dimitrova P, Zapryanova S, Hristova I, et al. Early pregnancy human decidua is enriched with activated, fully differentiated and pro-inflammatory gamma/delta T Cells with diverse TCR repertoires. Int J Mol Sci. (2019) 20:687. doi: 10.3390/ijms20030687
43. Hunter S, Willcox CR, Davey MS, Kasatskaya SA, Jeffery HC, Chudakov DM, et al. Human liver infiltrating γδ T cells are composed of clonally expanded circulating and tissue-resident populations. J Hepatol. (2018) 69:654–5. doi: 10.1016/j.jhep.2018.05.007
44. Mikulak J, Oriolo F, Bruni E, Roberto A, Colombo FS, Villa A, et al. NKp46-expressing human gut-resident intraepithelial Vδ1 T cell subpopulation exhibits high anti-tumor activity against colorectal cancer. Jci Insight. (2019) 4:e125884. doi: 10.1172/jci.insight.125884
45. Dunne MR, Elliott L, Hussey S, Mahmud N, Kelly J, Doherty DG, et al. Persistent changes in circulating and intestinal γδ T cell subsets, invariant natural killer T cells and mucosal-associated invariant T cells in children and adults with coeliac disease. PLoS One. (2013) 8:e76008. doi: 10.1371/journal.pone.0076008
46. Daniels J, Doukas PG, Escala MEM, Ringbloom KG, Shih DJH, Yang J, et al. Cellular origins and genetic landscape of cutaneous gamma delta T cell lymphomas. Nat Commun. (2020) 11:1806. doi: 10.1038/s41467-020-15572-7
47. Benakis C, Brea D, Caballero S, Faraco G, Moore J, Murphy M, et al. Commensal microbiota affects ischemic stroke outcome by regulating intestinal γδ T cells. Nat Med. (2016) 22:516–23. doi: 10.1038/nm.4068
48. Arunachalam P, Ludewig P, Melich P, Arumugam TV, Gerloff C, Prinz I, et al. CCR6 (CC Chemokine Receptor 6) is essential for the migration of detrimental natural Interleukin-17–producing γδ T cells in stroke. Stroke. (2017) 48:1957–65. doi: 10.1161/STROKEAHA.117.016753
49. Brea D, Poon C, Murphy M, Lubitz G, Iadecola C, Anrather J. Ablation of nasal-associated lymphoid tissue does not affect focal ischemic brain injury in mice. PLoS One. (2018) 13:e0205470. doi: 10.1371/journal.pone.0205470
50. Sutton CE, Lalor SJ, Sweeney CM, Brereton CF, Lavelle EC, Mills KHG. Interleukin-1 and IL-23 induce innate IL-17 production from γδ T Cells, amplifying Th17 responses and autoimmunity. Immunity. (2009) 31:331–41. doi: 10.1016/j.immuni.2009.08.001
51. Lalor SJ, Dungan LS, Sutton CE, Basdeo SA, Fletcher JM, Mills KHG. Caspase-1–processed cytokines IL-1β and IL-18 promote IL-17 production by γδ and CD4 T cells that mediate autoimmunity. J Immunol. (2011) 186:5738–48. doi: 10.4049/jimmunol.1003597
52. Shichita T, Sugiyama Y, Ooboshi H, Sugimori H, Nakagawa R, Takada I, et al. Pivotal role of cerebral interleukin-17–producing γδT cells in the delayed phase of ischemic brain injury. Nat Med. (2009) 15:946–50. doi: 10.1038/nm.1999
53. Kostulas N, Pelidou SH, Kivisakk P, Kostulas V, Link H. Increased IL-1β, IL-8, and IL-17 mRNA expression in blood mononuclear cells observed in a prospective ischemic stroke study. Stroke. (1999) 30:2174–9. doi: 10.1161/01.STR.30.10.2174
54. Derkow K, Krüger C, Dembny P, Lehnardt S. Microglia induce neurotoxic IL-17+ γδ T cells dependent on TLR2, TLR4, and TLR9 activation. PLoS One. (2015) 10:e0135898. doi: 10.1371/journal.pone.0135898
55. McCandless EE, Budde M, Lees JR, Dorsey D, Lyng E, Klein RS. IL-1R signaling within the central nervous system regulates CXCL12 expression at the blood-brain barrier and disease severity during experimental autoimmune encephalomyelitis. J Immunol. (2009) 183:613–20. doi: 10.4049/jimmunol.0802258
56. Tanaka Y, Morita CT, Tanaka Y, Nieves E, Brenner MB, Bloom BR. Natural and synthetic Non-peptide antigens recognized by human γδ T cells. Nature. (1995) 375:155–8. doi: 10.1038/375155a0
57. Hintz M, Reichenberg A, Altincicek B, Bahr U, Gschwind RM, Kollas A-K, et al. Identification of (E)-4-hydroxy-3-methyl-but-2-enyl pyrophosphate as a major activator for human γδ T cells in Escherichia coli. Febs Lett. (2001) 509:317–22. doi: 10.1016/S0014-5793(01)03191-X
58. Uldrich AP, Rigau M, Godfrey DI. Immune recognition of phosphoantigen-butyrophilin molecular complexes by γδ T cells. Immunol Rev. (2020) 298:74–83. doi: 10.1111/imr.12923
59. Zhang Q, Liao Y, Liu Z, Dai Y, Li Y, Li Y, et al. Interleukin-17 and ischaemic stroke. Immunology. (2021) 162:179–93. doi: 10.1111/imm.13265
60. Veldhoen M. Interleukin 17 is a chief orchestrator of immunity. Nat Immunol. (2017) 18:612–21. doi: 10.1038/ni.3742
61. Gelderblom M, Weymar A, Bernreuther C, Velden J, Arunachalam P, Steinbach K, et al. Neutralization of the IL-17 axis diminishes neutrophil invasion and protects from ischemic stroke. Blood. (2012) 120:3793–802. doi: 10.1182/blood-2012-02-412726
62. Sun H, Zhong D, Jin J, Liu Q, Wang H, Li G. Upregulation of miR-215 exerts neuroprotection effects against ischemic injury via negative regulation of Act1/IL-17RA signaling. Neurosci Lett. (2018) 662:233–41. doi: 10.1016/j.neulet.2017.10.046
63. Wojkowska DW, Szpakowski P, Glabinski A. Interleukin 17A promotes lymphocytes adhesion and induces CCL2 and CXCL1 release from brain endothelial cells. Int J Mol Sci. (2017) 18:1000. doi: 10.3390/ijms18051000
64. Hermann DM, Kleinschnitz C, Gunzer M. Implications of polymorphonuclear neutrophils for ischemic stroke and intracerebral hemorrhage: predictive value, pathophysiological consequences and utility as therapeutic target. J Neuroimmunol. (2018) 321:138–43. doi: 10.1016/j.jneuroim.2018.04.015
65. Hermann DM, Kleinschnitz C, Gunzer M. Role of polymorphonuclear neutrophils in the reperfused ischemic brain: insights from cell-type-specific immunodepletion and fluorescence microscopy studies. Ther Adv Neurol Diso. (2018) 11:1756286418798607. doi: 10.1177/1756286418798607
66. Lim K, Hyun Y-M, Lambert-Emo K, Capece T, Bae S, Miller R, et al. Neutrophil trails guide influenza-specific CD8+ T cells in the airways. Science. (2015) 349:aaa4352–aaa4352. doi: 10.1126/science.aaa4352
67. Ruhnau J, Schulze J, Dressel A, Vogelgesang A. Thrombosis, neuroinflammation, and poststroke infection: the multifaceted role of neutrophils in stroke. J Immunol Res. (2017) 2017:1–7. doi: 10.1155/2017/5140679
68. Huppert J, Closhen D, Croxford A, White R, Kulig P, Pietrowski E, et al. Cellular mechanisms of IL-17-induced blood-brain barrier disruption. Faseb J. (2010) 24:1023–34. doi: 10.1096/fj.09-141978
69. Ni P, Dong H, Wang Y, Zhou Q, Xu M, Qian Y, et al. IL-17A contributes to perioperative neurocognitive disorders through blood-brain barrier disruption in aged mice. J Neuroinflamm. (2018) 15:332. doi: 10.1186/s12974-018-1374-3
70. Zhu F, Wang Q, Guo C, Wang X, Cao X, Shi Y, et al. IL-17 induces apoptosis of vascular endothelial cells — a potential mechanism for human acute coronary syndrome. Clin Immunol. (2011) 141:152–60. doi: 10.1016/j.clim.2011.07.003
71. Li T, Zhang Y, Han D, Hua R, Guo B, Hu S, et al. Involvement of IL-17 in secondary brain injury after a traumatic brain injury in rats. Neuromol Med. (2017) 19:541–54. doi: 10.1007/s12017-017-8468-4
72. Liu T, Han S, Dai Q, Zheng J, Liu C, Li S, et al. IL-17A-mediated excessive autophagy aggravated neuronal ischemic injuries via Src-PP2B-mTOR pathway. Front Immunol. (2019) 10:2952. doi: 10.3389/fimmu.2019.02952
73. Li G-Z, Zhong D, Yang L-M, Sn B, Zhong Z-H, Yin Y-H, et al. Expression of Interleukin-17 in ischemic brain tissue. Scand J Immunol. (2005) 62:481–6. doi: 10.1111/j.1365-3083.2005.01683.x
74. Lin Y, Zhang J-C, Yao C-Y, Wu Y, Abdelgawad AF, Yao S-L, et al. Critical role of astrocytic interleukin-17 A in Post-stroke survival and neuronal differentiation of neural precursor cells in adult mice. Cell Death Dis. (2016) 7:e2273. doi: 10.1038/cddis.2015.284
75. Clarkson BDS, Ling C, Shi Y, Harris MG, Rayasam A, Sun D, et al. T cell–derived interleukin (IL)-21 promotes brain injury following stroke in mice. J Exp Medicine. (2014) 211:595–604. doi: 10.1084/jem.20131377
76. Li G, Xu R, Cao Y, Xie X, Zheng Z. Interleukin-21 polymorphism affects gene expression and is associated with risk of ischemic stroke. Inflammation. (2014) 37:2030–9. doi: 10.1007/s10753-014-9935-9
77. Weiner GM, Ducruet A. IL-21 receptor modulates ischemic severity in stroke. Neurosurgery. (2016) 79:N14–6. doi: 10.1227/01.neu.0000508602.53941.4e
78. Lee HK, Keum S, Sheng H, Warner DS, Lo DC, Marchuk DA. Natural allelic variation of the IL-21 receptor modulates ischemic stroke infarct volume. J Clin Invest. (2016) 126:2827–38. doi: 10.1172/JCI84491
79. Dong Y, Hu C, Huang C, Gao J, Niu W, Wang D, et al. Interleukin-22 plays a protective role by regulating the JAK2-STAT3 pathway to improve inflammation, oxidative stress, and neuronal apoptosis following cerebral ischemia-reperfusion injury. Mediat Inflamm. (2021) 2021:1–14. doi: 10.1155/2021/6621296
80. Hirota K, Duarte JH, Veldhoen M, Hornsby E, Li Y, Cua DJ, et al. Fate mapping of interleukin 17-producing T cells in inflammatory responses. Nat Immunol. (2011) 12:255–63. doi: 10.1038/ni.1993
81. Sun G, Yang S, Cao G, Wang Q, Hao J, Wen Q, et al. γδ T cells provide the early source of IFN-γ to aggravate lesions in spinal cord injury. J Exp Medicine. (2018) 215:521–35. doi: 10.1084/jem.20170686
82. Mendiola AS, Cardona AE. The IL-1β phenomena in neuroinflammatory diseases. J Neural Transm. (2018) 125:781–95. doi: 10.1007/s00702-017-1732-9
83. Zhang L, Huang Y, Lin Y, Shan Y, Tan S, Cai W, et al. Anti-inflammatory effect of cholera toxin B subunit in experimental stroke. J Neuroinflamm. (2016) 13:147. doi: 10.1186/s12974-016-0610-y
84. Zhang J, Mao X, Zhou T, Cheng X, Lin Y. IL-17A contributes to brain ischemia reperfusion injury through calpain-TRPC6 pathway in mice. Neuroscience. (2014) 274:419–28. doi: 10.1016/j.neuroscience.2014.06.001
85. Konoeda F, Shichita T, Yoshida H, Sugiyama Y, Muto G, Hasegawa E, et al. Therapeutic effect of IL-12/23 and their signaling pathway blockade on brain ischemia model. Biochem Bioph Res Co. (2010) 402:500–6. doi: 10.1016/j.bbrc.2010.10.058
86. Wang L, Lin Z, Zhang H, Shao B, Xiao L, Jiang H, et al. Timing and dose regimens of marrow mesenchymal stem cell transplantation affect the outcomes and neuroinflammatory response after ischemic stroke. Cns Neurosci Ther. (2014) 20:317–26. doi: 10.1111/cns.12216
87. Evans MA, Kim HA, Ling YH, Uong S, Vinh A, Silva TMD, et al. Vitamin D3 supplementation reduces subsequent brain injury and inflammation associated with ischemic stroke. Neuromol Med. (2018) 20:147–59. doi: 10.1007/s12017-018-8484-z
88. Gelderblom M, Gallizioli M, Ludewig P, Thom V, Arunachalam P, Rissiek B, et al. IL-23 (Interleukin-23)–producing conventional dendritic cells control the detrimental IL-17 (Interleukin-17) response in stroke. Stroke. (2018) 49:155–64. doi: 10.1161/STROKEAHA.117.019101
89. Pan Y, Tian D, Wang H, Zhao Y, Zhang C, Wang S, et al. Inhibition of perforin-mediated neurotoxicity attenuates neurological deficits after ischemic stroke. Front Cell Neurosci. (2021) 15:664312. doi: 10.3389/fncel.2021.664312
90. Kuo P, Scofield BA, Yu I, Chang F, Ganea D, Yen J. Interferon-β modulates inflammatory response in cerebral ischemia. J Am Hear Assoc Cardiovasc Cerebrovasc Dis. (2016) 5:e002610. doi: 10.1161/JAHA.115.002610
91. Caccamo N, Mendola CL, Orlando V, Meraviglia S, Todaro M, Stassi G, et al. Differentiation, phenotype, and function of interleukin-17–producing human Vγ9Vδ2 T cells. Blood. (2011) 118:129–38. doi: 10.1182/blood-2011-01-331298
92. Hueber W, Patel DD, Dryja T, Wright AM, Koroleva I, Bruin G, et al. Effects of AIN457, a fully human antibody to Interleukin-17A, on psoriasis, rheumatoid arthritis, and uveitis. Sci Transl Med. (2010) 2:52ra72. doi: 10.1126/scitranslmed.3001107
93. Adamski MG, Li Y, Wagner E, Yu H, Seales-Bailey C, Durkin H, et al. Pre-existing hypertension dominates γδT cell reduction in human ischemic stroke. PLoS One. (2014) 9:e97755. doi: 10.1371/journal.pone.0097755
94. Hueber W, Sands BE, Lewitzky S, Vandemeulebroecke M, Reinisch W, Higgins PDR, et al. Secukinumab, a human anti-IL-17A monoclonal antibody, for moderate to severe Crohn's disease: unexpected results of a randomised, double-blind placebo-controlled trial. Gut. (2012) 61:1693. doi: 10.1136/gutjnl-2011-301668
95. Maxwell JR, Zhang Y, Brown WA, Smith CL, Byrne FR, Fiorino M, et al. Differential roles for interleukin-23 and interleukin-17 in intestinal immunoregulation. Immunity. (2015) 43:739–50. doi: 10.1016/j.immuni.2015.08.019
Keywords: immunity, inflammation, γδ T, cerebral ischemia stroke, therapy
Citation: Wang L, Yao C, Chen J, Ge Y, Wang C, Wang Y, Wang F, Sun Y, Dai M, Lin Y and Yao S (2022) γδ T Cell in Cerebral Ischemic Stroke: Characteristic, Immunity-Inflammatory Role, and Therapy. Front. Neurol. 13:842212. doi: 10.3389/fneur.2022.842212
Received: 18 January 2022; Accepted: 01 March 2022;
Published: 31 March 2022.
Edited by:
Johannes Boltze, University of Warwick, United KingdomReviewed by:
Lisa Anna Mielke, Olivia Newton-John Cancer Research Institute, AustraliaCopyright © 2022 Wang, Yao, Chen, Ge, Wang, Wang, Wang, Sun, Dai, Lin and Yao. This is an open-access article distributed under the terms of the Creative Commons Attribution License (CC BY). The use, distribution or reproduction in other forums is permitted, provided the original author(s) and the copyright owner(s) are credited and that the original publication in this journal is cited, in accordance with accepted academic practice. No use, distribution or reproduction is permitted which does not comply with these terms.
*Correspondence: Yun Lin, ZnJhbmtsaW55dW5AaHVzdC5lZHUuY24=; Shanglong Yao, eWFvc2hhbmdsb25nQGh1c3QuZWR1LmNu
Disclaimer: All claims expressed in this article are solely those of the authors and do not necessarily represent those of their affiliated organizations, or those of the publisher, the editors and the reviewers. Any product that may be evaluated in this article or claim that may be made by its manufacturer is not guaranteed or endorsed by the publisher.
Research integrity at Frontiers
Learn more about the work of our research integrity team to safeguard the quality of each article we publish.