- 1Pathfinder Brain SPECT Imaging, Deerfield, IL, United States
- 2The International Society of Applied Neuroimaging (ISAN), Denver, CO, United States
- 3The Synaptic Space, Inc., Denver, CO, United States
- 4Neuro-Luminance, Inc., Denver, CO, United States
- 5Dr. Theodore Henderson, Inc., Denver, CO, United States
- 6Good Lion Imaging, Columbia, SC, United States
Brain perfusion single photon emission computed tomography (SPECT) scans were initially developed in 1970's. A key radiopharmaceutical, hexamethylpropyleneamine oxime (HMPAO), was originally approved in 1988, but was unstable. As a result, the quality of SPECT images varied greatly based on technique until 1993, when a method of stabilizing HMPAO was developed. In addition, most SPECT perfusion studies pre-1996 were performed on single-head gamma cameras. In 1996, the Therapeutics and Technology Assessment Subcommittee of the American Academy of Neurology (TTASAAN) issued a report regarding the use of SPECT in the evaluation of neurological disorders. Although the TTASAAN report was published in January 1996, it was approved for publication in October 1994. Consequently, the reported brain SPECT studies relied upon to derive the conclusions of the TTASAAN report largely pre-date the introduction of stabilized HMPAO. While only 12% of the studies on traumatic brain injury (TBI) in the TTASAAN report utilized stable tracers and multi-head cameras, 69 subsequent studies with more than 23,000 subjects describe the utility of perfusion SPECT scans in the evaluation of TBI. Similarly, dementia SPECT imaging has improved. Modern SPECT utilizing multi-headed gamma cameras and quantitative analysis has a sensitivity of 86% and a specificity of 89% for the diagnosis of mild to moderate Alzheimer's disease—comparable to fluorodeoxyglucose positron emission tomography. Advances also have occurred in seizure neuroimaging. Lastly, developments in SPECT imaging of neurotoxicity and neuropsychiatric disorders have been striking. At the 25-year anniversary of the publication of the TTASAAN report, it is time to re-examine the utility of perfusion SPECT brain imaging. Herein, we review studies cited by the TTASAAN report vs. current brain SPECT imaging research literature for the major indications addressed in the report, as well as for emerging indications. In Part II, we elaborate technical aspects of SPECT neuroimaging and discuss scan interpretation for the clinician.
Introduction
In 1996, the Therapeutics and Technology Assessment Subcommittee of the American Academy of Neurology (TTASAAN) issued a report regarding the use of single photon emission computed tomography (SPECT) in functional brain imaging (1). Although the TTASAAN report was published in January 1996, it was completed and approved for publication in October of 1994. Consequently, the referenced brain SPECT studies relied upon to derive the conclusions of the TTASAAN report predominately pre-dated the introduction of stabilized radiopharmaceuticals in 1993. In fact, of the 97 references in the TTASAAN report, only 12 are from 1993 or later. Furthermore, the early gamma cameras used for SPECT neuroimaging were single-headed cameras with limited resolution. Thus, the conclusions provided in the TTASAAN report were premature.
In the 25 years since the TTASAAN report, the American Academy of Neurology (AAN) has never deemed to re-examine their premature position on the use of brain perfusion SPECT in the evaluation of traumatic brain injury (TBI), stroke, seizure disorders, dementia, and neuropsychiatric conditions. Despite extensive advances in technology, software, and technique, as well as, for example, the publication of over 120 research studies and articles and published data from over 23,000 subjects on the use of SPECT just in the evaluation of TBI, the AAN has largely taken an overcautious, and sometimes dismissive, position toward the use of SPECT scans. In contrast, the European Association of Nuclear Medicine (EANM) (2) has deemed that brain perfusion SPECT scans are appropriate for the evaluation of TBI and that SPECT scans have predictive value in the clinical outcome of TBI (2). Moreover, the Canadian Association of Nuclear Medicine recently has issued guidelines on the use of SPECT imaging in the evaluation of TBI, stroke, dementia, neurotoxicity, and psychiatric conditions (3).
Curiously, the authors of the TTASAAN report made it clear that this report was neither a position paper nor a solidified assessment of SPECT. The authors unambiguously articulated that the assessment was to be revised as the field advanced. Quoting from the opening paragraph of the TTASAAN report (1):
“This paper, provided to the Academy membership as an educational tool, will be subjected to periodic revision as new information becomes available.”
The periodic revision has never occurred. In many ways, this is the equivalent of assessing Xray computed tomography (CT) in its infancy and then never re-assessing its merit thereafter. The first CT scanner became commercially available in 1972. CT neuroimaging was met with skepticism among neurologists (4). CT neuroimaging was deemed, “a passing fancy.” The American Neurological Association published a report in 1975 stating the new method of imaging the nervous system would greatly reduce the need for neurologists. Numerous articles in the late 1970's criticized CT neuroimaging for its failure to accurately detect brain pathology (5–8) during the early years of its clinical use. In 1975, a commission of the AAN reported
“A CT scan can give a more accurate localization in far less time than a neurologist. Ultrasound of the carotid arteries can localize and indicate the degree of stenosis more accurately than a clinician with a stethoscope. The portent for the future is that neurologists who rely exclusively on their wits and their pins and hammers, unaware the machine age has finally come to neurology, may become obsolete” (9).
Despite the initial skepticism and concern that CT neuroimaging would replace the need for neurologists, the use of CT scanning expanded exponentially. CT neuroimaging is now a cornerstone of neurological evaluations, particularly in the acute setting. Nonetheless, as will be elaborated below, CT is very limited in what it can reveal about brain function.
In the same 25-year period, functional magnetic resonance imaging (fMRI) has undergone explosive growth. Hundreds of millions of dollars in research funding in the United States have been poured into fMRI studies. According to PubMed, over 40,000 research articles have been published. Despite the massive investment of time and effort, fMRI has yet to provide a clinically useful diagnostic tool for assessing brain function in individual cases. Indeed, the American Psychiatric Association recently issued a position paper (10) stating
“(fMRI) neuroimaging has yet to have a significant impact on the diagnosis or treatment of individual patients in clinical settings.”
Moreover, a recent analysis of post-processing statistical validity revealed that a potentially staggering 70% of fMRI studies had false positive results (11), which could mean much of the fMRI research findings are invalid. The AAN has seemingly scrupulously ignored this serious caveat to the application of fMRI in research or clinical practice (12).
Similarly, extensive funding and effort has been invested in diffusion tensor imaging. However, it has been plagued by inconsistencies across centers due to technical elements arising from different hardware, competing software, varying sequences, dissimilar reconstruction algorithms, and technique. For example, eddy current distortion is often found to be larger than the acquisition voxel size (13). While a thorough analysis of diffusion tensor imaging is beyond the scope of this review, it will suffice to say that anisotropy can be either elevated or depressed following TBI. There have been numerous studies with contradictory findings (14, 15). Variations in technique or the unreliability of diffusion tensor imaging in TBI has been suggested as the cause for the conflicting data (14, 15).
Thus, while the AAN, and neurologists in general, have distanced themselves from SPECT neuroimaging based, in part, on the now outdated TTASAAN report and embraced other technologies, they have been left with technically flawed methods of visualizing brain function (11, 14, 15). Meanwhile, extensive advancements have been made in the practice and technology of perfusion SPECT neuroimaging and massive databases have been accumulated. Together, these factors lead to the need to re-examine the policy and practice set forth by the AAN in 1996.
Defining SPECT
SPECT is a type of nuclear medicine scan to create 2-dimensional (2-D) and 3-dimensional (3-D) pictures of functional processes within the patient's body. A radiopharmaceutical is administered to detect specific activities within the body. A gamma camera measures the radiation emitted by the radiopharmaceutical and rotates around the patient to acquire a set of 2-D planar images. Using a reconstruction technique, these planar images are reconstructed into a 3-D volume from which slices at various angles can be extracted to visualize the distribution of activity within the patient's body. In the case of perfusion SPECT neuroimaging, the radiopharmaceutical is transported via the bloodstream and is quickly taken up by neurons (16) (see below), such that the uptake of radiotracer is dependent upon, and therefore reflective of, the regional cerebral blood flow (rCBF). Cerebral blood flow at the level of cortical columns or functional subregions is regulated by neuronal activity. Increased activity induces increased local blood flow, while decreased activity results in reduced blood flow. The detection of the radiotracer uptake throughout the brain allows the clinician to identify both areas of hypoperfusion (hypo-functioning) and of increased perfusion (hyper-functioning). SPECT post-processing generates tomograms and a 3-D mapped representation of the brain, ideally with color-coded intensities proportional to rCBF which correlate with the brain function in that region.
Assessment of Guidelines and Implications
The Technical Aspects of Early SPECT Neuroimaging
Brain perfusion SPECT scans were initially developed in the 1970's. After the development of the Anger scintillation detector in 1950 (17) and the invention of the Anger circuitry in 1969 (17), several groups developed scintillation cameras. Paul Harper et al. with the University of Chicago first explored transaxial tomography using the Anger camera (18). The first whole body SPECT cameras were developed by 1976 (17). Brill et al. at Vanderbilt University and Jaszczak et al. at Searle Radiographics independently developed brain-specific gamma cameras.
While the technology of the scintillation camera was ongoing, the development of brain specific tracers was advancing rapidly. The early SPECT cerebral blood flow studies utilized 133Xenon which can provide a quantitative measure of cerebral blood flow. However, 133Xenon has relatively low energy and required a special breathing apparatus which was cumbersome and uncomfortable for the patient. Tracers with high energy gamma radiation were sought and 123Iodine (123I) or 99mTechnetium (99mTc) became the primary candidate radiolabels. An 123I tracer, 123I-N-isopropyl-iodo-amphetamine (123I-IMP), was developed in 1980 for cerebral perfusion using iodinated amphetamine. It could be tagged with either 123I or 125I. This tracer showed high brain extraction and linear uptake over a wide range of blood flow rates (19). It remains in use today; however, there were some distinct disadvantages to this tracer. The first is the use of 123I requires pretreatment to protect the thyroid. The second is that the tracer is redistributed from the lungs to the brain over the first 20 min after injection leading to a smearing of activity over time. Brain areas demonstrating high cerebral blood flow at the time of injection may not maintain that level of blood flow during the 20-min interval when 123I-IMP is cleared from the lungs and accumulates in the brain (16).
The more lipophilic agent propylene amine oxime, which could be labeled with 99mTc, was explored as a brain perfusion tracer during the late 1970's and early 1980's. Initial agents had rapid clearing and so required fast imaging. In 1985, a methylated version of the agent was developed, which had almost ideal properties—99mTc- hexamethylpropyleneamine oxime (HMPAO) (20). First, 99mTc has a long half-life of 6 h and can be made independent of a cyclotron. Second, neither 99mTc nor HMPAO interfere with any biological processes in the body, unlike 123I or the amphetamine tracer. Third, HMPAO is retained in the cell due to conversion to a less lipophilic form, which reduces washout and prolongs the window for scanning to several hours (16, 20–22). Fourth, the accumulation of HMPAO in the brain is very rapid, being virtually complete in 40 s and it maintains a fixed distribution after 5 min (16, 22). Thus, the scan measures the cerebral blood flow at the time of injection, not at the time the scan is actually performed. In essence, a SPECT scan captures a frozen image of brain function at the time of injection (16, 22). This makes 99mTc-HMPAO ideal for capturing brain activity during transient conditions (e.g., seizures, transient ischaemic attacks) or psychological challenges (e.g., concentration tasks). At very high perfusion rates, 99mTc-HMPAO accumulation and back diffusion becomes disproportional; thus, 99mTc-HMPAO uptake is non-linear compared to 133Xenon (16, 23). Lassen et al. (23) proposed a correction algorithm which has been shown to closely approximate rCBF in comparison to 15CO2 PET (24). Nevertheless, accumulation is linear within the normal physiological range in the human brain (16, 23).
Limited Stability of HMPAO Pre-1996
At the time that 99mTc-HMPAO was originally approved by the FDA in 1988, a key technical flaw still remained. The agent was unstable and would decompose rapidly after reconstitution. Realistically, it was only viable for 30 min after reconstitution (16). The work of quality checking, measuring the radioactivity, calculating and drawing up the dose, and patient preparation had to be accomplished very hastily prior to injecting the patient. As a result, the quality of the SPECT images varied greatly based on the deftness and technique of the technologist handling the tracer. In 1993, the addition of methylene blue proved effective in stabilizing HMPAO for several hours after reconstitution. This contributed to improved scan quality.
HMPAO was not truly stabilized for clinical application until 1993. In addition, most of the brain SPECT perfusion studies pre-1994 were performed on single-head gamma cameras. Since, then the quality of SPECT neuroimaging has greatly improved with the use of multi-head gamma cameras (See Figure 1). In addition, refinements in post-processing, as well as the introduction of statistical comparison to normative databases, have greatly enhanced the quality and diagnostic capacity of SPECT scans.
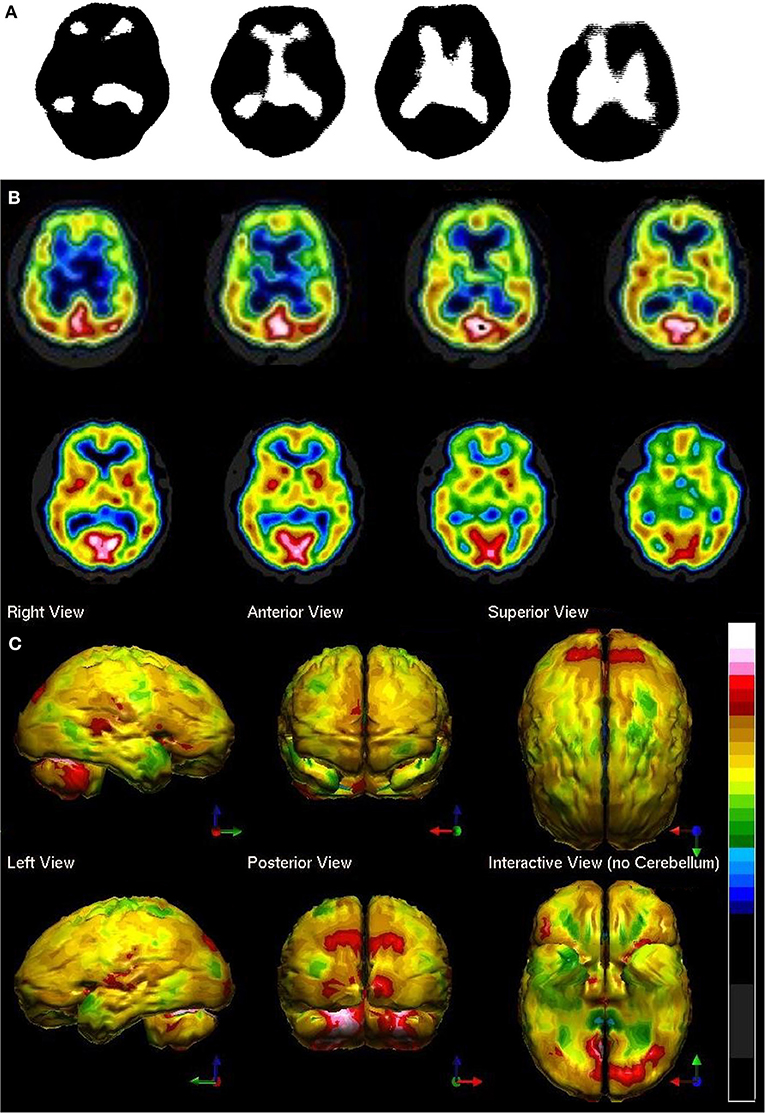
Figure 1. Perfusion SPECT scan of normal control. (A) Four horizontal tomograms from a 99mTc-ECD perfusion SPECT scan performed on a single-headed gamma camera in 1989 [The figure was originally published in JNM. © SNMMI (25)]. (B) Eight horizontal tomograms from a modern 99mTc-HMPAO perfusion SPECT scan obtained from a dual-headed gamma camera. The color scale is scaled relative to the patient's mean cerebral perfusion. Mean blood flow (72%) is in yellow. Color shifts occur at approximately every 0.5 SD (3%) relative to the patient's mean. Details of the brain can be appreciated, including the thalamus, head of the caudate nuclei, lentiform nuclei, anterior cingulate gyri, and distinct cortical regions. (C) The modern SPECT scan displayed in 3-dimensional reconstruction. Increased perfusion in the visual cortex and the slightly lower average perfusion level in the temporal lobes bilaterally can be appreciated. The color scale is the same as (B).
Display Limitations
Early SPECT studies were compromised by limitations of post-processing and display. Methods for correcting energy attenuation were imprecise. Displays were often essentially binomial—-above a certain threshold (1) the display showed black and below that threshold (0) the display showed an absence of black, as illustrated in Figures 1A, 3C. Some effort at a gray scale was introduced by Ismael Mena and his group at Harbor UCLA as illustrated in Figure 3B. The challenge with SPECT scans is that they are detecting changes in degree of function, which is displayed as changes in the intensity of the signal. Greyscale permits finer details to be seen, making it ideal for anatomical MRI; however, when discerning changes in intensity over large areas, color displays improve detection. For example, Stapleton et al. (29) examined this issue using SPECT scan data. The study involved the use of scan data from one-half of a brain, which was then inverted to create a symmetrical template of a brain. Then an artificial defect was created in the cerebellum by decreasing pixel values in the designated area by 1–12.5%. This construct was displayed in greyscale, a red color scale similar to “heated object,” and blue/green/red where low counts were blue, mid counts were green and high counts were red. Despite the expressed bias toward greyscale among the radiologists tested, subject readers detected the artificial lesion much better in either of the color scales. In fact, the more subtle the lesion (pixel value decreases < 10%), the better color aided in detecting the lesion.
Humans, like all primates, have superior discrimination of color vision (30). One need only look at a Monet painting in greyscale to see the importance of color is discerning complex visual information. While greyscale allows superior detail discrimination, it does not foster the detection of changes in intensity. This was more recently demonstrated in fluid-attenuated inversion recovery (FLAIR) anatomical MRI in stroke (31). A large retrospective sample of FLAIR images were displayed in greyscale and a color scale. The addition of color increased detection of stroke and inter-rater agreement by 23%. The positive predictive value similarly increased from 85.3 to 95.7% (31).
Ismael Mena et al. at Harbor UCLA did extensive studies with normal subjects with 133Xenon to determine quantitative data on the normal range of cerebral blood flow in humans (32). In a brief summary of an extensive body of work, mean cerebral blood flow was determined to be 70.3% of the maximum cerebral blood flow. The standard deviation (SD) was 8.35%. This work has been corroborated by several others and summarized by Devous et al. (33). Figure 2 illustrates the mean ± 1 and ± 2 SD set against various color scales and greyscale. One can quickly see that in greyscale neither an increase in 2 SD nor a decrease in 2 SD can be detected. In contrast, in the Heated Object color scale a decrease of 2 SD can be easily discerned. It is less clear that a decrease of 1 SD or an increase of 1 or 2 SD could be detected in Heated Object scale. In the Hot and Cold color scale, both increases and decreases of 2 SD could be easily detected, but changes of 1 SD might be more challenging. Lastly, the Ubiq 40 color scale, developed by Ismael Mena based on his quantitative data, and the DGP40, developed by one of the authors - DGP, has incremental color changes at approximately 2.7%. Changes in perfusion as small as 0.3 SD can be detected in either direction. The distinction between the DGP40 and the Ubiq40 is that the top 2% of the DGP40 is black, which allow easy identification of the most active or highly perfused part of the brain. An illustrative case is provided in Figure 2—a patient with signs of mild cognitive impairment and decreased performance on neurocognitive testing. The scan in greyscale is read as normal (Figure 2B). The tomograms in Ubiq40 show subtle decreases in perfusion in the frontal and parietal cortices (Figure 2C). However, when the scan is compared to a normal database of age-matched controls, statistical analysis reveals a pattern of hypoperfusion consistent with mild cognitive impairment of the frontal-temporal variant. This case illustrates the value of using color scale for SPECT scans wherein changes in intensity are more important than anatomical detail.
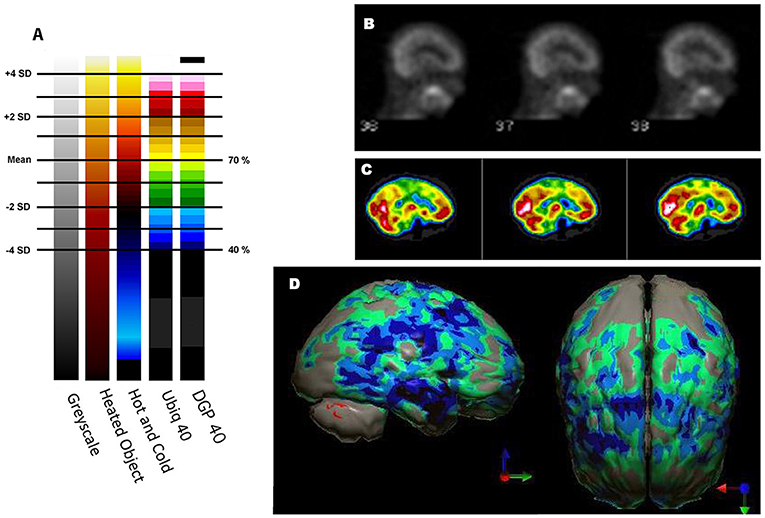
Figure 2. Humans, like all primates, have superior discrimination of color vision. While greyscale allows superior detail discrimination, it does not foster the detection of changes in intensity. Since functional neuroimaging is about changes in the intensity of the signal, it is important that the observer can readily detect small changes. (A) Various commonly used color scales and a greyscale are displayed. The mean cerebral perfusion in the human brain is 70.3% of the maximal flow with a standard deviation (SD) of 8.35%. The mean and ± 1, 2 SD, 3 SD, and 4 SD are indicated. A change of ± 2 SD is unlikely to be appreciated in greyscale but can be readily distinguished in Heated Object, Ubiq40, and DGP40 color scales. An increase of 2 SD can be distinguished in Hot and Cold color scale, but a decrease of 2 SD or less would not be discernable. A 1 SD increase or decrease would be difficult to discern in greyscale, Heated Object and Hot and Cold color scale, but are readily detected in Ubiq40 and DGP40. (B) A representative low quality 99mTc-HMPAO perfusion SPECT scan demonstrates poor technique with the inclusion of extracranial structures. The scan was read in greyscale and interpreted as a normal scan. (C) The same patient was rescanned with proper technique. Decreased perfusion in the posterior frontal and temporal cortices can be appreciated when viewed using the Ubiq40 color scale. (D) The patient's data is compared to a normative database (N = 68). A map of statistically significant differences can be generated using the Oasis software by Segami, Inc. Here, the color scale indicates gray for areas that do not differ significantly from the normative database. In contrast, areas of green, light blue, and dark blue represent areas of more than 2, 3, and 4 SD below the mean perfusion of the normative database, respectively. Statistically significant increases in perfusion are illustrated in the red color scale. Decreased perfusion in the bilateral temporal cortex and bilateral posterior frontal cortex, but with sparing of the anterior cingulate gyri, can be appreciated. The findings are consistent with mild cognitive impairment of the frontal-temporal variant and the patient showed consistent findings on neuropsychological assessment.
Throughout this article, early SPECT scans in greyscale will be contrasted with modern SPECT scans using color display and statistical comparison to a normal database. The wealth of information that becomes visible in color scale is self-evident. Nonetheless, despite extensive research supporting the value of color display, many radiologists and nuclear medicine physicians persist in using greyscale to read SPECT scans.
Assessment of the State of the Art—Spect in Brain Disorders
Herein, we will provide technical background on the studies cited by the TTASAAN report and then provide technical details from state-of-the-art studies in modern brain perfusion SPECT imaging. We will begin with and illustrate most extensively the state of the art in the evaluation of head trauma and traumatic brain injury (TBI), because this indication has been quite controversial and has raised the most strident criticisms.
Head Trauma
The definition of TBI has evolved since the publication of the TTASAAN report. At that time, “concussion” was considered a transient state. Now concussion is recognized as a form of TBI, despite an absence of a loss of consciousness (LOC). Because of this shift and the recognition that: 1) concussions can have persistent effects on the brain (34, 35), 2) repeated concussions can have cumulative damage (36), and 3) persistent pathological changes can occur following even a single concussive event (37, 38), we refer to the Centers for Disease Control (36) for a basic definition of the levels of severity of TBI.
The World Health Organization defines post-concussion syndrome (PCS) as “persistence of a constellation of physical, cognitive, emotional and sleep symptoms beyond the usual recovery period after a concussion” (39), including 3 or more of the following after head injury: headache, dizziness, fatigue, irritability, insomnia, reduced tolerance of stress, concentration difficulty, or memory difficulty.
The TTASAAN report (1) included seven early studies of TBI with a total of 253 subjects. All studies were conducted on single-head gamma cameras. Two studies utilized 125I-IMP and five studies utilized 99mTc-HMPAO with three of those studies conducted prior to the stabilization of HMPAO. All scans were assessed visually only. Jacobs et al. (40) will be discussed in detail below. Abdel-Dayem et al. (41) examined a series of 14 acute moderate-to-severe TBI cases with HMPAO SPECT scans performed within 72 h. Seven of the 14 cases did not survive. The number and extent of lesions observed by SPECT were compared to the number and extent of lesions seen by CT scan. Ducours et al. (42) examined 10 comatose TBI patients and 10 patients with TBI, but no LOC. All had a negative CT scan and a 125I-IMP perfusion SPECT scan. Patients without LOC had a normal 125I-IMP scan, while 9 out of 10 of the comatose patients had functional deficits on SPECT scan. Roper et al. (27) examined CT and HMPAO perfusion SPECT scans in 15 patients with mild, moderate or severe head injury (Figure 3B). SPECT revealed more focal lesions than CT. Gray et al. (28) examined 53 chronic (>6 months) TBI patients (20 mild, 33 severe TBI) compared to 14 normal controls using HMPAO SPECT (Figure 3C) and comparison to CT. Over 90% of the chronic severe TBI cases had areas of decreased perfusion on SPECT, but only 72% showed abnormalities on CT scan. Conversely, 100% of the patients with a normal SPECT scan had a normal CT scan. Ichise et al. (26) examined 29 chronic (> 6 months) TBI patients (15 mild, 14 severe TBI) and compared the HMPAO perfusion SPECT scans and neuropsychological testing results to those of 17 normal controls (Figure 3A). Trail Making A and B, Digit Symbol, and Wisconsin Card Sorting stood out as tests which strongly differentiated brain injured patients from controls (p < 0.001). Most lesions were in the frontal and temporal lobes and correlated with decreased neuropsychological scores on memory, attention, and executive function (26). Masdeu et al. (43) attempted to utilize negative controls (normal control) and positive controls (human immunodeficiency virus encephalopathy {HIV}) to examine mild TBI. Fourteen patients with mild TBI underwent CT scan and IMP or HMPAO perfusion SPECT scans within 48 h of head trauma. The results were compared to 15 normal controls and 12 patients with HIV encephalopathy. None of the normal controls were read as TBI; however, 40–50% of the TBI cases were read as HIV encephalopathy and 14–28% of the TBI cases were read as normal. The latter study highlights the jeopardy involved in visually interpreting SPECT scans, particularly in greyscale. As detailed above, the human eye is unable to separate 2 standard deviations in greyscale, because it is designed for color vision. Areas of hypoperfusion of <2 SD will be missed by visual read. Moreover, the absence of statistical comparison to a normative database or a matched set of normal also risks false negatives. This is strikingly demonstrated in Figures 3–5.
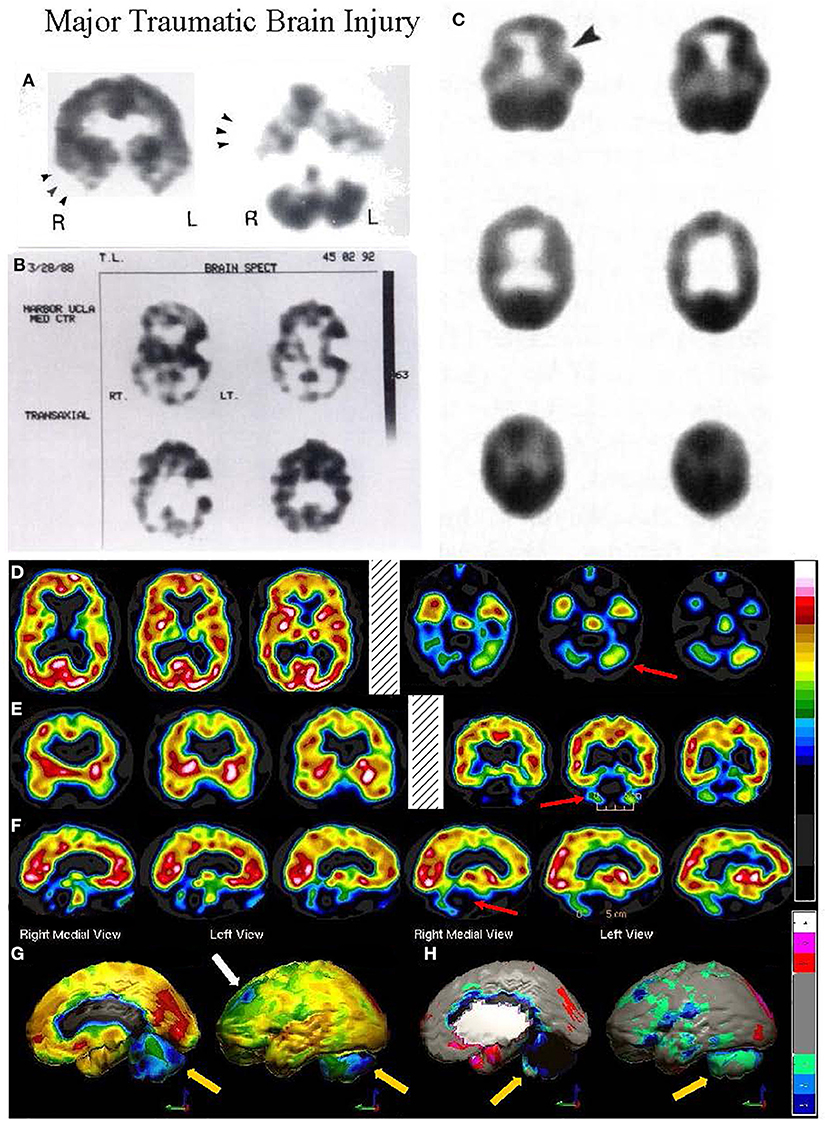
Figure 3. (A–C) Examples of SPECT scans cited in TTASAAN report. Anatomical details are lacking. (A) 40-year-old female with major head trauma showed decreased perfusion of the right temporal lobe, whilst CT and MRI scans were normal [The figure was originally published in JNM. © SNMMI (26)], (B) 45-year-old male thrown from a horse showed decreased bilateral occipital perfusion [The figure was originally published in JNM. © SNMMI (27)]; (C) 37-year-old female with major head trauma from a motor vehicle accident (MVA). Perfusion is decreased in the bilateral frontal and temporal lobes [The figure was originally published in JNM. © SNMMI (28)]. (D–F) A 19-year-old woman was involved in a head-on collision MVA as a passenger. She suffered severe trauma to the back of her head. A modern 99mmTc-HMPAO perfusion SPECT scan was performed with a dual-head camera. (D) Horizontal tomograms (non-sequential, break in sequence shown by cross-hatched bar) illustrate intact cortical function but marked hypoperfusion in the cerebellum bilaterally (red arrows). (E) Coronal tomograms (non-sequential). (F) Sagittal tomograms (sequential). (G) 3-D representation of SPECT scan data illustrating a small area of marked hypoperfusion in the left frontal cortex (white arrow) and profound hypoperfusion in the cerebellum (yellow arrows) which is more pronounced in the medial aspects. (H) The patient's data is compared to a normative database using Segami Inc. Oasis software. The color scale is the same as in Figure 2D. The injury to the left frontal cortex and lateral aspects of the frontal cortex can more clearly be visualized. Area of white in the right medial view is an area where there is no statistical comparison data.
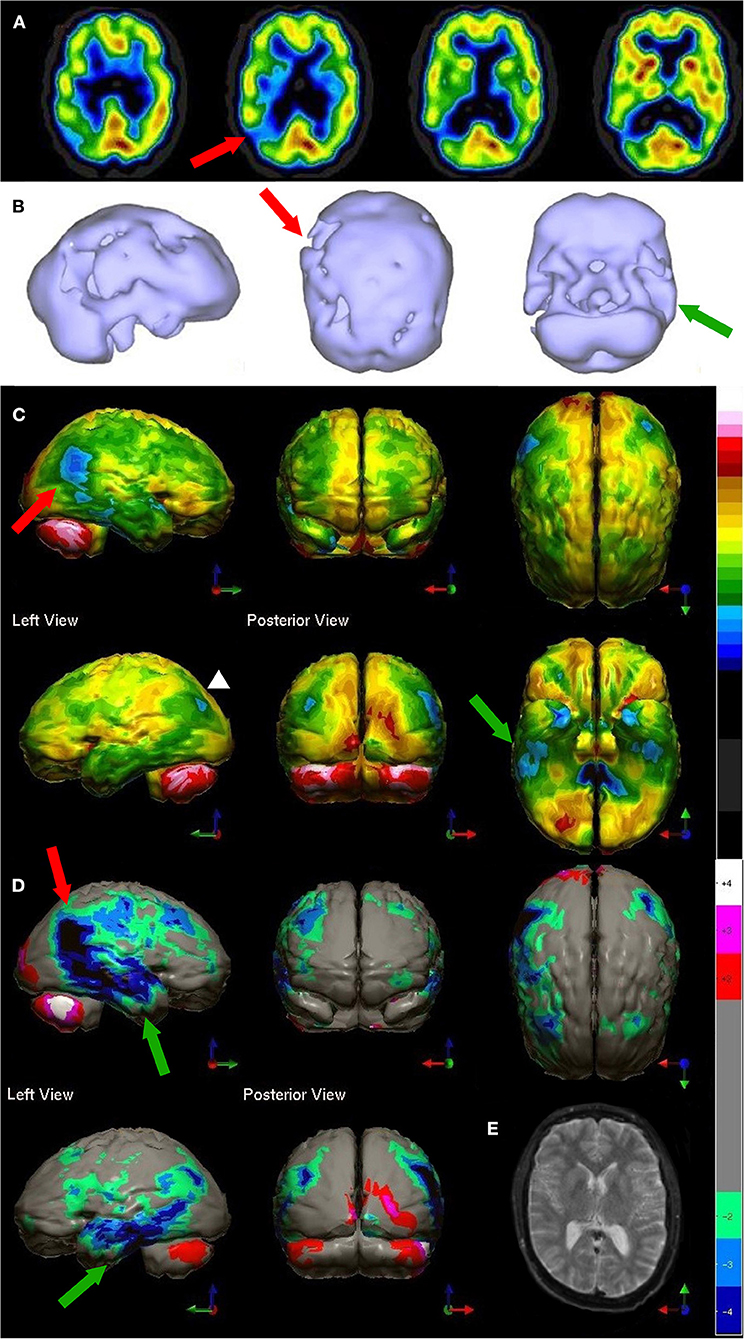
Figure 4. Tomographic and multiple 3-D representations of major TBI. A 58-yr-old female was struck on the right parietal region by a heavy object with loss of consciousness of approximately 2 hours. Perfusion SPECT scan was performed seven years after the injury with 99mmTc-HMPAO and a dual-head gamma camera. (A) 4mm horizontal sections illustrate decreased perfusion in the right parietal region (red arrow). The color scale is the same as Figure 1B. (B) SPECT data can be displayed in 3-D representations that facilitate the identification of large, diffuse, or subtle lesions. Here, data is presented as an isocontour display wherein cortical areas which fall below 60% of the maximal cerebral blood flow are displayed as a depression or hole. The large parietal defect is apparent on the right (red arrow), as well as bilateral temporal lobe hypoperfusion (green arrow). (C) Another 3-D representation utilizes the same color scale as (A). The right parietal defect appears as an area of blue and green (red arrow). A contra-coup injury can be visualized in this representation (white arrowhead). Temporal lobe hypoperfusion is again evident bilaterally (green arrow). (D) The patient's data is compared to a normative database using Segami Inc. Oasis software. The color scale is the same as in Figure 2D. The parietal lobe injury (red arrow) and the contra-coup injury are easily visualized, along with more diffuse penumbra injury and bilateral lateral temporal lobe hypoperfusion (green arrows). (E) Anatomical MRI completed at the time of the SPECT scan showed no abnormalities. Section at same level as far right horizontal tomogram in (A).
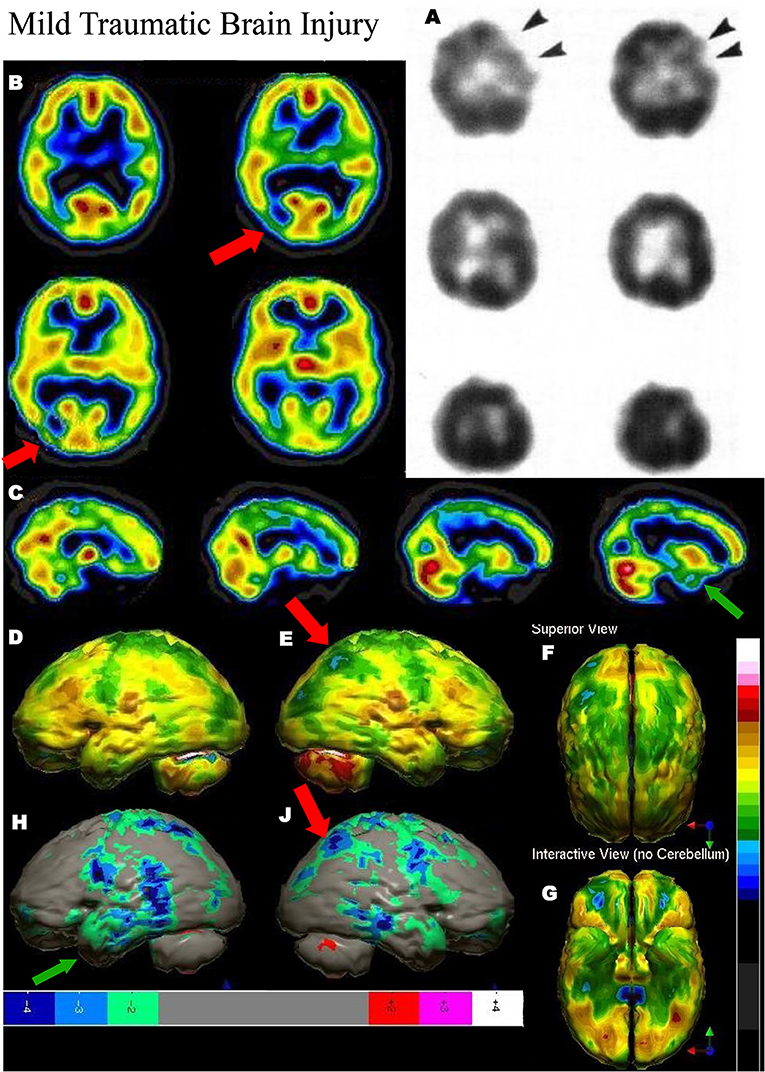
Figure 5. Mild TBI. (A) Example of SPECT scans cited in TTASAAN report. Anatomical details are lacking. 50-year-old male with minor head trauma after a motor vehicle accident showed decreased perfusion of the left inferior frontal and left anterior temporal lobe, whilst MRI scan was normal [The figure was originally published in JNM. © SNMMI (28)]. (B–G) A 2019 SPECT scan using a dual-headed gamma camera of an 18-year-old male who struck a tree while mountain biking and briefly lost consciousness. A modern 99mmTc-HMPAO perfusion SPECT scan was performed with a dual-head gamma camera. (B) Horizontal tomograms show detail of thalamus, anterior cingulate, caudate, and lentiform nuclei. A focal area of hypoperfusion can be seen in the right parietal cortex (red arrow). Color scale is the same as in Figure 1B. (C) Sagittal tomograms reveal decreased inferior frontal perfusion and decreased medial temporal perfusion bilaterally (green arrow). (D–G) 3-D representation of SPECT scan data showing left lateral (D), right lateral showing the area of hypoperfusion in the right parietal cortex (red arrow) (E), superior (F), and inferior views (G). (H–J) The patient's data is compared to a normative database using Segami Inc. Oasis software. The color scale is the same as in Figure 2D. Areas of relatively decreased perfusion are more evident, such as the area of hypoperfusion in the right parietal cortex (red arrow).
We (TAH, DGP), along with our colleagues, published a systematic review in 2014 which examined the entire extant literature on SPECT scans in the evaluation of TBI (44). The systematic review showed Level IIA evidence (at least one randomized controlled trial) for the utility of brain SPECT in TBI. The review identified 52 cross-sectional studies and 19 longitudinal studies including a total of 2,634 individuals over 30 years of literature. In addition, seven studies which were not included in the systematic review contain 223 subjects (45–51). Subsequently, two large retrospective studies comparing SPECT neuroimaging in TBI, post-traumatic stress disorder (PTSD), and normals containing over 21,399 subjects have been published (52, 53). Thus, besides the studies included in the TTASAAN report (1), there are an additional 69 studies containing 23,944 subjects on the utility of perfusion SPECT neuroimaging in the evaluation of TBI.
In addition, there have been numerous editorials and opinion pieces criticizing the use of SPECT to evaluate TBI (54–56). Central to the criticism is there is no gold-standard SPECT finding for TBI, particularly mild TBI. Another criticism is that many of the studies of TBI have a small number of subjects and lack a control group (54–56). A third criticism is SPECT may not provide additional benefit over CT or MRI (55, 56). A fourth criticism is that SPECT findings do not correlate with neuropsychological testing (54–56). A fifth criticism is that it has been unclear that SPECT scans can predict clinical outcome (54–56). Each of these criticisms will be addressed in turn.
Lack of Gold-Standard Finding
This position reflects an almost foolish belief that such a gold-standard could or should exist. By its very nature, TBI is highly variable. The mechanism of injury (impact, rotational, etc.), point of impact, presence or absence of contra coup injury, handedness, presence of prior injury, and other factors all contribute to the manifestation of TBI in each patient (34, 38, 57). The neuropsychological sequalae of injury will also depend upon what areas of the brain are affected, the inter-connectedness of affected areas, handedness, nutrition, toxic exposures, premorbid intelligence, and history of prior injury (58). This confound is not limited to SPECT, but also plagues other forms of neuroimaging, such as diffusion tensor imaging, when applied to the evaluation of TBI.
Small N Studies or Lack of a Control Group
By the very nature of TBI, it is not possible to have a randomized study of neuroimaging applied to TBI. How does one recruit a sample of subjects with normal baseline SPECT scans and then subject them randomly to a head injury followed by a repeat SPECT scan? As a result, there can never be true Class I evidence (well-designed, randomized, controlled clinical trial), as defined in the TTASAAN report (1), for the diagnostic and/or prognostic effectiveness of SPECT in the situation of TBI. This same limitation applies to studies of fMRI, CT, anatomical MRI, MEG, and diffusion tensor imaging. An early approach to this barrier was to randomly present cases of TBI with positive and negative controls to reading physicians (43). The study was technically flawed as described above.
Of the 54 published cross-sectional studies of perfusion SPECT neuroimaging in the evaluation of TBI, 26 had 20 subjects or less. Seven studies had 100 subjects or more (52, 53, 59–63). One study had over 7,600 subjects with TBI (53). Of the 19 longitudinal studies, nine had 20 subjects or less. Four longitudinal studies had 100 subjects or more (64–67). For example, Gowda et al. (66) prospectively performed CT and perfusion SPECT scans on 92 patients with acute TBI. Both scans were performed within 72 h of injury. Abnormal SPECT scans were found in 63% of cases—-half of these cases had normal CT scans. Two patients showed CT abnormalities without corresponding SPECT findings. A subarachnoid hemorrhage was the finding in both cases. The Newcastle-Ottawa Scale (NOS) was developed to assess the quality of non-randomized studies (68). The scale was applied to all the longitudinal SPECT studies by Raji et al. (44). The mean score for the 19 studies was 6 ± 1.4, which is considered to be high quality (NOS range 0–9).
Among the 71 studies included in the aforementioned systematic review (44), 15 included a control group. In addition, Stamatakis et al. examined SPECT scans and MRI from 51 subjects with TBI using statistical parametric mapping in comparison to 32 subjects in a control group (47). Atighechi et al. examined 21 subjects with TBI and anosmia compared to positive and negative control groups (50). Amen et al. conducted a retrospective comparison of TBI and PTSD (52). All patients underwent extensive psychiatric interview and completion of a battery of questionnaires. The diagnosis was made by Board-certified psychiatrists based on DSM-IV or V criteria. Baseline perfusion SPECT scans differentiated TBI from PTSD with a sensitivity of 92% and a specificity of 85% (52). Amen et al. replicated these findings in a separate retrospective evaluation of SPECT scans from distinct and closely matched groups of patients with TBI (N = 104), PTSD (N = 104), both TBI and PTSD (N = 73) and 116 healthy controls (53). All patients were diagnosed by a similar extensive battery of questionnaires and psychiatric interview. Controls were found to be free of psychiatric conditions, TBI, or substance abuse by extensive psychiatric interview and completion of a battery of questionnaires using DSM-IV or V criteria. The baseline perfusion SPECT scans were compared visually and by quantitative region of interest analysis. TBI could be distinguished from controls with a sensitivity of 100% and a specificity of 100% in both visual reads and quantitative analysis. In distinguishing TBI from PTSD, the sensitivity was 100% and the specificity was 100% for quantitative analysis and a sensitivity of 86% and specificity of 81% for visual reads. In addition, they conducted a larger comparison of 7,505 patients with TBI and other psychiatric comorbidities compared to 11,147 psychiatric patients without TBI who served as controls (53). With this more diverse group, sensitivity was 70% and specificity was 54% for both visual reads and quantitative analysis. The comparison of TBI and PTSD yielded somewhat higher accuracy with a sensitivity of 80% and a specificity of 60–62% (53).
In summary, there are numerous large-N cross-sectional, longitudinal and retrospective studies of the utility of SPECT in the evaluation of TBI. Indeed, thousands of subjects have been compared to hundreds of controls across 18 studies.
Does SPECT Provide Additional Information Over CT or Anatomical MRI?
Since perfusion in the gray matter is regulated by neuronal activity, as described above, perfusion SPECT provides a method of detecting neuronal dysfunction in the absence of anatomical change. Areas of the brain which are stunned, surviving, but not functioning (as in the ischemic, or otherwise functionally compromised) show no anatomical changes. However, the decreased function can lead to decreased perfusion. We (TAH) have demonstrated this in a case of chronic TBI wherein cerebral perfusion surrounding the injury and even in the contralateral hemisphere was decreased, despite normal appearance of the involved areas on MRI (69). These affected areas responded to treatment and showed improved perfusion upon repeat SPECT imaging. Acute TBI (within 72 h) represents a unique situation wherein perfusion can increase or decrease depending upon a number of factors, such as neuronal dysfunction and shutdown, inflammation, changes in blood-brain barrier permeability, excitotoxicity, and more. For example, Obrist et al. (70) performed serial quantitative perfusion SPECT (133Xenon) scans and found that rCBF was initially reduced (12 h) and then increased to hyperemic levels at 57 h after injury. Hyperemia was associated with increased intracranial pressure. The changes in perfusion may be due to loss of autoregulation (71) and/or transient disruption of the blood-brain barrier (72).
The collective literature (44) indicates that perfusion SPECT scans are superior to CT scans for detecting functional injury following head trauma in subacute and chronic TBI, and potentially acute TBI, as well. Over 96% of the studies which compared SPECT to CT found SPECT identified lesions which were not evident on CT. For example, Abdel-Dayem et al., evaluated 228 subjects with mild-to-moderate TBI and found abnormally low perfusion in the frontal, temporal, and parietal lobes (60). A follow up study by Abu-Judeh et al. (61) in the same population found that abnormalities that were identified on SPECT were often not seen or were underestimated in magnitude on CT scan in those receiving both SPECT and CT (61). Ichise et al. (26) found similar discordance with 79% of SPECT abnormalities lacking a matching abnormality of CT and concordant lesions were larger on perfusion SPECT scan than on CT scan (26). Emanuelson et al. (73) showed that SPECT lesions were concordant in severe TBI, but SPECT was more sensitive than CT in mild TBI. In another study, SPECT scans in the acute setting detected abnormalities in 75% of patients who had amnesia symptoms, while the CT scans were read as normal (74). Given that CT scans have become the cornerstone of evaluating concussion and TBI in the acute setting wherein they readily reveal hemorrhage and fractures, it becomes important to recognize that CT scans fail to show functional deficits seen on SPECT for which there may be no structural correlates. Thus, CT scans for head trauma in the emergency department may be negative, but do not rule out future functional deficits. To this point, all subjects in the longitudinal study by Jacobs had negative CT scans in the acute setting (40, 64); however, a positive baseline SPECT scan had high sensitivity and specificity for persistent neurological symptoms (see below).
Similarly, SPECT is more sensitive for TBI than anatomical MRI across multiple studies. In a series of 13 patients with moderate TBI, Shin et al. (75) found that MRI was negative in 50% of the cases, while the SPECT scans analyzed with statistical parametric analysis were positive for brain injury in 100% of cases. Abu-Judeh et al. examined 228 patients with mild to moderate TBI in a retrospective review (61). Both CT and MRI within 2 weeks of injury were negative, while SPECT scans revealed frontal lobe injury in 24% of cases and temporal lobe injury in 13% of the cases. Likewise, Stamatakis et al. (47) examined 62 patients with TBI using MRI and SPECT, which were performed within 2 weeks of injury. Using statistical parametric analysis, they found SPECT detected more lesions and more lesion volume than anatomical MRI. Ichise et al. (26) found SPECT scans more sensitive than MRI as well, with 79% of SPECT abnormalities lacking a concordant MRI lesion. Conversely, MRI detected white matter hyperintensities which did not show a matching lesion on SPECT (26). Kinuya et al. (48) found SPECT detected hypoperfusion in 94% of cases wherein MRI scans were normal; however, cases of subdural hematoma did not show abnormal SPECT findings. SPECT findings correlated strongly with symptoms, such as personality change or amnesia.
Does SPECT Correlate With Neuropsychological Findings?
The current trend in neuropsychological assessment is toward the profiling of functional performance to detect TBI. The field is still hampered using many tests that are antiquated, excessively long, or of dubious psychometric quality (76). A neuropsychological assessment can consist of a multitude of tests; there are over 100 separate neuropsychological assessment tests that are frequently utilized in TBI cases (77). Because no single neuropsychological test is particularly sensitive for TBI (78, 79), they are generally used in batteries. However, a lack of consensus exists about which tests are appropriate to include in a battery (79). Accordingly, the choice of tests to include is subjective. In addition, variances between how individual neuropsychologists administer the tests, interpret the results, apply failure criteria and decide whether to test for effort are additional subjective variables (80). Moreover, comorbid conditions, such as pain, anxiety, depression, sleep disturbance, medications, and alcohol use can interfere with cognitive performance obscuring the effects associated with mild or even much more significant brain injury (77, 80). Lastly, the validity of a neuropsychological assessment battery is based on the norms, decision rules, false positives, false negatives, hit rates, and the compounding of these variables when multiple tests are combined in a battery (77). Hence, neuropsychological testing is not considered diagnostic for TBI (81).
Brain SPECT imaging provides neuropsychologists an objective way to address these problems. SPECT has, in fact, been correlated with several individual neuropsychological assessment tests such as the Wisconsin Card Sort (82–85), the Stroop Colored Word Test (86, 87), the Tower of London Test (88, 89), the Clock Drawing test (90, 91), the Test of Verbal Fluency (92) and the Auditory Verbal Learning test (93). SPECT perfusion patterns have also been found to correlate with the predicted localization of neurological damage, based on neuropsychological battery testing, in a number of conditions including Lyme's disease (94), Sjorgren's syndrome (95), Klein-Levin syndrome (96), obsessive compulsive disorder (97), migraine headaches (98), paraneoplastic encephalitis (99), cerebral microvascular disease (100), chronic alcoholism (101), Alzheimer's disease and dementia (102), and neurological impairment following coronary artery bypass grafting (103).
Additionally, 18 out of 21 cross-sectional studies (81%) included in a systematic review showed correlation between abnormal SPECT findings and neuropsychological deficits (44). This suggests that abnormalities found with brain SPECT can correlate with and therefore can be predictive of functional outcomes and/or neuropsychological test performance. Davalos and Bennett (54) examined this question based on three studies (26, 104, 105); however, two of the studies lacked a control group and one included only four patients. Nevertheless, they concluded that this correlation warranted further study and that the confound of depression, possibly secondary to the TBI, must be carefully considered. We do not disagree with these conclusions, given the extensive literature presented above.
Do SPECT Scans Predict Clinical Outcome?
Neuroimaging for head trauma serves multiple purposes. Establishing the presence/absence of TBI is first and foremost. Predicting clinical outcome is an important additional benefit which may or may not be realistic. For example, diffusion tensor imaging has not shown clear predictive utility for clinical outcome (106). Nevertheless, critics are quick to hold SPECT in rebuke for failing to absolutely predict clinical outcome. For example, a critical opinion piece on the use of SPECT to evaluate mild TBI by Wortzel et al. (55), which was poorly referenced, cites an unnamed study in which an abnormal scan was predictive of persistent clinical symptoms in 59% of cases. Presumably, this unnamed study is Jacobs et al. (40) based on the reference in Davalos and Bennett (54), which Wortzel et al. (55) were discussing when describing this unnamed study. However, this reference is flawed on several levels. First, Jacobs et al. (40) included subjects with both mild and moderate TBI. Second, this study was examining SPECT findings in the subacute setting (within 1 week) as predictors of persisting symptoms. Recovery was an expected outcome for a significant proportion of subjects. Thirdly, Wortzel et al. (55) ignore the further longitudinal data from Jacobs et al. (64). Therefore, these results will be detailed here.
Jacobs et al. (40) published the first part of a two-part longitudinal study of the correlation between acute SPECT scan findings and persistent neuropsychological symptoms in 1994. It is one of a number of studies which have documented the positive predictive value (PPV) and negative predictive value (NPV) of SPECT in the prediction of lasting neuropsychological effects of TBI. It was included in the TTASAAN report and is also likely the study referenced by Wortzel et al. (55) above. Jacobs et al. (40) conducted a scrupulous study of 67 subjects with acute TBI (42 moderate TBI, 25 mild TBI) who were then followed over the subsequent year (64). Furthermore, Jacobs et al. added an additional 69 subjects with mild TBI to the longitudinal sample. All subjects had baseline perfusion SPECT scans and CT scans obtained within 4 weeks (83% within 1 week) of the head injury event. All subjects had baseline neuropsychological testing.
Subjects with a positive finding on SPECT had a repeat SPECT scan at 3 months, 6 months, and at 1 year, while all subjects underwent repeat neuropsychological testing at 3 months, 6 months, and 1 year (64). These studies captured three key concepts in the evolution of mild TBI. First, a substantial proportion of patients with mild TBI recover over the course of the year, regardless of whether they have positive SPECT findings at baseline. The second, not every patient with mild TBI will have a positive SPECT scan. The third, the sensitivity and specificity of baseline SPECT for predicting persistent neuropsychological symptoms and findings can be calculated. In addition, the PPV and the NPV of a baseline SPECT scan in acute TBI was determined (Table 1).
Based on this large sample of 136 subjects, a negative baseline SPECT scan was highly predictive of normal neuropsychological testing in the future. In other words, a negative SPECT scan shortly after initial injury predicts the absence of long-term functional deficits. This predictive value cannot be matched by other imaging modalities such as conventional CT or MRI. The positive predictive value of baseline SPECT scans was also quite high. An abnormal baseline SPECT scan that remained positive at 12 months predicted persistent neuropsychological deficits with a sensitivity of 100% and a specificity of 85% (64). This gives a strong argument for serial SPECT scans in cases with both positive baseline scan and neuropsychological symptoms. Similarly, a second prospective study by an independent group (107) found an abnormal baseline SPECT correlated strongly with abnormal neuropsychological testing in patients participating in a cognitive rehabilitation program.
Lastly, the lack of a randomized, placebo-controlled clinical trial of SPECT in the diagnosis of TBI is a deficit cited by both critics (54–56) and proponents (54, 108–110). The improbability of a gold-standard and randomizing patients to experience TBI make this critically needed study impossible. However, an additional, more nefarious, barrier has prevented such a study from occurring. One of us (TH) collaborated with Drs. Davalos and Bennett to implement a study to evaluate mild TBI with perfusion SPECT which addressed the concerns they raised in their review. Essentially, this study would have replicated Jacobs et al.' work (64) with better SPECT imaging, larger sample size, more extensive neuropsychological testing, and comparison to a carefully vetted normative dataset. Unfortunately, repeated funding attempts failed due to wholesale rejection of SPECT neuroimaging by grant reviewers. Comments such as, “The use of SPECT, a technique with poor spatial resolution, poses a concern.” and “use outdated techniques” peppered the peer review panel summary statements. Again, it seems odd that perfusion SPECT imaging is held to an unrealistic standard that fMRI, diffusion tensor imaging, FDG-PET, amyloid PET and other forms of neuroimaging do not meet. For example, numerous diffusion tensor imaging studies of mild TBI lack the rigorous criteria established by Davalos and Bennett (54) but these studies were still funded and published after these criteria were set (106, 111–118). For example, several diffusion tensor imaging studies lack control groups (115, 118), several have small sample sizes (111–115), several examined limited neuropsychological testing (112, 113, 115–119), and all lacked randomization.
Experts in the field are calling for greater collaboration between neurologists and nuclear medicine physicians to conduct the needed studies required to convince Neurology of the value of SPECT neuroimaging in the assessment of TBI (67, 108–110). Critics continue to claim that SPECT is not useful in the evaluation of TBI. Conversely, according to the criteria set forth in the TTASAAN report (1), the current literature supports the use of perfusion SPECT neuroimaging to evaluate TBI as a Type A Recommendation (strong positive recommendation) based on Class II evidence derived from multiple clinical studies with large N and control comparison groups (40, 47, 52, 53, 63, 64, 86) as presented above.
Stroke
Stroke remains a leading cause of death and disability throughout the world. In 1996, the rate of new stroke cases was ~269 per 100,000 (120) and the prevalence was 988 per 100,000. In 2017, the rate of new stroke cases declined 11% to 150 per 100,000 (121), while the prevalence increased by 37% to 1363.5 per 100,000 (121). As a result, ~104.2 million people worldwide have experienced a stroke (122). Neuroimaging has a pivotal role in the assessment and clinical management of stroke.
The TTASAAN report (1) included 12 research studies on stroke, in which 10 were performed using single-head gamma cameras (25, 123–127). Since the publication of the TTASAAN report, significant advancements have occurred in imaging techniques to assess vascular anatomy and integrity. Magnetic resonance angiography (MRA), computed tomography angiography (CTA) and diffusion weight MR (DWI) have proven effective, safe, and rapid in modern hospital settings (128). However, much of the world does not enjoy the technical riches of hospitals in the United States. Thus, many of the MRI and CT techniques employed in stroke assessment are not available elsewhere. Perfusion SPECT remains a valuable tool for assessing acute strokes prior to administering intravenous tissue plasminogen activator (IV tPA) (129, 130), assessing subacute strokes for viability and size of the penumbra (131, 132), and assessing persistent stroke-related symptoms (133). Perfusion SPECT may also contribute to stroke risk assessment (134, 135).
Nevertheless, the first step in assessing a patient with symptoms of stroke or transient ischaemic attack (TIA) is a non-contrast CT scan. CT remains a rapid, safe, and effective means of determining if a stroke is hemorrhagic or not. The introduction of IV tPA as a treatment for dissolving and clearing clots, as well as clot retrieval techniques, has increased the need for rapid determination that a stroke is not hemorrhagic. Candidates for IV tPA need to be treated within 4.5 h of stroke onset. Thus, MR techniques, which are more rapid, have largely replaced perfusion SPECT scans in the assessment of acute stroke/TIA patients.
CT and diffusion/perfusion-weighted MRI have largely replaced perfusion SPECT in the assessment of subacute or chronic cerebrovascular disease. However, in certain conditions in which the variability of clinical presentation can be high (e.g., transient ischemic attacks, Moyamoya disease), SPECT may still offer value as part of the overall assessment plan. Perfusion SPECT neuroimaging is useful in delineating the extent of ischemic infarction and correlates well with severity of neurologic deficits and clinical outcomes. It may be useful in demonstrating the ischaemic penumbra at the margins of an ischaemic infarct, which may be salvaged with neuro-interventional procedures (132). This is due to the fact that perfusion SPECT imaging does not simply demonstrate the presence or absence of vascular occlusion. Rather, localized cerebral blood flow is regulated by the brain region itself. As the activity of a particular brain region increases, so does its need for oxygen and glucose. By a signaling mechanism involving neurons, glial cells, and the arterioles, the brain region calls for increase localized blood flow to meet its needs. Thus, perfusion SPECT neuroimaging shows active brain tissue, inactive brain tissue, and compromised brain tissue. Similarly, perfusion SPECT can be useful in assessing response to treatment or interventions (136, 137). A smaller volume of penumbra (138) and the absence of crossed cerebellar diaschisis (139) can be predictive of better clinical response. The technical improvements in gamma cameras and in post-processing software have markedly improved the resolution, anatomical detail, and information density of perfusion SPECT scans (Figure 6). 3-dimensional reconstruction allows more complete understanding of the stroke and penumbra volume. Statistical parametric analysis, particularly with comparison to a normative database, reveal details which might otherwise not be discernible (Figures 6G–L).
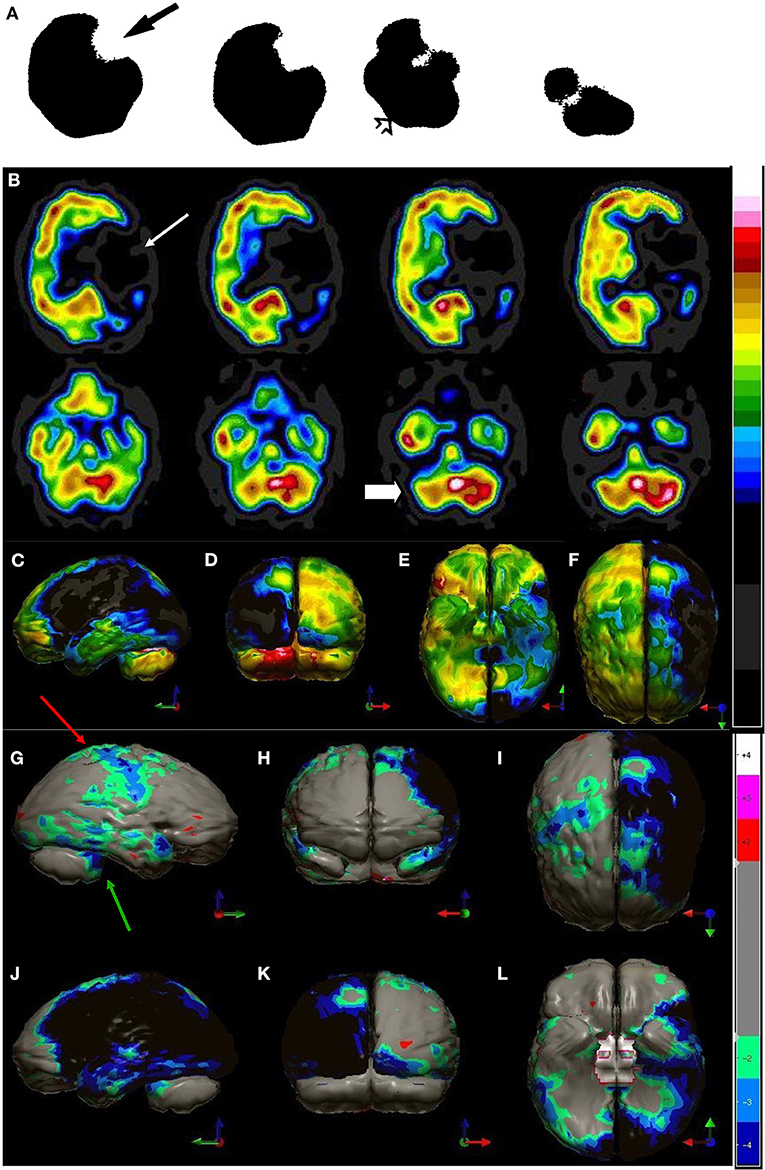
Figure 6. (A) Example of SPECT scan studies cited in TTASAAN report. Anatomical details are lacking. A 99mTc-ECD perfusion SPECT scan from 1989 illustrating a left middle cerebral artery stroke (black arrow) with crossed cerebellar diaschisis (open arrow). This scan was performed on a single-head gamma camera [The figure was originally published in JNM. © SNMMI (25)]. (B) Modern 99mTc-HMPAO SPECT scan using a dual-head gamma camera illustrating a left middle and posterior cerebral artery stroke (white arrow) involving the left posterior frontal, temporal, parietal, and occipital cortices. Color scale is the same as in Figure 1B. Crossed cerebellar diaschisis is apparent (white block arrow), as well as involvement of the left thalamus and left basal ganglia. (C–F) left lateral, posterior, inferior (cerebellum removed) and superior 3-dimensional views, respectively. (G–L) Right lateral, frontal, superior, left lateral, posterior, and inferior (cerebellum removed) views of scan compared to normative database. Color scale is the same as in Figure 2D. Involvement of the contralateral cortex (red arrow) and the crossed cerebellar diaschisis (green arrow) are evident.
Cerebrovascular reserve capacity (CVRC) is an important parameter which guides treatment decisions in chronic cerebrovascular diseases. The cerebral circulation is complex with multiple arterial inputs to the Circle of Willis, with altered hemodynamics resulting from gradual occlusion of one of more vessels over time. In additional, there is highly responsive intracerebral autoregulation to maintain blood flow, particularly when cerebral perfusion pressure is reduced. Determining reserve capacity can be critical in assessing a patient for carotid endarterectomy or carotid stenting. Perfusion SPECT neuroimaging allows a global assessment of the integrated effects of hemodynamic factors, as it measures cerebral cortical and subcortical gray matter blood flow (140). Furthermore, dynamic assessment using intravenous acetazolamide or inhaled CO2 followed by perfusion SPECT provides an accurate measure of cerebrovascular reserve. Both acetazolamide and inhaled CO2 cause vasodilation of cerebral microvasculature (141). By challenging the vascular system with additional flow demands, these techniques reveal if the smaller arteries fed by the carotid arteries can support the flow demand (141). This is illustrated in Figure 7. This technique can be useful in decision making for patients with Moyamoya disease, as well.
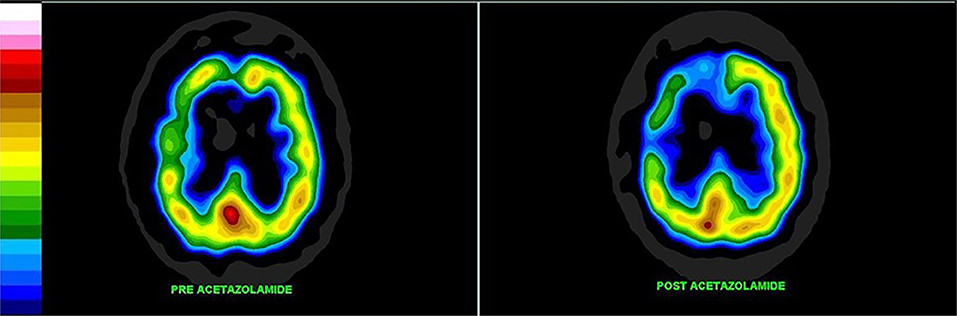
Figure 7. Patient with high grade carotid stenosis and multiple co-morbidities. The left hemisphere (on the right of the image) is able to augment its blood flow with acetazolamide, but there is restriction of flow to the right side leading to relative deterioration in right perfusion after acetazolamide administration, compared to the left. Color scale is the same as Figure 1B. Courtesy of J. Cardaci, MBBS, FAANMS, FRACP - Diagnostic Nuclear Imaging, Perth, West Australia; University of Notre Dame, Fremantle, Australia.
Epilepsy
The incidence of seizure disorders in the United States is 39 per 100,000, representing about 3.4 million cases (142). Approximately, one-third of all cases prove intractable or treatment-resistant—virtually unchanged from the time of the TTASAAN report, despite 25 years of new anticonvulsant medications. Surgical or laser ablation of the seizure focus/foci remains the most effective treatment in these cases. Ictal and inter-ictal perfusion SPECT scans remain an integral part of the pre-surgical evaluation of such cases (143–145).
The TTASAAN report (1) included 17 papers and reviews on the use of perfusion SPECT scans in the evaluation of epilepsy with a total of 182 patients (146–151). Based on this very preliminary data, the conclusion was that perfusion SPECT performed during the seizure event (ictal) could localize the seizure focus in 71–93% of cases with a positive predictive value of 95% (1).
In the last decade alone (2012–2021), there have been over 181 research articles and reviews containing a total of 8,516 patients on the topic of the utility of perfusion SPECT scans in the evaluation of epilepsy. The predominate topic was pre-surgical planning; however, diagnosis of pseudoseizures and seizures due to other medical conditions were also topics. In addition, over 85 cases studies of 1–3 patients each covered the utility of perfusion SPECT imaging in the differential diagnosis of a wide variety of medical causes of seizures, including TBI (152), tuberous sclerosis (153), vascular disease (154), neoplasms (155), systemic lupus (156), rare autoimmune disorders [e.g., anti-NMDA receptor autoimmune encephalitis (157, 158), hyperglycemia (159), and Lewy Body dementia (160)].
Two significant advancements in perfusion SPECT imaging, beyond the stabilization of HMPAO and the improvements in both gamma camera technology and post-processing software, have impacted the utility of SPECT imaging in the localization of seizure foci. The first is subtraction ictal SPECT co-registered to MRI (SISCOM). In essence, the interictal data is subtracted from the ictal data to reveal a focus or foci of increased perfusion during the ictus. The focus/foci are then mapped onto the patient's anatomical MRI (161–164). A retrospective study of 90 patients undergoing surgical resection of seizure foci between 1995 and 2013 revealed SISCOM predicted patients being seizure-free up to 5 years after ablative surgery (69.2%), comparable to FDG-PET localization (162). Smaller studies have found similar positive predictive value [76.9% - (165)]. The ability of SISCOM to accurately localize a seizure focus ranged from 73 to 85% (mean 77.3%) across multiple studies (164–172). A meta-analysis of 11 studies found SISCOM accurately localized seizure focus in 85.9% of cases and was concordant with EEG data in 65.3% of cases (166). SISCOM had an odds ratio of the patient being seizure-free post-operatively of 1.90 in non-concordant cases and of 6.23 in concordant cases (166). Recently, the diagnostic accuracy and predictive value of ictal perfusion SPECT was compared to magnetoencephalography (MEG) in a group of 158 surgical candidates. The accuracy of ictal SPECT was 78.57% in localizing the seizure focus, while the accuracy of MEG was 74.26% (1, 171). The odds ratio for a patient being seizure-free after surgery was 5.0 and 2.43 for ictal SPECT and MEG, respectively (171). Figure 8 illustrates the advances in perfusion SPECT imaging, including the SISCOM technique for localizing seizure foci.
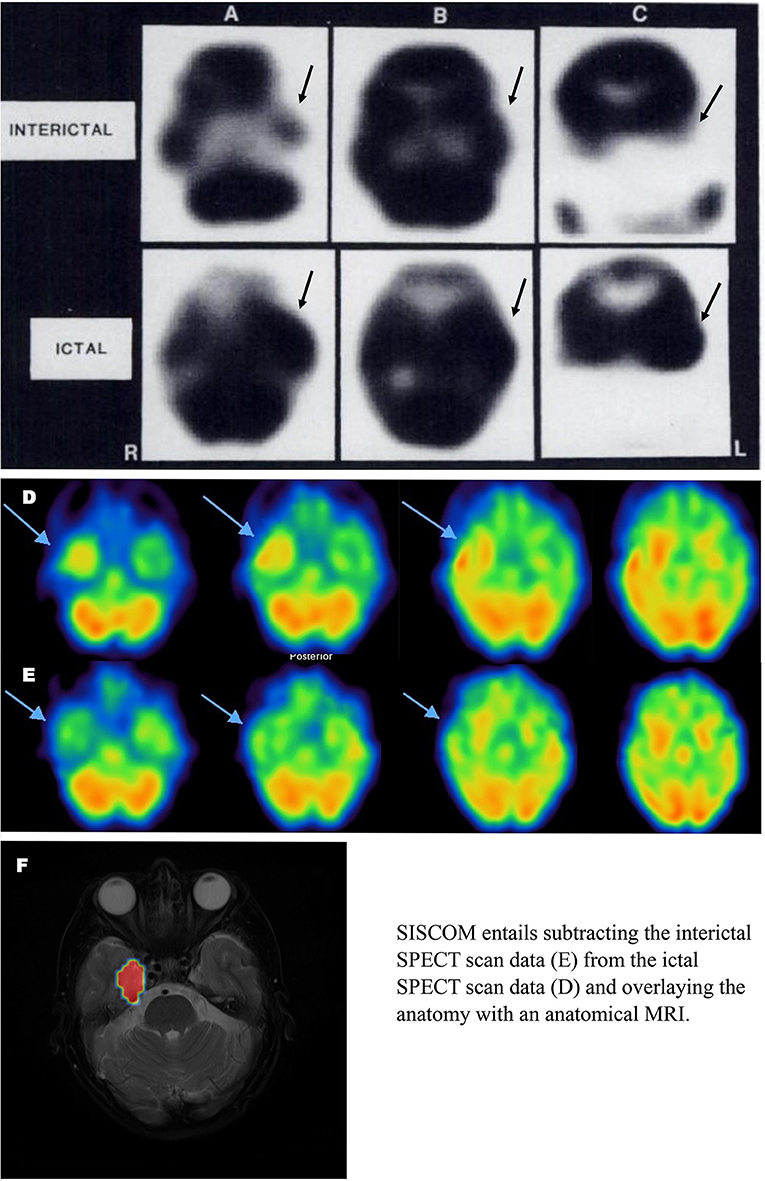
Figure 8. Ictal and Interictal SPECT (A–C) Example of SPECT scan studies cited in TTASAAN report. Perfusion tracer is iodinated N,N,N'-trimethyl-N'-(2-hydroxy-3– methyl-5-iodobenzyl)-1,3-propane-diamine (HIPDM). Anatomical details are lacking [The figure was originally published in JNM. © SNMMI (149)]. (D,E) Modern 2021 HMPAO SPECT perfusion scans for seizure localization. (D) Ictal scan. (E) Interictal scan. (F) SISCOM image wherein interictal scan data is subtracted from ictal scan data and the resulting data is overlayed on an anatomical MRI. Images courtesy of– Leonard Numerow MD FRCP(C), Radiology and Nuclear Medicine, Cumming School of Medicine, University of Calgary, Calgary, Alberta, Canada.
The second advancement has been the use of automated injectors to reduce the lag time between the onset of seizure activity and the injection of the tracer (173, 174). Automated injectors can reduce lag time from 60 s with hand administration to 18.5 s using an automated method (175). Since seizure activity is initially limited to the focus/foci, but then spreads to adjacent or even contralateral brain areas, capturing the perfusion pattern early increases the accuracy of the localization by perfusion SPECT (176, 177). For example, using automated injectors increased localization of seizure foci from 62.9 to 81.8% (175).
Perfusion SPECT also has proven useful in cases of non-convulsive status epilepticus (NSE) among medically complicated patients (178). NSE can prove to be a challenging diagnosis and is more likely in critically ill patients with multiple overlapping diagnoses. In a group of 55 patients, initial EEG had a sensitivity of 61.1% and a specificity of 89%. In contrast, a perfusion SPECT had a sensitivity of 80.5% and a specificity of 89.5% in diagnosing NSE (178).
Dementia and Alzheimer's Disease
The TTASAAN report (1) included 25 research articles and reviews on dementia (179–185). All of the studies were conducted using single-head gamma cameras, except one which used an annular crystal. Since the TTASAAN publication, over 600 research studies have been published on the subject of perfusion SPECT in the evaluation of dementia.
Currently, the most reliable neuroimaging findings for Alzheimer's disease (AD) are the observed decreased metabolic activity and associated decreased perfusion of the posterior parietal and temporal lobes bilaterally and the posterior cingulate gyri bilaterally with relative sparing of the basal ganglia, thalamus, and primary sensory-motor cortex (186–191). The early SPECT perfusion studies of AD relied on single-headed or low-resolution gamma cameras (192–194). Nevertheless, a meta-analysis of these early studies concluded perfusion SPECT has a sensitivity of 74% and a specificity of 81% for the differentiation of AD from elderly controls (195).
Since 2009, there have been three meta-analyses (196–198), a comprehensive review with meta-analysis (191), and a systematic review (199) addressing the clinical utility of perfusion SPECT neuroimaging in AD. Yuan et al. (196) found SPECT had a sensitivity of 84% and a specificity of 70%, while Bloudek et al. (197), performing a meta-analysis on selected research from 1990 to 2010, concluded that perfusion SPECT had a sensitivity of 79% and a specificity of 84%. Subsequently, Frisoni et al. examined 32 studies and found SPECT had a sensitivity of 76% and a specificity of 84% (198). In contrast, the sensitivity of FDG-PET ranged from 76 to 89% and the specificity ranged from 74 to 85% (191). The meta-analyses described above all co-mingled SPECT studies using single-headed gamma cameras with studies using multi-headed gamma cameras. Moreover, the summary sensitivity/specificity figures result from combined comparisons of AD cases vs. mild cognitive impairment (MCI), fronto-temporal dementia (FTD), vascular dementia (VaD), dementia with Lewy Bodies (DLB), and healthy elderly controls. As a result, these meta-analyses are not consistent with the conclusions of others (200, 201). For example, Bonte et al. (201) found that based on correlation to autopsy data, decreased posterior cingulate perfusion alone has a positive predictive value of 93% and a negative predictive value of 81%.
A more recent meta-analysis was conducted which grouped data based on camera type and comparator group (FTD, VaD, DLB, MCI, healthy elderly control) (191). When differentiating AD from healthy elderly controls, studies using relatively low-resolution, single-headed gamma cameras yielded an overall sensitivity of 84% and an overall specificity of 83% (187, 200, 202–211). Studies utilizing multi-headed gamma cameras and often quantitative analysis (91, 186, 212–231) yielded a modest, but clinically significant, increase in overall sensitivity to 89% and overall specificity to 89%. Figure 9 illustrates the advancements in the images produced by perfusion SPECT imaging including statistical parametric analysis to an age-matched normative database as depicted for AD.
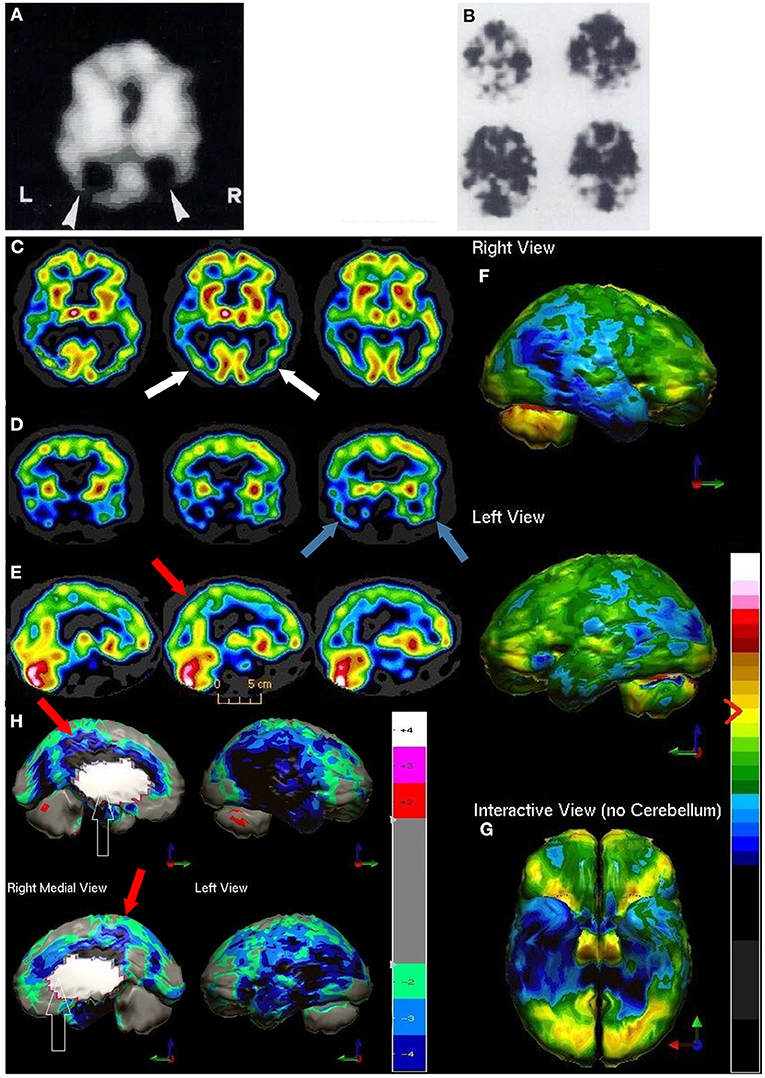
Figure 9. (A,B) Examples of SPECT scan studies cited in TTASAAN report. Anatomical details are lacking. (A) An 99mTc-HMPAO perfusion SPECT performed in 1988 using a single head gamma camera illustrated a 75-year-old male with severe dementia and showed decreased perfusion of the bilateral parietal cortices [The figure was originally published in JNM. © SNMMI (202)]. (B) Example of 133Xenon perfusion SPECT scan obtained with a ring-type tomogram circa 1988 [The figure was originally published in JNM. © SNMMI (180)]. C-H) Modern 99mTc-HMPAO SPECT scan performed on a dual-head camera illustrating Alzheimer's disease. (C–E) Horizontal, coronal, and sagittal tomograms, respectively, show decreased perfusion of the bilateral parietal cortices (white arrows), bilateral temporal cortices (blue arrows) and the posterior cingulate gyri (red arrow). Color scale is the same as in Figure 1B. (F,G) 3-D representation of SPECT scan data showing right lateral, left lateral (F), and inferior views (G). In the 3-D representations the asymmetry is much better seen with greater involvement of the right parietal and temporal lobes. (H) The patient's data is compared to a normative database using Segami Inc. Oasis software. The color scale is the same as in Figure 2D. Areas of relatively decreased perfusion are much more evident. The hypoperfusion of the posterior cingulate gyri (red arrows) is much better demonstrated.
Longitudinal clinical studies have corroborated that perfusion SPECT scans can predict the advancement of SPECT-identified AD to autopsy-proven AD, as well as the progression of MCI to AD. Jobst et al. (210) followed 200 patients with dementia and 119 controls over 7 years. Seventy patients were autopsied, and baseline clinical evaluation alone yielded a sensitivity of 93% and a specificity of 46% in predicting histopathology consistent with AD, while baseline SPECT scans combined with clinical diagnosis did not change the sensitivity, but increased the specificity to 84%. Hanyu et al. (200) examined a group of 219 patients and were able to distinguish 56 cases that would progress to AD based on decreased perfusion of the temporal and parietal lobes (sensitivity 82%, specificity 89%). Matsuda et al. (232) utilized Z-score analysis to demonstrate that decreased posterior cingulate/precuneus perfusion could distinguish 40 patients with MCI suspected of being the AD-type from 40 controls with an accuracy of 86%. Taken together, studies of perfusion SPECT in the diagnosis of AD with comparison to a longitudinal clinical course and/or histopathology demonstrate sensitivity in the range of 82–96% and specificity in the range of 83–89% (191).
SPECT neuroimaging can be extremely helpful in the evaluation of dementia of a vascular origin (VaD). VaD can show widely varying regional blood flow patterns, reflecting its variable vascular source (233). As such, there is not a single characteristic pattern of perfusion or metabolic activity that identifies VaD dementia (191, 233, 234). However, certain features are highly suggestive of vascular dementia (235), such as hypoperfusion of the anterior cingulate gyrus (which mitigates relatively against AD) or reduced perfusion of the pulvinar of the thalamus as seen in subcortical VaD (191). Rather, it is often the varied, bilaterally disparate, and irregular pattern that aids in the diagnosis of vascular dementia. A meta-analysis of perfusion SPECT studies utilizing single-headed gamma cameras with only visual interpretation to distinguish AD from VaD found sensitivity to be 70% and specificity to be 76.6% (191, 236–240). In contrast, the use of multi-headed gamma cameras and quantitative analysis improved sensitivity to 90%, while specificity remained slightly better than 76% (191, 218, 230, 241, 242). Neuroimaging data suggest that different types of VaD can be distinguished by SPECT; indeed, some authors feel that SPECT is more helpful in the diagnosis of different forms of vascular disease than PET (191, 233).
Frontal temporal dementia (FTD) can be characterized on functional brain imaging by decreased function and associated hypo- perfusion in the frontal lobes, caudate nuclei, and anterior temporal lobes (243–247). Hypoperfusion also can be found in the anterior cingulate gyrus. In FTD, perfusion is generally spared in the posterior cingulate gyrus/precuneus (91, 201, 248, 249). For example, Bonte et al. (186) followed 54 patients to autopsy, and a SPECT scan up to 1 year prior to death predicted the histopathology with a 96% sensitivity and an 84% specificity. The meta-analysis of all studies utilizing single-headed gamma cameras yielded a sensitivity of 71.5% and a specificity of 78.2% in the differentiation of AD from FTD (191, 244, 250–252). In contrast, a meta-analysis of studies utilizing multi-headed gamma cameras and quantitative analysis found the sensitivity was 96% and the specificity was 80% in differentiating AD from FTD (91, 191, 246, 249, 253). For example, in a careful study with quantitative analysis of SPECT data from a multi-headed gamma camera compared to autopsy findings, the temporal-parietal and posterior cingulate gyrus hypoperfusion had high sensitivity and specificity for distinguishing AD from FTD (91).
Similarly, the differentiation of AD from Lewy Body dementia (DLB) by perfusion SPECT imaging has benefited from improved hardware and analysis techniques. Shimizu et al. (254) examined the differentiation of DLB and AD using statistical parametric analysis to evaluate cortical, as well as deep nuclei, perfusion. They found that while hypoperfusion in the occipital lobe was predictive of DLB, the additional findings of increased perfusion in the thalamus and bilateral striatum strengthened the accuracy of the diagnosis of DLB. Sato et al. similarly found increased perfusion of the thalamus and striatum differentiated DLB from AD (255). Goto et al. (256) also found striatal parameters useful in differentiating early mild DLB from early mild AD. They found striatal volume to be reduced in early DLB, along with reduced occipital perfusion. The sensitivity of these parameters was 89%, while the specificity was 84%.
The accurate and early identification of Mild Cognitive Impairment (MCI) with perfusion SPECT has been examined extensively in several longitudinal studies. A total of 495 patients with MCI have been followed over 2–3 years [one study to 5 years (257)] in 10 longitudinal studies that included a baseline SPECT scan. All the studies used multi-headed gamma cameras and quantitative analysis (191, 257–266). Overall, 43% of MCI patients showing decreased perfusion in the: 1) posterior cingulate gyri, 2) posterior parietal cortices, and/or 3) temporal cortices converted to AD with an average conversion rate of 20% per annum. The remaining patients showed no change in cognition (stable MCI). Thus, the key SPECT findings found in AD were already present 2–3 years before the onset of the clinical symptoms of AD (191, 228, 229, 266). With quantitative analysis, the predictive value of perfusion SPECT in MCI can be increased considerably. Quantitative analysis yielded a sensitivity of 97%, a specificity of 100%, and an accuracy of 99% in one study (228). SPECT outperforms clinical assessment for MCI, which is generally 49–63% sensitive and 89–94% specific (267). Using older, single-headed gamma cameras and visual inspection, several studies found that SPECT can differentiate MCI with a sensitivity of ~84% and a specificity of 83% (191). In contrast, multi-headed gamma cameras and quantitative analysis yields sensitivity and specificity exceeding 97% depending on the study (191, 228, 268).
Given that gamma cameras are much more readily available than PET scanners in much of the world and perfusion SPECT scans can be performed at a much lower cost than FDG-PET scans (191, 269–271), it is important to assess the value-added benefit of choosing PET or SPECT imaging (See Table 2). A relatively small number of studies have examined the distinction between SPECT and PET imaging in the evaluation of AD in the same patients. For example, a pivotal study by Herholz et al. (269) compared FDG-PET and perfusion SPECT in the same 26 patients with probable AD. In the key areas of the temporal, parietal, and posterior cingulate cortices, FDG-PET and SPECT yielded corresponding findings (r = 0.90) using statistical parametric analysis. Silverman (188) summarized the comparison of PET and SPECT well, noting that the sensitivity and specificity of PET and SPECT have considerable overlap. Similarly, a systematic review of studies comparing perfusion SPECT and FDG_PET in the diagnosis of AD and other dementias (199) found the two techniques quite comparable in terms of accuracy of diagnosis. The application of quantitative analysis greatly enhances both PET and SPECT. With appropriate quantitative analysis, both neuroimaging modalities can achieve high sensitivity and specificity in the diagnostic evaluation of AD and MCI, and in the differentiation of AD from LBD. Specifically, FDG-PET was found to predict the conversion from MCI to AD with a sensitivity of 70–90% and a specificity of 82.4–90% depending on the study (191, 196, 272, 273), while perfusion SPECT scans predict the conversion from MCI to AD with a sensitivity ranging from 89 to 97% and a specificity of 89–100% depending on the study (191, 196, 221, 225, 263, 274). Amyloid PET scans also should be included in this discussion given the growing number of amyloid tracers available. PET scans using amyloid-specific markers, such as florbetaben or florbetapir, yield very high sensitivity. A widely held belief is that a positive amyloid scan is 100% predictive of a progression to AD. However, specificity varies with age. A caveat for amyloid markers, regardless of the marker, is non-specific binding does occur and increases with age. Such non-specific binding occurs in ~20% of 60-year-old controls, but this increases with age to ~40% of 80-year-olds (275, 276). A recent Cochrane Review (277) found that amyloid markers predicted the progression from MCI to AD over a 4-year period with a sensitivity of 67% and a specificity of 71%. Shorter follow-up time courses yielded different metrics with a sensitivity of 89% and a specificity of 58% at 2 years (277).
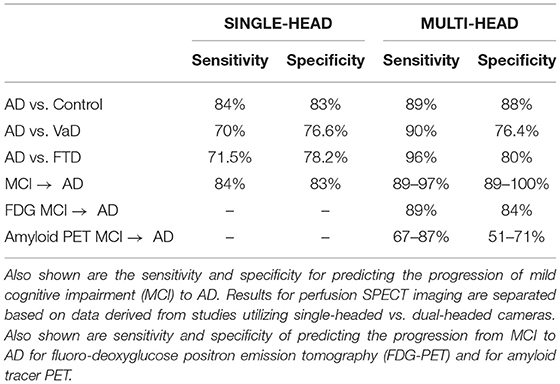
Table 2. Sensitivity and specificity of perfusion SPECT neuroimaging for differentiating Alzheimer's disease (AD) from controls, vascular dementia (VaD), and fronto-temporal dementia (FTD).
Neuropsychiatric Conditions
Neurotoxicity
While not addressed in the TTASAAN report (1), perfusion SPECT neuroimaging has proven valuable in the evaluation of encephalopathy and of psychiatric cases wherein toxic encephalopathy may be a contributing factor to symptomatology. Solvent-induced encephalopathy has been investigated with perfusion SPECT (109, 278–280). Perfusion SPECT revealed diffuse hypoperfusion in 94% of cases in one study (279), while CT and MRI identified abnormalities in only 7 and 29%, respectively. Perfusion SPECT reveals diffuse hypoperfusion in metal toxicity (281), mold toxicity (282), and other toxin exposure (109, 283, 284), including recreational toxins (284–290). SPECT is also beneficial in the identification and grading of severity of hepatic encephalopathy due to ammonia toxicity (291–295), even in mild cases (296), as well as for tracking progress (295). Carbon monoxide poisoning is characterized by decreased perfusion of the bilateral frontal cortex, bilateral temporal cortex, and the globus pallidus (297–301) and perfusion SPECT has been used to document these findings. To the extent that toxicity can induce symptoms which can be confused with psychiatric symptoms, perfusion SPECT can lead to clarification of the diagnosis and detoxification, rather than psychiatric pharmacology. For example, in a patient with signs of decreased frontal lobe function (e.g., ADHD, aggression, anti-social personality disorder) a perfusion SPECT finding of diffuse hypoperfusion increases the likelihood of toxicity as the true cause of the frontal lobe dysfunction (109, 110, 302).
Specifically concerning recreational drugs, perfusion SPECT imaging reveals diffuse hypoperfusion throughout the cerebral cortices, but predominately in the frontal and temporal cortices (303–307). Dopamine transporter SPECT scans (DaTscans) demonstrate the presence and availability of dopamine transporter sites (DAT). A recent meta-analysis demonstrated reduced DAT density in the striatum, as well as all areas of cortex, among abusers of cocaine, methamphetamine, and amphetamine, consistent with down-regulation of the dopamine system among stimulant abusers (308, 309). In contrast, nicotine abusers did not show decreased DAT availability (309). Similar decreases in DAT density have been reported in opiate abusers (310). Similar findings have been reported with PET tracers for the DAT (311).
Carbon monoxide (CO) poisoning warrants a separate description because of its distinct findings on perfusion neuroimaging. In addition to the acute toxic effects of CO, victims can experience delayed neurological symptoms. Often significant recovery from the acute neurological insult can occur followed by a dramatic decline in neurological function, including loss of cognitive function, behavioral changes, gait disturbances, Parkinsonian symptoms, incontinence, and aphasia (301, 312–315). In acute CO toxicity, perfusion SPECT neuroimaging can reveal decreased perfusion in the basal ganglia (300, 316–318), along with decreased perfusion of the bilateral frontal, temporal, and (to a lesser extent) parietal cortices (300, 301, 316, 318). The degree of cortical hypoperfusion can be correlated with the degree of neuropsychological impairment (300, 317, 319, 320). Often CT scans are normal, with 0% sensitivity vs. 70% sensitivity for SPECT scan in one study (309). Perfusion SPECT scans were also more sensitive (66%) than EEG (25%) in the acute setting (321).
Similarly, perfusion SPECT neuroimaging has proven useful in the evaluation of delayed neuropsychiatric sequalae of CO poisoning, as well in assessing response to treatments (301, 314, 315, 322–325). Hypoperfusion of the basal ganglia have been correlated with the well-established finding of T2 hyperintensities in the globus pallidus by MRI (314, 320, 324). Similarly, hypoperfusion in bilateral frontal and temporal cortices has been correlated with reduced anisotropy by diffusion tensor imaging (325). The degree of cerebral hypoperfusion has been correlated with cognitive impairment (299, 301, 320) and with symptomatic improvement following treatments, such as hyperbaric oxygen therapy (314, 315, 322, 325). While bilateral globus pallidus injury, edema, and necrosis are considered a hallmark MRI finding in chronic CO toxicity, not every patient manifests these severe findings. For example, in a series of 21 patients with chronic CO toxicity, 38% had abnormal MRI findings, while 67% had abnormal perfusion SPECT scans (319). This question was again examined with a group of 30 patients with chronic CO toxicity (320). MRI demonstrated greater sensitivity, while perfusion SPECT had higher specificity, positive predictive value, and negative predictive value for persistent neuropsychiatric symptoms (320).
Psychiatric Indications
The use of perfusion SPECT neuroimaging for psychiatric indications has increased significantly over the past two decades. Unfortunately, it has not been widely adopted in either nuclear medicine or psychiatry for several reasons. First unlike neurological diagnoses, which are ultimately verifiable by biopsy, there are no recognized histopathological markers for psychiatric diagnoses, which is the case with Alzheimer's disease, stroke, or other dementias. Rather, psychiatric disorders are defined by the Diagnostic and Statistical Manual of Mental Disorders Version 5 (DSM-5). Psychiatric diagnoses are based not on pathology but upon a constellation of symptoms. However, there is often tremendous range in how patient's symptoms present. For example, using the DSM-5 (326) diagnostic criteria for Major Depressive Disorder (110, 327), there are over 20 possible distinct phenotypes of this single diagnosis. The failure of clinical trials to effectively treat depression has led experts to state
“…that major depressive disorder is biologically heterogeneous, such that different treatments differ in the likelihood of achieving remission in different patients” (328).
Since psychiatry has difficulty establishing correct diagnoses and therapies, it is not surprising that perfusion SPECT has not established pathognomonic perfusion patterns.
Second, comorbidity is the rule rather than the exception in psychiatric conditions. It is difficult to identify a patient with pure bipolar disorder, for example, when the comorbidity of attention-deficit-hyperactivity-disorder (ADHD) occurs in ~57% of adult bipolar patients (329) and up to 98% of pediatric bipolar cases (330). Similarly, in depression, in addition to having a multiplicity of presentations, many depressed patients also are comorbid for anxiety in up to 60% of cases (327, 331, 332). Patients with ADHD frequently have coexisting mood disorders (59%), anxiety, oppositional disorders, or learning disorders (327, 333–336). For all these reasons, it is highly unlikely that a pathognomonic finding or a “neuroimaging fingerprint” will be found for depression, anxiety, bipolar disorder, obsessive compulsive disorder (OCD), or ADHD. Indeed, these complexities result in widespread failure of the diagnostic criteria in field testing (337).
Despite these limitations, a substantial body of research literature exists for brain perfusion SPECT in the evaluation of psychiatric disorders. In addition, hundreds of thousands of perfusion SPECT scans have been performed worldwide since the development of the technology in the 1990's. Certain patterns or highly consistent findings have been replicated in the research literature. Findings with extensive clinical correlation will be noted here.
Attention Deficit Hyperactivity Disorder
Decreased frontal lobe perfusion is a consistent finding in ADHD across multiple SPECT studies (327, 338–345) and confirmed by multiple functional MRI studies (346, 347) and infrared spectroscopy (348). For example, SPECT scans of medication-naïve children with ADHD (N = 40) were compared to normal controls using statistical parametric analysis (338). Decreased perfusion was found in the prefrontal cortex, orbitofrontal cortex, and middle temporal gyri, while increased perfusion was found in the somatosensory cortex and anterior cingulate gyri (338). With stimulant treatment, perfusion increased in the prefrontal cortex (338, 343) corresponding with clinical improvement of ADHD symptoms. Clinical experience has heavily supported these findings (109, 302, 349).
Perfusion SPECT neuroimaging also is beneficial in the differential diagnosis of ADHD. Since inattention, impulsivity, and hyperactivity are non-specific signs of frontal lobe dysfunction, it is not surprising that toxicity, concussive brain injury, incipient bipolar disorder, infection, and inflammation can produce similar symptom constellations as ADHD. SPECT can reveal these alternative causes (109, 110, 302, 349). For example, infection or toxicity likely will appear as diffuse hypoperfusion as elaborated in the section on Neurotoxicity above. Brain injury will likely appear as an asymmetrical area of hypoperfusion. In contrast, bipolar disorder will present as described in the next section.
Bipolar Disorder
In contrast, bipolar mania, which can present symptomatically like ADHD, often demonstrates increased perfusion in the frontal cortex, particularly the dorsolateral prefrontal cortex and possibly greater on the left (350, 351). Patients with bipolar mania also typically do not show the decrease in prefrontal perfusion unless they have comorbid ADHD as described above (109). While the total number of subjects studied in ADHD and bipolar disorder number <200, the clinical experience across hundreds of thousands of scans supports the correlation of these disease processes with these perfusion patterns.
Increased and asymmetric perfusion of the thalamus may serve as a possible endophenotypic pattern of bipolar disorder in the manic or euthymic states (352–354). Bipolar depression may be similar to unipolar depression in terms of decreased frontal cortex perfusion (355), but it is possible the two can be distinguished by differences in the perfusion of the thalamus and basal ganglia in the depressed state. Perfusion, whether measured by SPECT or fMRI, is increased in the thalamus in bipolar disorder (350, 353–357). It must be emphasized that these types of endophenotypic patterns may not be evident upon visual inspection of tomographic data for an individual SPECT scan. Rather, these findings may only be manifest in the statistical comparison of perfusion data to normative databases.
Depression
Over 150 studies of perfusion SPECT imaging in depression containing more than 12,100 subjects have been completed. A consistent finding in early SPECT (Xenon or HMPAO) studies of depression was decreased perfusion in the frontal, and often temporal, cortices, as well as the superior anterior cingulate gyri (358–361). Later, two distinct patterns of perfusion were recognized – decreased perfusion in typical and melancholic depression and increased frontal lobe perfusion in atypical depression (362–365). Increased perfusion in the subgenual anterior cingulate gyrus in treatment-resistant depression was first described by Goodwin et al. (366) and has been recognized as a hallmark sign of treatment resistant depression, subsequently (365, 367, 368). Remission or response to treatment is characteristically followed by increased perfusion in the affected areas (366, 369, 370). Response could be predicted by the degree of frontal hypoperfusion and of subgenual hyperperfusion. Notably, response to serotonin reuptake inhibitors was predicted by higher frontal and cingulate perfusion (368, 371, 372), while response to electroconvulsive therapy (ECT) or transcranial magnetic stimulation (TMS) was predicted by lower frontal and cingulate perfusion. Increased metabolic activity and perfusion in the thalamus (373, 374) is also a frequently reported finding in unipolar depression. Increased symmetrical perfusion of the thalamus has been consistently seen by clinicians on tens of thousands of perfusion SPECT scans.
Obsessive Compulsive Disorder
OCD is considered to result from an abnormal overactivity of a circuit involving the frontal cortices, anterior cingulate gyri, caudate nuclei and the thalami (375). Increased perfusion of the caudate nuclei and the anterior cingulate gyri have been reliable perfusion SPECT findings across 10 studies involving 196 subjects with OCD vs. 117 controls (376–385). Similar increased metabolism in these same areas has been found in studies utilizing FDG-PET (386, 387) and fMRI (388, 389). These findings were recently reviewed by Hazari et al. (390). Moreover, these findings have been consistently seen by clinicians on tens of thousands of perfusion SPECT scans.
Post-traumatic Stress Disorder
The symptom overlap between post-traumatic stress disorder (PTSD) and traumatic brain injury has complicated the correct diagnosis, particularly among military personnel (391, 392). Perfusion SPECT studies and FDG-PET studies have made similar findings in PTSD. Increased perfusion of the caudate nuclei is often found in PTSD (52, 53, 393). Another SPECT study showed that compared to controls, PTSD patients had increased cerebral blood flow in the limbic regions along with decreased perfusion in the superior frontal, parietal, and temporal regions (394). Systematic analyses of multiple regions of the default mode network involving 10,076 patients with TBI, PTSD, or both compared to 11,263 controls, revealed that PTSD resulted in increased perfusion in the basal ganglia, cingulate gyri, thalami, prefrontal cortices, and medial temporal cortices in both military (52) and civilian (53) populations. Provocation studies using perfusion SPECT, perfusion PET, and fMRI have shown increased perfusion in the amygdala, hippocampus, insula, but decreased perfusion in the medial prefrontal cortex (395–398).
Discussion and Actionable Recommendations
Given the major advances in our understanding of the SPECT functional neuroimaging findings for both neurological and psychiatric conditions as iterated above, the position assumed in the wake of the TTASAAN report by both the fields of Neurology and Psychiatry do not appear tenable. As a result, the following recommendations are made for the revisions of the current policies and practices as they relate to perfusion SPECT functional neuroimaging:
1) Increase awareness of the actual current state of the art as iterated above.
2) Replace assumptions about the inferiority of SPECT neuroimaging compared to PET, fMRI, diffusion tensor imaging, and MEG neuroimaging, particularly in the areas of dementia, TBI, seizure disorders, and neuropsychiatric indications with updated comparisons as elaborated herein.
3) Foster collaboration and communication between Nuclear Medicine physicians knowledgeable about perfusion SPECT neuroimaging and neurologists, psychiatrists, and other prescribers.
4) Improve knowledge of the technical aspects of perfusion SPECT neuroimaging to improve understanding of the limitations and strengths of the procedure. This will be addressed in Part II of this two-part series (399).
5) Revise Nuclear Medicine procedure guidelines to match the current state of the art as elaborated herein. This process has already begun with the publication of the new Canadian Association of Nuclear Medicine Guidelines for Brain Perfusion Single Photon Emission Computed Tomography (SPECT) (3).
6) Revise Neurology practice guidelines to include the use of perfusion SPECT neuroimaging in the areas for which it has been shown to be most effective or on par with FDG-PET neuroimaging (e.g., seizure disorders, dementia, stroke). The case is made herein that SPECT is a potent tool in the evaluation of TBI and warrants inclusion in Neurology practice guidelines based on Level IIa evidence meeting the criteria for a Type A recommendation by the standards set forth in the TTASAAN report (1) and the moral impossibility of achieving Class I evidence.
7) Revise Neurology and Psychiatry practice guidelines to recognize the advances in the state of the art of SPECT neuroimaging in the evaluation of neuropsychiatric indications. As the Canadian Association of Nuclear Medicine Guidelines for Brain Perfusion Single Photon Emission Computed Tomography (SPECT) (3) describes, the diagnostic picture for all of neuroimaging is clouded due to the subjective and non-physiological bases of psychiatric diagnoses. This remains an unresolved issue.
8) Understand the basics of reading and interpreting a perfusion brain SPECT scan based on the findings summarized herein. This will be addressed in Part II of this two-part series (399).
Perfusion SPECT scans require rigorous technique and correct adjustment of the equipment. An enormous variability in SPECT images results from technical inconsistency. The number of brain SPECT procedures performed annually is relatively small, largely due to the small number of scans ordered by treating physicians. One of the reasons why brain SPECT imaging is not prescribed more often is that prior encounters with sub-optimal images resulting from poor technique and lack of experience, have produced unhelpful findings. These negative experiences deter further use of SPECT scans and leads to fewer referrals. In part because of this dynamic and the parallel academic pressure to move to PET-based procedures, the attention, experience, and interest of nuclear medicine physicians has shifted away from SPECT imaging. In addition, the wholesale bias against perfusion SPECT scans discussed heretofore has stymied the clinical growth of this inexpensive, useful, and readily available procedure. The technical aspects of correctly performing and reading these scans will be detailed in part II of this two-part series (399).
Although the focus within the nuclear medicine community has shifted toward brain PET imaging, there currently is a growing interest in brain SPECT from the field of psychiatry as it becomes more evident that these scans can be immensely helpful in developing treatment plans for certain conditions. Moreover, with the recent introduction of new camera technologies detailed in part II of this two-part series (399), it is possible to produce images that rival FDG-PET quality with lower radiation exposure and less cost.
Conclusions
Simply put—this is not your father's Oldsmobile. The SPECT scans of the early 1990's were limited due to single-headed gamma cameras, unstable or rapidly decaying tracers, limited post-processing techniques, and absence of quantitative analysis. Twenty-five years later, SPECT scans have much greater resolution, multiple normative databases, numerous studies often with quite large sample sizes, and sophisticated post-processing software. Moreover, the introduction of a new solid-state detector system utilizing cadmium-zinc-telluride (CZT) diodes will greatly increase the resolution of SPECT and reduce the radiation dose required. These improvements will address two limitations often cited concerning SPECT. These technical aspects are detailed in Part II of this series.
The path into the future will be paved with collaborations between nuclear medicine physicians, neurologists, and psychiatrists. Hopefully, enlightened clinicians in these fields will join together to deepen their clinical experience; hence, advancing and expand our understanding of the perfusion SPECT neuroimaging correlates in neurology and neuropsychiatry yielding improved treatment outcomes as has been demonstrated in several pilot studies (109, 110, 302, 349, 400).
Author Contributions
DP and TH conceived the manuscript. DP, TH, and SD wrote initial drafts. TH and SD prepared revisions and prepared the figures. All authors contributed to the article and approved the submitted version.
Conflict of Interest
DP is Director of PathFinder Brain SPECT which is a clinical service corporation providing SPECT functional neuroimaging and had no research funding. He is deceased. TH is the president and principal owner of The Synaptic Space, a neuroimaging consulting firm. He is also CEO and Chairman of the Board of Neuro-Luminance Corporation, a medical service company. He is also president and principal owner of Dr. Theodore Henderson, Inc., a medical service company. He is also Vice-President of the Neuro-Laser Foundation, a non-profit organization. He is a member of and a former officer of the Brain Imaging Council Board of the Society of Nuclear Medicine and Molecular Imaging (SNMMI). Since 2017, he has served in the SNMMI Brain Imaging Outreach Working Group. Currently, he serves as president of the International Society of Applied Neuroimaging. TH has no ownership in, and receives no remuneration from, any neuroimaging company. No more than 5% of his income is derived from neuroimaging. SD is President of Good Lion Imaging LLC involved in the post-processing and display of functional brain scan data.
The reviewer DA declared a past co-authorship with one of the authors TH to the handling editor.
Publisher's Note
All claims expressed in this article are solely those of the authors and do not necessarily represent those of their affiliated organizations, or those of the publisher, the editors and the reviewers. Any product that may be evaluated in this article, or claim that may be made by its manufacturer, is not guaranteed or endorsed by the publisher.
Acknowledgments
We would like to acknowledge the following individuals, as well as the entire International Society of Applied Neuroimaging, for their contributions to the discussions of these matters reviewed herein: Phil Cohen, MD, FRCPC; Muriel J. van Lierop, MBBS, MDPAC(M); Mary McLean, MB, ChB, FRCP; John Michael Uszler, MD, MS; John F. Rossiter-Thornton, MB, FRCPC; J. Cardaci, MBBS, FAANMS, FRACP; Leonard Numerow MD FRCP(C); Yin-Hui Siow, MD, FRCPC. We also acknowledge Jenny Faherty for technical assistance and copy-editing. We acknowledge Alaina M. Henderson for assistance with the preparation of the figures.
References
1. Report of the Therapeutics Technology Assessment Subcommittee of the American Academy of Neurology. Assessment of brain SPECT. Neurology. (1996) 46:278–85. doi: 10.1212/WNL.46.1.278
2. Kapucu OL, Nobili F, Varrone A, Booij J, Vander Borght T, Någren K, et al. EANM procedure guideline for brain perfusion SPECT using 99mTc-labelled radiopharmaceuticals, version 2. Eur J Nucl Med Mol Imaging. (2009) 36:2093–102. doi: 10.1007/s00259-009-1266-y
3. Canadian Association of Nuclear Medicine. CANM Guidelines for Brain Perfusion Single Photon Emission Computed Tomography (SPECT). (2021). Available online at: https://canm-acmn.ca/guidelines/ (accessed July 14, 2021).
4. Mazziotta JC, Collins RC., William H., Oldendorf M.D. (1925-1992). J Comput Assist Tomogr. (1993) 17:169–71. doi: 10.1097/00004728-199303000-00001
5. van Dellen JR, Danziger A. Failure of computerized tomography to differentiate between radiation necrosis and cerebral tumour. S Afr Med J. (1978) 53:171–2.
7. French BN. Limitations and pitfalls of computed tomography in the evaluation of craniocerebral injury. Surg Neurol. (1978) 10:395–401.
8. Stockburger WT. CT imaging, then and now: a 30-year review of the economics of computed tomography. Radiol Manage. (2004) 26: 24–7.
9. McKinney W. History of Neuroimaging. The American Society of Neuroimaging. (2021). Available online at: https://www.asnweb.org/i4a/pages/index.cfm?pageID=3334&activateFull=true (accessed June 6, 2021).
10. First MB, Drevets WC, Carter C, Dickstein DP, Kasoff L, Kim KL. Clinical applications of neuroimaging in psychiatric disorders. Am J Psychiatry. (2018) 175:915–16. doi: 10.1176/appi.ajp.2018.1750701
11. Eklund A, Nichols TE, Knutsson H. Cluster failure: why fMRI inferences for spatial extent have inflated false-positive rates. Proc Natl Acad Sci USA. (2016) 113:7900–5. doi: 10.1073/pnas.1602413113
12. Szaflarski JP, Gloss D, Binder JR, Gaillard WD, Golby AJ, Holland SK, et al. Practice guideline summary: Use of fMRI in the presurgical evaluation of patients with epilepsy: Report of the Guideline Development, Dissemination, and Implementation Subcommittee of the American Academy of Neurology. Neurology. (2017) 88:395–402. doi: 10.1212/WNL.0000000000003532
13. Wang ZJ, Seo Y, Babcock E, Huang H, Bluml S, Wisnowski J, et al. Assessment of diffusion tensor image quality across sites and vendors using the American College of Radiology head phantom. J Appl Clin Med Phys. (2016) 17:442–51. doi: 10.1120/jacmp.v17i3.5972
14. Eierud C, Craddock RC, Fletcher S, Aulakh M, King-Casas B, Kuehl D, et al. Neuroimaging after mild traumatic brain injury: review and meta-analysis. Neuroimage Clin. (2014) 4:283–94. doi: 10.1016/j.nicl.2013.12.009
15. Asken BM, DeKosky ST, Clugston JR, Jaffee MS, Bauer RM. Diffusion tensor imaging (DTI) findings in adult civilian, military, and sport-related mild traumatic brain injury (mTBI): a systematic critical review. Brain Imaging Behav. (2018) 2:585–612. doi: 10.1007/s11682-017-9708-9
16. Patterson J Wyper DJ. Basics of SPECT. In: Duncan R, editors. SPECT Imaging of the Brain. Lancaster: Kluwer Academic Publishers (1997). doi: 10.1007/978-94-011-5398-0_1
17. Jaszczak RJ. The early years of single photon emission computed tomography (SPECT): an anthology of selected reminiscences. Phys Med Biol. (2006) 51:R99–115. doi: 10.1088/0031-9155/51/13/R07
18. Harper PV, Beck RM, Charleston DE, Brunsten B, Lathrop KA. Three-dimensional mapping and display of radioisotope distributions. J Nucl Med. (1965) 6:332.
19. Winchell HS, Horst WD, Braun L, Oldendorf WH, Hattner R, Parker H. N-isopropyl-[123I] p-iodoamphetamine: single-pass brain uptake and washout; binding to brain synaptosomes; and localization in dog and monkey brain. J Nucl Med. (1980) 21:947–52.
20. Ell PJ, Hocknell JM, Jarritt PH, Cullum I, Lui D, Campos-Costa D, et al. A 99Tcm-labelled radiotracer for the investigation of cerebral vascular disease. Nucl Med Commun. (1985) 6:437–41. doi: 10.1097/00006231-198508000-00002
21. Neirinckx RD, Burke JF, Harrison RC, Forster AM, Andersen AR, Lassen NA. The retention mechanism of technetium-99m-HM-PAO: intracellular reaction with glutathione. J Cereb Blood Flow Metab. (1988) 8:S4–12. doi: 10.1038/jcbfm.1988.27
22. Sharp PF, Smith FW, Gemmell HG, Lyall D, Evans NT, Gvozdanovic D, et al. Technetium-99m HM-PAO stereoisomers as potential agents for imaging regional cerebral blood flow: human volunteer studies. J Nucl Med. (1986) 27:171–7.
23. Lassen NA, Andersen AR, Friberg L, Paulson OB. The retention of [99mTc]-d,l-HM-PAO in the human brain after intracarotid bolus injection: a kinetic analysis. J Cereb Blood Flow Metab. (1988) 8:S13–22. doi: 10.1038/jcbfm.1988.28
24. Inugami A, Kanno I, Uemura K, Shishido F, Murakami M, Tomura N, et al. Linearization correction of 99mTc-labeled hexamethyl-propylene amine oxime (HM-PAO) image in terms of regional CBF distribution: comparison to C15O2 inhalation steady-state method measured by positron emission tomography. J Cereb Blood Flow Metab. (1988) 8:S52–60. doi: 10.1038/jcbfm.1988.33
25. Holman BL, Hellman RS, Goldsmith SJ, Mena IG, Leveille J, Gherardi PG, et al. Biodistribution, dosimetry, and clinical evaluation of technetium-99m ethyl cysteinate dimer in normal subjects and in patients with chronic cerebral infarction. J Nucl Med. (1989) 30:1018–24.
26. Ichise M, Chung DG, Wang P, Wortzman G, Gray BG, Franks W. Technetium-99m-HMPAO SPECT, CT and MRI in the evaluation of patients with chronic traumatic brain injury: a correlation with neuropsychological performance. J Nucl Med. (1994) 35:217–26.
27. Roper SN, Mena I, King WA, Schweitzer J, Garrett K, Mehringer CM, et al. An analysis of cerebral blood flow in acute closed-head injury using technetium-99m-HMPAO SPECT and computed tomography. J Nucl Med. (1991) 32:1684–7.
28. Gray BG, Ichise M, Chung DG, Kirsh JC, Franks W. Technetium-99m-HMPAO SPECT in the evaluation of patients with a remote history of traumatic brain injury: a comparison with x-ray computed tomography. J Nucl Med. (1992) 33:52–8.
29. Stapleton SJ, Caldwell CB, Leonhardt CL, Ehrlich LE, Black SE, Yaffe MJ. Determination of thresholds for detection of cerebellar blood flow deficits in brain SPECT images. J Nucl Med. (1994) 35:1547–55.
30. Krupinski EA. The role of perception in imaging: past and future. Semin Nucl Med. (2011) 41:392–400. doi: 10.1053/j.semnuclmed.2011.05.002
31. Kim BJ, Kim YH, Kim YJ, Ahn SH, Lee DH, Kwon SU, et al. Color-coded fluid-attenuated inversion recovery images improve inter-rater reliability of fluid-attenuated inversion recovery signal changes within acute diffusion-weighted image lesions. Stroke. (2014) 45:2801–4. doi: 10.1161/STROKEAHA.114.006515
32. Mena FJ, Mena I, Alamos F, Solé C, Neurbauer S, et al. SPECT Tc99m-HMPAO brain uptake in normal children: a comparison to normal elderly subjects. ALASBINM J. (1998) 1:4055.
33. Devous MD Sr, Stokely EM, Chehabi HH, Bonte FJ. Normal distribution of regional cerebral blood flow measured by dynamic single-photon emission tomography. J Cereb Blood Flow Metab. (1986) 6:95–104. doi: 10.1038/jcbfm.1986.12
34. Bigler ED. Neurobiology and neuropathology underlie the neuropsychological deficits associated with traumatic brain injury. Arch Clin Neuropsychol. (2003) 18:595–621. doi: 10.1016/S0887-6177(02)00156-7
35. Bigler ED, Maxwell WL. Neuropathology of mild traumatic brain injury: relationship to neuroimaging findings. Brain Imaging Behav. (2012) 6:108–36. doi: 10.1007/s11682-011-9145-0
36. Centers for Disease Control and Prevention, National Center for Injury Prevention and Control. Report to Congress on Traumatic Brain Injury in the United States: Epidemiology and Rehabilitation. Atlanta, GA: Centers for Disease Control and Prevention (2015).
37. Mountney A, Boutté AM, Cartagena CM, Flerlage WF, Johnson WD, Rho C, et al. Functional and molecular correlates after single and repeated rat closed-head concussion: indices of vulnerability after brain injury. J Neurotrauma. (2017) 34:2768–89. doi: 10.1089/neu.2016.4679
38. Barkhoudarian G, Hovda DA, Giza CC. The molecular pathophysiology of concussive brain injury - an update. Phys Med Rehabil Clin N Am. (2016) 27:373–93. doi: 10.1016/j.pmr.2016.01.003
39. WHO (World Health Organization). International Statistical Classification of Diseases and Related Health Problems. (2010). Available online at: http://apps.who.int/classifications/icd10/browse/2010/en (accessed May 24, 2018).
40. Jacobs A, Put E, Ingels M, Bossuyt A. Prospective evaluation of technetium-99m-HMPAO SPECT in mild and moderate traumatic brain injury. J Nucl Med. (1994) 35:942–7.
41. Abdel-Dayem HM, Sadek SA, Kouris K, Bahar RH, Higazi I, Eriksson S, et al. Changes in cerebral perfusion after acute head injury: comparison of CT with Tc-99m HM-PAO SPECT. Radiology. (1987) 165:221–6. doi: 10.1148/radiology.165.1.3498184
42. Ducours JL, Role C, Guillet J, San Galli F, Caix P, Wynchank S. Cranio-facial trauma and cerebral SPECT studies using N-isopropyl-iodo-amphetamine (123I). Nucl Med Commun. (1990) 11:361–7. doi: 10.1097/00006231-199005000-00003
43. Masdeu JC, Van Heertum RL, Kleiman A, Anselmi G, Kissane K, Horng J, et al. Early single-photon emission computed tomography in mild head trauma. A controlled study. J Neuroimaging. (1994) 4:177–81. doi: 10.1111/jon199444177
44. Raji CA, Tarzwell R, Pavel D, Schneider H, Uszler M, Thornton J, et al. Clinical utility of SPECT neuroimaging in the diagnosis and treatment of traumatic brain injury: a systematic review. PLoS ONE. (2014) 9:e91088. doi: 10.1371/journal.pone.0091088
45. Nedd K, Sfakianakis G, Ganz W, Uricchio B, Vernberg D, Villanueva P, et al. 99mTc-HMPAO SPECT of the brain in mild to moderate traumatic brain injury patients: compared with CT-a prospective study. Brain Inj. (1993) 7:469–79. doi: 10.3109/02699059309008174
46. Kesler SR, Adams HF, Bigler ED. SPECT, MR and quantitative MR imaging: correlates with neuropsychological and psychological outcome in traumatic brain injury. Brain Inj. (2000) 14:851–7. doi: 10.1080/026990500445682
47. Stamatakis EA, Wilson JT, Hadley DM, Wyper DJ. SPECT imaging in head injury interpreted with statistical parametric mapping. J Nucl Med. (2002) 43:476–83.
48. Kinuya K, Kakuda K, Nobata K, Sakai S, Yamamoto K, Itoh S, et al. Role of brain perfusion single-photon emission tomography in traumatic head injury. Nucl Med Commun. (2004) 25:333–7. doi: 10.1097/00006231-200404000-00004
49. Pavel D, Jobe T, Devore-Best S, Davis G, Epstein P, Sinha S, et al. Viewing the functional consequences of traumatic brain injury by using brain SPECT. Brain Cogn. (2006) 60:211–3.
50. Atighechi S, Salari H, Baradarantar MH, Jafari R, Karimi G, Mirjali M. A comparative study of brain perfusion single-photon emission computed tomography and magnetic resonance imaging in patients with post-traumatic anosmia. Am J Rhinol Allergy. (2009) 23:409–12. doi: 10.2500/ajra.2009.23.3345
51. Joglekar SS, Bell JR, Caroline M, Chase PJ, Domesek J, Patel PS, et al. Evaluating the role of single-photon emission computed tomography in the assessment of neurotologic complaints. Ear Nose Throat J. (2014) 93:168–73. doi: 10.1177/0145561314093004-512
52. Raji CA, Willeumier K, Taylor D, Tarzwell R, Newberg A, Henderson TA, et al. Functional neuroimaging with default mode network regions distinguishes PTSD from TBI in a military veteran population. Brain Imaging Behav. (2015) 9:527–34. doi: 10.1007/s11682-015-9385-5
53. Amen DG, Raji CA, Willeumier K, Taylor D, Tarzwell R, Newberg A, et al. Functional neuroimaging distinguishes posttraumatic stress disorder from traumatic brain injury in focused and large community datasets. PLoS ONE. (2015) 10:e0129659. doi: 10.1371/journal.pone.0129659
54. Davalos DB, Bennett TL. A review of the use of single-photon emission computerized tomography as a diagnostic tool in mild traumatic brain injury. Appl Neuropsychol. (2002) 9:92–105. doi: 10.1207/S15324826AN0902_4
55. Wortzel HS, Filley CM, Anderson CA, Oster T, Arciniegas DB. Forensic applications of cerebral single photon emission computed tomography in mild traumatic brain injury. J Am Acad Psychiatry Law. (2008) 36:310–22.
56. Amyot F, Arciniegas DB, Brazaitis MP, Curley KC, Diaz-Arrastia R, Gandjbakhche A, et al. A review of the effectiveness of neuroimaging modalities for the detection of traumatic brain injury. J Neurotrauma. (2015) 32:1693–721. doi: 10.1089/neu.2013.3306
57. Bigler ED. Systems biology, neuroimaging, neuropsychology, neuroconnectivity and traumatic brain injury. Front Syst Neurosci. (2016) 10:55. doi: 10.3389/fnsys.2016.00055
58. Yeates KO, Levin HS, Ponsford J. The neuropsychology of traumatic brain injury: looking back, peering ahead. J Int Neuropsychol Soc. (2017) 23:806–17. doi: 10.1017/S1355617717000686
59. Sataloff RT, Mandel S, Muscal E, Park CH, Rosen DC, Kim SM, et al. Single-photon-emission computed tomography (SPECT) in neurotologic assessment: a preliminary report. Am J Otol. (1996)17:909–16.
60. Abdel-Dayem HM, Abu-Judeh H, Kumar M, Atay S, Naddaf S, El-Zeftawy H, et al. SPECT brain perfusion abnormalities in mild or moderate traumatic brain injury. Clin Nucl Med. (1998) 23:309–17. doi: 10.1097/00003072-199805000-00009
61. Abu-Judeh HH, Parker R, Aleksic S, Singh ML, Naddaf S, Atay S. SPECT brain perfusion findings in mild or moderate traumatic brain injury. Nucl Med Rev Cent East Eur. (2000) 3:5–11.
62. Mazzini L, Cossa FM, Angelino E, Campini R, Pastore I, Monaco F. Posttraumatic epilepsy: neuroradiologic and neuropsychological assessment of long-term outcome. Epilepsia. (2003) 44:569–74. doi: 10.1046/j.1528-1157.2003.34902.x
63. Amen DG, Newberg A, Thatcher R, Jin Y, Wu J, Keator D, et al. Impact of playing American professional football on long-term brain function. J Neuropsychiatry Clin Neurosci. (2011) 23:98–106. doi: 10.1176/appi.neuropsych.23.1.98
64. Jacobs A, Put E, Ingels M, Put T, Bossuyt A. One-year follow-up of technetium-99m-HMPAO SPECT in mild head injury. J Nucl Med. (1996) 37:1605–9.
65. Mazzini L, Campini R, Angelino E, Rognone F, Pastore I, Oliveri G. Posttraumatic hydrocephalus: a clinical, neuroradiologic, and neuropsychologic assessment of long-term outcome. Arch Phys Med Rehabil. (2003) 84:1637–41. doi: 10.1053/S0003-9993(03)00314-9
66. Gowda NK, Agrawal D, Bal C, Chandrashekar N, Tripati M, Bandopadhyaya GP, et al. Technetium Tc-99m ethyl cysteinate dimer brain single-photon emission CT in mild traumatic brain injury: a prospective study. Am J Neuroradiol. (2006) 27:447–51.
67. Amen DG, Wu JC, Taylor D, Willeumier K. Reversing brain damage in former NFL players: implications for traumatic brain injury and substance abuse rehabilitation. J Psychoactive Drugs. (2011) 43:1–5. doi: 10.1080/02791072.2011.566489
68. Wells GA, Shea B, O'Connell D, Peterson J, Welch V, Losos M.. The Newcastle-Ottawa Scale (NOS) for Assessing the Quality of Nonrandomized Studies in Meta-Analyses. The Ottawa Hospital Research Institute. (2021). Available online at: http://www.ohri.ca/programs/clinical_epidemiology/oxford.asp (accessed June 20, 2021).
69. Henderson TA, Morries LD. SPECT perfusion imaging demonstrates improvement of traumatic brain injury with transcranial near-infrared laser phototherapy. Adv Mind Body Med. (2015) 29:27–33. doi: 10.2147/NDT.S65809
70. Obrist WD, Gennarelli TA, Segawa H, Dolinskas CA, Langfitt TW. Relation of cerebral blood flow to neurological status and outcome in head-injured patients. J Neurosurg. (1979) 51:292–300. doi: 10.3171/jns.1979.51.3.0292
71. Vavilala MS, Farr CK, Watanitanon A, Clark-Bell BC, Chandee T, Moore A, et al. Early changes in cerebral autoregulation among youth hospitalized after sports-related traumatic brain injury. Brain Inj. (2018) 32:269–75. doi: 10.1080/02699052.2017.1408145
72. Mathew P, Bullock R, Teasdale G, McCulloch J. Changes in local microvascular permeability and in the effect of intervention with 21-aminosteroid (Tirilazad) in a new experimental model of focal cortical injury in the rat. J Neurotrauma. (1996) 13:465–72. doi: 10.1089/neu.1996.13.465
73. Emanuelson IM, von Wendt L, Bjure J, Wiklund LM, Uvebrant P. Computed tomography and single-photon emission computed tomography as diagnostic tools in acquired brain injury among children and adolescents. Dev Med Child Neurol. (1997) 39:502–7. doi: 10.1111/j.1469-8749.1997.tb07477.x
74. Lorberboym M, Lampl Y, Gerzon I, Sadeh M. Brain SPECT evaluation of amnestic ED patients after mild head trauma. Am J Emerg Med. (2002) 20:310–3. doi: 10.1053/ajem.2002.34201
75. Shin YB, Kim SJ, Kim IJ, Kim YK, Kim DS, Park JH, et al. Voxel-based statistical analysis of cerebral blood flow using Tc-99m ECD brain SPECT in patients with traumatic brain injury: group and individual analyses. Brain Inj. (2006) 20:661–7. doi: 10.1080/02699050600677071
76. Randolph C. Neuropsychological testing: evolution and emerging trends. CNS Spectr. (2002) 7:307–12. doi: 10.1017/S1092852900017727
77. Sherman E, Brooks B, Iverson G, Slick D, Strauss E. Reliability and Validity in Neuropsychology. In: Schoenberg MR, Scott JG, editors. The Little Black Book of Neuropsychology: A Syndrome-Based Approach. New York, NY: Springer Science+Business Media, LLC (2011). doi: 10.1007/978-0-387-76978-3_30
78. Oestreicher JM, O'Donnell JP. Validation of the general neuropsychological deficit scale with nondisabled, learning-abled and head-injured young adults. Arch Clin Neuropsychol. (1995) 10:185–91. doi: 10.1016/0887-6177(94)E0039-R
79. Reitan RM, Wolfson D. The two faces of mild head injury. Arch Clin Neuropsychol. (1999) 14:191–202. doi: 10.1093/arclin/14.2.191
80. Green P. Why clinicians often disagree about the validity of test results. NeuroRehabilitation. (2001) 16:231–6. doi: 10.3233/NRE-2001-16407
81. Wade JB, DeMatteo D, Hart RP. Neuropsychologists diagnose traumatic brain injury. Brain Inj. (2004) 18:629–43. doi: 10.1080/02699050310001646198
82. Marenco S, Coppola R, Daniel DG, Zigun JR, Weinberger DR. Regional cerebral blood flow during the Wisconsin Card Sorting Test in normal subjects studied by xenon-133 dynamic SPECT: comparison of absolute values, percent distribution values, and covariance analysis. Psychiatry Res. (1993) 50:177–92. doi: 10.1016/0925-4927(93)90029-H
83. Kawasaki Y, Maeda Y, Suzuki M, Urata K, Higashima M, Kiba K, et al. SPECT analysis of regional cerebral blood flow changes in patients with schizophrenia during the Wisconsin Card Sorting Test. Schizophr Res. (1993) 10:109–16. doi: 10.1016/0920-9964(93)90045-K
84. Catafau AM, Parellada E, Lomena F, Bernardo M, Setoain J, Catarineu S, et al. Role of the cingulate gyrus during the Wisconsin Card Sorting Test: a single photon emission computed tomography study in normal volunteers. Psychiatry Res. (1998) 83:67–74. doi: 10.1016/S0925-4927(98)00031-6
85. Yang YK, Chen CC, Lee IH, Chou YH, Chiu NT, Jeffries KJ, et al. Association between regional cerebral blood flow and eye-tracking performance and the Wisconsin Sorting Test in schizophrenics: a single photon emission computed tomography study. Psychiatry Res. (2003) 123:37–48. doi: 10.1016/S0925-4927(03)00021-0
86. Goethals I, Audennaert K, Jacobs F, Lannoo E, Van de White C, Ham H, et al. Cognitive neuroactiviation using SPECT and the Stroop Colored Word Test in patients with diffuse brain injury. J Neurotrauma. (2004) 21:1059–69. doi: 10.1089/0897715041651051
87. Audenaert K, Lahorte P, Brans B, van Laere K, Goethals I, van Heeringen K, et al. The classical stroop interference task as a prefrontal activation probe: a validation study using 99T cm-ECD brain SPECT. Nucl Med Commun. (2001) 22:135–43. doi: 10.1097/00006231-200102000-00004
88. Goethals I, Audenaert K, Jacobs F, Van de Wiele C, Pyck H, Ham H, et al. Application of neuropsychological activation probe with SPECT: the “Tower of London” task in healthy volunteers. Nucl Med Commun. (2004) 25:177–82. doi: 10.1097/00006231-200402000-00015
89. Morris RG, Ahmed S, Syed GM, Toone BK. Neural correlates of planning ability: frontal lobe activation during the Tower of London test. Neuropsychologia. (1993) 31:1367–78. doi: 10.1016/0028-3932(93)90104-8
90. Ueda H, Kitabayashi Y, Narumoto J, Nakamura K, Kita H, Kishikawa Y, et al. Relationship between clock drawing test performance and regional cerebral blood flow in Alzheimer's disease: a single photon emission computed tomography study. Psychiatry Clin Neurosci. (2002) 56:25–9. doi: 10.1046/j.1440-1819.2002.00940.x
91. Bonte FJ, Harris TS, Roney CA, Hynan LS. Differential diagnosis between Alzheimer's disease and frontotemporal disease by the posterior cingulate sign. J Nucl Med. (2004) 45:771–74.
92. Audenaert K, Goethals I, Van Laere K, Lahorte P, Brans B, Versijpt J, et al. SPECT neuropsychological activation procedure with the Verbal Fluency Test in attempted suicide patients. Nucl Med Commun. (2002) 23:907–16. doi: 10.1097/00006231-200209000-00015
93. Wszolek ZK, Herkes GK, Lagerlund TD, Kokmen E. Comparison of EEG background frequency analysis, psychologic test scores, short test of mental status, and quantitative SPECT in dementia. J Geratr Psychiatry Neurol. (1992) 5:22–30. doi: 10.1177/002383099200500104
94. Fallon BA, Das S, Plutchok JJ, Tager F, Liegner K, Van Heertum R. Functional brain imaging and neuropsychological resting in Lyme disease. Clin Infect Dis. (1997) 25:S57–63. doi: 10.1086/516175
95. Belin C, Moroni C, Caillat-Vigneron N, Debray M, Baudin M, Dumas JL, et al. Central nervous system development in Sjogren's syndrome: evidence from neuropsychological testing and HMPAO-SPECT. Ann Med Interne. (1999) 150:598–604.
96. Landtblom AM, Dige N, Schwerdt K, Safstrom P, Granerus G. A case of Kleine-Levin Syndrome examined with SPECT and neuropsychological testing. Acta Neurol Scand. (2002) 105:318–21. doi: 10.1034/j.1600-0404.2002.1c162.x
97. Lacerda AL, Dalgalarrondo P, Caetano D, Haas GL, Camargo EE, Keshavan MS. Neuropsychological performance and regional cerebral blood flow in obsessive-compulsive disorder. Prog Neuropsychopharmacol Biol Psychiatry. (2003) 27:657–65. doi: 10.1016/S0278-5846(03)00076-9
98. Calandre EP, Bembibre J, Arnedo ML, Becerra D. Cognitive disturbances and regional cerebral blood flow abnormalities in migraine patients: their relationship with the clinical manifestations of the illness. Cephalalgia. (2002) 22:291–302. doi: 10.1046/j.1468-2982.2002.00370.x
99. Bak TH, Antoun N, Balan KK, Hodges JR. Memory lost, memory regained: neuropsychological findings and neuroimaging in two cases of paraneoplastic limbic encephalitis with radically different outcomes. J Neurol Neurosurg Psychiatry. (2001) 71:40–7. doi: 10.1136/jnnp.71.1.40
100. Sabri O, Ringelstein EB, Hellwig D, Schneider R, Schreckenberger M, Kaiser HJ, et al. Neuropsychological impairment correlates with hypoperfusion and hypometabolism but not with severity of white matter lesions on MRI in patients with cerebral microangiopathy. Stroke. (1999) 30:556–66. doi: 10.1161/01.STR.30.3.556
101. Nicolas JM, Catafau AM, Estruch R, Lomena FJ, Salamero M, Herranz R, et al. Regional cerebral blood flow-SPECT in chronic alcoholism: relation to neuropsychological testing. J Nucl Med. (1993) 34:1452–9.
102. Arbizu J, Larumbe R, Gamez C, Marti J, Martinez-Lage J, Richter J. Correlations between brain SPECT and neuropsychology in assessments in mild and moderate stages of Alzheimer's disease. Rev Esp Med Nucl. (1999) 18:252–60.
103. Hall RA, Fordyce DJ, Lee ME, Eisenberg B, Lee RF, Holmes JH, et al. Brain SPECT imaging and neuropsychological testing in coronary artery bypass patients: single photon emission computed tomography. Ann Thorac Surg. (1999) 68:2082–8. doi: 10.1016/S0003-4975(99)01213-8
104. Kant R, Smith-Seemiller L, Isaac G, Duffy J. Tc-HMPAO SPECT in persistent post-concussion syndrome after mild head injury: comparison with MRI/CT. Brain Inj. (1997) 11:115–24. doi: 10.1080/026990597123700
105. Umile EM, Plotkin RC, Sandel ME. Functional assessment of mild traumatic brain injury using SPECT and neuropsychological testing. Brain Inj. (1998) 12:577–94. doi: 10.1080/026990598122340
106. Matsushita M, Hosoda K, Naitoh Y, Yamashita H, Kohmura E. Utility of diffusion tensor imaging in the acute stage of mild to moderate traumatic brain injury for detecting white matter lesions and predicting long-term cognitive function in adults. J Neurosurg. (2011) 115:130–9. doi: 10.3171/2011.2.JNS101547
107. Laatsch L, Pavel D, Jobe T, Lin Q, Quintana JC. Incorporation of SPECT imaging in a longitudinal cognitive rehabilitation therapy programme. Brain Inj. (1999) 13:555–70. doi: 10.1080/026990599121304
108. Mez J, Stern RA, McKee AC. Chronic traumatic encephalopathy: where are we and where are we going? Curr Neurol Neurosci Rep. (2013) 13:407. doi: 10.1007/s11910-013-0407-7
109. Henderson TA, Uszler JM, Rossiter-Thornton JF, Siow Y-H, Pavel DG, McLean M, et al. The American Psychiatric Association fails to recognize the value of neuroimaging in psychiatry. Interv Med Clin Imaging. (2019) 1:1–8. Available online at: https://researchopenworld.com/the-american-psychiatric-association-fails-to-recognize-the-value-of-neuroimaging-in-psychiatry/
110. Henderson TA, van Lierop MJ, McLean M, Uszler JM, Thornton JF, Siow YH. Functional neuroimaging in psychiatry-aiding in diagnosis and guiding treatment. What the American Psychiatric Association does not know. Front Psychiatry. (2020) 11:276. doi: 10.3389/fpsyt.2020.00276
111. Wozniak JR, Krach L, Ward E, Mueller BA, Muetzel R, Schnoebelen S, et al. Neurocognitive and neuroimaging correlates of pediatric traumatic brain injury: a diffusion tensor imaging (DTI) study. Arch Clin Neuropsychol. (2007) 22:555–68. doi: 10.1016/j.acn.2007.03.004
112. Newcombe VF, Williams GB, Nortje J, Bradley PG, Harding SG, Smielewski P, et al. Analysis of acute traumatic axonal injury using diffusion tensor imaging. Br J Neurosurg. (2007) 21:340–8. doi: 10.1080/02688690701400882
113. Avants B, Duda JT, Kim J, Zhang H, Pluta J, Gee JC, et al. Multivariate analysis of structural and diffusion imaging in traumatic brain injury. Acad Radiol. (2008) 15:1360–75. doi: 10.1016/j.acra.2008.07.007
114. Mayer AR, Ling J, Mannell MV, Gasparovic C, Phillips JP, Doezema D, et al. A prospective diffusion tensor imaging study in mild traumatic brain injury. Neurology. (2010) 74:643–50. doi: 10.1212/WNL.0b013e3181d0ccdd
115. Matthews SC, Strigo IA, Simmons AN, O'Connell RM, Reinhardt LE, Moseley SA. A multimodal imaging study in U.S. veterans of Operations Iraqi and Enduring Freedom with and without major depression after blast-related concussion. Neuroimage. (2011) 54:S69–75. doi: 10.1016/j.neuroimage.2010.04.269
116. Lange RT, Iverson GL, Brubacher JR, Mädler B, Heran MK. Diffusion tensor imaging findings are not strongly associated with postconcussional disorder 2 months following mild traumatic brain injury. J Head Trauma Rehabil. (2012) 27:188–98. doi: 10.1097/HTR.0b013e318217f0ad
117. Yuh EL, Cooper SR, Mukherjee P, Yue JK, Lingsma HF, Gordon WA, et al. Diffusion tensor imaging for outcome prediction in mild traumatic brain injury: a TRACK-TBI study. J Neurotrauma. (2014) 31:1457–77. doi: 10.1089/neu.2013.3171
118. Gonzalez AC, Kim M, Keser Z, Ibrahim L, Singh SK, Ahmad MJ, et al. Diffusion tensor imaging correlates of concussion related cognitive impairment. Front Neurol. (2021) 12:639179. doi: 10.3389/fneur.2021.639179
119. Jones DK, Dardis R, Ervine M, Horsfield MA, Jeffree M, Simmons A, et al. Cluster analysis of diffusion tensor magnetic resonance images in human head injury. Neurosurgery. (2000) 47:306–13. doi: 10.1097/00006123-200008000-00008
120. Williams GR. Incidence and characteristics of total stroke in the United States. BMC Neurol. (2001) 1:2. doi: 10.1186/1471-2377-1-2
121. Avan A, Digaleh H, Di Napoli M, Stranges S, Behrouz R, Shojaeianbabaei G, et al. Socioeconomic status and stroke incidence, prevalence, mortality, and worldwide burden: an ecological analysis from the Global Burden of Disease Study 2017. BMC Med. (2019) 17:191. doi: 10.1186/s12916-019-1397-3
122. Kim J, Thayabaranathan T, Donnan GA, Howard G, Howard VJ, Rothwell PM, et al. Global stroke statistics 2019. Int J Stroke. (2020) 15:819–38. doi: 10.1177/1747493020909545
123. Yeh SH, Liu RS, Hu HH, Wong WJ, Lo YK, Lai ZY, et al. Brain SPECT imaging with 99Tcm-hexamethylpropyleneamine oxime in the early detection of cerebral infarction: comparison with transmission computed tomography. Nucl Med Commun. (1986) 7:873–8. doi: 10.1097/00006231-198612000-00004
124. Vallar G, Perani D, Cappa SF, Messa C, Lenzi GL, Fazio F. Recovery from aphasia and neglect after subcortical stroke: neuropsychological and cerebral perfusion study. J Neurol Neurosurg Psychiatry. (1988) 51:1269–76. doi: 10.1136/jnnp.51.10.1269
125. Rango M, Candelise L, Perani D, Messa C, Scarlato G, Canal N, Franceschi M, Fazio F. Cortical pathophysiology and clinical neurologic abnormalities in acute cerebral ischemia. A serial study with single photon emission computed tomography. Arch Neurol. (1989) 46:1318–22. doi: 10.1001/archneur.1989.00520480060021
126. De Roo M, Mortelmans L, Devos P, Verbruggen A, Wilms G, Carton H, et al. Clinical experience with Tc-99m HM-PAO high resolution SPECT of the brain in patients with cerebrovascular accidents. Eur J Nucl Med. (1989) 15:9–15. doi: 10.1007/BF00253592
127. Brass LM, Walovitch RC, Joseph JL, Léveillé J, Marchand L, Hellman RS, et al. The role of single photon emission computed tomography brain imaging with 99mTc-bicisate in the localization and definition of mechanism of ischemic stroke. J Cereb Blood Flow Metab. (1994) 1:S91–8.
128. Wintermark M, Sanelli PC, Albers GW, Bello JA, Derdeyn CP, Hetts SW, et al. Imaging recommendations for acute stroke and transient ischemic attack patients: A joint statement by the American Society of Neuroradiology, the American College of Radiology, and the Society of NeuroInterventional Surgery. AJNR Am J Neuroradiol. (2013) 34:E117–27. doi: 10.3174/ajnr.A3690
129. Alexandrov AV, Masdeu JC, Devous MD Sr, Black SE, Grotta JC. Brain single-photon emission CT with HMPAO and safety of thrombolytic therapy in acute ischemic stroke. Proceedings of the meeting of the SPECT Safe Thrombolysis Study Collaborators and the members of the Brain Imaging Council of the Society of Nuclear Medicine. Stroke. (1997) 28:1830–4. doi: 10.1161/01.STR.28.9.1830
130. Rizk H, Allam M, Hegazy A, Khalil H, Helmy H, Hashem HS, et al. Predictors of poor cerebral collaterals and cerebrovascular reserve in patients with chronic total carotid occlusion. Int J Neurosci. (2019) 129:455–60. doi: 10.1080/00207454.2018.1538990
131. Alexandrov AV, Ehrlich LE, Bladin CF, Black SE. Clinical significance of increased uptake of HMPAO on brain SPECT scans in acute stroke. J Neuroimaging. (1996) 6:150–5. doi: 10.1111/jon199663150
132. Thakkar MD, Qavi A, Singh AK, Maurya PK, Kulshreshtha D, Thacker AK, et al. Clinical value of perilesional perfusion deficit measured by Technetium-99m-ECD single-photon emission computed tomography in hypertensive intracerebral hemorrhage. World J Nucl Med. (2020) 19:246–54. doi: 10.4103/wjnm.WJNM_76_19
133. Okamoto K, Shiga H, Nakamura H, Matsui M, Miwa T. Relationship between olfactory disturbance after acute ischemic stroke and latent thalamic hypoperfusion. Chem Senses. (2020) 45:111–8. doi: 10.1093/chemse/bjz077
134. Sato K, Shimizu H, Inoue T, Fujimura M, Endo H, Tominaga T. Temporal and spatial changes in cerebral blood flow during management for preventing delayed cerebral ischemia after aneurysmal subarachnoid hemorrhage: serial semiquantitative analysis. J Stroke Cerebrovasc Dis. (2017) 26:2027–37. doi: 10.1016/j.jstrokecerebrovasdis.2017.06.006
135. Hashimoto H, Nakanishi R, Mizumura S, Hashimoto Y, Okamura Y, Yamanaka K, et al. Prognostic value of (99m)Tc-ECD brain perfusion SPECT in patients with atrial fibrillation and dementia. EJNMMI Res. (2020) 10:3. doi: 10.1186/s13550-019-0589-3
136. Hara T, Abo M, Kobayashi K, Watanabe M, Kakuda W, Senoo A. Effects of low-frequency repetitive transcranial magnetic stimulation combined with intensive speech therapy on cerebral blood flow in post-stroke aphasia. Transl Stroke Res. (2015) 6:365–74. doi: 10.1007/s12975-015-0417-7
137. Ward A, Carrico C, Powell E, Westgate PM, Nichols L, Fleischer A, et al. Safety and improvement of movement function after stroke with atomoxetine: A pilot randomized trial. Restor Neurol Neurosci. (2017) 35:1–10. doi: 10.3233/RNN-160673
138. Golan H, Makogon B, Volkov O, Smolyakov Y, Hadanny A, Efrati S. Imaging-based predictors for hyperbaric oxygen therapy outcome in post-stroke patients. Report 1. Med Hypotheses. (2020) 136:109510. doi: 10.1016/j.mehy.2019.109510
139. Kim Y, Lim SH, Park GY. Crossed cerebellar diaschisis has an adverse effect on functional outcome in the subacute rehabilitation phase of stroke: a case-control study. Arch Phys Med Rehabil. (2019) 100:1308–16. doi: 10.1016/j.apmr.2019.01.026
140. Wong TH, Shagera QA, Ryoo HG, Ha S, Lee DS. Basal and acetazolamide brain perfusion SPECT in internal carotid artery stenosis. Nucl Med Mol Imaging. (2020) 54:9–27. doi: 10.1007/s13139-019-00633-7
141. Matsuda H, Higashi S, Kinuya K, Tsuji S, Nozaki J, Sumiya H, et al. SPECT evaluation of brain perfusion reserve by the acetazolamide test using Tc-99m HMPAO. J Clin Nucl Med. (1991) 16:572–9. doi: 10.1097/00003072-199108000-00008
142. CDC. (2021). Available online at: https://www.cdc.gov/epilepsy/data/index.html. (accessed May 30, 2021).
143. Reddy SD, Younus I, Sridhar V, Reddy DS. Neuroimaging biomarkers of experimental epileptogenesis and refractory epilepsy. Int J Mol Sci. (2019) 20:220. doi: 10.3390/ijms20010220
144. Moreau JT, Saint-Martin C, Baillet S, Dudley RWR. MNI SISCOM: an open-source tool for computing subtraction ictal single-photon emission CT coregistered to MRI. J Digit Imaging. (2021) 34:357–61. doi: 10.1007/s10278-021-00422-9
145. Goffin K, van Laere K. Single-photon emission tomography. Handb Clin Neurol. (2016) 135:241–50. doi: 10.1016/B978-0-444-53485-9.00013-1
146. Lee BI, Markand ON, Wellman HN, Siddiqui AR, Park HM, Mock B, et al. HIPDM-SPECT in patients with medically intractable complex partial seizures. Ictal study Arch Neurol. (1988) 45:397–402. doi: 10.1001/archneur.1988.00520280043014
147. Rowe CC, Berkovic SF, Sia ST, Austin M, McKay WJ, Kalnins RM, et al. Localization of epileptic foci with postictal single photon emission computed tomography. Ann Neurol. (1989) 26:660–8. doi: 10.1002/ana.410260512
148. Stefan H, Bauer J, Feistel H, Schulemann H, Neubauer U, Wenzel B, et al. Regional cerebral blood flow during focal seizures of temporal and frontocentral onset. Ann Neurol. (1990) 27:162–6. doi: 10.1002/ana.410270211
149. Shen W, Lee BI, Park HM, Siddiqui AR, Wellman HH, Worth RM, et al. HIPDM-SPECT brain imaging in the presurgical evaluation of patients with intractable seizures. J Nucl Med. (1990) 31:1280–4.
150. Rowe CC, Berkovic SF, Austin MC, Saling M, Kalnins RM, McKay WJ, et al. Visual and quantitative analysis of interictal SPECT with technetium-99m-HMPAO in temporal lobe epilepsy. J Nucl Med. (1991) 32:1688–94.
151. Marks DA, Katz A, Hoffer P, Spencer SS. Localization of extratemporal epileptic foci during ictal single photon emission computed tomography. Ann Neurol. (1992) 31:250–5. doi: 10.1002/ana.410310304
152. Takeda T, Osawa M, Toi S, Mizuno S, Shimizu Y, Uchiyama S. Adversive seizures associated with periodic lateralised epileptiform discharges (PLEDs) after left orbital contusion. Epileptic Disord. (2012) 14:422–5. doi: 10.1684/epd.2012.0537
153. Tovar-Spinoza Z, Ziechmann R, Zyck S. Single and staged laser interstitial thermal therapy ablation for cortical tubers causing refractory epilepsy in pediatric patients. Neurosurg Focus. (2018) 45:E9. doi: 10.3171/2018.6.FOCUS18228
154. Volkan-Salanci B, Lay Ergün E, Genc Sel Ç, Yalnizoglu D, Turanli G. The role of brain perfusion SPECT in Moyamoya disease. Rev Esp Med Nucl Imagen Mol. (2012) 31:216–8. doi: 10.1016/j.remn.2012.02.006
155. Siasios I, Valotassiou V, Kapsalaki E, Tsougos I, Georgoulias P, Fotiadou A, et al. Magnetic resonance spectroscopy and single-photon emission computed tomography in the evaluation of cerebral tumors: a case report. J Clin Med Res. (2017) 9:74–8. doi: 10.14740/jocmr2775w
156. Tsuchiya H, Iwasaki Y, Shoda H, Takahashi Y, Fujio K. Limbic encephalitis in a patient with systemic lupus erythematosus successfully treated with high-dose glucocorticoids and intravenous cyclophosphamide therapy: the potential pathogenicity of anti-glutamate receptor antibodies. Mod Rheumatol Case Rep. (2021) 9:1–6. doi: 10.1080/24725625.2021.1876340
157. Barros P, Brito H, Ferreira PC, Ramalheira J, Lopes J, Rangel R, et al. Resective surgery in the treatment of super-refractory partial status epilepticus secondary to NMDAR antibody encephalitis. Eur J Paediatr Neurol. (2014) 18:449–52. doi: 10.1016/j.ejpn.2014.01.013
158. Kasahara H, Sato M, Nagamine S, Makioka K, Tanaka K, Ikeda Y. Temporal changes on (123)I-Iomazenil and cerebral blood flow single-photon emission computed tomography in a patient with anti-N-methyl-D-aspartate receptor encephalitis. Intern Med. (2019) 58:1501–5. doi: 10.2169/internalmedicine.0987-18
159. Kang KW, Kim SH, Kim JM, Nam TS, Choi KH, Kim MK. Ictal SPECT in diagnosis of non-ketotic hyperglycemia-related seizure manifesting as speech arrest. J Clin Neurol. (2019) 15:253–5. doi: 10.3988/jcn.2019.15.2.253
160. Tun MZ, Soo WK, Wu K, Kane R. Dementia with Lewy bodies presenting as probable epileptic seizure. BMJ Case Rep. (2017) 2017:bcr2017221454. doi: 10.1136/bcr-2017-221454
161. Chiron C. SPECT (single photon emission computed tomography) in pediatrics. Handb Clin Neurol. (2013) 111:759–65. doi: 10.1016/B978-0-444-52891-9.00078-6
162. Cho EB, Joo EY, Seo DW, Hong SC, Hong SB. Prognostic role of functional neuroimaging after multilobar resection in patients with localization-related epilepsy. PLoS ONE. (2015) 10:e0136565. doi: 10.1371/journal.pone.0136565
163. Jin P, Wu D, Li X, Ren L, Wang Y. Towards precision medicine in epilepsy surgery. Ann Transl Med. (2016) 4:24. doi: 10.3978/j.issn.2305-5839.2015.12.65
164. Jalota A, Rossi MA, Pylypyuk V, Stein M, Stoub T, Balabanov A, et al. Resecting critical nodes from an epileptogenic circuit in refractory focal-onset epilepsy patients using subtraction ictal SPECT coregistered to MRI. J Neurosurg. (2016) 125:1565–76. doi: 10.3171/2015.6.JNS141719
165. Tepmongkol S, Tangtrairattanakul K, Lerdlum S, Desudchit T. Comparison of brain perfusion SPECT parameters accuracy for seizure localization in extratemporal lobe epilepsy with discordant pre-surgical data. Ann Nucl Med. (2015) 29:21–8. doi: 10.1007/s12149-014-0905-y
166. Chen T, Guo L. The role of SISCOM in preoperative evaluation for patients with epilepsy surgery: a meta-analysis. Seizure. (2016) 41:43–50. doi: 10.1016/j.seizure.2016.06.024
167. Perissinotti A, Niñerola-Baizán A, Rubí S, Carreño M, Marti-Fuster B, Aparicio J, et al. PISCOM: a new procedure for epilepsy combining ictal SPECT and interictal PET. Eur J Nucl Med Mol Imaging. (2018) 45:2358–67. doi: 10.1007/s00259-018-4080-6
168. Jayalakshmi S, Nanda SK, Vooturi S, Vadapalli R, Sudhakar P, Madigubba S, et al. Focal cortical dysplasia and refractory epilepsy: role of multimodality imaging and outcome of surgery. AJNR Am J Neuroradiol. (2019) 40:892–8. doi: 10.3174/ajnr.A6041
169. Pawar SU, Ravat SH, Muzumdar DP, Sankhe SS, Chheda AH, Manglunia AS. Does Tc-99m ECD ictal brain SPECT have incremental value in localization of epileptogenic zone and predicting postoperative seizure freedom in cases with discordant video electroencephalogram and MRI findings? Nucl Med Commun. (2020) 41:858–70. doi: 10.1097/MNM.0000000000001240
170. Oliveira Young C, Etchbehere ECSC, Souza EM, Brunetto SQ, Santos AO, Lima MCL, et al. Clinical usefulness of SISCOM-SPM compared to visual analysis to locate the epileptogenic zone. Front Neurol. (2020) 11:467. doi: 10.3389/fneur.2020.00467
171. Kaur K, Garg A, Tripathi M, Chandra SP, Singh G, Viswanathan V, et al. Comparative contribution of magnetoencephalography (MEG) and single-photon emission computed tomography (SPECT) in pre-operative localization for epilepsy surgery: A prospective blinded study. Seizure. (2021) 86:181–8. doi: 10.1016/j.seizure.2021.02.005
172. Yassin A, El-Salem K, Al-Mistarehi AH, Momani A, Zein Alaabdin AM, Shah P, et al. Use of innovative SPECT techniques in the presurgical evaluation of patients with nonlesional extratemporal drug-resistant epilepsy. Mol Imaging. (2021) 2021:6614356. doi: 10.1155/2021/6614356
173. Lee JJ, Lee SK, Choi JW, Kim DW, Park KI, Kim BS, et al. Ictal SPECT using an attachable automated injector: clinical usefulness in the prediction of ictal onset zone. Acta Radiol. (2009) 50:1160–8. doi: 10.3109/02841850903215926
174. Kim S, Holder DL, Laymon CM, Tudorascu DL, Deeb EL, Panigrahy A, et al. Clinical value of the first dedicated, commercially available automatic injector for ictal brain SPECT in presurgical evaluation of pediatric epilepsy: comparison with manual injection. J Nucl Med. (2013) 54:732–8. doi: 10.2967/jnumed.112.105189
175. Yassin A, Al-Mistarehi AH, El-Salem K, Urban A, Plummer C, Mohammadi S, et al. Effect of automatic injectors on the injection latency, safety, and seizure onset zone localization of ictal single photon emission computed tomography studies in adult epilepsy monitoring unit. Epilepsy Res. (2021) 169:106522. doi: 10.1016/j.eplepsyres.2020.106522
176. Stamoulis C, Connolly J, Axeen E, Kaulas H, Bolton J, Dorfman K, et al. Non-invasive seizure localization with ictal single-photon emission computed tomography is impacted by preictal/early ictal network dynamics. IEEE Trans Biomed Eng. (2018) 66:1863–71. doi: 10.1109/TBME.2018.2880575
177. Foiadelli T, Lagae L, Goffin K, Theys T, De Amici M, Sacchi L, et al. Subtraction ictal SPECT coregistered to MRI (SISCOM) as a guide in localizing childhood epilepsy. Epilepsia Open. (2019) 5:61–72. doi: 10.1002/epi4.12373
178. Jaraba S, Reynés-Llompart G, Sala-Padró J, Veciana M, Miró J, Pedro J, et al. Usefulness of HMPAO-SPECT in the diagnosis of nonconvulsive status epilepticus. Epilepsy Behav. (2019) 101:106544. doi: 10.1016/j.yebeh.2019.106544
179. Bonte FJ, Ross ED, Chehabi HH, Devous MD Sr. SPECT study of regional cerebral blood flow in Alzheimer disease. J Comput Assist Tomogr. (1986) 10:579–83. doi: 10.1097/00004728-198607000-00005
180. Komatani A, Yamaguchi K, Sugai Y, Takanashi T, Kera M, Shinohara M, et al. Assessment of demented patients by dynamic SPECT of inhaled xenon-133. J Nucl Med. (1988) 29:1621–6.
181. Gemmell HG, Sharp PF, Smith FW, Besson JA, Ebmeier KP, Davidson J, et al. Cerebral blood flow measured by SPECT as a diagnostic tool in the study of dementia. Psychiatry Res. (1989) 29:327–9. doi: 10.1016/0165-1781(89)90079-6
182. Montaldi D, Brooks DN, McColl JH, Wyper D, Patterson J, Barron E, et al. Measurements of regional cerebral blood flow and cognitive performance in Alzheimer's disease. J Neurol Neurosurg Psychiatry. (1990) 53:33–8. doi: 10.1136/jnnp.53.1.33
183. Holman BL, Johnson KA, Gerada B, Carvalho PA, Satlin A. The scintigraphic appearance of Alzheimer's disease: a prospective study using technetium-99m-HMPAO SPECT. J Nucl Med. (1992) 33:181–5.
184. Jobst KA, Smith AD, Barker CS, Wear A, King EM, Smith A, et al. Association of atrophy of the medial temporal lobe with reduced blood flow in the posterior parietotemporal cortex in patients with a clinical and pathological diagnosis of Alzheimer's disease. J Neurol Neurosurg Psychiatry. (1992) 55:190–4. doi: 10.1136/jnnp.55.3.190
185. Bonte FJ, Tintner R, Weiner MF, Bigio EH, White CL 3rd. Brain blood flow in the dementias: SPECT with histopathologic correlation. Radiol. (1993) 186:361–5. doi: 10.1148/radiology.186.2.8421735
186. Bonte FJ, Weiner MF, Bigio EH, White CL. Brain blood flow in the dementias: SPECT with histopathologic correlation in 54 patients. Radiology. (1997) 202:793–7. doi: 10.1148/radiology.202.3.9051035
187. Jagust W, Thisted R, Devous MD Sr, Van Heertum R, Mayberg H, et al. SPECT perfusion imaging in the diagnosis of Alzheimer's disease: a clinical-pathologic study. Neurology. (2001) 56:950–6. doi: 10.1212/WNL.56.7.950
188. Silverman DH. Brain 18F-FDG PET in the diagnosis of neurodegenerative dementias: comparison with perfusion SPECT and with clinical evaluations lacking nuclear imaging. J Nucl Med. (2004) 45:594–607.
189. Pavel D, Devore-Best S, Craita I. Routine use of high-resolution brain SPECT and multiple display modes for dementia differential and follow-up. In: 9th International Conference on Alzheimer's Disease and Related Disorders. Philadelphia, PA (2004).
190. Mistur R, Mosconi L, Santi SD, Guzman M, Li Y, Tsui W, et al. Current challenges for the early detection of Alzheimer's disease: brain imaging and CSF studies. J Clin Neurol. (2009) 5:153–66. doi: 10.3988/jcn.2009.5.4.153
191. Henderson TA. The diagnosis and evaluation of dementia and mild cognitive impairment with emphasis on SPECT perfusion neuroimaging. CNS Spectr. (2012) 17:176–206. doi: 10.1017/S1092852912000636
192. Battistin L, Pizzolato G, Dam M, Ponza I, Borsato N, Zanco PL, et al. Regional cerebral blood flow study with 99mTc-hexamethyl-propyleneamine oxime single photon emission computed tomography in Alzheimer's and multi-infarct dementia. Eur Neurol. (1990) 30:296–301. doi: 10.1159/000117384
193. Knapp WH, Dannenberg C, Marschall B, Zedlick D, Löschmann K, Bettin S, et al. Changes in local cerebral blood flow by neuroactivation and vasoactivation in patients with impaired cognitive function. Eur J Nucl Med. (1996) 23:878–88. doi: 10.1007/BF01084360
194. Mattman A, Feldman H, Forster B, Li D, Szasz I, Beattie BL, et al. Regional HmPAO SPECT and CT measurements in the diagnosis of Alzheimer's disease. Can J Neurol Sci. (1997) 24:22–8. doi: 10.1017/S0317167100021041
195. Dougall NJ, Bruggink S, Ebmeier KP. Systematic review of the diagnostic accuracy of 99mTc-HMPAO-SPECT in dementia. Am J Geriatr Psychiatry. (2004) 12:554–70. doi: 10.1097/00019442-200411000-00002
196. Yuan Y, Gu ZX, Wei WS. Fluorodeoxyglucose-positron-emission tomography, single-photon emission tomography, and structural MR imaging for prediction of rapid conversion to Alzheimer disease in patients with mild cognitive impairment: a meta-analysis. AJNR Am J Neuroradiol. (2009) 30:404–10. doi: 10.3174/ajnr.A1357
197. Bloudek LM, Spackman DE, Blankenburg M, Sullivan SD. Review and meta-analysis of biomarkers and diagnostic imaging in Alzheimer's disease. J Alzheimer's Dis. (2011) 26:627–45. doi: 10.3233/JAD-2011-110458
198. Frisoni GB, Bocchetta M, Chételat G, Rabinovici GD, de Leon MJ, Kaye J, et al. ISTAART's neuroimaging professional interest area. Imaging markers for Alzheimer disease: which vs how. Neurology. (2013) 81:487–500. doi: 10.1212/WNL.0b013e31829d86e8
199. Davison CM, O'Brien JT. A comparison of FDG-PET and blood flow SPECT in the diagnosis of neurodegenerative dementias: a systematic review. Int J Geriatr Psychiatry. (2014) 229:551–61. doi: 10.1002/gps.4036
200. Hanyu H, Abe S, Arai H, Asano T, Iwamoto T, Takasaki M. Diagnostic accuracy of single photon emission computed tomography in Alzheimer's disease. Gerontology. (1993) 39:260–6. doi: 10.1159/000213541
201. Bonte FJ, Harris TS, Hynan LS, Bigio EH, White CL. Tc-99m HMPAO SPECT in the differential diagnosis of the dementias with histopathologic confirmation. Clin Nucl Med. (2006) 31:376–8. doi: 10.1097/01.rlu.0000222736.81365.63
202. Perani D, Di Piero V, Vallar G, Cappa S, Messa C, Bottini G, et al. Technetium-99m HM-PAO-SPECT study of regional cerebral perfusion in early Alzheimer's disease. J Nucl Med. (1988) 29:1507–14.
203. Leys D, Steinling M, Petit H, Salomez JL, Gaudet Y, Ovelacq E, et al. [Alzheimer's disease: study by single photon emission tomography (Hm PAO Tc99m)]. Rev Neurol. (1989) 145:443–50.
204. Hurwitz TA, Ammann W, Chu D, Clark C, Holden J, Brownstone R. Single photon emission computed tomography using 99mTc-HM-PAO in the routine evaluation of Alzheimer's disease. Can J Neurol Sci. (1991) 18:59–62. doi: 10.1017/S0317167100031292
205. O'Brien JT, Eagger S, Syed GM, Sahakian BJ, Levy R. A study of regional cerebral blood flow and cognitive performance in Alzheimer's disease. J Neurol Neurosurg Psychiatry. (1992) 55:1182–7. doi: 10.1136/jnnp.55.12.1182
206. Claus JJ, van Harskamp F, Breteler MM, Krenning EP, van der Cammen TJ, Hofman A, et al. Assessment of cerebral perfusion with single-photon emission tomography in normal subjects and in patients with Alzheimer's disease: effects of region of interest selection. Eur J Nucl Med. (1994) 21:1044–51. doi: 10.1007/BF00181058
207. O'Mahony D, Coffey J, Murphy J, O'Hare N, Hamilton D, Freyne P, et al. The discriminant value of semiquantitative SPECT data in mild Alzheimer's disease. J Nucl Med. (1994) 35:1450–5.
208. Hellman RS, Tikofsky RS, Van Heertum R, Coade G, Carretta R, Hoffmann RG. A multi-institutional study of interobserver agreement in the evaluation of dementia with rCBF/SPET technetium-99m exametazime (HMPAO). Eur J Nucl Med. (1994) 21:306–13. doi: 10.1007/BF00947965
209. Sloan EP, Fenton GW, Kennedy NS, MacLennan JM. Electroencephalography and single photon emission computed tomography in dementia: a comparative study. Psychol Med. (1995) 25:631–8. doi: 10.1017/S0033291700033535
210. Jobst KA, Barnetson LP, Shepstone BJ. Accurate prediction of histologically confirmed Alzheimer's disease and the differential diagnosis of dementia: the use of NINCDS-ADRDA and DSM-III-R criteria, SPECT, X-ray CT, and Apo E4 in medial temporal lobe dementias. Oxford project to investigate memory and aging. Int Psychogeriatr. (1998) 10:271–302. doi: 10.1017/S1041610298005389
211. O'Brien JT, Ames D, Desmond P, Lichtenstein M, Binns D, Schweitzer I, et al. Combined magnetic resonance imaging and single-photon emission tomography scanning in the discrimination of Alzheimer's disease from age-matched controls. Int Psychogeriatr. (2001) 13:149–61. doi: 10.1017/S1041610201007554
212. Johnson KA, Kijewski MF, Becker JA, Garada B, Satlin A, Holman BL. Quantitative brain SPECT in Alzheimer's disease and normal aging. J Nucl Med. (1993) 34:2044–8.
213. Waldemar G, Bruhn P, Kristensen M, Johnsen A, Paulson OB, Lassen NA. Heterogeneity of neocortical cerebral blood flow deficits in dementia of the Alzheimer type: a [99mTc]-d,l-HMPAO SPECT study. J Neurol Neurosurg Psychiatry. (1994) 57:285–95. doi: 10.1136/jnnp.57.3.285
214. Karbe H, Kertesz A, Davis J, Kemp BJ, Prato FS, Nicholson RL. Quantification of functional deficit in Alzheimer's disease using a computer-assisted mapping program for 99mTc-HMPAO SPECT. Neuroradiology. (1994) 36:1–6. doi: 10.1007/BF00599183
215. Messa C, Perani D, Lucignani G, Zenorini A, Zito F, Rizzo G, et al. High-resolution technetium-99m-HMPAO SPECT in patients with probable Alzheimer's disease: comparison with fluorine-18-FDG PET. J Nucl Med. (1994) 35:210–6.
216. Ichise M, Crisp S, Ganguli N, Tsai S, Gray BG. A method of two-dimensional mapping of cortical perfusion by cylindrical transformation of HMPAO SPET data. Nucl Med Commun. (1995) 16:386–94. doi: 10.1097/00006231-199505000-00011
217. Hashikawa K, Matsumoto M, Moriwaki H, Oku N, Okazaki Y, Seike Y, et al. Three-dimensional display of surface cortical perfusion by SPECT: application in assessing Alzheimer's disease. J Nucl Med. (1995) 36:690–6.
218. deFigueiredo RJ, Shankle WR, Maccato A, Dick MB, Mundkur P, Mena I, et al. Neural-network-based classification of cognitively normal, demented, Alzheimer disease and vascular dementia from single photon emission with computed tomography image data from brain. Proc Natl Acad Sci USA. (1995) 92:5530–4. doi: 10.1073/pnas.92.12.5530
219. van Dyck CH, Lin CH, Smith EO, Wisniewski G, Cellar J, Robinson R, et al. Comparison of technetium-99m-HMPAO and technetium-99m-ECD cerebral SPECT images in Alzheimer's disease. J Nucl Med. (1996) 37:1749–55.
220. Bartenstein P, Minoshima S, Hirsch C, Buch K, Willoch F, Mösch D, et al. Quantitative assessment of cerebral blood flow in patients with Alzheimer's disease by SPECT. J Nucl Med. (1997) 38:1095–101.
221. Johnson KA, Jones K, Holman BL, Becker JA, Spiers PA, Satlin A, et al. Preclinical prediction of Alzheimer's disease using SPECT. Neurology. (1998) 50:1563–71. doi: 10.1212/WNL.50.6.1563
222. Honda N, Machida K, Hosono M, Matsumoto T, Matsuda H, Oshima M, et al. Interobserver variation in diagnosis of dementia by brain perfusion SPECT. Radiat Med. (2002) 20:281–9.
223. Soonawala D, Amin T, Ebmeier KP, Steele JD, Dougall NJ, Best J, et al. Statistical parametric mapping of (99m)Tc-HMPAO-SPECT images for the diagnosis of Alzheimer's disease: normalizing to cerebellar tracer uptake. Neuroimage. (2002) 17:1193–202. doi: 10.1006/nimg.2002.1259
224. Elgh E, Sundström T, Näsman B, Ahlström R, Nyberg L. Memory functions and rCBF (99m)Tc-HMPAO SPET: developing diagnostics in Alzheimer's disease. Eur J Nucl Med Mol Imaging. (2002) 29:1140–8. doi: 10.1007/s00259-002-0829-y
225. Honda N, Machida K, Matsumoto T, Matsuda H, Imabayashi E, Hashimoto J, et al. Three-dimensional stereotactic surface projection of brain perfusion SPECT improves diagnosis of Alzheimer's disease. Ann Nucl Med. (2003) 17:641–8. doi: 10.1007/BF02984969
226. Kubota T, Ushijima Y, Yamada K, Okuyama C, Kizu O, Nishimura T. Diagnosis of Alzheimer's disease using brain perfusion SPECT and MR imaging: which modality achieves better diagnostic accuracy? Eur J Nucl Med Mol Imaging. (2005) 32:414–21. doi: 10.1007/s00259-004-1704-9
227. Kemp PM, Hoffmann SA, Holmes C, Bolt L, Ward T, Holmes RB, et al. The contribution of statistical parametric mapping in the assessment of precuneal and medial temporal lobe perfusion by 99mTc-HMPAO SPECT in mild Alzheimer's and Lewy body dementia. Nucl Med Commun. (2005) 26:1099–106. doi: 10.1097/00006231-200512000-00009
228. Chaves R, Ramírez J, Górriz JM, López M, Salas-Gonzalez D, Alvarez I, et al. SVM-based computer-aided diagnosis of the Alzheimer's disease using t-test NMSE feature selection with feature correlation weighting. Neurosci Lett. (2009) 461:293–7. doi: 10.1016/j.neulet.2009.06.052
229. Pagani M, Salmaso D, Rodriguez G, Nardo D, Nobili F. Principal component analysis in mild and moderate Alzheimer's disease–a novel approach to clinical diagnosis. Psychiatry Res. (2009) 173:8–14. doi: 10.1016/j.pscychresns.2008.07.016
230. Ishii S, Shishido F, Miyajima M, Sakuma K, Shigihara T, Tameta T, et al. Comparison of Alzheimer's disease with vascular dementia and non-dementia using specific voxel-based Z score maps. Ann Nucl Med. (2009) 23:25–31. doi: 10.1007/s12149-008-0210-8
231. Rusina R, Kukal J, Belícek T, Buncová M, Matej R. Use of fuzzy edge single-photon emission computed tomography analysis in definite Alzheimer's disease–a retrospective study. BMC Med Imaging. (2010) 10:20. doi: 10.1186/1471-2342-10-20
232. Matsuda H, Mizumura S, Nagao T, Ota T, Iizuka T, Nemoto K, et al. Automated discrimination between very early Alzheimer disease and controls using an easy Z-score imaging system for multicenter brain perfusion single-photon emission tomography. AJNR Am J Neuroradiol. (2007) 28:731–6. doi: 10.1097/MNM.0b013e328013eb8b
233. Wu CC, Mungas D, Eberling JL, Reed BR, Jagust WJ. Imaging interactions between Alzheimer's disease and cerebrovascular disease. Ann N Y Acad Sci. (2002) 977:403–10. doi: 10.1111/j.1749-6632.2002.tb04844.x
234. Ravona-Springer R, Davidson M, Noy S. The role of cardiovascular risk factors in Alzheimer's disease. CNS Spectr. (2003) 8:824–33. doi: 10.1017/S109285290001926X
235. Hanyu H, Shimuzu S, Tanaka Y, Takasaki M, Koizumi K, Abe K. Cerebral blood flow patterns in Binswanger's disease: a SPECT study using three-dimensional stereotactic surface projections. J Neurol Sci. (2004) 220:79–84. doi: 10.1016/j.jns.2004.02.011
236. Launes J, Sulkava R, Erkinjuntti T, Nikkinen P, Lindroth L, Liewendahl K, et al. 99Tcm-HMPAO SPECT in suspected dementia. Nucl Med Commun. (1991) 12:757–65. doi: 10.1097/00006231-199109000-00002
237. Houston AS, Kemp PM, Macleod MA. A method for assessing the significance of abnormalities in HMPO brain SPECT images. J Nucl Med. (1994) 35:239–44.
238. Mielke R, Pietrzyk U, Jacobs A, Fink GR, Ichimiya A, Kessler J, et al. HMPAO SPET and FDG PET in Alzheimer's disease and vascular dementia: comparison of perfusion and metabolic pattern. Eur J Nucl Med. (1994) 21:1052–60. doi: 10.1007/BF00181059
239. Bergman H, Chertkow H, Wolfson C, Stern J, Rush C, Whitehead V, et al. HM-PAO (CERETEC) SPECT brain scanning in the diagnosis of Alzheimer's disease. J Am Geriatr Soc. (1997) 45:15–20. doi: 10.1111/j.1532-5415.1997.tb00972.x
240. Pávics L, Grünwald F, Reichmann K, Horn R, Kitschenberg A, Hartmann A, et al. Regional cerebral blood flow single-photon emission tomography with 99mTc-HMPAO and the acetazolamide test in the evaluation of vascular and Alzheimer's dementia. Eur J Nucl Med. (1999) 26:239–45. doi: 10.1007/s002590050383
241. Butler RE, Costa DC, Greco A, Ell PJ, Katona CLE. Differentiation between Alzheimer's disease and multi-infarct dementia: SPECT vs MR imaging. Int J Geriatr Psychiatry. (1995) 10:121–8. doi: 10.1002/gps.930100207
242. Uchida Y, Minoshima S, Okada S, Kawata T, Ito H. Diagnosis of dementia using perfusion SPECT imaging at the patient's initial visit to a cognitive disorder clinic. Clin Nucl Med. (2006) 31:764–73. doi: 10.1097/01.rlu.0000246818.24566.54
243. Talbot PR, Goulding PJ, Lloyd JJ, Snowden JS, Neary D, Testa HJ. Inter-relation between “classic” motor neuron disease and frontotemporal dementia: neuropsychological and single photon emission computed tomography study. J Neurol Neurosurg Psychiatry. (1995) 58:541–7. doi: 10.1136/jnnp.58.5.541
244. Talbot PR, Lloyd JJ, Snowden JS, Neary D, Testa HJ. A clinical role for 99mTc-HMPAO SPECT in the investigation of dementia? J Neurol Neurosurg Psychiatry. (1998) 64:306–13. doi: 10.1136/jnnp.64.3.306
245. Small GW. What does imaging add to the management of Alzheimer's disease? CNS Spectr. (2004) 9:20–3. doi: 10.1017/S1092852900024779
246. Tranfaglia C, Palumbo B, Siepi D, Sinzinger H, Parnetti L. Semi-quantitative analysis of perfusion of Brodmann areas in the differential diagnosis of cognitive impairment in Alzheimer's disease, fronto-temporal dementia and mild cognitive impairment. Hell J Nucl Med. (2009) 12:110–4.
247. Van Heertum RL. Dementia: diagnosis of dementia. In: Van Heertum RL, Tikofsky RS, Ichise M, editors. Functional Cerebral SPECT and PET Imaging, 4th ed. Philadelphia, PA: Lippincott Williams & Wilkins. (2010) p. 81–95.
248. Devous MD. Functional brain imaging in the dementias: role in early detection, differential diagnosis, and longitudinal studies. Eur J Nucl Med Mol Imaging. (2002) 29:1685–96. doi: 10.1007/s00259-002-0967-2
249. Horn JF, Habert MO, Kas A, Malek Z, Maksud P, Lacomblez L, et al. Differential automatic diagnosis between Alzheimer's disease and frontotemporal dementia based on perfusion SPECT images. Artif Intell Med. (2009) 47:147–58. doi: 10.1016/j.artmed.2009.05.001
250. Neary D, Snowden JS, Shields RA, Burjan AW, Northen B, MacDermott N, et al. Single photon emission tomography using 99mTc-HM-PAO in the investigation of dementia. J Neurol Neurosurg Psychiatry. (1987) 50:1101–9. doi: 10.1136/jnnp.50.9.1101
251. Testa HJ, Snowden JS, Neary D, Shields RA, Burjan AW, Prescott MC, et al. The use of [99mTc]-HM-PAO in the diagnosis of primary degenerative dementia. J Cereb Blood Flow Metab. (1988) 8:S123–6. doi: 10.1038/jcbfm.1988.42
252. McNeill R, Sare GM, Manoharan M, Testa HJ, Mann DM, Neary D, et al. Accuracy of single-photon emission computed tomography in differentiating frontotemporal dementia from Alzheimer's disease. J Neurol Neurosurg Psychiatry. (2007) 78:350–5. doi: 10.1136/jnnp.2006.106054
253. Charpentier P, Lavenu I, Defebvre L, Duhamel A, Lecouffe P, Pasquier F, et al. Alzheimer's disease and frontotemporal dementia are differentiated by discriminant analysis applied to (99m)Tc HmPAO SPECT data. J Neurol Neurosurg Psychiatry. (2000) 69:661–3. doi: 10.1136/jnnp.69.5.661
254. Shimizu S, Hanyu H, Kanetaka H, Iwamoto T, Koizumi K, Abe K. Differentiation of dementia with Lewy bodies from Alzheimer's disease using brain SPECT. Dement Geriatr Cogn Disord. (2005) 20:25–30. doi: 10.1159/000085070
255. Sato T, Hanyu H, Hirao K, Shimizu S, Kanetaka H, Iwamoto T. Deep gray matter hyperperfusion with occipital hypoperfusion in dementia with Lewy bodies. T Eur J Neurol. (2007) 14:1299–301. doi: 10.1111/j.1468-1331.2007.01951.x
256. Goto H, Ishii K, Uemura T, Miyamoto N, Yoshikawa T, Shimada K, et al. Differential diagnosis of dementia with Lewy Bodies and Alzheimer Disease using combined MR imaging and brain perfusion single-photon emission tomography. AJNR Am J Neuroradiol. (2010) 31:720–5. doi: 10.3174/ajnr.A1926
257. Johnson KA, Moran EK, Becker JA, Blacker D, Fischman AJ, Albert MS. Single photon emission computed tomography perfusion differences in mild cognitive impairment. J Neurol Neurosurg Psychiatry. (2007) 78:240–7. doi: 10.1136/jnnp.2006.096800
258. Kogure D, Matsuda H, Ohnishi T, Asada T, Uno M, Kunihiro T, et al. Longitudinal evaluation of early Alzheimer's disease using brain perfusion SPECT. J Nucl Med. (2000) 41:1155–62.
259. Huang C, Wahlund LO, Svensson L, Winblad B, Julin P. Cingulate cortex hypoperfusion predicts Alzheimer's disease in mild cognitive impairment. BMC Neurol. (2002) 2:9. doi: 10.1186/1471-2377-2-9
260. Encinas M, De Juan R, Marcos A, Gil P, Barabash A, Fernández C, et al. Regional cerebral blood flow assessed with 99mTc-ECD SPET as a marker of progression of mild cognitive impairment to Alzheimer's disease. Eur J Nucl Med Mol Imaging. (2003) 30:1473–80. doi: 10.1007/s00259-003-1277-z
261. Borroni B, Anchisi D, Paghera B, Vicini B, Kerrouche N, Garibotto et al. Combined 99mTc-ECD SPECT and neuropsychological studies in MCI for the assessment of conversion to AD. Neurobiol Aging. (2006) 27:24–31. doi: 10.1016/j.neurobiolaging.2004.12.010
262. Ishiwata A, Sakayori O, Minoshima S, Mizumura S, Kitamura S, Katayama Y. Preclinical evidence of Alzheimer changes in progressive mild cognitive impairment: a qualitative and quantitative SPECT study. Acta Neurol Scand. (2006) 114:91–6. doi: 10.1111/j.1600-0404.2006.00661.x
263. Caroli A, Testa C, Geroldi C, Nobili F, Barnden LR, Guerra UP, et al. Cerebral perfusion correlates of conversion to Alzheimer's disease in amnestic mild cognitive impairment. J Neurol. (2007) 254:1698–707. doi: 10.1007/s00415-007-0631-7
264. Nobili F, De Carli F, Frisoni GB, Portet F, Verhey F, Rodriguez G, et al. SPECT predictors of cognitive decline and Alzheimer's disease in mild cognitive impairment. J Alzheimer's Dis. (2009) 17:761–72. doi: 10.3233/JAD-2009-1091
265. Edman A, Edenbrandt L, Fredén-Lindqvist J, Nilsson M, Wallin A. Asymmetric cerebral blood flow in patients with mild cognitive impairment: possible relationship to further cognitive deterioration. Dement Geriatr Cogn Dis Extra. (2011) 1:228–36. doi: 10.1159/000329447
266. Habert MO, Horn JF, Sarazin M, Lotterie JA, Puel M, Onen F, et al. Brain perfusion SPECT with an automated quantitative tool can identify prodromal Alzheimer's disease among patients with mild cognitive impairment. Neurobiol Aging. (2011) 32:15–23. doi: 10.1016/j.neurobiolaging.2009.01.013
267. Petersen RC, Stevens JC, Ganguli M, Tangalos EG, Cummings JL, DeKosky ST. Practice parameter: early detection of dementia: mild cognitive impairment (an evidence-based review). Report of the Quality Standards Subcommittee of the American Academy of Neurology. Neurology. (2001) 56:1133–42. doi: 10.1212/WNL.56.9.1133
268. Hirao K, Ohnishi T, Hirata Y, Yamashita F, Mori T, Moriguchi Y, et al. The prediction of rapid conversion to Alzheimer's disease in mild cognitive impairment using regional cerebral blood flow SPECT. Neuroimage. (2005) 28:1014–21. doi: 10.1016/j.neuroimage.2005.06.066
269. Herholz K, Schopphoff H, Schmidt M, Mielke R, Eschner W, Scheidhauer K, et al. Direct comparison of spatially normalized PET and SPECT scans in Alzheimer's disease. J Nucl Med. (2002) 43:21–6.
270. Pimlott SL, Ebmeier KP. SPECT imaging in dementia. Br J Radiol. (2007) 80:S153–9. doi: 10.1259/bjr/89285735
271. Weih M, Degirmenci U, Kreil S, Lewczuk P, Schmidt D, Kornhuber J, et al. Perfusion imaging with SPECT in the era of pathophysiology-based biomarkers for Alzheimer's disease. Int J Alzheimer's Dis. (2010) 2010:109618. doi: 10.4061/2010/109618
272. Ito K, Fukuyama H, Senda M, Ishii K, Maeda K, Yamamoto Y, et al. Prediction of Outcomes in Mild Cognitive Impairment by Using 18F-FDG-PET: A Multicenter Study. J Alzheimers Dis. (2015) 45:543–52. doi: 10.3233/JAD-141338
273. Inui Y, Ito K, Kato T. Longer-term investigation of the value of 18F-FDG-PET and magnetic resonance imaging for predicting the conversion of mild cognitive impairment to Alzheimer's disease: A multicenter study. J Alzheimers Dis. (2017) 60:877–87. doi: 10.3233/JAD-170395
274. Sanchez-Catasus CA, Stormezand GN, van Laar PJ, De Deyn PP, Sanchez MA, Dierckx RA. FDG-PET for prediction of AD dementia in mild cognitive impairment. A review of the state of the art with particular emphasis on the comparison with other neuroimaging modalities (MRI and Perfusion SPECT). Curr Alzheimer Res. (2017) 14:127–42. doi: 10.2174/1567205013666160629081956
275. Rowe CC, Ng S, Ackermann U, Gong SJ, Pike K, Savage G, et al. Imaging beta-amyloid burden in aging and dementia. Neurology. (2007) 68:1718–25. doi: 10.1212/01.wnl.0000261919.22630.ea
276. Rodrigue KM, Kennedy KM, Park DC. Beta-amyloid deposition and the aging brain. Neuropsychol Rev. (2009) 19:436–50. doi: 10.1007/s11065-009-9118-x
277. Martínez G, Vernooij RW, Fuentes Padilla P, Zamora J, Bonfill Cosp X, Flicker L. 18F PET with florbetapir for the early diagnosis of Alzheimer's disease dementia and other dementias in people with mild cognitive impairment (MCI). Cochrane Database Syst Rev. (2017) 11:CD012216. doi: 10.1002/14651858.CD012216.pub2
278. Hagstadius S, Orbaek P, Risberg J, Lindgren M. Regional cerebral blood flow at the time of diagnosis of chronic toxic encephalopathy induced by organic-solvent exposure and after the cessation of exposure. Scand J Work Environ Health. (1989) 15:130–5. doi: 10.5271/sjweh.1872
279. Callender TJ, Morrow L, Subramanian K, Duhon D, Ristovv M. Three-dimensional brain metabolic imaging in patients with toxic encephalopathy. Environ Res. (1993) 60:295–319. doi: 10.1006/enrs.1993.1039
280. Visser I, Lavini C, Booij J, Reneman L, Majoie C, de Boer AG, et al. Cerebral impairment in chronic solvent-induced encephalopathy. Ann Neurol. (2008) 63:572–80. doi: 10.1002/ana.21364
281. Geier DA, Pretorius HT, Richards NM, Geier MR. A quantitative evaluation of brain dysfunction and body-burden of toxic metals. Med Sci Monit. (2012) 18:CR425–431. doi: 10.12659/MSM.883210
282. Rea WJ, Didriksen N, Simon TR, Pan Y, Fenyves EJ, Griffiths B. Effects of toxic exposure to molds and mycotoxins in building-related illnesses. Arch Environ Health. (2003) 58:399–405. doi: 10.1080/00039896.2003.11879140
283. Véra P, Rohrlich P, Stiévenart JL, Elmaleh M, Duval M, Bonnin F, et al. Contribution of single-photon emission computed tomography in the diagnosis and follow-up of CNS toxicity of a cytarabine-containing regimen in pediatric leukemia. J Clin Oncol. (1999) 17:2804–10. doi: 10.1200/JCO.1999.17.9.2804
284. Küçük NO, Kiliç EO, Ibis E, Aysev A, Gençoglu EA, Aras G, et al. Brain SPECT findings in long-term inhalant abuse. Nucl Med Commun. (2000) 21:769–73. doi: 10.1097/00006231-200008000-00011
285. Holman BL, Carvalho PA, Mendelson J, Teoh SK, Nardin R, Hallgring E, et al. Brain perfusion is abnormal in cocaine-dependent polydrug users: a study using technetium-99m-HMPAO and ASPECT. J Nucl Med. (1991) 32:1206–10.
286. Devous MD. Comparison of SPECT applications in neurology and psychiatry. J Clin Psychiatry. (1992) 53:13–9.
287. Miller BL, Mena I, Giombetti R, Villanueva-Meyer J, Djenderedjian AH. Neuropsychiatric effects of cocaine: SPECT measurements. J Addict Dis. (1992) 11:47–58. doi: 10.1300/J069v11n04_04
288. Holman BL, Mendelson J, Garada B, Teoh SK, Hallgring E, Johnson KA, et al. Regional cerebral blood flow improves with treatment in chronic cocaine polydrug users. J Nucl Med. (1993) 34:723–7.
289. Kuruoglu AC, Arikan Z, Vural G, Karataş M, Araç M, Işik E. Single photon emission computerised tomography in chronic alcoholism. Antisocial personality disorder may be associated with decreased frontal perfusion. Br J Psychiatry. (1996) 169:348–54. doi: 10.1192/bjp.169.3.348
290. Noël X, Paternot J, Van der Linden M, Sferrazza R, Verhas M, Hanak C, et al. Correlation between inhibition, working memory and delimited frontal area blood flow measure by 99mTc-Bicisate SPECT in alcohol-dependent patients. Alcohol Alcohol. (2001) 36:556–63. doi: 10.1093/alcalc/36.6.556
291. Ohta H, Matsumoto R, Kato T, Tomono N, Shimizu T. Tc-99m HMPAO brain perfusion SPECT images in a patient with portal-systemic encephalopathy. Clin Nucl Med. (1998) 23:634–6. doi: 10.1097/00003072-199809000-00025
292. Catafau AM, Kulisevsky J, Bernà L, Pujol J, Martin JC, Otermin P, et al. Relationship between cerebral perfusion in frontal-limbic-basal ganglia circuits and neuropsychologic impairment in patients with subclinical hepatic encephalopathy. J Nucl Med. (2000) 41:405–10.
293. Iwasa M, Matsumura K, Kaito M, Ikoma J, Kobayashi Y, Nakagawa N, et al. Decrease of regional cerebral blood flow in liver cirrhosis. Eur J Gastroenterol Hepatol. (2000) 12:1001–6. doi: 10.1097/00042737-200012090-00006
294. Nakagawa Y, Matsumura K, Iwasa M, Kaito M, Adachi Y, Takeda K. Single photon emission computed tomography and statistical parametric mapping analysis in cirrhotic patients with and without minimal hepatic encephalopathy. Ann Nucl Med. (2004) 18:123–9. doi: 10.1007/BF02985102
295. Romeiro FG, Ietsugu MDV, Franzoni LC, Augusti L, Alvarez M, Santos LAA, et al. Which of the branched-chain amino acids increases cerebral blood flow in hepatic encephalopathy? A double-blind randomized trial. Neuroimage Clin. (2018) 19:302–10. doi: 10.1016/j.nicl.2018.03.028
296. Sunil HV, Mittal BR, Kurmi R, Chawla YK, Dhiman RK. Brain perfusion single photon emission computed tomography abnormalities in patients with minimal hepatic encephalopathy. J Clin Exp Hepatol. (2012) 2:116–21. doi: 10.1016/S0973-6883(12)60099-1
297. Choi IS, Lee MS. Early hypoperfusion of technetium-99m hexamethylprophylene amine oxime brain single photon emission computed tomography in a patient with carbon monoxide poisoning. Eur Neurol. (1993) 33:461–4. doi: 10.1159/000116994
298. Denays R, Tondeur M, Noël P, Ham HR. Bilateral cerebral mediofrontal hypoactivity in Tc-99m HMPAO SPECT imaging. Clin Nucl Med. (1994) 19:873–6. doi: 10.1097/00003072-199410000-00006
299. Chu K, Jung KH, Kim HJ, Jeong SW, Kang DW, Roh JK. Diffusion-weighted MRI and 99mTc-HMPAO SPECT in delayed relapsing type of carbon monoxide poisoning: evidence of delayed cytotoxic edema. Eur Neurol. (2004) 51:98–103. doi: 10.1159/000076536
300. Pach D, Hubalewska A, Huszno B, Pach J. Evaluation of regional cerebral perfusion using 99mTc-HmPAO single photon emission tomography (SPET) in carbon monoxide acutely poisoned patients. Przegl Lek. (2004) 61:217–21.
301. Tsai CF, Yip PK, Chen SY, Lin JC, Yeh ZT, Kung LY, et al. The impacts of acute carbon monoxide poisoning on the brain: Longitudinal clinical and 99mTc ethyl cysteinate brain SPECT characterization of patients with persistent and delayed neurological sequelae. Clin Neurol Neurosurg. (2014) 119:21–27. doi: 10.1016/j.clineuro.2014.01.005
302. Amen DG, Jourdain M, Taylor DV, Pigott HE, Willeumier K. Multi-site six month outcome study of complex psychiatric patients evaluated with addition of brain SPECT imaging. Adv Mind Body Med. (2013) 27:6–16.
303. Danos P, Kasper S, Grünwald F, Klemm E, Krappel C, Broich K, et al. Pathological regional cerebral blood flow in opiate-dependent patients during withdrawal: a HMPAO-SPECT study. Neuropsychobiology. (1998) 37:194–9. doi: 10.1159/000026502
304. Gerra G, Calbiani B, Zaimovic A, Sartori R, Ugolotti G, Ippolito L et al. Regional cerebral blood flow and comorbid diagnosis in abstinent opioid addicts. Psychiatry Res. (1998) 83:117–26. doi: 10.1016/S0925-4927(98)00030-4
305. Amen DG, Waugh M. High resolution brain SPECT imaging of marijuana smokers with AD/HD. J Psychoactive Drugs. (1998) 2:209–14. doi: 10.1080/02791072.1998.10399692
306. Amen DG, Darmal B, Raji CA, Bao W, Jorandby L, Meysami S, et al. Discriminative properties of hippocampal hypoperfusion in marijuana users compared to healthy controls: implications for marijuana administration in Alzheimer's dementia. J Alzheimer's Dis. (2017) 56:261–73. doi: 10.3233/JAD-160833
307. Yang W, Yang R, Tang F, Luo J, Zhang J, Chen C, et al. Decreased relative cerebral blood flow in unmedicated heroin-dependent individuals. Front Psychiatry. (2020) 11:643. doi: 10.3389/fpsyt.2020.00643
308. Ashok AH, Mizuno Y, Volkow ND, Howes OD. Association of stimulant use with dopaminergic alterations in users of cocaine, amphetamine, or methamphetamine: a systematic review and meta-analysis. JAMA Psychiatry. (2017) 74:511–9. doi: 10.1001/jamapsychiatry.2017.0135
309. Proebstl L, Kamp F, Manz K, Krause D, Adorjan K, Pogarell O, et al. Effects of stimulant drug use on the dopaminergic system: A systematic review and meta-analysis of in vivo neuroimaging studies. Eur Psychiatry. (2019) 59:15–24. doi: 10.1016/j.eurpsy.2019.03.003
310. Liang CS, Ho PS, Yen CH, Yeh YW, Kuo SC, Huang CC, et al. Reduced striatal dopamine transporter density associated with working memory deficits in opioid-dependent male subjects: a SPECT study. Addict Biol. (2016) 21:196–204. doi: 10.1111/adb.12203
311. Fowler JS, Volkow ND, Wang GJ, Gatley SJ. Logan [(11)] Cocaine: PET studies of cocaine pharmacokinetics, dopamine transporter availability dopamine transporter occupancy. J Nucl Med Biol. (2001) 28:561–72. doi: 10.1016/S0969-8051(01)00211-6
312. Ernst A, Zibrak JD. Carbon monoxide poisoning. N Engl J Med. (1998) 339:1603–8. doi: 10.1056/NEJM199811263392206
313. Bhatia R, Chacko F, Lal V, Mittal BR. Reversible delayed neuropsychiatric syndrome following acute carbon monoxide exposure. Indian J Occup Environ Med. (2007) 11:80–2. doi: 10.4103/0019-5278.34534
314. Sung YF, Chen MH, Peng GS, Lee JT. Generalized chorea due to delayed encephalopathy after acute carbon monoxide intoxication. Ann Indian Acad Neurol. (2015) 18:108–10. doi: 10.4103/0972-2327.144288
315. Chen SY, Lin CC, Lin YT, Lo CP, Wang CH, Fan YM. Reversible changes of brain perfusion SPECT for carbon monoxide poisoning-induced severe akinetic mutism. Clin Nucl Med. (2016) 41:e221–7. doi: 10.1097/RLU.0000000000001121
316. Wu CI, Changlai SP, Huang WS, Tsai CH, Lee CC, Kao CH. Usefulness of 99mTc ethyl cysteinate dimer brain SPECT to detect abnormal regional cerebral blood flow in patients with acute carbon monoxide poisoning. Nucl Med Commun. (2003) 24:1185–8. doi: 10.1097/00006231-200311000-00009
317. Ozyurt G, Kaya FN, Kahveci F, Alper E. Comparison of SPECT findings and neuropsychological sequelae in carbon monoxide and organophosphate poisoning. Clin Toxicol. (2008) 46:218–21. doi: 10.1080/15563650701378704
318. Lu YY, Tsai SC, Kao CH, Lin WY. Regional cerebral blood flow in patients with carbon monoxide intoxication. Ann Nucl Med. (2012) 26:771–6. doi: 10.1007/s12149-012-0638-8
319. Gale SD, Hopkins RO, Weaver LK, Bigler ED, Booth EJ, Blatter DD. MRI, quantitative MRI, SPECT, and neuropsychological findings following carbon monoxide poisoning. Brain Inj. (1999) 13:229–43. doi: 10.1080/026990599121601
320. Chen NC, Chang WN, Lui CC, Huang SH, Lee CC, Huang CW, et al. Detection of gray matter damage using brain MRI and SPECT in carbon monoxide intoxication: a comparison study with neuropsychological correlation. Clin Nucl Med. (2013) 38:e53–9. doi: 10.1097/RLU.0b013e31827082a7
321. Denays R, Makhoul E, Dachy B, Tondeur M, Noel P, Ham HR, et al. Electroencephalographic mapping and 99mTc HMPAO single-photon emission computed tomography in carbon monoxide poisoning. Ann Emerg Med. (1994) 24:947–52. doi: 10.1016/S0196-0644(94)70212-8
322. Chang DC, Lee JT, Lo CP, Fan YM, Huang KL, Kang BH, et al. Hyperbaric oxygen ameliorates delayed neuropsychiatric syndrome of carbon monoxide poisoning. Undersea Hyperb Med. (2010) 37:23–33.
323. Chang CC, Chang WN, Lui CC, Huang SH, Lee CC, Chen C, et al. Clinical significance of the pallidoreticular pathway in patients with carbon monoxide intoxication. Brain. (2011) 134:3632–46. doi: 10.1093/brain/awr287
324. Iwamoto K, Ikeda K, Mizumura S, Tachiki K, Yanagihashi M, Iwasaki Y. Combined treatment of methylprednisolone pulse and memantine hydrochloride prompts recovery from neurological dysfunction and cerebral hypoperfusion in carbon monoxide poisoning: a case report. J Stroke Cerebrovasc Dis. (2014) 23:592–5. doi: 10.1016/j.jstrokecerebrovasdis.2013.05.014
325. Lin YT, Chen SY, Lo CP, Lee JT, Tsai CF, Yip PK, et al. Utilizing cerebral perfusion scan and diffusion-tensor MR imaging to evaluate the effect of hyperbaric oxygen therapy in carbon monoxide-induced delayed neuropsychiatric sequalae - a case report and literature review. Acta Neurol Taiwan. (2015) 24:57–62.
326. American Psychiatric Association. Diagnostic and Statistical Manual of Mental Disorders. Arlington, VA: American Psychiatric Association (2013). doi: 10.1176/appi.books.9780890425596
327. Henderson TA. Brain SPECT imaging in neuropsychiatric diagnosis and monitoring. EPatient. (2018) 1:40–7. Available online at: http://nmpangea.com/2018/10/09/738/
328. Rush AJ, Trivedi MH, Wisniewski SR, Nierenberg AA, Stewart JW, Warden D, et al. Acute and longer-term outcomes in depressed outpatients requiring one or several treatment steps: a STAR*D report. Am J Psychiatry. (2006) 163:1905–17. doi: 10.1176/ajp.2006.163.11.1905
329. West SA, McElroy SL, Strakowski SM, Keck PE Jr, McConville BJ. Attention deficit hyperactivity disorder in adolescent mania. Am J Psychiatry. (1995) 152:271–3. doi: 10.1176/ajp.152.2.271
330. Scheffer RE, Kowatch RA, Carmody T, Rush AJ. Randomized, placebo-controlled trial of mixed amphetamine salts for symptoms of comorbid ADHD in pediatric bipolar disorder after mood stabilization with divalproex sodium. Am J Psychiatry. (2005) 162:58–64. doi: 10.1176/appi.ajp.162.1.58
331. McElroy E, Fearon P, Belsky J, Fonagy P, Patalay P. Networks of depression and anxiety symptoms across development. J Am Acad Child Adolesc. Psychiatry. (2018) 57:964–73. doi: 10.1016/j.jaac.2018.05.027
332. Kaufman J, Charney D. Comorbidity of mood and anxiety disorders. Depress Anxiety. (2000) 12:69–76. doi: 10.1002/1520-6394(2000)12:1+<69::AID-DA9>3.0.CO;2-K
333. Dilsaver SC, Henderson-Fuller S, Akiskal HS. Occult mood disorders in 104 consecutively presenting children referred for the treatment of attention-deficit/hyperactivity disorder in a community mental health clinic. J Clin Psychiatry. (2003) 64:1170–6. doi: 10.4088/JCP.v64n1005
334. Spencer T, Biederman J, Wilens T. Attention-deficit/hyperactivity disorder and comorbidity. Pediatr Clin North Am. (1999) 46:915–27. doi: 10.1016/S0031-3955(05)70163-2
335. Newcorn J, Halperin JM, Jensen PS, Abikoff HB, Arnold LE, Cantwell DP, et al. Symptom profiles in children with ADHD: effects of comorbidity and gender. J Am Acad Child Adolesc Psychiatry. (2001) 40:137–46. doi: 10.1097/00004583-200102000-00008
336. Pliszka SR. Psychiatric comorbidities in children with attention deficit hyperactivity disorder: implications for management. Paediatr Drugs. (2003) 5:741–50. doi: 10.2165/00148581-200305110-00003
337. Regier DA, Narrow WE, Clarke DE, Kraemer HC, Kuramoto SJ, et al. DSM-5 field trials in the United States and Canada, Part II: test-retest reliability of selected categorical diagnoses. Am J Psychiatry. (2013) 170:59–70. doi: 10.1176/appi.ajp.2012.12070999
338. Lee JS, Kim BN, Kang E, Lee DS, Kim YK, Chung JK, et al. Regional cerebral blood flow in children with attention deficit hyperactivity disorder: comparison before and after methylphenidate treatment. Hum Brain Mapp. (2005) 24:157–64. doi: 10.1002/hbm.20067
339. Gustafsson P, Thernlund G, Ryding E, Rosen I, Cederblad M. Associations between cerebral blood-flow measured by single photon emission computed tomography (SPECT), electro-encephalogram (EEG), behaviour symptoms, cognition and neurological soft signs in children with attention-deficit hyperactivity disorder (ADHD). Acta Paediatr. (2000) 89:830–5. doi: 10.1111/j.1651-2227.2000.tb00391.x
340. Spalletta G, Pasini A, Pau F, Guido G, Menghini L, Caltagirone C. Prefrontal blood flow dysregulation in drug naive ADHD children without structural abnormalities. J Neural Transm. (2001) 108:1203–16. doi: 10.1007/s007020170010
341. Langleben DD, Acton PD, Austin G, Elman I, Krikorian G, Monterosso JR, et al. Effects of methylphenidate discontinuation on cerebral blood flow in prepubescent boys with attention deficit hyperactivity disorder. J Nucl Med. (2002) 43:1624–9.
342. Kim BN, Lee JS, Shin MS, Cho SC, Lee DS. Regional cerebral perfusion abnormalities in attention deficit/hyperactivity disorder. Statistical parametric mapping analysis. Eur Arch Psychiatry Clin Neurosci. (2002) 252:219–25. doi: 10.1007/s00406-002-0384-3
343. Cho SC, Hwang JW, Kim BN, Lee HY, Kim HW, Lee JS, et al. The relationship between regional cerebral blood flow and response to methylphenidate in children with attention-deficit hyperactivity disorder: comparison between non-responders to methylphenidate and responders. J Psychiatr Res. (2007) 41:459–65. doi: 10.1016/j.jpsychires.2006.05.011
344. Cherkasova MV, Hechtman L. Neuroimaging in attention-deficit hyperactivity disorder: beyond the frontostriatal circuitry. Can J Psychiatry. (2009) 54:651–64. doi: 10.1177/070674370905401002
345. Kim BN, Kim JW, Kang H, Cho SC, Shin MS, Yoo HJ, et al. Regional differences in cerebral perfusion associated with the alpha-2A-adrenergic receptor genotypes in attention deficit hyperactivity disorder. J Psychiatry Neurosci. (2010) 35:330–6. doi: 10.1503/jpn.090168
346. Pliszka SR, Glahn DC, Semrud-Clikeman M, Franklin C, Perez R, Xiong J, et al. Neuroimaging of inhibitory control areas in children with attention deficit hyperactivity disorder who were treatment naive or in long-term treatment. Am J Psychiatry. (2006) 163:1052–60. doi: 10.1176/ajp.2006.163.6.1052
347. Smith AB, Taylor E, Brammer M, Toone B, Rubia K. Task-specific hypoactivation in prefrontal and temporoparietal brain regions during motor inhibition and task switching in medication-naive children and adolescents with attention deficit hyperactivity disorder. Am J Psychiatry. (2006) 163:1044–51. doi: 10.1176/ajp.2006.163.6.1044
348. Skalski S, Dobrakowski P. Vigilance, inhibitory control and regional cerebral blood oxygenation in the PFC - differences in ADHD types of presentations. Adv Cogn Psychol. (2020) 16:202–12. doi: 10.5709/acp-0297-5
349. Thornton JF, Schneider H, McLean MK, van Lierop MJ, Tarzwell R. Improved outcomes using brain SPECT-guided treatment versus treatment-as-usual in community psychiatric outpatients: a retrospective case-control study. J Neuropsychiatry Clin Neurosci. (2014) 26:51–6. doi: 10.1176/appi.neuropsych.12100238
350. Mena I, Correa R, Nader A, Boehme V. Bipolar affective disorders: assessment of functional brain changes by means of Tc99mHMPAO neuroSPECT. ALASBIMN Journal. (2004) 6:18.
351. Mena I, Correa R, Nader A. Bipolar disorder complicated by self-mutilation: neurofunctional changes demonstrated by Tc99mHMPAO neuroSPECT. ALASBIMN J. (2007) 10:15.
352. Lenox RH, Gould TD, Manji HK. Endophenotypes in bipolar disorder. Am J Med Genet. (2002) 114:391–406. doi: 10.1002/ajmg.10360
354. McLean M, Henderson TA, Pavel DG, Cohen P. Increased asymmetric perfusion of the cerebral cortices and thalamus indicates individuals at risk for bipolar disorder: a family cohort single photon emission computed tomography neuroimaging study. Front. Psychiatry. (2022) 13:829561. doi: 10.3389/fpsyt.2022.829561
355. Price JL, Drevets WC. Neurocircuitry of mood disorders. Neuropsychopharmacology. (2010) 35:192–216. doi: 10.1038/npp.2009.104
356. Caligiuri MP, Brown GG, Meloy MJ, Eberson SC, Kindermann SS, Frank LR, et al. An fMRI study of affective state and medication on cortical and subcortical brain regions during motor performance in bipolar disorder. Psychiatry Res. (2003) 123:171–82. doi: 10.1016/S0925-4927(03)00075-1
357. Hasler G, Drevets WC, Manji HK, Charney DS. Discovering endophenotypes for major depression. Neuropsychopharmacology. (2004) 29:1765–81. doi: 10.1038/sj.npp.1300506
358. Lesser IM, Mena I, Boone KB, Miller BL, Mehringer CM, Wohl M. Reduction of cerebral blood flow in older depressed patients. Arch Gen Psychiatry. (1994) 51:677–86. doi: 10.1001/archpsyc.1994.03950090009002
359. Ito H, Kawashima R, Awata S, Ono S, Sato K, Goto R, et al. Hypoperfusion in the limbic system and prefrontal cortex in depression: SPECT with anatomic standardization technique. J Nucl Med. (1996) 37:410–4.
360. Ebmeier KP, Cavanagh JT, Moffoot AP, Glabus MF, O'Carroll RE, Goodwin GM. Cerebral perfusion correlates of depressed mood. Br J Psychiatry. (1997) 170:77–81. doi: 10.1192/bjp.170.1.77
361. Galynker II, Cai J, Ongseng F, Finestone H, Dutta E, Serseni D. Hypofrontality and negative symptoms in major depressive disorder. J Nucl Med. (1998) 39:608–12.
362. Fountoulakis KN, Iacovides A, Gerasimou G, Fotiou F, Ioannidou C, Bascialla F, et al. The relationship of regional cerebral blood flow with subtypes of major depression. Prog Neuropsychopharmacol Biol Psychiatry. (2004) 28:537–46. doi: 10.1016/j.pnpbp.2004.01.006
363. Pagani M, Salmaso D, Nardo D, Jonsson C, Jacobsson H, Larsson SA, et al. Imaging the neurobiological substrate of atypical depression by SPECT. Eur J Nucl Med Mol Imaging. (2007) 34:110–20. doi: 10.1007/s00259-006-0177-4
364. Nagafusa Y, Okamoto N, Sakamoto K, Yamashita F, Kawaguchi A, Higuchi T, et al. Assessment of cerebral blood flow findings using 99mTc-ECD single-photon emission computed tomography in patients diagnosed with major depressive disorder. J Affect Disord. (2012) 140:296–9. doi: 10.1016/j.jad.2012.03.026
365. Li J, Yang Y, Zhu Y, Zhou L, Han Y, Yin T, et al. Towards characterizing the regional cerebral perfusion in evaluating the severity of major depression disorder with SPECT/CT. BMC Psychiatry. (2018) 18:70. doi: 10.1186/s12888-018-1654-6
366. Goodwin GM, Austin MP, Dougall N, Ross M, Murray C, O'Carroll RE, et al. State changes in brain activity shown by the uptake of 99mTc-exametazime with single photon emission tomography in major depression before and after treatment. J Affect Disord. (1993) 29:243–53. doi: 10.1016/0165-0327(93)90014-B
367. Drevets WC, Savitz J, Trimble M. The subgenual anterior cingulate cortex in mood disorders. CNS Spectr. (2008) 13:663–81. doi: 10.1017/S1092852900013754
368. Brockmann H, Zobel A, Joe A, Biermann K, Scheef L, Schuhmacher A, et al. The value of HMPAO SPECT in predicting treatment response to citalopram in patients with major depression. Psychiatry Res. (2009) 173:107–12. doi: 10.1016/j.pscychresns.2008.10.006
369. Ogura A, Morinobu S, Kawakatsu S, Totsuka S, Komatani A. Changes in regional brain activity in major depression after successful treatment with antidepressant drugs. Acta Psychiatr Scand. (1998) 98:54–9. doi: 10.1111/j.1600-0447.1998.tb10042.x
370. Loo CK, Sachdev PS, Haindl W, Wen W, Mitchell PB, Croker VM, et al. High (15 Hz) and low (1 Hz) frequency transcranial magnetic stimulation have different acute effects on regional cerebral blood flow in depressed patients. Psychol Med. (2003) 33:997–1006. doi: 10.1017/S0033291703007955
371. Hanada H, Imanaga J, Yoshiiwa A, Yoshikawa T, Tanaka Y, Tsuru J, et al. The value of ethyl cysteinate dimer single photon emission computed tomography in predicting antidepressant treatment response in patients with major depression. Int J Geriatr Psychiatry. (2013) 28:756–65. doi: 10.1002/gps.3887
372. Amen DG, Taylor DV, Meysami S, Raji CA. Deficits in regional cerebral blood flow on brain SPECT predict treatment resistant depression. J Alzheimer's Dis. (2018) 63:529–38. doi: 10.3233/JAD-170855
373. Drevets WC. Functional neuroimaging studies of depression: the anatomy of melancholia. Annu Rev Med. (1998) 49:341–61. doi: 10.1146/annurev.med.49.1.341
374. Milak MS, Parsey RV, Keilp J, Oquendo MA, Malone KM, Mann JJ. Neuroanatomic correlates of psychopathologic components of major depressive disorder. Arch Gen Psychiatry. (2005) 62:397–408. doi: 10.1001/archpsyc.62.4.397
375. Saxena S, Brody AL, Schwartz JM, Baxter LR. Neuroimaging and frontal-subcortical circuitry in obsessive-compulsive disorder. Br J Psychiatry Suppl. (1998) 35:26–37 doi: 10.1192/S0007125000297870
376. Rubin RT, Villanueva-Meyer J, Ananth J, Trajmar PG, Mena I. Regional xenon 133 cerebral blood flow and cerebral technetium 99m HMPAO uptake in unmedicated patients with obsessive-compulsive disorder and matched normal control subjects. Determination by high-resolution single-photon emission computed tomography. Arch Gen Psychiatry. (1992) 49:695–702. doi: 10.1001/archpsyc.1992.01820090023004
377. Harris GJ, Hoehn-Saric R, Lewis R, Pearlson GD, Streeter C. Mapping of SPECT regional cerebral perfusion abnormalities in obsessive-compulsive disorder. Hum Brain Mapp. (1994) 1:237–48. doi: 10.1002/hbm.460010403
378. Crespo-Facorro B, Cabranes JA, López-Ibor Alcocer MI, Payá B, Fernández Pérez C, Encinas M, et al. Regional cerebral blood flow in obsessive-compulsive patients with and without a chronic tic disorder. A SPECT study. Eur Arch Psychiatry Clin Neurosci. (1999) 249:156–61. doi: 10.1007/s004060050081
379. Busatto GF, Buchpiguel CA, Zamignani DR, Garrido GE, Glabus MF, Rosario-Campos MC, et al. Regional cerebral blood flow abnormalities in early-onset obsessive-compulsive disorder: an exploratory SPECT study. J Am Acad Child Adolesc Psychiatry. (2001) 40:347–54. doi: 10.1097/00004583-200103000-00015
380. Diler RS, Kibar M, Avci A. Pharmacotherapy and regional cerebral blood flow in children with obsessive compulsive disorder. Yonsei Med J. (2004) 45:90–9. doi: 10.3349/ymj.2004.45.1.90
381. Castillo AR, Buchpiguel CA, de Araújo LA, Castillo JC, Asbahr FR, Maia AK, et al. Brain SPECT imaging in children and adolescents with obsessive-compulsive disorder. J Neural Transm. (2005) 112:1115–29. doi: 10.1007/s00702-004-0240-x
382. Ho Pian KL, van Megen HJ, Ramsey NF, Mandl R, van Rijk PP, Wynne HJ, et al. Decreased thalamic blood flow in obsessive-compulsive disorder patients responding to fluvoxamine. Psychiatry Res. (2005) 138:89–97. doi: 10.1016/j.pscychresns.2004.12.003
383. Yamanishi T, Nakaaki S, Omori IM, Hashimoto N, Shinagawa Y, Hongo J, et al. Changes after behavior therapy among responsive and nonresponsive patients with obsessive-compulsive disorder. Psychiatry Res. (2009) 172:242–50. doi: 10.1016/j.pscychresns.2008.07.004
384. Karadag F, Kalkan Oguzhanoglu N, Yüksel D, Kiraç S, Cura C, Ozdel O, et al. The comparison of pre- and post-treatment (99m)Tc HMPAO brain SPECT images in patients with obsessive-compulsive disorder. Psychiatry Res. (2013) 213:169–77. doi: 10.1016/j.pscychresns.2012.07.005
385. Wen SL, Cheng MH, Cheng MF, Yue JH, Wang H. Pharmacotherapy response and regional cerebral blood flow characteristics in patients with obsessive-compulsive disorder. Behav Brain Funct. (2013) 9:31. doi: 10.1186/1744-9081-9-31
386. Perani D, Colombo C, Bressi S, Bonfanti A, Grassi F, Scarone S, et al. [18F]FDG PET study in obsessive-compulsive disorder. A clinical/metabolic correlation study after treatment. Br J Psychiatry. (1995) 166:244–50. doi: 10.1192/bjp.166.2.244
387. Brody AL, Saxena S, Schwartz JM, Stoessel PW, Maidment K, Phelps ME, et al. FDG-PET predictors of response to behavioral therapy and pharmacotherapy in obsessive compulsive disorder. Psychiatry Res. (1998) 84:1–6. doi: 10.1016/S0925-4927(98)00041-9
388. Hou JM, Zhao M, Zhang W, Song LH, Wu WJ, Wang J, et al. Resting-state functional connectivity abnormalities in patients with obsessive-compulsive disorder and their healthy first-degree relatives. J Psychiatry Neurosci. (2014) 39:304–11. doi: 10.1503/jpn.130220
389. Tomiyama H, Nakao T, Murayama K, Nemoto K, Ikari K, Yamada S, et al. Dysfunction between dorsal caudate and salience network associated with impaired cognitive flexibility in obsessive-compulsive disorder: A resting-state fMRI study. Neuroimage Clin. (2019) 24:102004. doi: 10.1016/j.nicl.2019.102004
390. Hazari N, Narayanaswamy JC, Venkatasubramanian G. Neuroimaging findings in obsessive-compulsive disorder: A narrative review to elucidate neurobiological underpinnings. Indian J Psychiatry. (2019) 61(Suppl 1):S9–29. doi: 10.4103/psychiatry.IndianJPsychiatry_525_18
391. Tanielan T, Jaycox LH. Invisible Wounds of War: Psychological and Cognitive Injuries, Their Consequences, and Services to Assist Recovery. Santa Monica, CA: RAND Corporation (2008). doi: 10.1037/e527612010-001
392. Rosenfeld JV, McFarlane AC, Bragge P, Armonda RA, Grimes JB, Ling GS. Blast-related traumatic brain injury. Lancet Neurol. (2013) 12:882–93. doi: 10.1016/S1474-4422(13)70161-3
393. Sachinvala N, Kling A, Suffin S, Lake R, Cohen M. Increased regional cerebral perfusion by 99mTc hexamethyl propylene amine oxime single photon emission computed tomography in post-traumatic stress disorder. Mil Med. (2000) 165:473–9. doi: 10.1093/milmed/165.6.473
394. Chung YA, Kim SH, Chung SK, Chae JH, Yang DW, Sohn HS, et al. Alterations in cerebral perfusion in posttraumatic stress disorder patients without re-exposure to accident-related stimuli. Clin Neurophysiol. (2006) 117:637–42. doi: 10.1016/j.clinph.2005.10.020
395. Rauch SL, van der Kolk BA, Fisler RE. A symptom provocation study of posttraumatic stress disorder using positron emission tomography and script-driven imagery. Arch Gen Psychiatry. (1996) 53:380–7. doi: 10.1001/archpsyc.1996.01830050014003
396. Liberzon I, Taylor SF, Amdur R, Jung TD, Chamberlain KR, Minoshima S, et al. Brain activation in PTSD in response to trauma-related stimuli. Biol Psychiatry. (1999) 45:817–26. doi: 10.1016/S0006-3223(98)00246-7
397. Lindauer RJ, Booij J, Habraken JB, van Meijel EP, Uylings HB, Olff M, et al. Effects of psychotherapy on regional cerebral blood flow during trauma imagery in patients with post-traumatic stress disorder: a randomized clinical trial. Psychol Med. (2008) 38:543–54. doi: 10.1017/S0033291707001432
398. Hughes KC, Shin LM. Functional neuroimaging studies of post-traumatic stress disorder. Expert Rev Neurother. (2011) 11:275–85. doi: 10.1586/ern.10.198
399. Pavel DG, Henderson TA, DeBruin S. The legacy of the TTASAAN report premature conclusions and forgotten promises: A review of policy and practice Part II. Front Neuro. (in press).
Keywords: dementia, traumatic brain injury, seizure, neurotoxicity, depression, bipolar disorder, ADHD, PTSD-posttraumatic stress disorder
Citation: Pavel DG, Henderson TA and DeBruin S (2022) The Legacy of the TTASAAN Report—Premature Conclusions and Forgotten Promises: A Review of Policy and Practice Part I. Front. Neurol. 12:749579. doi: 10.3389/fneur.2021.749579
Received: 29 July 2021; Accepted: 14 December 2021;
Published: 28 March 2022.
Edited by:
Kristen Willeumier, Independent Researcher, Beverly Hills, United StatesReviewed by:
Daniel Gregory Amen, Amen Clinics, Inc., United StatesRodolfo Gabriel Gatto, University of Illinois at Chicago, United States
Copyright © 2022 Pavel, Henderson and DeBruin. This is an open-access article distributed under the terms of the Creative Commons Attribution License (CC BY). The use, distribution or reproduction in other forums is permitted, provided the original author(s) and the copyright owner(s) are credited and that the original publication in this journal is cited, in accordance with accepted academic practice. No use, distribution or reproduction is permitted which does not comply with these terms.
*Correspondence: Theodore A. Henderson, dGhlc3luYXB0aWNzcGFjZTdAZ21haWwuY29t
†Deceased