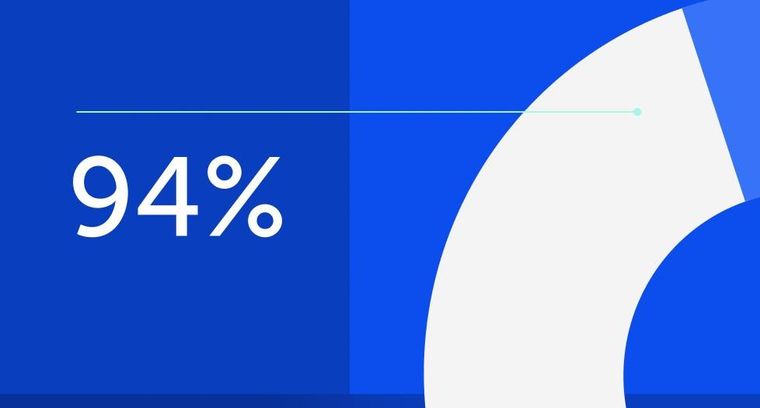
94% of researchers rate our articles as excellent or good
Learn more about the work of our research integrity team to safeguard the quality of each article we publish.
Find out more
ORIGINAL RESEARCH article
Front. Neurol., 28 February 2022
Sec. Stroke
Volume 13 - 2022 | https://doi.org/10.3389/fneur.2022.766622
Objectives: To investigate the proactive and reactive control process when executing a complex task in patients with stroke. Proactive control is the preparatory process before the target stimulus, whereas reactive control is an imperative resolution of interference after the target stimulus.
Methods: In total, 17 patients with chronic stroke and 17 healthy individuals were recruited. The proactive and reactive control of executive function was assessed by the task-switching paradigm and the AX version of the Continuous Performance Task (AX-CPT). The general executive function was assessed by Color Trial Test (CTT) and Stroop Test. The behavioral data of the task-switching paradigm were analyzed by a three-way repeated-measures ANOVA, and the AX-CPT data were analyzed by two-way repeated-measures ANOVA.
Results: For efficiency scores in the task-switching paradigm, trial (repeat vs. switch) × group (stroke or control group) interaction effect was significant. Post-hoc analysis on trial × group effect showed a significant between-trial difference in accuracy rates in the repeat trial in the control group regardless of 100 or 50% validity. For the AX-CPT, the main effects of condition and group on response time were statistically significant. The interaction effect of condition (AY or BX) × group (stroke or control group) was also significant. Post-hoc analysis for condition × group indicated that the stroke group had a significantly longer response time in the BX condition than the control group and longer completion time in CTT2 and larger word interference for completion time in the Stroop test than the control cohort.
Conclusions: Post-stroke survivors showed deficits in the performance of proactive control but not in the performance of reactive control. Deficits in proactive control may be related to the impairment of working memory. Interventions that focus on proactive control may result in improved clinical outcomes.
Approximately 50–75% of chronic post-stroke survivors have mild-to-moderate cognitive dysfunction (Desmond, Moroney) (1). Executive functions are higher order of cognitive functions that consist of set-shifting, initiation, monitoring one's behavior, and self-regulating functions (2). Basic cognitive functions could be modulated and organized by executive functions to achieve goal-oriented behaviors (3). The research conducted by Laakso et al. (2) showed that ischemic stroke patients aged 55–85 years, who participated in the Helsinki (Finland) Stroke Aging Memory Study (SAM), had worse performance in executive functions assessments than healthy individuals, including the measures of set-shifting, initiation, response inhibition, and strategy formation. Executive function was considered to be the main factor that influenced the clinical outcome and the overall functional status in patients with stroke after rehabilitation (4). This subsequently contributes to limitations in performing daily activities and participating in social activities (2, 5). Therefore, the precise evaluation of executive function capacity in patients with stroke is essential.
The Montreal Cognitive Assessment (MoCA) is commonly adopted as a brief screening test of general cognitive function. Three subtests of the MoCA, which are the Trail Making B task, a phonemic fluency task, and a two-item verbal abstraction task, could be applied to assess executive functions (6). Executive dysfunctions are indicated if the patient could not complete the subtests. There are others specifically designed assessments to evaluate executive functions such as the Stroop test or the Color Trail Test (CTT) (2). A previous study showed that a large sample of stroke or brain tumor patients with lesions in the frontal region performed worse in the Stroop test than healthy individuals. This suggested an inhibition impairment in stroke patients who had unilateral frontal cortex lesions (7). However, the MoCA, Stroop test, and CTT do not consider the patients' capacity in different executive control processes, e.g., after a cue or target stimulus. Braver (2012) proposed the theory of dual mechanisms of control (DMC), which comprised of proactive and reactive controls. Proactive control is an early selection of goal-relevant information that is processed in a sustained manner of the working memory before the occurrence of the cognitively demanding events (8). It plays an important role in orienting the behavior before the event occurs in our daily life. On the contrary, reactive control refers to the immediate resolution of the current conflict or interference (8), especially when the conflict is without any preparation. The DMC model has been employed to explore the performance of executive function in a different sample population. For instance, published literature reported that elderly people and children tended to employ reactive control, while young adults were more reliant on proactive control in the response-compatibility task (9) and in the AX version of Continuous Performance Task (AX-CPT)(10). Thus, the DMC theory provides a better way to understand the flexibility of behavior regulation in complex situations in our daily life (8). However, most of the studies published to date explored executive dysfunctions without investigating the proactive and reactive controls in patients with chronic stroke.
Proactive control impairment is presented as the inability to employ the information conveyed by the cue to prepare for a response to resolve an upcoming conflict, which required working memory and sustain attention (8, 11). Execution of a functional movement requires an extent of motor anticipation (12), which was comparable to that in the proactive control of the DMC model (13). The results of a study that utilized an electroencephalogram to assess motor anticipation in patients with stroke indicated that motor anticipation impairment and hand motor function were moderately associated with motor planning (14). In Delphine et al.'s study, eight stroke patients were required to grasp and hold a brisk loading under predictive and reactive conditions. The onset time of grip force after the impact was significantly later in the paretic hand of stroke patients than in controls during both predictive and reactive conditions (15). The findings of these previous studies suggested that stroke patients have deficit in proactive control in a simple motor reaction task. This deficit may be related to the prominent impairments of motor task initiation and generation with anticipation loss (16). It remains unclear in terms of the behavioral regulation that involves proactive and reactive controls in patients with chronic stroke when executing a complex task that demands executive function.
This cross-sectional study aimed to explore the performance of proactive and reactive controls of executive functions in post-stroke survivors by the task-switching paradigm and AX-CPT. As impairment of working memory, sustained attention, and motor anticipation was previously reported in stroke patients (16–18), the hypothesis of the present study was that proactive control was more affected than reactive control when performing a complex functional task in patients with stroke. The findings of the present study extend the knowledge pool on executive dysfunction in patients with stroke and would be of interest to a wide audience.
Participants were recruited from the inpatient unit of the Department of Rehabilitation Medicine, the First Affiliated Hospital of the Sun Yat-sen University. All of the participants were screened by a trained doctor according to the following inclusion and exclusion criteria. Inclusion criteria of the stroke cohort were as follows: (1) age between 40 and 65 years old; (2) a time of at 3 months after stroke occurrence; (3) self-reported or informant-reported memory or cognitive complaints; (4) being right-handed. The exclusion criteria of the stroke cohort were as follows: (1) a MoCA score of ≤ 14; (2) severe sensorimotor impairment; (3) aphasia identified by the Western Aphasia Battery with an aphasia quotient <93.8; (4) a history of neurological or psychiatrist conditions prior to stroke occurrence; (5) participating in another intervention trial. Healthy participants who had matched age and education level were recruited as the control cohort. Written informed consent was obtained from the participants prior to enrollment. Ethical approval for the present study was granted by the Institutional Ethical Committee for Clinical Research and Animal Trials of the First Affiliated Hospital of Sun Yat-sen University (Approval No. [2020]073).
The task-switching paradigm and AX-CPT were employed to assess the performance of proactive and reactive controls of executive function. The design of the task-switching paradigm and AX-CPT made reference to the published studies (13, 19). The general executive function was accessed by the CTT and the Stroop test. The task-switching paradigm and AX-CPT were conducted in the software E-prime 2.0 (Psychological Software Tools, Pittsburgh, PA, USA).
In the task-switching paradigm, each trial began with a task cue (4 cm × 4 cm) at the center of the screen for 1,500 ms (Figure 1). The cue was followed by a target stimulus (4 cm × 4 cm) that lasted for 2,000ms. Participants were instructed to give a response to the target stimulus as soon as possible and within the 2,000 ms after the onset of the target stimulus. A blank interval of 1,000 ms then appeared, and the next trial began. There were two cue validities: 100 and 50%. The 100% valid cues were shown as a solid square () or a diamond (
). The 50% valid cue was shown as a solid star (
). The cues with 100% validity asked the participants to prepare for the response selection rules based on the cue, whereas the cues with 50% validity did not convey any information about the following target stimulus. Thus, proactive and reactive controls were manipulated by the 100 and 50% validities cues, respectively. The ratio of the two types of cue validities was 1:1. The target stimulus formed by a digit (1 or 2) appeared inside the shape (a square or diamond) denoted two sets of response selection rules in this paradigm. One response selection rule was that a square with the digit “1” meant for the participant to press the button v on the computer keyboard, and a square with the digit “2” meant for the participant to press the button b. The other response selection rule was that the diamond with the digit “1” corresponded to the button b, and the diamond with the digit “2” corresponded to button v. In each trial, participants were required to make a response by following a rule that was different from (switch trial) or the same as (repeat trial) the previous trial. In the repeat trial, the response rule was the same as the previous trial, whereas in the switch trial the response rule was different from the previous one. The response time and accuracy rates were recorded during the experiment. There were two blocks and 61 trials for each block, in which the first trial was excluded for data analysis. It took around 6 min to complete one block followed by a 2–3 min break. The mixed block included the same ratio of both repeat (non-switch) trials and switch trials.
AX-CPT included four cue-probe pairs, which were A-X, A-Y, B-X, and B-Y. A or B served as a cue, and X or Y served as a probe (Figure 2). The cues (4 cm × 4 cm) and probe stimulus were presented at the center of the screen for 250 ms. A blank screen appeared in an inter-stimulus interval of 1,000 ms. After the onset of the probe stimulus, the participant was asked to give a response to the probe as soon as possible within the 1,000 ms. Participants were informed to press the button “v” on the keyboard as a target response for the probe “X” following the cue “A” and to press the button “b” as a non-target response for the other three cue-probe pairs. 70% of the trials were for AX condition, while 10% of the trials were for each of the other three conditions. The order of these four conditions was randomized. In total, there were two blocks, and each block was composed of 30 trials. It took approximately 2 min to complete one block with a short break between two blocks.
Figure 2. Schematic illustration of the time course of one trial in AX-CPT. Each trial of the AX-CPT began with a cue stimulus of 250 ms followed by a blank interval of 1,000 ms. The probe stimulus then appeared for 250 ms. After the onset of the probe stimulus, the participant was asked to give a response to the probe as soon as possible within 1,000 ms.
The AX-CPT was designed to assess the proactive control to maintain the cue in the working memory and reactive control to inhibit a prepotent response (20). Proactive control was primarily measured by the BX condition, in which adequate preparation elicited by the cue could enhance the reaction time for the probe. In contrast, reactive control was primarily measured by the AY condition, which required the greatest amount of reactive control to inhibit the prepotent motor response predicted by the cue of “A”.
CTT consisted of two parts (CTT1 and CTT2). For CTT1, numbered circles from 1 to 25 were randomly printed on a sheet of paper. Participants were asked to draw a line to join up all the circles in numerical order. CTT2 involved two circles for each number. One circle was in yellow and the other one was in red. Participants were required to draw a line to join up all the numbers from 1 to 25, alternating between the two colors (21). The time it took for completion, the number sequence, and color sequence errors were recorded by the examiner. An interference effect reflecting the shifting function was calculated by the completion time of CTT2 minus the completion time of CTT1 (Interference effect = CTT2 – CTT1).
A Stroop test was performed to assess the function of conflict inhibition. The Stroop test consisted of two sub-tasks: naming color and naming the color in the printed words. The naming color task required participants to name the color patches printed in red, yellow, green, and blue colors. The task of naming color in printed word required participants to name the ink color of a set word, ignoring the words which were themselves the names of other colors. The 100 words or color patches of each sub-task were arranged in a 10 × 10 matrix. Participants were required to complete the subtasks correctly as quickly as they could. The response times it took for completion and the number of errors during each sub-task were recorded. The word interference of completion time, which is the completion time of naming colors minus the completion time of naming colors in printed words, was also included in the data analysis.
Background information (age, sex, height, weight, education experience, months after stroke, type of stroke, and lesion side and location) was recorded for all participants. In the task-switching paradigm and AX-CPT, the participant was seated in a quiet room in a relaxed position. A laptop computer for displaying the visual stimulus in the task-switching paradigm and AX-CPT was placed at a distance of 65–75cm in front of the participant. The task-switching paradigm and AX-CPT were randomly assigned to the participants. Before testing, the participant had the practice session to be familiar with each task. After finishing the task-switching paradigm and AX-CPT, the participant completed the MoCA, CTT, and Stroop test in a random order. Each participant completed all the tasks in two sessions (morning or afternoon) within 2 days. The task-switching paradigm and AX-CPT were conducted in one session which lasted for one hour. The MoCA, CTT, and Stroop test were conducted in the other session that lasted for around 1 h. Each participant was given sufficient time to rest between any two tasks.
Descriptive analysis was conducted to describe the characteristics of the sample populations. An independent t-test was applied to analyze the descriptive characteristics, the behavior data of CTT, Stroop test of the two groups of participants. A Chi-square test was conducted to analyze the between-group difference in sex. The Kolmogrov-Smirnov Test was conducted on the 24 variables of behavioral data to assess the normality of the data set. The result of the Kolmogrov-Smirnov Test showed that seven variables were significant (p < 0.050). The 17 variables of the data set were normally distributed. The behavioral data of the task-switching paradigm were analyzed by a three-way repeated-measures ANOVA: the within-subject factor was validity (100 or 50%) and trial (repeat or switch), and the between-subject factor was group (stroke or control group). The data of task switching paradigm showed significant correlations between participants' accuracy rates and reaction times in the control group (repeat trials with 100% cue validity: r = −0.693, p = 0.002; switch trials with 100% cue validity: r = −0.537, p = 0.026; repeat trials with 50% cue validity: r = −0.729, p = 0.001; switch trials with 50% cue validity: r = −0.517, p = 0.033). These significant correlations suggested a potential accuracy-speed trade-off, which would confound the results. To tackle the problem of accuracy-speed trade-off, the efficiency score (accuracy rate divided by reaction time) was used to analyze the behavioral data in each condition of the task-switching paradigm (22, 23). The behavior data of the AX-CPT were analyzed using a two-way repeated-measures ANOVA: the within-subject factor was condition (AY or BX), and the between-subject factor was group (stroke or control group). The dependent variables were the response time and accuracy rates in the AX-CPT and the response times, accuracy rates, and efficiency scores in repeat and switch trials in the task-switching paradigm. When significant main or interaction effects were observed in the two-way/three-way repeated-measures ANOVA, posthoc pairwise comparisons with the Bonferroni adjustment were applied. Pearson's correlation analysis was conducted to test the relationship between MOCA scores, general executive functions, and the performance in the task-switching paradigm and AX-CPT of the two cohorts. If multiple variables in the task-switching paradigm or AX-CPT were significantly related to general executive functions, a multiple regression model with a stepwise method was then conducted to verify the contributions of each variable to the capacity of general executive functions. All analyses were performed using the SPSS 20.0 (IBM SPSS Inc. Chicago, IL, USA) Windows software. The level of significance in the multivariate analyses was set at p < 0.05.
In total, 17 participants with stroke (12 males and 5 females) and 17 control participants (8 males and 9 females) were recruited in this study. The sample characteristics of the two cohorts are presented in Table 1. The between-group difference in sex was not significant (p = 0.163). No significant between-group difference was shown in terms of age, weight, height, BMI, and education experience (see Table 1). The MoCA score in the stroke group was significantly lower than the control group (p < 0.001). Table 2 presents the clinical characteristics of each participant in the stroke cohort.
Participants' accuracy rates of the repeat and switch trials in the task-switching paradigm are presented in Table 3. The proactive and reactive controls in the task-switching paradigm were manipulated by the 100 and 50% validities cues, respectively. The three-way repeated-measures ANOVA showed that the main effects of validity [F(1, 32) = 1.319, p = 0.259, ηp2 = 0.040] and trial [F(1, 32) = 1.315, p = 0.260, ηp2 = 0.039] were not statistically significant. However, the group main effect was statistically significant [F(1, 32) = 12.174, p = 0.001, ηp2 = 0.276]. The interaction effect of validity × trial × group was not significant [F(1, 32)= 0.429, p = 0.517, ηp2 = 0.013]. Significant group main effect suggested that participants in the stroke group had lower accuracy rates than the controls regardless of validity and trial.
Table 3. Participants' accuracy rates (%) and response times (ms) of repeat and switch trials in task-switching paradigm stratified by group and validity.
Participants' response time of repeat and switch trials in task-switching paradigm are shown in Table 3. The main effects of validity [F(1, 32) = 12.508, p = 0.001, ηp2 = 0.281] and trial [F(1, 32) = 36.513, p < 0.001, ηp2 = 0.533] were significant. However, the group effect was not significant [F(1, 32) = 0.097, p = 0.758, ηp2 = 0.003]. The interaction effect of validity × trial × group was not significant [F(1, 32) = 0.1.186, p = 0.284, ηp2 = 0.036]. The interaction effect of trial × group was also not significant [F(1, 32) = 1.328, p = 0.258, ηp2 = 0.040].
Participants' efficiency scores (accuracy rate divided by reaction time) of repeat and switch trials in the task-switching paradigm are shown in Table 4. The main effects of validity [F(1, 32) = 1.444, p = 0.238, ηp2 = 0.043] were not statistically significant. However, the main effects of trial [F(1, 32) = 23.352, p < 0.001, ηp2 = 0.422] and group were statistically significant [F(1, 32) = 8.181, p = 0.007, ηp2 = 0.204]. The interaction effect of validity × trial × group was not significant [F(1, 32)= 0.014, p = 0.906, ηp2 < 0.001], whereas the trial × group interaction effect was significant [F(1, 32) = 8.546, p = 0.006, ηp2 = 0.211]. A post hoc analysis of the trial × group effect on efficiency scores showed that no significant between-trial difference in efficiency scores in the stroke group (p = 0.187) in both 100 or 50% validity, whereas the efficiency scores in the repeat trial were significantly higher than the switch trial in the control group regardless of 100 or 50% validity (p < 0.001). Participants in the stroke group had lower efficiency scores than the controls in both repeat (p = 0.002) and switch trials (p = 0.068). These findings also suggested that the stroke group did not perform worse than the control group in the proactive and reactive controls of switching functions.
Table 4. Participants' efficiency scores (%/ms) of repeat and switch trials in task-switching paradigm stratified by group and validity.
The main effect of condition on accuracy rates was significant [F(1, 32) = 8.196, p = 0.007, ηp2 = 0.204]. However, the group effect was not significant [F(1, 32) = 3.344, p = 0.077, ηp2 = 0.095]. The interaction effect of condition × group was also not significant [F(1, 32) = 0.719, p = 0.403, ηp2 = 0.022].
The main effects of condition and group on response time were statistically significant, [F(1, 32) = 61.151, p < 0.001, ηp2 = 0.656], [F(1, 32) = 8.899, p = 0.005, ηp2 = 0.218]. The interaction effect of condition × group was also significant [F(1, 32)= 4.414, p = 0.044, ηp2 = 0.121] (Figure 3). Post-hoc analysis for condition × group indicated that the stroke participants had a significantly longer response time in the BX condition than the control group (p < 0.001) (Figure 3). No significant between-group difference in response time was found in the AY condition (p = 0.169). These findings suggested that the proactive control that was related to working memory was impaired in patients with stroke.
Figure 3. The accuracy rates and response time in AY and BX conditions of stroke and control participants in the AX-CPT. (A) Presents the results of accuracy rate. (B) Presents the results of response time. Triangles denote the single subject distribution of the stroke group. Dots denote the single subject distribution of the control group. Error bars denote +/– SD. ** Denotes p < 0.001.
No significant between-group difference was observed in the error rates of CTT1 and CTT2 (p > 0.050). However, participants in the stroke group showed significantly longer completion time in CTT1 (p = 0.002), CTT2 (p < 0.001) and a larger interference effect (p = 0.019) than the control group (Table 5).
The error rates and the self-corrected error rates showed no significant between-group difference in naming color and naming the color of the printed word of the Stroop test (p > 0.050). However, the stroke group showed a significantly longer completion time in naming the color (p = 0.002), naming the color of the printed word (p = 0.001), and the word interference of CT (p = 0.029) (Table 6).
The relationship between general executive functions and proactive and reactive controls of the two groups are presented in Table 7. In the task-switching paradigm, the stroke group showed negative correlations between the accuracy rates of any trial type under 100 and 50% validities and the interference effect of CTT (r = −0.494 to −0.507, p < 0.050). Thus, the accuracy rates of repeat and switch trials under both 100 and 50% validities were put into the multiple regression model as the independent variables for the stroke group. The results of multiple regression analysis showed that only the accuracy rate of the switch trials under the 50% validity condition was a significant factor (R2 = 0.326, B = −0.571, p = 0.017). However, the accuracy rate of the switch trials under the 50% validity was only correlated with the interference effect in CTT in the control group (r = −0.614, p = 0.009). No significant association was found between the outcome variables in the task-switching paradigm and the word interference in the Stroop test (p > 0.050). The associations between MOCA scores and accuracy rates/response time of any trial type under 100 and 50% validities in two cohorts were also not significant (p > 0.050).
Table 7. The relationship between the general executive functions and the performance in the task-switching paradigm and AX-CPT of the two groups of participants.
In the AX-CPT, there was no significant correlation between the outcome variables in the AX-CPT, the interference effect in CTT, and MOCA scores in the two groups (p > 0.050). Significant correlations were found between the word interference of the Stroop test and the response time in AY (r = 0.675, p = 0.003) and BX (r = 0.829, p < 0.001) conditions in the control group but not in the stroke group. Thus, the response time of AY and BX conditions were put into the multiple regression model as the independent variables for the control group. The results of multiple regression analysis showed that only the response time in the BX condition was a significant factor (R2 = 0.688, B = 0.829, p < 0.001), whereas the response time in the AY condition was not significant (p = 0.433). These results further confirmed that stroke patients had impaired proactive control, which was related to conflict monitoring.
The present study investigated the performance of proactive and reactive controls of executive functions in post-stroke survivors by the dual cognitive control model. The main findings indicated that post-stroke participants require longer response times in the BX condition of the AX-CPT, but did not exhibit a reduction in the proactive and reactive control of switching compared with controls during the task-switching paradigm. These novel findings supported the hypothesis that proactive control was more affected than reactive control when performing a complex functional task.
In the BX condition, participants needed to maintain the “B” cue in the working memory, so that adequate preparation, which could enhance the reaction time for the probe (20). This study found that the post-stroke participants required a longer response time in the BX condition of the AX-CPT, suggesting that proactive control was impaired in post-stroke survivors. This finding was supported by two other previous studies that explored the proactive and reactive controls without involving executive functions in stroke patients (15, 24). One study showed impairment in the feed-forward adjustments of the multi-finger synergies prior to quick action in patients after a single cortical stroke with mild motor impairments (24). Dispa et al. found that the onset time of grip force after the impact was significantly later in the paretic hand of stroke patients than in controls in both predictive and reactive conditions (15). These findings suggested that stroke patients had deficits in proactive control in a motor reaction task. In the present study, the worse performance in proactive control of AX-CPT observed in the stroke group maybe resulted from the impairment of working memory, sustained attention, and anticipation loss. The execution of a complex task places a high demand on cognitive resources, including working memory, anticipation, and sustained attention during the proactive control period. The potential reasons for the worse performance in the proactive control in the stroke group are as follows. First, proactive control in AX-CPT was reported to be reliant upon the anticipation and prevention of interference before it occurred in the working memory (8, 20). Several studies have indicated working memory deficits in patients with stroke (18, 25). In the study by van Geldorp et al. (18), the participants were required to remember which cue was presented after a short temporal delay in a computerized delayed-match-to-sample task which included spatial, object, or binding (spatial + object) cues. Compared to healthy controls, the stroke participants showed working memory deficits in the conditions of spatial and object cues. Second, the impaired proactive control might be related to the anticipation loss in stroke patients. A study conducted by Roussel and Martinaud (16) indicated that in post-stroke participants, the dysexecutive profile was characterized by prominent impairments of motor initiation and generation with anticipation loss in the behavioral domain. In addition, several studies reported that proactive control is a sustained top-down attentional control (11, 13, 26). Manard et al. found that impaired proactive control of elderly people was related to a reduction in sustained neural activity at the frontal cortex (26). The sustained neural activity was related to sustained attention (11, 13). Patients with stroke were reported to have sustained attention deficits (17, 27), which could reduce the ability to expect and prepare for an upcoming stimulus (28). Therefore, deficits in sustained attention are likely to be a contributing factor to the impaired proactive control of stroke patients. However, previous studies indicated that motivation, fatigue, and mind wandering might have a negative effect on sustained attention for elderly people (29). Proactive control generally requires more effort, subsequently leads to more fatigue, which is most likely associated with the tendency to favor reactive over proactive control. Even though the participants in this study rested between any two tasks, it could not be ascertained that whether motivation, fatigue, and mind wondering might affect the behavior performance of proactive control. A future study that investigates the effect of these factors on the behavioral performance of proactive control would be beneficial to substantiate the findings of the present study.
According to the results of the task-switching paradigm, the stroke group had lower accuracy rates and efficiency scores in both repeat and switch trials than the control group. The two cohorts, however, did not show the between-trial difference in the 100 and 50% validities, which may suggest participants in the stroke group did not perform worse than healthy participants in the proactive and reactive controls of set-shifting function. These findings were consistent with the previous study, which demonstrated patients with stroke had different switch costs of accuracy rates compared with the control group in alternating switch tasks, but not in the cued task-switching (30). The findings in Pohl et al.'s study suggested that task switching in alternating switch tasks with a high demand of endogenous proactive control was impaired in adults after stroke, whereas task switching in the cued task-switching requiring exogenous proactive control was not impaired. The first reason was likely due to the higher difficulty level under endogenous proactive control than under exogenous proactive control. The second reason may be that the key neural structures that are responsible for the proactive and reactive control in task switching are different. Proactive control involves the frontal and/or parietal cortices (13, 20), whereas a wider network of brain regions are responsible for the reactive control in task switching, which involved the dorsolateral–ventrolateral plane of the prefrontal cortex and anterior cingulate cortex (ACC) (31, 32). However, both the present study and Pohl et al.'s study did not consider the different lesion locations between the two cohorts. This might potentially contribute to the lack of observed difference between the proactive and reactive controls of switching function in the stroke group.
The efficiency scores in the task-switching paradigm showed no significant between-trial difference in the stroke group, whereas the efficiency scores in the repeat trial of the control group were significantly higher than those in the switch trial regardless of 100 or 50% validity. These findings suggested that post-stroke survivors were less susceptible to proactive interference, which may be due to their lower working memory function. Allport and colleagues assumed that switch costs arose from proactive interference (33). Higher susceptibility of proactive interference in task switching was associated with higher working memory capacity (34, 35). Several studies had indicated working memory deficits in post-stroke survivors (18, 36), which was likely to be a factor that contributed to lower susceptibility to proactive interference in post-stroke survivors than healthy individuals. Impaired proactive control in the AX-CPT of stroke patients also supported deficits in the working memory since proactive control in the AX-CPT was related to working memory (20).
A larger interference effect in CTT and higher word interference of completion time in Stroop test were observed in stroke participants compared with healthy controls. The interference effect in CTT assessed the shifting function (24), while the word interference of completion time in the Stroop test taps on the conflict inhibition during the naming color task (37). The results of the CTT and Stroop test suggested that the general executive function was impaired in stroke participants. These findings were consistent with a previous study (2), which showed that stroke patients performed poorly in the Trail Making test and Stroop test. The results of the correlation and regression analysis between the Stroop test and the performance in BX condition of AX-CPT of the two groups further supported the notion that the proactive control for working memory function was impaired in post-stroke survivors. The process of interference control in the Stroop test included the top-down control process (38), which was also required during proactive control in AX-CPT (26). These two previous studies reported that the prefrontal cortex was the key node activated in the top-down control process in the Stroop test and proactive control. Thus, the response time in the BX condition related to proactive control was a significant factor related to the performance in the Stroop test.
There are several limitations in the present study that limit the interpretation of the data. First, the sample size was not power calculated and was likely to contain type II error. This might contribute to the lack of between-group differences in the task-switching paradigm. Second, the effects of lesion locations or lesion size on the proactive and reactive controls were not considered in the present study. Future study should consider recruiting sample group that has homogeneous lesion site and size. Third, the data related to the lesion such as side, temporal proximity, and the extent of the lesion were not taken into consideration in the present study. Future studies should consider the lesion data to further assess the impact on behavioral data. Forth, the sample population of the present study was heterogeneous which limits the interpretation of the findings. Fifth, two-way/three-way repeated-measures ANOVA applied to non-parametric data might lead to Type-I error. Sixth, the present study only employed a simple algorithm to explore the between-group difference in the speed-accuracy trade-off. Future studies should employ the diffusion drift models or other methodologies for in-depth exploring the between-group difference in the behavioral responses. In addition to behavioral outcomes, future research could adopt electrophysiology and brain-imaging techniques to investigate the underlying neural mechanisms (39). Last but not least, other research results showed a significant gender difference in proactive and reactive controls (40). Thus, there might be gender differences in proactive and reactive control performance of stroke patients. Further studies are required to clarify the gender effect on the performance of proactive and reactive controls in post-stroke survivors.
Proactive control is the preparatory process before the target stimulus, whereas reactive control is an imperative process of interference resolution after the target stimulus. Post-stroke survivors showed a deficit in the performance of proactive control that is related to working memory. However, post-stroke patients seemed not to show a significant deficit in the reactive control of executive functions. Interventions that focus on proactive control may result in improved clinical outcomes.
The raw data supporting the conclusions of this article will be made available by the authors, without undue reservation.
The studies involving human participants were reviewed and approved by the Institutional Ethical Committee for Clinical Research and Animal Trials of the First Affiliated Hospital of Sun Yat-sen University (Approval No. [2020]073). The patients/participants provided their written informed consent to participate in this study.
All authors meet the four primary ICMJE criteria for authorship. In addition, all authors have been actively involved in the study in different capacities: QY and WL: designed the study and conducted all stages of the study including data collection, analysis, interpretation, and drafting of the manuscript. XH, ZH, BZ, and ZLi: participated in the design of the research protocol, data analysis, and drafting of the manuscript. TZ, ZH, MD, and ZLia: participated in data collection, analysis, and interpretation of the data. WL and ZLia: revised the manuscript, interpreted the data, and managed the trial. All authors have read and approved the final manuscript.
This research project was supported by the National Natural Science Foundation of China (Grant Numbers: 81971224 and 82002375); Science and Technology Program of Guangzhou (Grant Number: 201803010083).
The authors declare that the research was conducted in the absence of any commercial or financial relationships that could be construed as a potential conflict of interest.
All claims expressed in this article are solely those of the authors and do not necessarily represent those of their affiliated organizations, or those of the publisher, the editors and the reviewers. Any product that may be evaluated in this article, or claim that may be made by its manufacturer, is not guaranteed or endorsed by the publisher.
We would like to thank all the participants who took part in the study and the clinical supporting staff of the First Affiliated Hospital, Sun Yat-sen University.
1. Desmond DW, Moroney JT, Paik MC, Sano M, Mohr JP, Aboumatar S, et al. Frequency and clinical determinants of dementia after ischemic stroke. Neurology. (2000) 54:1124–31. doi: 10.1212/WNL.54.5.1124
2. Laakso HM, Hietanen M, Melkas S, Sibolt G, Curtze S, Virta M, et al. Executive function subdomains are associated with post-stroke functional outcome and permanent institutionalization. Eur J Neurol. (2019) 26:546–52. doi: 10.1111/ene.13854
3. Tsuchida A, Fellows LK. Are core component processes of executive function dissociable within the frontal lobes? Evidence from humans with focal prefrontal damage. Cortex. (2013) 49:1790–800. doi: 10.1016/j.cortex.2012.10.014
4. Skidmore ER, Whyte EM, Holm MB, Becker JT, Butters MA, Dew MA, et al. Cognitive and affective predictors of rehabilitation participation after stroke. Arch Phys Med Rehabil. (2010) 91:203–7. doi: 10.1016/j.apmr.2009.10.026
5. Zahuranec DB, Skolarus LE, Feng C, Freedman VA, Burke JF. Activity limitations and subjective well-being after stroke. Neurology. (2017) 89:944–50. doi: 10.1212/WNL.0000000000004286
6. Nasreddine ZS, Phillips NA, Bedirian V, Charbonneau S, Whitehead V, Collin I, et al. The Montreal Cognitive Assessment, MoCA: a brief screening tool for mild cognitive impairment. J Am Geriatr Soc. (2005) 53:695–9. doi: 10.1111/j.1532-5415.2005.53221.x
7. Cipolotti L, Healy C, Chan E, MacPherson SE, White M, Woollett K, et al. The effect of age on cognitive performance of frontal patients. Neuropsychologia. (2015) 75:233–41. doi: 10.1016/j.neuropsychologia.2015.06.011
8. Braver TS. The variable nature of cognitive control: a dual mechanisms framework. Trends Cogn Sci. (2012) 16:106–13. doi: 10.1016/j.tics.2011.12.010
9. Friedman D, Nessler D, Cycowicz YM, Horton C. Development of and change in cognitive control: a comparison of children, young adults, and older adults. Cogn Affect Behav Neurosci. (2009) 9:91–102. doi: 10.3758/CABN.9.1.91
10. Braver TS, Paxton JL, Locke HS, Barch DM. Flexible neural mechanisms of cognitive control within human prefrontal cortex. Proc Natl Acad Sci USA. (2009) 106:7351–6. doi: 10.1073/pnas.0808187106
11. Rainey VR, Stockdale L, Flores-Lamb V, Kahrilas IJ, Mullins TL, Gjorgieva E, et al. Neural differences in the temporal cascade of reactive and proactive control for bilinguals and monolinguals. Psychophysiology. (2021) 58:e13813. doi: 10.1111/psyp.13813
12. Kalaska JF. From intention to action: motor cortex and the control of reaching movements. Adv Exp Med Biol. (2009) 629:139–78. doi: 10.1007/978-0-387-77064-2_8
13. Yu Q, Chau BKH, Lam BYH, Wong AWK, Peng J, Chan CCH. Neural processes of proactive and reactive controls modulated by motor-skill experiences. Front Hum Neurosci. (2019) 13:404. doi: 10.3389/fnhum.2019.00404
14. Chen L, Mao Y, Ding M, Li L, Leng Y, Zhao J, et al. Assessing the relationship between motor anticipation and cortical excitability in subacute stroke patients with movement-related potentials. Front Neurol. (2018) 9:881. doi: 10.3389/fneur.2018.00881
15. Dispa D, Thonnard JL, Bleyenheuft Y. Impaired predictive and reactive control of precision grip in chronic stroke patients. Int J Rehabil Res. (2014) 37:130–7. doi: 10.1097/MRR.0000000000000045
16. Roussel M, Martinaud O, Henon H, Vercelletto M, Bindschadler C, Joseph PA, et al. The behavioral and cognitive executive disorders of stroke: the GREFEX study. PLoS ONE. (2016) 11:e0147602. doi: 10.1371/journal.pone.0147602
17. Loetscher T, Potter KJ, Wong D, das Nair R. Cognitive rehabilitation for attention deficits following stroke. Cochrane Database Syst Rev. (2019) 2019:CD002842. doi: 10.1002/14651858.CD002842.pub3
18. van Geldorp B, Kessels RP, Hendriks MP. Single-item and associative working memory in stroke patients. Behav Neurol. (2013) 26:199–201. doi: 10.1155/2013/516804
19. Gonthier C, Macnamara BN, Chow M, Conway AR, Braver TS. Inducing proactive control shifts in the AX-CPT. Front Psychol. (2016) 7:1822. doi: 10.3389/fpsyg.2016.01822
20. Ryman SG, El Shaikh AA, Shaff NA, Hanlon FM, Dodd AB, Wertz CJ, et al. Proactive and reactive cognitive control rely on flexible use of the ventrolateral prefrontal cortex. Hum Brain Mapp. (2019) 40:955–66. doi: 10.1002/hbm.24424
21. Zhou FC, Hou WM, Wang CY, Ungvari GS, Chiu HF, Correll CU, et al. Prospective memory performance in non-psychotic first-degree relatives of patients with schizophrenia: a controlled study. PLoS ONE. (2014) 9:e111562. doi: 10.1371/journal.pone.0111562
22. Zou Z, Chau BKH, Ting KH, Chan CCH. Aging effect on audiovisual integrative processing in spatial discrimination task. Front Aging Neurosci. (2017) 9:374. doi: 10.3389/fnagi.2017.00374
23. Gonzalez-Valenzuela MJ, Diaz-Giraldez F, Lopez-Montiel MD. Cognitive predictors of word and pseudoword reading in Spanish first-grade children. Front Psychol. (2016) 7:774. doi: 10.3389/fpsyg.2016.00774
24. Jo HJ, Maenza C, Good DC, Huang X, Park J, Sainburg RL, et al. Effects of unilateral stroke on multi-finger synergies and their feed-forward adjustments. Neuroscience. (2016) 319:194–205. doi: 10.1016/j.neuroscience.2016.01.054
25. Povroznik JM, Ozga JE, Vonder Haar C, Engler-Chiurazzi EB. Executive (dys)function after stroke: special considerations for behavioral pharmacology. Behav Pharmacol. (2018) 29:638–53. doi: 10.1097/FBP.0000000000000432
26. Manard M, Francois S, Phillips C, Salmon E, Collette F. The neural bases of proactive and reactive control processes in normal aging. Behav Brain Res. (2017) 320:504–16. doi: 10.1016/j.bbr.2016.10.026
27. Hyndman D, Ashburn A. People with stroke living in the community: Attention deficits, balance, ADL ability and falls. Disabil Rehabil. (2003) 25:817–22. doi: 10.1080/0963828031000122221
28. Doricchi F, Pinto M, Pellegrino M, Marson F, Aiello M, Campana S, et al. Deficits of hierarchical predictive coding in left spatial neglect. Brain Commun. (2021) 3:fcab111. doi: 10.1093/braincomms/fcab111
29. Vallesi A, Tronelli V, Lomi F, Pezzetta R. Age differences in sustained attention tasks: a meta-analysis. Psychon Bull Rev. (2021). doi: 10.3758/s13423-021-01908-x
30. Pohl PS, McDowd JM, Filion D, Richards LG, Stiers W, Kluding P. Task switching after stroke. Phys Ther. (2007) 87:66–73. doi: 10.2522/ptj.20060093
31. Braver TS, Reynolds JR, Donaldson DI. Neural mechanisms of transient and sustained cognitive control during task switching. Neuron. (2003) 39:713–26. doi: 10.1016/S0896-6273(03)00466-5
32. Liston C, Matalon S, Hare TA, Davidson MC, Casey BJ. Anterior cingulate and posterior parietal cortices are sensitive to dissociable forms of conflict in a task-switching paradigm. Neuron. (2006) 50:643–53. doi: 10.1016/j.neuron.2006.04.015
33. Wylie G, Allport A. Task switching and the measurement of “switch costs”. Psychol Res. (2000) 63:212–33. doi: 10.1007/s004269900003
34. Steinwascher MA, Meiser T. How a high working memory capacity can increase proactive interference. Conscious Cogn. (2016) 44:130–45. doi: 10.1016/j.concog.2016.07.002
35. Pettigrew C, Martin RC. The role of working memory capacity and interference resolution mechanisms in task switching. Q J Exp Psychol. (2016) 69:2431–51. doi: 10.1080/17470218.2015.1121282
36. Roussel M, Dujardin K, Henon H, Godefroy O. Is the frontal dysexecutive syndrome due to a working memory deficit? Evidence from patients with stroke. Brain: J Neurol. (2012) 135:2192–201. doi: 10.1093/brain/aws132
37. Cheng Y, Wu W, Feng W, Wang J, Chen Y, Shen Y, et al. The effects of multi-domain versus single-domain cognitive training in non-demented older people: a randomized controlled trial. BMC Med. (2012) 10:30. doi: 10.1186/1741-7015-10-30
38. Popov T, Westner BU, Silton RL, Sass SM, Spielberg JM, Rockstroh B, et al. Time Course of Brain Network Reconfiguration Supporting Inhibitory Control. J Neurosci. (2018) 38:4348–56. doi: 10.1523/JNEUROSCI.2639-17.2018
39. Redick TS. Cognitive control in context: working memory capacity and proactive control. Acta Psychol. (2014) 145:1–9. doi: 10.1016/j.actpsy.2013.10.010
Keywords: executive function (EF), proactive control, reactive control, stroke, working memory
Citation: Yu Q, Huang X, Zhang B, Li Z, Zhang T, Hu Z, Ding M, Liang Z and Lo WLA (2022) A Novel Perspective on the Proactive and Reactive Controls of Executive Function in Chronic Stroke Patients. Front. Neurol. 13:766622. doi: 10.3389/fneur.2022.766622
Received: 29 August 2021; Accepted: 06 January 2022;
Published: 28 February 2022.
Edited by:
Swathi Kiran, Boston University, United StatesReviewed by:
Rachele Pezzetta, San Camillo Hospital (IRCCS), ItalyCopyright © 2022 Yu, Huang, Zhang, Li, Zhang, Hu, Ding, Liang and Lo. This is an open-access article distributed under the terms of the Creative Commons Attribution License (CC BY). The use, distribution or reproduction in other forums is permitted, provided the original author(s) and the copyright owner(s) are credited and that the original publication in this journal is cited, in accordance with accepted academic practice. No use, distribution or reproduction is permitted which does not comply with these terms.
*Correspondence: Zhenwen Liang, bGlhbmd6aGVud2VAam51LmVkdS5jbg==; Wai Leung Ambrose Lo, bHVvd2xpYW5nQG1haWwuc3lzdS5lZHUuY24=
Disclaimer: All claims expressed in this article are solely those of the authors and do not necessarily represent those of their affiliated organizations, or those of the publisher, the editors and the reviewers. Any product that may be evaluated in this article or claim that may be made by its manufacturer is not guaranteed or endorsed by the publisher.
Research integrity at Frontiers
Learn more about the work of our research integrity team to safeguard the quality of each article we publish.