- 1Department of Neurosurgery, Icahn School of Medicine at Mount Sinai, New York, NY, United States
- 2Sinai BioDesign, Icahn School of Medicine at Mount Sinai, New York, NY, United States
- 3The Grove School of Engineering, The City College of New York, New York, NY, United States
- 4Department of Otolaryngology, Icahn School of Medicine at Mount Sinai, New York, NY, United States
Acute ischemic stroke (AIS) is a common devastating disease that has increased yearly in absolute number of cases since 1990. While mechanical thrombectomy and tissue plasminogen activator (tPA) have proven to be effective treatments, their window-of-efficacy time is very short, leaving many patients with no viable treatment option. Over recent years there has been a growing interest in stimulating the facial nerves or ganglions to treat AIS. Pre-clinical studies have consistently demonstrated an increase in collateral blood flow (CBF) following ganglion stimulation, with positive indications in infarct size and neurological scores. Extensive human trials have focused on trans-oral electrical stimulation of the sphenopalatine ganglion, but have suffered from operational limitations and non-significant clinical findings. Regardless, the potential of ganglion stimulation to treat AIS or elongate the window-of-efficacy for current stroke treatments remains extremely promising. This review aims to summarize results from recent trial publications, highlight current innovations, and discuss future directions for the field. Importantly, this review comes after the release of four important clinical trials that were published in mid 2019.
Introduction
Acute Ischemic Stroke
Stroke is the leading cause of disability and the fifth leading cause of death in the United States (US). Approximately 795,000 people experience a new or recurrent stroke each year (1). Acute ischemic stroke (AIS) occurs when an obstruction within a blood vessel decreases cerebral blood flow, depriving nerve cells of oxygen and leading to severe metabolic failure and neural death (2–4). Immediately following stroke, a section of the brain referred to as the ischemic core is subject to extreme hypoxia, leading to irreversible brain damage (5). The area surrounding the ischemic core, the ischemic penumbra, is severely hypoperfused and non-functioning, yet can regain functionality if blood flow is restored to the area (5, 6). This recovery is highly time-dependent, as the penumbra rapidly evolves into the ischemic core (6, 7). The recovery of the penumbra has been demonstrated to have a significant effect on clinical outcomes; Meretoja et al. showed that for every 20-min reduction in time to reperfusion increases the average disability-free life span by 3 months (8).
Current Treatments: Endovascular Thrombectomy and Tissue Plasminogen Activator
Management for AIS relies on rapid treatment times to avoid penumbra evolution. The goal of modern stroke treatment facilities is to reperfuse the ischemic area via endovascular thrombectomy, mechanically removing the blood clot with catheter-based devices (9). The faster the patient achieves reperfusion, the more likely the patient will have excellent neurological outcomes at 90 days (10). The second treatment paradigm for AIS is the use of intravenous (IV) tissue plasminogen activator (tPA), which acts to chemically break down clots (11–14). IV-tPA is commonly used prior to patient transport for thrombectomy.
Both endovascular thrombectomy and IV-tPA suffer from a limited window-of-efficacy. Currently, stroke guidelines list the acceptable window of treatment for mechanical thrombectomy at 24 h (15, 16), and a recommended IV-tPA door-to-treatment time of 60 min (10, 17, 18). These windows are frequently missed, with fewer than a third of patients in the U.S. treated within the IV-tPA 60 min window (19). Mandatory neuroimaging, presence of a highly-trained neurointerventionalist, and hospital transfer times all reduce event-to-treatment times and result in reductions of successful functional outcomes following recanalization (16, 20, 21).
Thrombectomy is a well-established treatment (15, 16, 22), but patients routinely fail to achieve functional independence (mRS ≤ 2) due to extensive event-to-treatment times (15, 16). There is a clear need for innovative approaches to extend the window-of-efficacy for endovascular thrombectomy and IV-tPA.
Time Is Brain: Inhibiting the Evolution of the Ischemic Penumbra
Researchers have recently sought to find new approaches to arrest the evolution of the ischemic penumbra and keep this susceptible region from becoming irreversibly damaged. Current approaches aim to either enhance oxygen delivery to the penumbra or reduce tissue oxygen demand (6). In addition to door-to-treatment time limitations, many patients also become ineligible for mechanical thrombectomy due to large ischemic core volumes. Inhibiting the evolution of the penumbra may help buy time by limiting core volume growth from reaching recommended exclusionary levels (23, 24). Inhibition of penumbra evolution could also be highly beneficial when combined with IV-tPA, potentially increasing its effectiveness and elongating its window-of-efficacy (6).
Increased Collateral Blood Circulation Through Ganglion Stimulation
Facial nerve-induced vasodilation of the cerebral arteries is an emerging therapeutic target that seeks to increase collateral blood flow in ischemic brain tissue, improve oxygen availability, and improve patient functional outcomes after stroke. Collateral circulation refers to alternative, pre-existing vascular pathways that deliver blood to target tissue when the primary vessel is occluded (25). Imaging of the brain and vessels has shown that collateral blood flow can preserve brain tissue for hours after major arteries to the brain are blocked (26). Figure 1 illustrates how facial nerve-induced increased collateral blood flow has the ability to ameliorate clot-induced tissue death by limiting ischemic penumbra evolution.
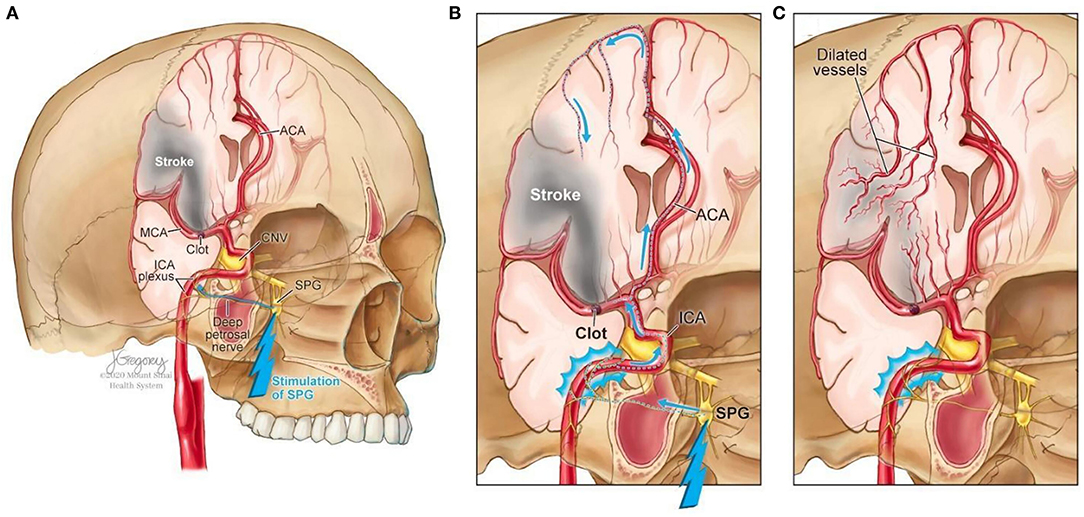
Figure 1. Increased collateral blood circulation by sphenopalatine ganglion (SPG) stimulation. (A) Anatomical overview of stroke affected brain region before SPG stimulation. (B) The SPG contains parasympathetic fibers that synapse in the ganglion and innervate the internal carotid artery (ICA) through the deep petrosal nerve. (C) SPG stimulation induces vasodilation of blood vessels in the anterior circulation, increasing blood flow to the affected area limiting the evolution of the penumbra and reducing infarct volume.
The sphenopalatine ganglion (SPG), also known as the pterygopalatine ganglion, is the largest and most superior ganglion of the sympathetic and parasympathetic nervous system and contains the largest collection of neurons in the calvarium outside of the brain (27). Humans have two SPGs, on each side of the midface, located within the viscerocranium in a space called the pterygopalatine fossa. This fossa has direct connections to the middle cranial fossa, nasal cavity, orbit, infratemporal fossa and oral cavity (28, 29). The SPG contains parasympathetic fibers that synapse in the ganglion and innervate the internal carotid artery (ICA) through the deep petrosal nerve (30). Electrical stimulation of the SPG activates the fibers, releasing several neurotransmitters such as acetylcholine, vasoactive intestinal polypeptide, peptide histidine isoleucine, and nitrous oxide that play a role in inducing vasodilation of blood vessels in the anterior circulation (26, 31).
The geniculate ganglion is a small collection of somatosensory and gustatory ganglion cells (32) located within the temporal bone along the axis of the ear canal. Similar to the SPG, the geniculate ganglion has parasympathetic connections to cerebral arteries and has also been demonstrated to increase cerebral blood flow (33, 34). Its curved shape allows for greater susceptibility to be activated location makes it easier to access through non-invasive routes (33, 35).
Methods
Search Strategy and Selection Criteria
The literature search was conducted in three electronic databases MEDLINE, EMBASE, and SCOPUS, to obtain pre-clinical and clinical studies dating from 1986 to 2019. Search terms included “isch(a)emic,” “brain,” “cerebr*,” “core,” and “penumbra” with intervention approach terms such as “parasympathetic,” “ganglion,” “facial nerve,” “stimulation,” “neuromodulate*,” “electrical acupuncture,” and “magnet*.”
Studies selected for final review included those assessing the effects of stimulation in the ischemic stroke indication, as well as the effects on blood flow in the brain from ganglion stimulation. The inclusion criteria for pre-clinical studies had at least one of the following outcome measures: (1) healthy and ischemic stroke-induced animal models, (2) invasive or non-invasive ganglia stimulation, and/or (3) studies providing at least one outcome measurement of cerebral or collateral blood flow, brain infarct size, neuronal survival, and neurological outcomes. Respectively, the inclusion criteria for clinical studies had at least one of the following outcome measures: (1) healthy human volunteers or patients with ischemic stroke, (2) invasive or non-invasive ganglia stimulation, (3) sham stimulation control group, (4) safety and tolerability of intervention, and/or (5) studies providing at least one outcome measurement of cerebral or collateral blood flow, brain infarct size, neuronal survival, functional and neurological outcomes including but not limited to mRS at 90 days post-stroke.
Pre-clinical and clinical studies were excluded if they evaluated facial nerve stimulation for other indications such as hemorrhagic stroke, chronic headache, migraine, rhinitis, or stroke rehabilitation. Reviews, meta-analyses, observational studies, and commentaries were also excluded.
Risk of Bias Analysis
The final emerging pre-clinical studies were subjected to the Systematic Review Center for Laboratory Animal Experimentation (SYRCLE) risk of bias tool (36). The SYRCLE's tool for assessing risk of bias contains 10 domains which fall under six types of bias; selection bias, performance bias, detection bias, attrition bias, reporting bias, and other. Judgement of bias was indicated as low by a positive (“yes”), high by a negative (“no”), and imprecise (“unclear”) when details reported were insufficient. Physiotherapy Evidence Database (PEDro) scale was selected as the risk of bias assessment tool for clinical studies (37). The PEDro scale consists of 10 items; they are random allocation, concealment of allocation, baseline equivalence, blind subjects, blind therapists, blind assessors, intention to treat analysis, adequate follow-up, between-group statistical analysis, and measurement of data variability and point estimates. Studies with a PEDro score of more than 6 were considered good quality.
Results
The initial search yielded 1,106 studies: 263 duplicates were removed, 843 studies were screened by title and abstract, and 717 studies were deemed irrelevant articles. A total of 126 studies were assessed for eligibility and were subjected to the predefined inclusion and exclusion criteria via full-text review, resulting in the removal of 100 studies. The final emerging 26 studies combined in this review assessed 22 pre-clinical studies and 5 human studies. One publication contained both a human and a pre-clinical trial. Figure 2 outlines the flow of study selection.
Quality of Studies
The methodological quality assessments of included pre-clinical and clinical studies are provided in Tables 1, 2, respectively. Animal studies assessed by the SYRCLE found a prevalence of 41.3% for items classified as “unclear,” and 5.9% for items classified as “no” (Table 1). Human trials assessed by the PEDro scale had an average score of 6.0 with scores ranging from 4 to 7 (Table 2).
Invasive Ganglion Stimulation: Pre-clinical Studies
Of the 22 pre-clinical studies included in this review, a majority (38–43, 46–50, 53–55, 57–59) were invasive electrical stimulation procedures (Table 3). Targeted ganglions included SPG, geniculate ganglion (43, 44, 56), trigeminal ganglion (48–52), vagus nerve (38–40, 45), petrosal nerve (46), nasociliary nerve (47), and dorsal facial area (54).
Key parameters of stimulation protocols included intensity, frequency, pulse, and duration (Tables 3, 4). Several studies sought to find a dose-response relationship or a maximum tolerated frequency and intensity, causing variability between reported stimulation protocols. Electrical stimulations reported intensity in Amps or Volts, ranging from 0.5 to 5mA and 2 to 20V. CBF significantly increased along with blood pressure in a stimulation frequency-dependent manner (41, 46). Even in studies where this dependency was not clear, lower powers and durations were markedly less effective in increasing CBF (56).
Intervention groups undergoing stimulation routinely demonstrated increased CBF. Three studies did not report an increase in CBF, with one not recording CBF (42), one reporting no change (64), and another reporting a decrease following vagus nerve stimulation (39). Goadsby et al. (49) reported the largest shift from baseline following stimulation, reporting a 150% increase following 200 s of stimulation. CBF increased throughout the brain without an obvious preference for the hemisphere ipsilateral to stimulation (54, 56). The ability for stimulation to increase CBF flow was found to be lower in ischemic models compared to CBF increase in health models (39, 40, 53). Animals that underwent invasive stimulation were found to have improved neurological scores in five of the seventeen studies (38, 39, 42, 53, 59). Only one study tracking neurological scores found a decrease following ganglion stimulation, however, they also reported a reduction in infarct volume compared to controls (40). In all studies that reported infarct volume stimulated models performed better (38–40, 42, 53, 55, 59). Bar-Shir et al. (42) found both improved neurological scores and infarct volumes in stimulated models at 8 days compared to control, but no difference in a 28 day follow-up.
Invasive Ganglion Stimulation: Clinical Studies
Four clinical studies using invasive electrical stimulation to treat AIS were identified (Table 5) (60–63). All four targeted the SPG using an implanted device (ISS, BrainsGate, Israel). The procedure involved surgically placing a platinum-iridium stimulator trans-orally through the greater palatine canal in the extracranial pterygopalatine fossa, adjacent to the SPG. A transmitter coil was then placed on the patient's cheek to induce an electronic circuit.
Khurana et al. (60) reported the outcomes of the implant for augmentation of cerebral blood flow trial-1 (ImpACT-1). The prospective, single-arm, feasibility trial was conducted between 2006 and 2009. The trial recruited 98 subjects and reported no major safety concerns. At least one SAE was reported in 23 patients, with three classified as “related” or “possibly related” to the intervention, mostly due to the re-implantation and/or implant misplacement. Pain during stimulation (15/92) was the most frequently reported AE. Secondary efficacy analysis demonstrated a very slight reduction in mRS score (−0.76) between stimulation and historical controls. There was also a significant improvement in functional independence (0–2 mRS) at 90 days, with 48% of stimulated patients (40/84) reaching functional independence compared to 29% (48/165) in the historical control group (60).
Bornstein et al. (61) reported the outcomes of the implant for augmentation of cerebral blood flow trial-24A (ImpACT-24A), the follow-up efficacy trial of the ImpACT-1. The trial used a two-arm, randomized, double-blind design with a sham-control. Within the sham procedures the trans-oral device was implanted but no electrical current was activated. The trial was conducted from 2009 to 2011. The trial recruited patients with evidence of stroke in the anterior circulation, were able to undergo treatment within 24 h, and were ineligible for IV-tPA or endovascular thrombectomy. Bornstein et al. reported enrollment of 327 patients, however six exited prior to implantation and 18 had incomplete implantations. Patients were randomized 2:1, with 202 undergoing stimulation and 101 undergoing sham. Due to issues during implantation a new optic navigation system was introduced midway through the trial, however, only 75.7% of patients in the active group received stimulation. Due to the inconsistency of accurate device placement the trial was ended at the first interim analysis. A modified intent to treat (mITT) analysis looking at patients who underwent at least one full successful active or sham treatment found no benefits in either the primary outcome or any of the secondary endpoints. Post-hoc analysis demonstrated significant improvements within a subsection of the population that had confirmed cortical involvement (CCI), defined as patients with NIHSS score ≥10 and signs of hypodensity or tissue swelling in at least one cortical region on initial imaging (61).
The results of the follow-up trial, ImpACT24B, was conducted between 2011 and 2018 and reported by Bornstein et al. (62). Trial set-up was nearly identical to ImpACT24A, however, the CCI subsection was added as a primary outcome measure of interest. The trial protocol was changed several times, including the introduction of a new guidance system, neurostimulator, implantation technique, and electrical transmitter-control unit. Of the 1,078 patients recruited, 481 underwent stimulation and 519 underwent a sham operation. Again, no benefit in the mITT population was seen in any analysis, however the CCI subgroup showed statistically significant improvements in every endpoint. An inverted U-shape relationship between stimulation intensity and effectiveness in the CCI subgroup, with lower-mid-level stimulation indicated as most effective (62).
The ImpACT24M study, reported by Saver et al. (63), was a single-arm study assessing the effect of two changes to the SPG stimulation procedure required for implanting and optimizing the device. Primary outcomes were difference in 7-day NIH Stroke Scale (NIHSS), and proportion of patients with improvement in stroke symptoms, compared to historical controls (65). The 49 patients that received stimulation demonstrated a median NIHSS improvement of 75% compared to 50% in the historical control. Stimulation was also shown to significantly increase CBF and hand motor function within the cohort, but was not compared to the historical control (63).
There is one ongoing trial (NCT04014621) to determine if 6 h of SPG stimulation using the same procedure in the ImpACT studies will “freeze” the ischemic penumbra in patients with acute ischemic stroke and reduce brain tissue death. The primary outcome measure is volume of core expansion determined by CT scan post SPG stimulation. This trial is expected to complete in spring 2021.
Non-invasive Ganglion Stimulation: Pre-clinical Studies
Six of the 26 publications identified assessed non-invasive interventions to stimulate facial nerves or ganglions (Table 4) (43–45, 51, 52, 56). Three studies used a non-invasive magnetic intervention, only one of which used an ischemic stroke model (43, 44, 56). Four studies used a non-invasive electrical intervention, all of which were tested in healthy models (43, 45, 51, 52). Borsody et al. (43) reported outcomes on both a magnetic and electrical non-invasive intervention, with each intervention targeting separate ganglions.
Magnetic stimulation interventions were primarily deployed through tesla coils located on either side of the subject's head. Magnetic intensities were reported in Tesla, and ranged from 0.5 to 1.9 T in the three studies (43, 44, 56). Non-invasive electrical stimulation was induced over 30–90 min, while the magnetic stimulation was induced over several 5 min increments with a 30 min recovery period.
All non-invasive stimulation studies found an increase in CBF compared to controls (43–45, 51, 52, 56), however, Borsody et al. (43) found a reduction in CBF following non-invasive electrical stimulation through the middle ear. They also reported the largest change in CBF following magnetic stimulation, with a 120% increase compared to baseline using a 10 Hz frequency at 1.5 T. Comparatively, Sanchez et al. (56) found the increase in CBF occurred throughout the brain without obvious preference for the hemisphere ipsilateral to stimulation, however this finding was not reported in any other study. No studies recorded neurological scores, and only two reported on infarct size (44, 45), with non-invasive stimulation significantly reducing infarct size compared to control in both studies.
Non-invasive Ganglion Stimulation: Clinical Studies
Sanchez et al. (56) was the only clinical study that assessed the ability of non-invasive ganglion stimulation (Table 5). The geniculate ganglion was targeted and stimulated at 1, 1.3, 1.6, and 1.9 T in 280 μs pulses for 3 min in health volunteers. All tests were conducted using a single device (VitalFlow, NeuroSpring, California). CBF was found to significantly increase following stimulation, with higher intensity correlating to larger CBF flux. No adverse effects were reported within a 24-h follow-up.
Discussion
There is a substantial amount of pre-clinical and clinical data to suggest that stimulation of the parasympathetic fibers of the facial nerve system is a promising option for rapid AIS treatment. Data from early trials with both invasive and non-invasive approaches have proven safe with very minimal reports of treatment-associated adverse events. Stimulation of the SPG or geniculate ganglion clearly results in increased CBF, and the technological means required to rapidly induce ganglion stimulation are certainly feasible. While the SPG has been the most well-studied ganglion in clinical trials to date, pre-clinical evidence suggests a variety of targets for the potential therapeutic development.
Despite the tremendous upside it poses for treating AIS, ganglion stimulation has yet to demonstrate clinically meaningful outcomes. To date the clinical trials for SPG stimulation via a trans-oral device have suffered from various methodological shortcomings that obscure the reasons behind the lack of positive results. A recurring issue with incorrect device placement resulted in the study protocols for both the ImpACT-24A and ImpACT-24B trials to be repeatedly modified during the trial course. The mid-trial change of the neurostimulator, implantation technique and electrical transmitter-control unit in the ImpACT-24B trial is especially disconcerting and was not addressed in the outcome analysis. Additionally, while the trials claim to be double or triple blinded, it is unclear how successful the blinding can be for the subject and physician when a key element of the intervention's deployment is mild facial discomfort. It is also not clear why the ImpACT-1 and ImpACT-24A were published almost 10 years after completion, and simultaneously with ImpACT-24B and ImpACT-24M.
Current strategies for employing ganglion stimulation to treat AIS suffer from three core limitations: (1) current trial design, (2), time-to-deployment, and (3) accessibility to trained physicians.
The ImpACT trials have focused on independently improving AIS-related disability, but their negative results suggest that tPA and mechanical thrombectomy will remain the gold standard for AIS treatment. It is therefore optimal for facial nerve stimulation to augment and improve the applicability of these existing treatments. There is a clear need for interventions that elongate the current window-of-efficacy (19), with extended door-to-treatment times leaving only 3–22% of AIS patients eligible for mechanical thrombectomy (66), and <5% eligible for tPA (67). Primary outcomes within ganglion stimulation trials should be focused on increasing the efficacy of mechanical thrombectomy for AIS patients that miss the current window-of-efficacy. The upcoming trial (NCT04014621) looking at penumbra “freezing” suggests future ImpACT studies involving invasive approaches may soon follow this suggestion.
However, if the goal of future trials is to stop the evolution of the penumbra prior to recanalization, it is key that ganglion stimulation protocols add minimal delay to the already extensive door-to-treatment time. The design of the current clinical stimulation devices and associated procedures are limited in their ability to accomplish this. The ImpACT-24B trial saw a 1.2-h difference in time from “last known well” on NIHSS to the first stimulation session between sham and control groups; this is likely due to the procedure associated with finding the ideal stimulation intensity, a step inherently missing from the sham procedure. Even without this optimization step, the implantation of the invasive device takes a reported average of 20 min, not including time for setting a sterile environment. This substantially eats away at thrombectomy's window-of-efficacy or the even shorter 60-min window for tPA induction (33, 68). Transfer time from intake hospitals to stroke centers is an ideal time for rapid ganglion stimulation, however, requirements associated with invasive implantation limit their applicability in an ambulatory care setting. Even the non-invasive methods used by Sanchez et al. (56) require TMS coils, which are often large and require substantial energy supply to induce magnetic fields.
The requirement of highly trained physicians, specialized imaging, and sterile fields further limit the currently studied innovations. Current invasive techniques for ganglion stimulation can be employed only by specialized physicians, demonstrated by 58% of implantations in the ImpACT-24B trial being performed by surgeons and anesthesiologists, with the remaining performed by neurologists (62). Implanting the device also requires an advanced optical guidance system that would likely only be available to stroke centers, limiting its ability to be implemented in peripheral hospitals prior to transfer.
The research to date on ganglion stimulation is extremely promising, but further innovation is required to find a workable integration of ganglion stimulation into current clinical procedures. An inherent focus must be on limiting the evolution of the penumbra and minimizing the size of the ischemic core, with the primary goal of elongating the window-of-efficacy for mechanical thrombectomy or tPA. Solutions should look to benefit these current treatment options instead of supplanting them, and find a way to be used primarily at peripheral non-stroke centers.
Data Availability Statement
The original contributions presented in the study are included in the article/supplementary material, further inquiries can be directed to the corresponding author/s.
Author Contributions
AC is the guarantor. TB conceptualized the study. TB and JR developed the search strategy and drafted the manuscript. JR extracted the data. All authors contributed to the development of the selection criteria, risk of bias assessment strategy, and data extraction criteria and also read, provided feedback, and approved the final manuscript.
Conflict of Interest
The authors declare that the research was conducted in the absence of any commercial or financial relationships that could be construed as a potential conflict of interest.
Publisher's Note
All claims expressed in this article are solely those of the authors and do not necessarily represent those of their affiliated organizations, or those of the publisher, the editors and the reviewers. Any product that may be evaluated in this article, or claim that may be made by its manufacturer, is not guaranteed or endorsed by the publisher.
References
1. Benjamin EJ, Muntner P, Alonso A, Bittencourt MS, Callaway CW, Carson AP. Heart disease and stroke statistics-2019 UPDATE: A REPORT FROM the American Heart Association. Circulation. (2019) 139:e56–528. doi: 10.1161/CIR.0000000000000659
2. Subramanian G, Silva J, Silver FL, Fang J, Karpal MK, Oczkowski W. Risk factors for posterior compared to anterior ischemic stroke: an observational study of the Registry of the Canadian Stroke Network. Neuroepidemiology. (2009) 33:12–6. doi: 10.1159/000209282
3. Zürcher E, Richoz B, Faouzi M, Michel P. Differences in ischemic anterior and posterior circulation strokes: a clinico-radiological and outcome analysis. J Stroke Cerebrovasc Dis. (2019) 28:710–8. doi: 10.1016/j.jstrokecerebrovasdis.2018.11.016
4. Astrup J, Siesjö BK, Symon L. Thresholds in cerebral ischemia - the ischemic penumbra. Stroke. (1981) 12:723–5. doi: 10.1161/01.STR.12.6.723
5. Ford AL, An H, Vo KD, Lin, Lee W, Lee JM. Defining the ischemic penumbra using hyperacute neuroimaging: deriving quantitative ischemic thresholds. Transl Stroke Res. (2012) 3:198–204. doi: 10.1007/s12975-012-0181-x
6. Baron J-C. Protecting the ischaemic penumbra as an adjunct to thrombectomy for acute stroke. Nat Rev Neurol. (2018) 14:325–37. doi: 10.1038/s41582-018-0002-2
7. Desai SM, Rocha M, Jovin TG, Jadhav AP. High variability in neuronal loss: time is brain, requantified. Stroke. (2019) 50:34–7. doi: 10.1161/STROKEAHA.118.023499
8. Meretoja A, Keshtkaran M, Tatlisumak T, Donnan GA, Churilov L. Endovascular therapy for ischemic stroke: save a minute—save a week. Neurology. (2017) 88:2123–7. doi: 10.1212/WNL.0000000000003981
9. Prabhakaran S, Ruff I, Bernstein RA. Acute stroke intervention: a systematic review. JAMA. (2015) 313:1451–62. doi: 10.1001/jama.2015.3058
10. Lees KR, Bluhmki E, von Kummer R, Brott TG, Toni D, Grotta JC. Time to treatment with intravenous alteplase and outcome in stroke: an updated pooled analysis of ECASS, ATLANTIS, NINDS, and EPITHET trials. Lancet. (2010) 375:1695–703. doi: 10.1016/S0140-6736(10)60491-6
11. The NINDS rt-PA Stroke Study Group. Tissue plasminogen activator for acute ischemic stroke. N Engl J Med. (1995) 333:1581–7. doi: 10.1056/NEJM199512143332401
12. Hacke W, Kaste M, Bluhmki E, Brozman M, Davalos A, Guidetti D. Thrombolysis with alteplase 3 to 4.5 hours after acute ischemic stroke. N Engl J Med. (2008) 359:1317–29. doi: 10.1056/NEJMoa0804656
13. Bluhmki E, Chamorro Á, Dávalos A, Machnig T, Sauce C, Wahlgren N. Stroke treatment with alteplase given 3·0–4·5 h after onset of acute ischaemic stroke (ECASS III): additional outcomes and subgroup analysis of a randomised controlled trial. Lancet Neurol. (2009) 8:1095–102. doi: 10.1016/S1474-4422(09)70264-9
14. Del Zoppo GJ, Saver JL, Jauch EC, Adams HP. Expansion of the time window for treatment of acute ischemic stroke with intravenous tissue plasminogen activator: a science advisory from the American Heart Association/American Stroke Association. Stroke. (2009) 40:2945–8. doi: 10.1161/STROKEAHA.109.192535
15. Powers WJ, Rabinstein AA, Ackerson T, Adeoye OM, Bambakidis NC, Becker K. 2018 Guidelines for the early management of patients with acute ischemic stroke: a guideline for healthcare professionals from the American Heart Association/American Stroke Association. Stroke. (2018) 49:e46–110. doi: 10.1161/STR.0000000000000163
16. Nogueira RG, Jadhav AP, Haussen DC, Bonafe A, Budzik RF, Bhuva P. Thrombectomy 6 to 24 hours after stroke with a mismatch between deficit and infarct. N Engl J Med. (2018) 378:11–21. doi: 10.1056/NEJMoa1706442
17. Saver JL, Fonarow GC, Smith EE, Reeves MJ, Grau-Sepulveda MV, Pan W. Time to treatment with intravenous tissue plasminogen activator and outcome from acute ischemic stroke. JAMA. (2013) 309:2480–8. doi: 10.1001/jama.2013.6959
18. Jauch EC, Cucchiara B, Adeoye O, Meurer W, Brice J, Chan YYF, et al. Part 11: adult stroke: 2010 American Heart Association Guidelines for Cardiopulmonary Resuscitation and Emergency Cardiovascular Care. Circulation. (2010) 122:S818–28. doi: 10.1161/CIRCULATIONAHA.110.971044
19. Fonarow GC, Smith EE, Saver JL, Reeves MJ, Bhatt DL, Grau-Sepulveda MV. Timeliness of tissue-type plasminogen activator therapy in acute ischemic stroke: patient characteristics, hospital factors, and outcomes associated with door-to-needle times within 60 minutes. Circulation. (2011) 123:750–8. doi: 10.1161/CIRCULATIONAHA.110.974675
20. Mokin M, Abou-Chebl A, Castonguay AC, Nogueira RG, English JD, Farid H. Real-world stent retriever thrombectomy for acute ischemic stroke beyond 6 hours of onset: analysis of the NASA and TRACK registries. J Neurointerv Surg. (2019) 11:334–7. doi: 10.1136/neurintsurg-2018-014272
21. Berkhemer OA, Fransen PSS, Beumer D, van den Berg LA, Lingsma HF, Yoo AJ. A randomized trial of intraarterial treatment for acute ischemic stroke. N Engl J Med. (2015) 372:11–20. doi: 10.1056/NEJMoa1411587
22. Goyal M, Menon BK, van Zwam WH, Dipple DW, Mitchell PJ, Demchuk AM. Endovascular thrombectomy after large-vessel ischaemic stroke: a meta-analysis of individual patient data from five randomised trials. Lancet. (2016) 387:1723–31. doi: 10.1016/S0140-6736(16)00163-X
23. Powers WJ, Derdeyn CP, Biller J, Coffey CS, Hoh BL, Jauch EC. 2015 American Heart Association/American Stroke Association Focused Update of the 2013 guidelines for the early management of patients with acute ischemic stroke regarding endovascular treatment. Stroke. (2015) 46:3020–35. doi: 10.1161/STR.0000000000000074
24. Kim HY, Singhal AB, Lo EH. Normobaric hyperoxia extends the reperfusion window in focal cerebral ischemia. Ann Neurol. (2005) 57:571–5. doi: 10.1002/ana.20430
25. Winship IR. Cerebral collaterals and collateral therapeutics for acute ischemic stroke. Microcirculation. (2015) 22:228–36. doi: 10.1111/micc.12177
26. Shuaib A, Butcher K, Mohammad AA, Saqqur M, Liebeskind DS. Collateral blood vessels in acute ischaemic stroke: a potential therapeutic target. Lancet Neurol. (2011) 10:909–21. doi: 10.1016/S1474-4422(11)70195-8
27. Ho KWD, Przkora R, Kumar S. Sphenopalatine ganglion: block, radiofrequency ablation and neurostimulation - a systematic review. J Headache Pain. (2017) 18:118. doi: 10.1186/s10194-017-0826-y
28. Tepper SJ, Caparso A. Sphenopalatine Ganglion (SPG): stimulation mechanism, safety, and efficacy. Headache. (2017) 57(Suppl. 1):14–28. doi: 10.1111/head.13035
29. Vuksanovic-Bozaric A, Vukcevic B, Abramovic M, Vukcevic N, Popovic N, Radunovic M. The pterygopalatine fossa: morphometric CT study with clinical implications. Surg Radiol Anat. (2019) 41:161–8. doi: 10.1007/s00276-018-2136-8
30. Goosmann MM, Dalvin M. Anatomy, head and neck, deep petrosal nerve. In: StatPearls. Treasure Island, FL: StatPearls Publishing (2018).
31. Talman WT, Nitschke Dragon D. Neuronal nitric oxide mediates cerebral vasodilatation during acute hypertension. Brain Res. (2007) 1139:126–32. doi: 10.1016/j.brainres.2007.01.008
32. DoŽić A, Cetković M, Marinković S, Mitrović D, Grujičić M, Mićović M. Vascularisation of the geniculate ganglion. Folia Morphol. (2014) 73:414–21. doi: 10.5603/FM.2014.0063
33. Borsody MK, Sacristan E. Facial nerve stimulation as a future treatment for ischemic stroke. Brain Circ. (2016) 2:164. doi: 10.4103/2394-8108.195281
34. Forbes HS, Nason GI, Cobb S, Wortman RC. Cerebral circulation: XLV. vasodilation in the PIA following stimulation of the geniculate ganglion. Arch NeurPsych. (1937) 37:776–81. doi: 10.1001/archneurpsyc.1937.02260160076008
35. Rotem A, Moses E. Magnetic stimulation of curved nerves. IEEE Trans Biomed Eng. (2006) 53:414–20. doi: 10.1109/TBME.2005.869770
36. Hooijmans CR, Rovers MM, de Vries RBM, Leenaars M, Ritskes-Hoitinga M, Langendam MW. SYRCLE's risk of bias tool for animal studies. BMC Med Res Methodolo. (2014) 14:43. doi: 10.1186/1471-2288-14-43
37. Bhogal SK, Teasell RW, Foley NC, Speechley MR. The PEDro scale provides a more comprehensive measure of methodological quality than the Jadad Scale in stroke rehabilitation literature. J Clin Epidemiol. (2005) 58:668–73. doi: 10.1016/j.jclinepi.2005.01.002
38. Ay I, Lu J, Ay H, Sorensen AG. Vagus nerve stimulation reduces infarct size in rat focal cerebral ischemia. Neurosci Lett. (2009) 459:147–51. doi: 10.1016/j.neulet.2009.05.018
39. Ay I, Sorensen AG, Ay H. Vagus nerve stimulation reduces infarct size in rat focal cerebral ischemia: an unlikely role for cerebral blood flow. Brain Res. (2011) 1392:110–5. doi: 10.1016/j.brainres.2011.03.060
40. Ay I, Ay H. Ablation of the sphenopalatine ganglion does not attenuate the infarct reducing effect of vagus nerve stimulation. Auton Neurosci. (2013) 174:31–5. doi: 10.1016/j.autneu.2012.12.001
41. Ayajiki K, Fujioka H, Shinozaki K, Okamura T. Effects of capsaicin and nitric oxide synthase inhibitor on increase in cerebral blood flow induced by sensory and parasympathetic nerve stimulation in the rat. J Appl Physiol. (2005) 98:1792–8. doi: 10.1152/japplphysiol.00690.2004
42. Bar-Shir A, Shemesh N, Nossin-Manor R, Cohen Y. Late stimulation of the sphenopalatine-ganglion in ischemic rats: improvement in N-acetyl-aspartate levels and diffusion weighted imaging characteristics as seen by MR. J Magn Reson Imaging. (2010) 31:1355–63. doi: 10.1002/jmri.22110
43. Borsody MK, Yamada C, Bielawski D, Heaton T, Lyeth B, Garcia A. Effect of pulsed magnetic stimulation of the facial nerve on cerebral blood flow. Brain Res. (2013) 1528:58–67. doi: 10.1016/j.brainres.2013.06.022
44. Borsody MK, Yamada C, Bielawski D, Heaton T, Prado FC, Garcia A. Effects of noninvasive facial nerve stimulation in the dog middle cerebral artery occlusion model of ischemic stroke. Stroke. (2014) 45:1102–7. doi: 10.1161/STROKEAHA.113.003243
45. Chi L, Du K, Liu D, Bo Y, Li W. Electroacupuncture brain protection during ischemic stroke: a role for the parasympathetic nervous system. J Cereb Blood Flow Metab. (2018) 38:479–91. doi: 10.1177/0271678X17697988
46. D'Alecy LG, Rose CJ. Parasympathetic cholinergic control of cerebral blood flow in dogs. Circ Res. (1977) 41:324–31. doi: 10.1161/01.RES.41.3.324
47. Edvinsson L, Mulder H, Goadsby PJ, Uddman R. Calcitonin gene-related peptide and nitric oxide in the trigeminal ganglion: cerebral vasodilatation from trigeminal nerve stimulation involves mainly calcitonin gene-related peptide. J Autonomic Nervous Syst. (1998) 70:15–22. doi: 10.1016/S0165-1838(98)00033-2
48. Goadsby PJ, Lambert GA, Lance JW. Stimulation of the trigeminal ganglion increases flow in the extracerebral but not the cerebral circulation of the monkey. Brain Res. (1986) 381:63–7. doi: 10.1016/0006-8993(86)90690-6
49. Goadsby PJ, Hoskin KL. Cerebral blood flow is not coupled to neuronal activity during stimulation of the facial nerve vasodilator system. Brain Res. (1994) 647:192–8. doi: 10.1016/0006-8993(94)91317-X
50. Goadsby PJ, Knight YE, Hoskin KL, Butler P. Stimulation of an intracranial trigeminally-innervated structure selectively increases cerebral blood flow. Brain Res. (1997) 751:247–52. doi: 10.1016/S0006-8993(96)01344-3
51. Gulturk S, Gedik R, Develioglu H, Oztoprak I, Cetin A. Assessment of the outcomes of cerebral blood flow measurements after electrical stimulation of upper right incisor tooth in rabbits. Int J Neurosci. (2009) 119:1292–302. doi: 10.1080/00207450802335610
52. Gürelik M, Karadag O, Polat S, Özüm Ü, Aslan A, Gürelik B. The effects of the electrical stimulation of the nasal mucosa on cortical cerebral blood flow in rabbits. Neurosci Lett. (2004) 365:210–3. doi: 10.1016/j.neulet.2004.04.079
53. Henninger N, Fisher M. Stimulating circle of Willis nerve fibers preserves the diffusion-perfusion mismatch in experimental stroke. Stroke. (2007) 38:2779–86. doi: 10.1161/STROKEAHA.107.485581
54. Kuo JS, Chyi T, Yang MC, Chai CY. Changes in intra- and extracranial tissue blood flow upon stimulation of a reticular area dorsal to the facial nucleus in cats. Clin Exp Pharmacol Physiol. (1995) 22:87–93. doi: 10.1111/j.1440-1681.1995.tb01961.x
55. Levi H, Schoknecht K, Prager O, Chassidim Y, Weissberg I, Serlin Y. Stimulation of the sphenopalatine ganglion induces reperfusion and blood-brain barrier protection in the photothrombotic stroke model. PLoS ONE. (2012) 7:e39636. doi: 10.1371/journal.pone.0039636
56. Sanchez O, García A, Castro-Prado F, Perez M, Lara-Estrada R, Ramirez-Meza M. Facial nerve stimulation in normal pigs and healthy human volunteers: transitional development of a medical device for the emergency treatment of ischemic stroke. J Transl Med. (2018) 16:27. doi: 10.1186/s12967-018-1398-6
57. Seylaz J, Hara H, Pinard E, Mraovitch S, Edvinsson L. Effect of stimulation of the sphenopalatine ganglion on cortical blood flow in the rat. J Cereb Blood Flow Metab. (1988) 8:875–8. doi: 10.1038/jcbfm.1988.145
58. Suzuki N, Hardebo JE, Kåhrström J, Owman C. Selective electrical stimulation of postganglionic cerebrovascular parasympathetic nerve fibers originating from the sphenopalatine ganglion enhances cortical blood flow in the rat. J Cereb Blood Flow Metab. (1990) 10:383–91. doi: 10.1038/jcbfm.1990.68
59. Talman WT, Corr J, Nitschke Dragon D, Wang D. Parasympathetic stimulation elicits cerebral vasodilatation in rat. Auton Neurosci. (2007) 133:153–7. doi: 10.1016/j.autneu.2006.12.002
60. Khurana D, Kaul S, Schneider D, Csanyi A, Adam I, Ichaporia NR. Implant for Augmentation of Cerebral Blood Flow Trial-1 (ImpACT-1). A single-arm feasibility study evaluating the safety and potential benefit of the Ischemic Stroke System for treatment of acute ischemic stroke. PLoS ONE. (2019) 14:e0217472. doi: 10.1371/journal.pone.0217472
61. Bornstein NM, Saver JL, Diener H-C, Gorelick PB, Shuaib A, Solberg Y. Sphenopalatine ganglion stimulation to augment cerebral blood flow: a randomized, sham-controlled trial. Stroke. (2019) 50:2108–17. doi: 10.1161/STROKEAHA.118.024582
62. Bornstein NM, Saver JL, Diener HC, Borelick PB, Shuaib A, Solberg Y. An injectable implant to stimulate the sphenopalatine ganglion for treatment of acute ischaemic stroke up to 24 h from onset (ImpACT-24B): an international, randomised, double-blind, sham-controlled, pivotal trial. Lancet. (2019) 394:219–29. doi: 10.1016/S0140-6736(19)31192-4
63. Saver JL, Kharaishvili N, Janelidze T, Beridze M, Zarqua N, Solberg Y. Refined sphenopalatine ganglion stimulator placement and intensity setting to augment blood flow and neurologic function. Stroke. (2019) 50:3512–8. doi: 10.1161/STROKEAHA.119.027177
64. Goadsby PJ, Uddman R, Edvinsson L. Cerebral vasodilatation in the cat involves nitric oxide from parasympathetic nerves. Brain Res. (1996) 707:110–8. doi: 10.1016/0006-8993(95)01206-0
65. Saver JL, Gornbein J, Starkman S. Graphic reanalysis of the two NINDS-tPA trials confirms substantial treatment benefit. Stroke. (2010) 41:2381–90. doi: 10.1161/STROKEAHA.110.583807
66. Mokin M, Ansari SA, McTaggart RA, Bulsara K, Goyal M, Chen M. Indications for thrombectomy in acute ischemic stroke from emergent large vessel occlusion (ELVO): report of the SNIS Standards and Guidelines Committee. J Neurointerv Surg. (2019) 11:215–20. doi: 10.1136/neurintsurg-2018-014640
67. Mendez AA, Samaniego EA, Sheth SA, Dandapat S, Hasan D, Limaye KS. Update in the early management and reperfusion strategies of patients with acute ischemic stroke. Crit Care Res Pract. (2018) 2018:1–15. doi: 10.1155/2018/9168731
68. Kaul S, Khurana D, Csani A, Bornstein NM. Implant for augmentation of cerebral blood flow clinical trial-(ImpACT-1). An interim analysis of safety and effectiveness of the Neuropath IS system in the treatment of acute ischemic stroke. In: Stroke. Philadelphia, PA: Lippincott Williams & Wilkins (2008). p. 56.
Keywords: animal studies, cerebral blood flow, cerebrovascular disease, vascular surgery, stroke
Citation: Baker TS, Robeny J, Cruz D, Bruhat A, Iloreta A-M, Costa A and Oxley TJ (2021) Stimulating the Facial Nerve to Treat Ischemic Stroke: A Systematic Review. Front. Neurol. 12:753182. doi: 10.3389/fneur.2021.753182
Received: 04 August 2021; Accepted: 01 September 2021;
Published: 18 November 2021.
Edited by:
Mike Modo, University of Pittsburgh, United StatesReviewed by:
Aaron Del Pozo Sanz, Health Research Institute of the Hospital Clínico San Carlos (IdISSC), SpainJames St. John, Griffith University, Australia
Copyright © 2021 Baker, Robeny, Cruz, Bruhat, Iloreta, Costa and Oxley. This is an open-access article distributed under the terms of the Creative Commons Attribution License (CC BY). The use, distribution or reproduction in other forums is permitted, provided the original author(s) and the copyright owner(s) are credited and that the original publication in this journal is cited, in accordance with accepted academic practice. No use, distribution or reproduction is permitted which does not comply with these terms.
*Correspondence: Turner S. Baker, dHVybmVyLmJha2VyJiN4MDAwNDA7aWNhaG4ubXNzbS5lZHU=; orcid.org/0000-0002-3440-9563
†These authors share first authorship