- 1Department of Otorhinolaryngology, Head and Neck Surgery, Martin Luther University Halle-Wittenberg, Halle, Germany
- 2Department of Otorhinolaryngology, Head and Neck Surgery, Friedrichshain Clinic, Vivantes Hearing Center, Berlin, Germany
- 3Department of Otorhinolaryngology, Head and Neck Surgery, University Medicine of Greifswald, Greifswald, Germany
- 4Department of Otorhinolaryngology, Head and Neck Surgery “Otto Körner”, Rostock University Medical Center, Rostock, Germany
Objective: To analyze intensity-latency functions of intraoperative auditory evoked brainstem responses (ABRs) to stimulation by the Vibrant Soundbridge (VSB) active middle ear implant with respect to coupling efficiency, VSB evoked ABR thresholds, and coupling modality [oval window (OW) placement vs. Incus placement and vs. round window (RW) placement].
Study Design: Exploratory study.
Setting: Bi-centric study at tertiary referral centers.
Patients: Twenty-four patients (10 female, 14 male, mean age: 58 years) who received a VSB.
Outcome Measures: Wave-V intensity-latency functions of intraoperative VSB evoked ABRs using a modified audio processor programmed to preoperative bone conduction thresholds for stimulation. Threshold level correction to coupling efficiency and ABR thresholds. Individual plots and exponential function fits.
Results: After ABR threshold level correction, the latency functions could be aligned. A large variance of latencies was observed at individual threshold level. Wave-V latency was longest in the Incus placement subgroup (9.73 ms, SD: 1.04) as compared to OW placement subgroup (9.47 ms, SD: 1.05), with the shortest latency in the RW placement subgroup (8.99 ms, SD: 0.68). For increasing stimulation levels, the variance decreased with intensity-latency function slopes converging toward a steady-state (saturation) latency caused by saturation of audio processor (stimulation) gain. Latency saturation was reached at a stimulation level of 50 dB nHL for the OW placement subgroup, 35 dB nHL for the Incus placement subgroup, and 30 dB nHL for the RW placement subgroup. The latency and saturation results indicated decreased dynamic range for RW placement, i.e., reverse stimulation.
Conclusions: VSB evoked ABR wave-V intensity-latency function slopes were similar to acoustic stimulation at high stimulation levels with a shift toward longer latencies caused by audio processor signal delay. Saturation of latencies occurred for higher stimulation levels due to saturation of audio processor gain. Thus, the analysis of VSB evoked intensity-latency functions appears to allow for the objective assessment of a patient's individual dynamic range. This can further improve diagnostics as well as intraoperative and postoperative quality control.
Introduction
Active middle ear implants (AMEI) are widely used for hearing rehabilitation in patients with sensorineural, conductive, and mixed hearing loss. This has become an appropriate solution for those who cannot be treated with conventional hearing aids due to technical issues such as feedback or sound distortion, or patient related issues like recurrent infections of the auditory canal (1). The Vibrant Soundbridge™ (VSB) (MED-EL, Innsbruck, Austria) is one of the available AMEI systems transforming sound into mechanical vibrations by its electromagnetic miniature floating mass transducer (FMT) (2, 3). The device was originally designed for treatment of sensorineural hearing loss where the only option for vibrational energy transfer was to couple the FMT to the long process of the incus (4). With the development of news couplers, the FMT can now be coupled to the long process (LP) of the incus (4), the short process (SP) and the head of the incus (5), the stapes suprastructure or the stapes footplate, i.e., the oval window (OW) (6, 7), and the round window (RW) membrane (8). The surgical procedure is referred to as vibroplasty. The performance of AMEIs is determined by biomechanical factors (9) as well as by the surgical procedure itself. With coupling location and coupling direction, which will be summarized and referred to as coupling modality here, the effective direction of stimulation varies. The output of AMEIs can be investigated by Laser-Doppler vibrometry which is also a feasible method to compare transfer functions for different coupling modalities (10). It has been shown that transfer functions for electromechanical stimulation by the FMT are different from acoustic stimulation and variations for different coupling modalities have been reported. Coupling to the stapes suprastructure or stapes footplate (OW placement) resulting in stimulation in the natural, normal direction (i.e., perpendicular to the OW) are biomechanically equally efficient (11). Regarding Incus placement, coupling to the incus body (SP) is a more complex coupling point, because in normal middle ears the malleus-incus motion rotation axis changes with frequency (12, 13). Thus, there is a risk of ineffective movements with FMT resulting in a reduction of the transfer function (9). However, this was not visible in experimental Laser-Doppler vibrometry measurements with the SP-Coupler (5). Similar velocity responses (transfer functions) have been obtained for coupling to the LP (14). In contrast, coupling to the RW (RW placement) results in reverse stimulation of the cochlea, which is a different mechanism compared to forward stimulation as was shown by intracochlear differential pressure measurements (15). Reverse stimulation was found to be less effective than stimulation at the stapes footplate (natural, normal direction) (16), and the efficiency of RW coupling was influenced significantly by technical and surgical factors (17).
Hearing improvement in patients treated with a VSB is highly dependent on sufficient energy transfer to the inner ear, i.e., on efficient coupling between the FMT and the middle ear structure or the RW (18). Clinically, the coupling efficiency can be quantified by the difference between vibroplasty in situ thresholds (vibrogram—VIB), which are measured as behavioral thresholds by ordinary pure-tone audiometry to stimulation via the implanted FMT, and bone conduction (BC) thresholds. Small differences indicate good coupling, i.e., efficient energy transmission to the inner ear without loss of energy. To determine the coupling efficiency objectively, auditory evoked potentials like auditory steady state responses (ASSRs) (19, 20), compound action potentials (CAPs) (21–23), and auditory brainstem responses (ABRs) (24–27) have been recorded in patients treated with a VSB. Custom-made experimental set-ups were used in all studies for providing stimulus transmission by the FMT. While most studies only aimed on relative measurements, it has recently been reported that coupling efficiency could also be determined quantitatively by measuring VSB evoked ABRs (26, 27). A modified AP404 audio processor (MED-EL, Innsbruck, Austria) programmed to preoperative BC thresholds and fitted with an insert earphone sound tube attached to the microphone aperture was used for providing stimulation by the implanted FMT. The VSB evoked ABR thresholds in this set-up have been shown to directly predict the mean coupling efficiency at 1, 2, and 4 kHz (3PTAcoupling–pure tone average of coupling efficiency at 1, 2, and 4 kHz). However, VSB evoked ABR wave-V intensity-latency functions have not been evaluated so far with respect to coupling efficiency or other parameters.
ABR measurements, as first described by Jewett in 1970 (28), are regularly used today for threshold determination in infants and objective evaluation of the auditory pathway. ABR wave-V intensity-latency functions can be used to assess and differentiate between types and magnitude of hearing loss objectively (29–31). However, for clinical interpretations it has to be considered that intensity-latency functions are also dependent on EEG (electroencephalogram) filters, stimulation rate (32), the stimulus itself, and stimulation mode, i.e., longer latencies but comparable variance have been reported for stimulation by BC vs. air conduction (AC) (33). Typical stimuli for ABR recordings are clicks but also other stimuli such as specific chirps, e.g., the CE-Chirp®, have been developed in recent years (34). Studies have reported larger amplitudes for chirps as compared to traditional click stimuli helping in threshold determination (35, 36). Due to the variability of ABRs and ABR wave-V intensity-latency functions for different stimulation parameters, normative data were recorded for clicks and CE-Chirps (34, 37–39), respectively.
With the growing number of implantation of AMEIs and other implantable hearing devices such as cochlear implants, the field of auditory evoked potential recording becomes increasingly important in objective assessment of implant performance and outcome prediction. Thus, research in the field is urgently necessary. For cochlear implants, the use of auditory evoked potentials to electrical stimulation is already part of clinical routine measurements [see for example (40) for an overview]. Normative data for electrically evoked ABR wave V latencies and interpeak latencies have also been established (41). However, standardized ABR peak V latencies as well as intensity-latency functions obtained by aided stimulation with AMEIs are currently lacking in the literature. The studies investigating VSB evoked ABRs did not report latency data but focused on thresholds to predict coupling efficiency (25–27). In electrocochleography measurements, Colletti et al. found reduced CAP latencies and higher amplitudes in case of better coupling (23). Verhaert et al. investigated ABRs to stimulation by a Codacs™ implant (Cochlear, Sydney, Australia) in three subjects and reported latencies longer than for normal hearing subjects to acoustic stimulation with some interindividual variability (42). Wave-V intensity-latency functions were not analyzed in their study. Cebulla et al. described an optimized CE-Chirp for ABR measurements with the VSB and reported larger amplitudes compared to using standard CE-Chirps but did not elaborate on intensity-latency functions (24).
The objective of this study was to analyze and describe VSB evoked ABR wave-V intensity-latency functions with respect to coupling efficiency, response thresholds, and coupling modality (OW placement vs. Incus placement and vs. RW placement).
Materials and Methods
Study Design and Participants
An exploratory study was conducted on adult patients who were regularly scheduled for hearing rehabilitation with the VSB between September 2017 and March 2021. The audiological and patient criteria for implantation as provided by the manufacturer were adhered (absence of active middle ear infections; ability to get benefit from amplification; ear anatomy allows FMT positioning; stable BC thresholds ≤45 dB HL at 0.5 kHz, ≤50 dB HL at 1 kHz, ≤55 dB at 1.5 kHz, and ≤65 at 2, 3, and 4 kHz). Patients suffering from retro-cochlear, or central auditory disorders as well as patients suffering from conditions that would interfere with the ability to adequately perform the psychoacoustic tests were excluded from the study. If postoperative BC thresholds deteriorated by more than 10 dB compared to preoperative BC thresholds, the patients were excluded from further analysis as well. Informed written consent was obtained from all participants for being included in the study. The study took place at the University Hospital Halle (Saale), Germany and the Friedrichshain Clinic, Vivantes Hearing Center, Berlin, Germany. The protocol was approved by the ethical committee of the Medical Faculty of the Martin Luther University Halle-Wittenberg (approval number 2018-34) and the study was conducted in accordance with the Declaration of Helsinki.
Patients were grouped according to biomechanically comparable FMT coupling modalities to the OW (OW placement subgroup), to the incus (Incus placement subgroup), and to the RW (RW placement subgroup). The OW placement subgroup included all patients with coupling to the stapes suprastructure via a CliP-Coupler or via a Symphonix-Coupler (modified and off-label use for coupling to the stapes head and anterior crus), or to the stapes footplate via an OW-Coupler. All patients with SP-, LP-, or Symphonix-Couplers with standard use (incus vibroplasty) were included in the Incus placement subgroup. The RW placement subgroup included all patients with RWS- (round window soft), or RW-Couplers as well as direct coupling to the RW without a specific coupler (RW vibroplasty).
Pure-Tone Audiometry
Before surgery, the patients' AC and BC thresholds (preoperative AC and BC) were measured as behavioral pure-tone thresholds at frequencies of 0.5, 1, 2, 3, 4, and 6 kHz with clinical routine audiometers and transducers (circumaural headphones and BC transducers) in a soundproof booth. Approximately 6 weeks after surgery, BC pure tone thresholds and the VIB thresholds were measured as behavioral thresholds using Symfit fitting software (MED-EL, Innsbruck, Austria) within Connexx software (Sivantos GmbH under Trademark License of Siemens AG, Erlangen, Germany) and a Samba Lo audio processor (MED-EL, Innsbruck, Austria). Pure-tone thresholds at 0.5, 1, 2, and 4 kHz were averaged (4PTAAC, 4PTABC, 4PTAVIB,). Coupling efficiency was determined by computing the difference of postoperatively measured VIB threshold minus BC thresholds, averaged over frequencies of 1, 2, and 4 kHz (3PTAcoupling).
VSB Evoked ABR Recordings
VSB evoked ABRs were recorded intraoperatively after positioning of the FMT as described in Froehlich et al. (27). Broadband CE-Chirps were generated by an Eclipse EP25 (Interacoustics A/S, Middelfart, Denmark) clinical auditory evoked potential stimulation and recording system. The acoustic stimuli were delivered at a rate of 49.1 Hz and alternating polarity via a sound tube of EAR-3A insert earphones (3 M, St. Paul, MS, USA) attached to the microphone aperture of an AP404 audio processor (MEDL-EL, Innsbruck, Austria). The gain of the audio processor was set according to the patients' preoperative BC thresholds. The output limitation, compression, and special options (noise reduction, speech enhancement features, etc.) were deactivated.
ABRs were recorded in a two-channel set-up. Self-adhesive surface electrodes were placed at the hairline (active), ~1 cm below this electrode (ground), and on the contralateral mastoids (reference) of the patients. The ipsilateral reference electrode was located at the neck to provide adequate distance to the surgical field. All impedances were kept below 5 kΩ. The EEG signal was sampled at 30 kHz with an A/D resolution of 16 bits and bandpass-filtered between 33 Hz and 1.5 kHz. Epochs with an RMS amplitude below the artifact level of 40 μV were averaged to at least 1,000 stimuli or less, if the residual noise was below 40 nV.
All stimulation levels in the described experimental set-up will be provided in “dB nHL” according to the intrinsic calibration of the Eclipse system. The stimulus intensity level (stimulation level) was increased in steps of 10 dB, or 5 dB close to the ABR threshold and wave V was identified. The lowest level that reproducibly evoked an ABR response was defined as VSB evoked ABR threshold (LABR).
Data Analysis
Descriptive statistics were used to report pre- and postoperative 4PTAAC and 4PTABC thresholds, as well as intraoperative LABR and latency data for the different subgroups. Quantitative data were presented as mean and standard deviation (SD).
Individual intensity-latency functions were computed and depicted for each patient in the different subgroups as uncorrected, 3PTAcoupling-corrected, and LABR-corrected intensity-latency functions. 3PTAcoupling-corrected intensity-latency functions were computed by subtracting the individual 3PTA coupling efficiency from the original stimulation levels, i.e., shifting the intensity-latency function toward the perceived loudness. LABR-corrected intensity-latency functions were computed by subtracting the individual LABR (thresholds) from the original stimulation levels. Those intensity-latency functions were aligned to 10 dB nHL, i.e., the lowest stimulation level for which normative intensity-latency functions were available.
Using a Python software script, individual LABR-corrected intensity-latency functions were fitted to the function Latency (ms) = a∧(Stimulation level–b)+c to compute interpolated latencies for all stimulation levels.
Interpolated wave-V latencies at LABR threshold level were compared between the different coupling modality subgroups by a one-way analysis of variance (ANOVA). Group averages of interpolated intensity-latency functions were computed for the different subgroups. According to the slope of normal latency-intensity functions at large stimulation levels, the lowest stimulation level at which the mean wave-V latency dropped below 0.10 ms/dB was defined as saturation level.
IBM SPSS-Software version 25 (IBM, Ehningen, Germany) was used for all statistical analyses. Alpha was set to 0.05.
Results
Twenty-four patients (10 female, 14 male) aged between 33 and 81 years (mean: 58 years) were initially included in the study. Two patients were excluded from further analysis due to reduced 4PTABC by at least 10 dB compared to the preoperatively measured 4PTABC. Table 1 shows the demographic data including FMT-coupling modality (n = 13 patients in OW placement subgroup, n = 4 patients in Incus placement subgroup, and n = 5 patients in RW placement subgroup) and audiological results. For all patients included in the final analysis (n = 22), VSB evoked ABRs could be measured intraoperatively with mean VSB evoked ABR thresholds (LABR) of 6.2 (SD: 5.6) dB nHL (OW placement), 11.0 (SD: 2.2) dB nHL (Incus placement), and 12.04 (SD: 4.0) dB nHL (RW placement).
Wave-V latencies could be discerned in all patients. Figure 1A shows the individual intensity-latency functions. Close to the individual LABR, wave-V latency decreased with increasing stimulation level with a slope comparable to that of reference latency data for acoustic stimulation (37–39). At stimulation levels of 30 dB and more above LABR, the slopes converged into a steady-state latency of 7–8 ms. Individual wave-V intensity-latency functions as level-corrected by the individual 3PTAcoupling are shown in Figure 1B. Figure 1C shows the LABR-corrected wave-V intensity-latency functions by aligning the lowest stimulation level with a clear wave-V response to 10 dB nHL. At individual threshold level LABR, wave-V latencies showed a large interindividual variance. For increasing stimulus levels, the variance decreased with intensity-latency functions converging toward the saturation latency.
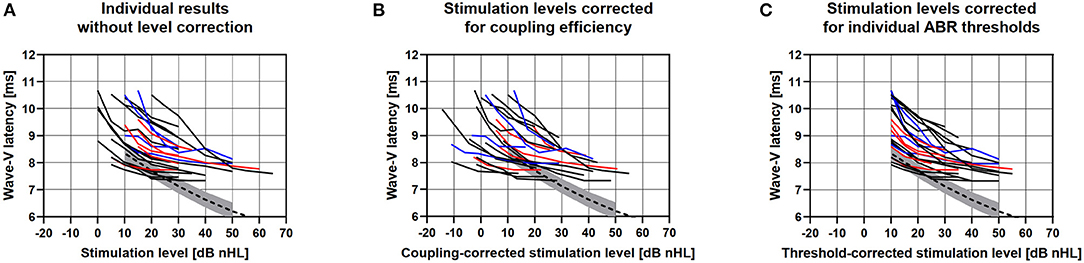
Figure 1. (A) Individual VSB evoked ABR wave-V latencies for all applied stimulation levels of all included patients with OW placement (black), Incus placement (blue), and RW placement (red). The dashed curves show the mean wave-V reference data of an acoustic click stimulation for the ABR system used in the study. Gray areas show the standard deviations. (B) Individual wave-V latencies after level correction for coupling efficiency, i.e., reduction by 3PTAVIB minus 3PTABC. (C) Individual wave-V latencies after correction for the individual ABR threshold, aligned to a stimulation level of 10 dB nHL.
The mean intensity-latency functions for all patients and subgroups of OW-, Incus-, and RW placement, were computed from the interpolated LABR-corrected intensity-latency functions and depicted in Figure 2. Over all patients and subgroups, the wave-V latencies were largest at 10 dB nHL (9.4 ms; SD: 0.93) and decreased with increasing stimulation levels to 8.06 ms (SD: 0.46) at 40 dB nHL and 7.84 ms (SD: 0.49) at 70 dB nHL. At threshold level LABR, the wave-V latency was longest in the Incus placement subgroup (9.73 ms, SD: 1.04), followed by the OW placement subgroup (9.47 ms, SD: 1.05), and the shortest latency was observed in the RW placement subgroup (8.99 ms, SD: 0.68). The reference for acoustic stimulation, i.e., wave-V latency for acoustic click stimulation, was 8.35 ms (SD: 0.55) at 10 dB nHL. The effect of coupling modality subgroup on wave-V latency at threshold level LABR was not statistically significant [F(2,19) = 0.706, p = 0.506]. In the RW placement subgroup, mean wave-V latencies were lower as compared with the Incus placement subgroup at all stimulation levels. Latency saturation was reached at a stimulation level of 50 dB nHL for the OW placement subgroup, 35 dB nHL for the Incus placement subgroup, and 30 dB nHL for the RW placement subgroup.
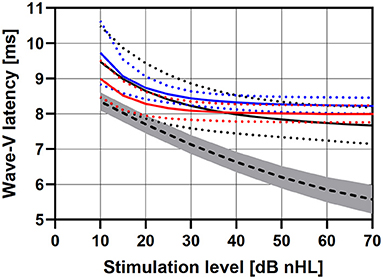
Figure 2. VSB evoked ABR wave-V latencies as group averages for subgroups of OW placement (black), Incus placement (blue), and RW placement (red). Reference data for an acoustic click stimulation are shown as dashed line. The dotted lines, errors bars, and the gray area depict standard deviations.
Discussion
The results from our study showed no correlation of VSB evoked ABR wave-V intensity-latency functions with respect to coupling efficiency (see Figure 1B) and rather large variance of the individual wave-V latencies at threshold level LABR. However, it was observed that intensity-latency function slopes converged into a steady-state latency of 7–8 ms at stimulation levels between of 30 dB and more above LABR. This is in contrast to acoustic stimulation where the latencies continue to decrease with increasing stimulation levels. Saturation in VSB evoked ABR wave-V latencies most likely occurred to due limited dynamic range of the audio processor used for signal transmission. It was already reported in the study investigating the relation between LABR and coupling efficiency that the audio processor output saturated between 35 and 40 dB nHL stimulation level depending on gain settings [see Figure 1 in (27)]. Stimulation levels at threshold level LABR were perceived by the patient as very soft sounds. Accordingly, the perceived loudness by the patient did not increase with saturation of output by the audio processor, resulting in saturation of ABR latencies. Thus, the analysis of VSB evoked ABR wave-V intensity-latency functions enables the objective prediction of dynamic range for the individual patient treated with the AMEI.
It was observed that the interpolated LABR-corrected intensity-latency functions showed differences between wave-V latencies at LABR with respect to coupling modality although not statistically significant. However, the difference between latencies at high stimulation levels above saturation level was unexpected and most likely caused mathematically by the applied approximation functions. A saturation at equal latencies was expected based on technical limitations (see above). Shorter latencies and lower levels for saturation as found for the RW placement group in comparison to the OW- and Incus placement group indicated differences in signal transmission between the different coupling modalities. That is, a lower dynamic range was observed for patients with reverse stimulation for RW placement especially in comparison to OW placement. This is clinically significant as it suggests that there is a difference between activation of the auditory system by direct stimulation in the natural normal direction via the oval window—similar to acoustic stimulation—and reverse stimulation via the RW. The method could therefore be used to investigate the clinical implications of different coupling modalities. The total offset between VSB evoked wave-V latencies and normative data for acoustic stimulation was most likely caused by a signal processing delay in the audio processor. Reference data for acoustic click stimulation were used here due to technical measurements which showed that the CE-chirp used for stimulation emerged as a click-like stimulus after transmission through the audio processor and VSB (24, 27).
The finding of reduced dynamic range in RW coupling modalities is of special clinical interest and complements findings in other studies showing that RW placement is surgically and technically less favorable compared to OW placement or Incus placement. With respect to coupling efficiency, OW and Incus placement showed better efficiency (smaller differences between VIB and BC thresholds) compared to RW placement in a study by Müller et al. (18). If the loss of energy transmission to the inner ear cannot be compensated technically by adjusting gain settings, insufficient coupling can result in the need for revision surgeries. Data from the literature for revision surgeries in RW vibroplasty vary between 9.5% (43) and 29.0–71.0%, depending on the use of couplers (44). In comparison to other coupling modalities, RW coupling showed the highest complication rates (52.6%) in a study by Brkic et al. (45). Postoperative BC deterioration is one of the problems reported for RW vibroplasty. Data in the literature vary between 3.0% (46) and 20.0% in patients with RW vibroplasty (compared to 11.1% of patients with incus vibroplasty) (47).
Comparison between acoustic stimulation and electromechanical stimulation by an FMT has only been performed in experimental studies using Laser-Doppler vibrometry so far. These studies provided objective evidence that acoustic stimulation is mechanically different from electromechanical stimulation by the VSB (9, 10). However, normative data on the characteristics of VSB evoked ABRs as a new objective method were not available in the literature so far. Thus, comparison of our data to data in the literature is limited because only very few studies reported AMEI evoked wave-V latencies. Verhaert et al. (42) observed interindividual wave-V latency differences in their study. This is in line with our findings although comparison is limited due to the difference between AMEIs investigated in the studies and the experimental stimulation set-ups applied for signal transmission. Generally, variability in latency is also affected by hearing loss, age, and sex among other factors (48, 49).
In summary, the results showed that the measurement of VSB evoked ABR wave-V intensity-latency functions allows for the assessment of the patient's individual dynamic range as well as comparison between coupling modalities. In this aspect, it is similar to measuring transfer functions for different coupling modalities but with respect to the actual coupling situation in the individual patient. Thus, postoperative recordings of VSB evoked intensity-latency functions could presumably be used to qualitatively assess coupling efficiency within one patient. With the transmission characteristics, i.e., the coupling efficiency, changing over time, a change of intensity-latency functions would be expected. Thus, VSB evoked ABR wave-V intensity-latency functions may be used for postoperative quality control in follow-up visits where the patients serve as their own control.
One of the major limiting factors of the current study is the limited number of data points for wave V-latencies, i.e., the limited range of stimulation intensities for which VSB evoked ABRs were recorded. More data points for higher stimulation levels above threshold level have to be recorded in future investigations to acquire data for a larger dynamic range. The different number of patients in each subgroup was another factor limiting the interpretation of the data. Both, the limited number of data points and unequal size of subgroups, account for the explorative, hypotheses generating nature of the current study. Another challenging factor was the multidimensionality of the analysis, as intensity-latency functions could depend on various different factors such as the patients' hearing loss, i.e., dynamic range provided by the audio processor by its gain settings, the coupling efficiency, the coupling modality, and the frequency specific output of the VSB itself. For the analysis, we concentrated on high frequencies between 1 and 4 kHz (3PTA) where contribution to signal transmission with the VSB is largest. The current study only concentrates on CE-Chirp stimulation which results in click-like stimulation with the VSB. The use of a VSB specific chirp as suggested by Cebulla et al. (24) may give different results. Besides these limitations, the current study only focuses on the analysis of intensity-latency functions but does not relate the findings to postoperative speech reception. This should be part of future research especially with respect to the prediction of the patient's dynamic range, which—besides coupling efficiency—significantly influences speech reception (50, 51). The findings should also be complemented by measuring the patient's individual dynamic range behaviorally by loudness scaling methods.
Conclusion
In conclusion, the data show that VSB evoked wave-V intensity-latency function slopes were similar to acoustic stimulation at high stimulation levels. Absolute latencies were longer compared to acoustic stimulation, most likely caused by a signal processing delay in the VSB audio processor. Saturation of latencies occurred for higher stimulation levels due to saturation of audio processor gain. Thus, the analysis of VSB evoked intensity-latency functions can be useful for the objective assessment of a patient's individual dynamic range with the AMEI. Wave-V latencies were found to be longer for Incus and OW placement of the FMT compared to RW placement, i.e., reverse stimulation, showing differences in activation of the auditory system for different coupling modalities.
In summary, the analysis of VSB evoked ABR wave-V intensity-latency functions likely enables the objective quantification of coupling efficiency by the response threshold LABR, providing a patient with the maximum possible individual dynamic range with the AMEI. In addition, it may become a useful tool for the objective assessment of the patient's individual dynamic range, the comparison of different coupling modalities and continuous postoperative quality control.
Data Availability Statement
The datasets generated for this study are available on request to the corresponding author.
Ethics Statement
The studies involving human participants were reviewed and approved by Ethical Committee of the Medical Faculty of the Martin Luther University Halle-Wittenberg. The patients/participants provided their written informed consent to participate in this study.
Author Contributions
LF: conceptualization, methodology, formal analysis, investigation, and writing—original draft. TR: conceptualization, methodology, formal analysis, investigation, writing—original draft, and review and editing. PM-S and SP: surgeries. AM, MK, PM-S, SP, OD, and TO: conceptualization, methodology, investigation, and writing—review and editing. All authors contributed to the article and approved the submitted version.
Conflict of Interest
LF, TR, SP, AM, OD, and TO received travel expenses from MED-EL (Innsbruck, Austria). SP received speaker honorarium on bone conduction hearing devices (including a different product from MED-EL, Innsbruck) and conducted contract research for MED-EL, Innsbruck, on cochlear implants.
The remaining authors declare that the research was conducted in the absence of any commercial or financial relationships that could be construed as a potential conflict of interest.
Publisher's Note
All claims expressed in this article are solely those of the authors and do not necessarily represent those of their affiliated organizations, or those of the publisher, the editors and the reviewers. Any product that may be evaluated in this article, or claim that may be made by its manufacturer, is not guaranteed or endorsed by the publisher.
Acknowledgments
The authors thank Hamidreza Mojallal and MED-EL for support in organizing study meetings and for providing the modified audio processors used in this study.
Abbreviations
AMEI, active middle ear implant; VSB, Vibrant Soundbridge; FMT, floating mass transducer; LP, long process (of the incus); SP, short process (of the incus); OW, oval window; RW, round window; VIB, vibrogram (thresholds); BC, bone conduction (thresholds); ASSR, auditory steady state response; CAP, compound action potential; ABR, auditory brainstem response; 3PTAcoupling, pure tone average of coupling efficiency at 1, 2, and 4 kHz; AC, air conduction; 4PTAAC, pure tone average of air conduction thresholds at 0.5, 1, 2, and 4 kHz; 4PTABC, pure tone average of bone conduction thresholds at 0.5, 1, 2, and 4 kHz; 4PTAVIB, pure tone average of vibrogram conduction thresholds at 0.5, 1, 2, and 4 kHz; LABR, VSB evoked ABR thresholds; SD, standard deviation.
References
1. Beutner D, Delb W, Frenzel H, Hoppe U, Hüttenbrink KB, Mlynski R, et al. Guideline “Implantable hearing aids” —short version: German S2k guideline of the Working Group of German-Speaking Audiologists, Neurootologists and Otologists (ADANO), of the German Society of Oto-Rhino-Laryngology, Head and Neck Surgery (DGHNO) in collaboration with the German Society of Audiology (DGA), the German Society of Phoniatrics and Pediatric Audiology (DGPP), and patient representatives. HNO. (2018) 66:71–6. doi: 10.1007/s00106-018-0533-2
2. Ball GR. The vibrant soundbridge: design and development. In: Böheim K, editor. Advances in Oto-Rhino-Laryngology. Basel: KARGER (2010). p. 1–13. doi: 10.1159/000318516
3. Labassi S, Beliaeff M, Péan V, Van de Heyning P. The Vibrant Soundbridge ® middle ear implant: a historical overview. Cochlear Implants Int. (2017) 18:314–23. doi: 10.1080/14670100.2017.1358913
4. Fisch U, Cremers WRJ, Lenarz T, Weber B, Babighian G, Uziel SS, et al. Clinical experience with the vibrant soundbridge implant device. Otol Neurotol. (2001) 22:962–72. doi: 10.1097/00129492-200111000-00042
5. Mlynski R, Dalhoff E, Heyd A, Wildenstein D, Rak K, Radeloff A, et al. Standardized active middle-ear implant coupling to the short incus process. Otol Neurotol. (2015) 36:1390–8. doi: 10.1097/MAO.0000000000000822
6. Huber AM, Mlynski R, Muller J, Dillier N, Holzmann D, Wolframm MD, et al. A new vibroplasty coupling technique as a treatment for conductive and mixed hearing losses: a report of 4 cases. Otol Neurotol. (2012) 33:613–7. doi: 10.1097/MAO.0b013e31824bae6e
7. Mlynski R, Dalhoff E, Heyd A, Wildenstein D, Hagen R, Gummer AW, et al. Reinforced active middle ear implant fixation in incus vibroplasty. Ear Hear. (2015) 36:72–81. doi: 10.1097/AUD.0000000000000078
8. Colletti V, Soli SD, Carner M, Colletti L. Treatment of mixed hearing losses via implantation of a vibratory transducer on the round window. Int J Audiol. (2006) 45:600–8. doi: 10.1080/14992020600840903
9. Bornitz M, Lasurashvili N, Neudert M, Beleites T, Zahnert T. Ankopplung aktiver Mittelohrimplantate – biomechanische Aspekte [Coupling of active middle ear implants—biomechanical aspects]. HNO. (2021) 69:464–74. doi: 10.1007/s00106-021-00994-6
10. Schraven SP, Dohr D, Weiss NM, Mlynski R, Dalhoff E. Laser-Doppler-vibrometrische Messungen an humanen Felsenbeinen [Laser Doppler vibrometric measurements on human temporal bones]. HNO. (2021) 69:491–500. doi: 10.1007/s00106-021-00995-5
11. Bornitz M, Hardtke H-J, Zahnert T. Evaluation of implantable actuators by means of a middle ear simulation model. Hear Res. (2010) 263:145–51. doi: 10.1016/j.heares.2010.02.007
12. Decraemer WF, de La Rochefoucauld O, Funnell WRJ, Olson ES. Three-dimensional vibration of the malleus and incus in the living gerbil. J Assoc Res Otolaryngol. (2014) 15:483–510. doi: 10.1007/s10162-014-0452-1
13. Gyo K, Aritomo H, Goode RL. Measurement of the ossicular vibration ratio in human temporal bones by use of a video measuring system. Acta Otolaryngol. (1987) 103:87–95. doi: 10.3109/00016488709134702
14. Schraven SP, Dalhoff E, Wildenstein D, Hagen R, Gummer AW, Mlynski R. Alternative fixation of an active middle ear implant at the short incus process. Audiol Neurotol. (2014) 19:1–11. doi: 10.1159/000354981
15. Stieger C, Rosowski JJ, Nakajima HH. Comparison of forward (ear-canal) and reverse (round-window) sound stimulation of the cochlea. Hear Res. (2013) 301:105–14. doi: 10.1016/j.heares.2012.11.005
16. Zhang J, Zou D, Tian J, Ta N, Rao Z. A comparative finite-element analysis of acoustic transmission in human cochlea during forward and reverse stimulations. Appl Acoust. (2019) 145:278–89. doi: 10.1016/j.apacoust.2018.10.023
17. Nakajima HH, Dong W, Olson ES, Rosowski JJ, Ravicz ME, Merchant SN. Evaluation of round window stimulation using the floating mass transducer by intracochlear sound pressure measurements in human temporal bones. Otol Neurotol. (2010) 31:506–11. doi: 10.1097/MAO.0b013e3181c0ea9f
18. Müller A, Mir-Salim P, Zellhuber N, Helbig R, Bloching M, Schmidt T, et al. Influence of floating-mass transducer coupling efficiency for active middle-ear implants on speech recognition. Otol Neurotol. (2017) 38:809–14. doi: 10.1097/MAO.0000000000001412
19. Verhaegen V, Mulder JJS, Noten JFP, Luijten BMA. Intraoperative auditory steady state response measurements during vibrant soundbridge middle ear implantation in patients with mixed hearing loss: preliminary results. Otol Neurotol. (2010) 31:1365–8. doi: 10.1097/MAO.0b013e3181f0c612
20. Fröhlich L, Plontke SK, Rahne T. A quantitative approach for the objective assessment of coupling efficiency for an active middle ear implant by recording auditory steady-state responses. Otol Neurotol. (2020) 41:e906–11. doi: 10.1097/MAO.0000000000002484
21. Radeloff A, Shehata-Dieler W, Rak K, Scherzed A, Tolsdorff B, Hagen R, et al. Intraoperative monitoring of active middle ear implant function in patients with normal and pathologic middle ears. Otol Neurotol. (2011) 32:104–7. doi: 10.1097/MAO.0b013e3181fcf167
22. Mandala M, Colletti L, Colletti V. Treatment of the atretic ear with round window vibrant soundbridge implantation in infants and children: electrocochleography and audiologic outcomes. Otol Neurotol. (2011) 32:1250–5. doi: 10.1097/MAO.0b013e31822e9513
23. Colletti V, Mandalà M, Colletti L. Electrocochleography in round window vibrant soundbridge implantation. Otolaryngol Neck Surg. (2012) 146:633–40. doi: 10.1177/0194599811430808
24. Cebulla M, Geiger U, Hagen R, Radeloff A. Device optimised chirp stimulus for ABR measurements with an active middle ear implant. Int J Audiol. (2018) 56:607–11. doi: 10.1080/14992027.2017.1314558
25. Geiger U, Radeloff A, Hagen R, Cebulla M. intraoperative estimation of the coupling efficiency and clinical outcomes of the vibrant soundbridge active middle ear implant using auditory brainstem response measurements. Am J Audiol. (2019) 28:553–9. doi: 10.1044/2019_AJA-18-0066
26. Fröhlich L, Rahne T, Plontke SK, Oberhoffner T, Dziemba O, Gadyuchko M, et al. Intraoperative recording of auditory brainstem responses for monitoring of floating mass transducer coupling efficacy during revision surgery - proof of concept. Otol Neurotol. (2020) 41:e168–71. doi: 10.1097/MAO.0000000000002511
27. Fröhlich L, Rahne T, Plontke SK, Oberhoffner T, Dahl R, Mlynski R, et al. Intraoperative quantification of floating mass transducer coupling quality in active middle ear implants: a multicenter study. Eur Arch Otorhinolaryngol. (2021) 278:2277–88. doi: 10.1007/s00405-020-06313-z
28. Jewett DL. Volume-conducted potentials in response to auditory stimuli as detected by averaging in the cat. Electroencephalogr Clin Neurophysiol. (1970) 28:609–18. doi: 10.1016/0013-4694(70)90203-8
29. Serpanos YC, O'Malley H, Gravel JS. The relationship between loudness intensity functions and the click-ABR wave V latency. Ear Hear. (1997) 18:409–19. doi: 10.1097/00003446-199710000-00006
30. Steinhoff HJ, Böhnke F, Janssen T. Click ABR intensity-latency characteristics in diagnosing conductive and cochlear hearing losses. Arch Otorhinolaryngol. (1988) 245:259–65. doi: 10.1007/BF00464627
31. Janssen Th, Steinhoff H-J, Böhnke F. Zusammenhang zwischen der Latenzverschiebung der Hirnstammpotentiale bei basokochleärer Schwerhörigkeit und dem Zeitverlauf der durch den Klick-Reiz ausgelösten Erregungswelle in der Kochlea. Laryngo Rhino Otol. (1989) 68:379–82. doi: 10.1055/s-2007-998358
32. Don M. Effect of click rate on the latency of auditory brain stem responses in humans. Ann Otol Rhinol Laryngol. (1977) 86:186–95. doi: 10.1177/000348947708600209
33. Schratzenstaller B, Janssen T, Arnold W. Untersuchung kochleärer Laufzeiteffekte bei Knochenleitung mittels Hirnstammpotentialen. Oto Rhino Laryngol Nova. (1994) 4:231–9. doi: 10.1159/000313130
34. Elberling C, Don M. A direct approach for the design of chirp stimuli used for the recording of auditory brainstem responses. J Acoust Soc Am. (2010) 128:2955–64. doi: 10.1121/1.3489111
35. Dau T, Wegner O, Mellert V, Kollmeier B. Auditory brainstem responses with optimized chirp signals compensating basilar-membrane dispersion. J Acoust Soc Am. (2000) 107:1530–40. doi: 10.1121/1.428438
36. Elberling C, Don M, Cebulla M, Stürzebecher E. Auditory steady-state responses to chirp stimuli based on cochlear traveling wave delay. J Acoust Soc Am. (2007) 122:2772. doi: 10.1121/1.2783985
37. Kristensen SGB, Elberling C. auditory brainstem responses to level-specific chirps in normal-hearing adults. J Am Acad Audiol. (2012) 23:712–21. doi: 10.3766/jaaa.23.9.5
38. Elberling C, Don M, Kristensen SGB. Auditory brainstem responses to chirps delivered by an insert earphone with equalized frequency response. J Acoust Soc Am. (2012) 132:EL149–54. doi: 10.1121/1.4737915
39. Elberling C, Kristensen SGB, Don M. Auditory brainstem responses to chirps delivered by different insert earphones. J Acoust Soc Am. (2012) 131:2091–100. doi: 10.1121/1.3677257
40. Hoth S. The role of auditory evoked potentials in the context of cochlear implant provision. Otol Neurotol. (2017) 38:e522–30. doi: 10.1097/MAO.0000000000001480
41. Dziemba OC. Referenzwerte elektrisch evozierter Potentiale zur objektiven Diagnostik des peripheren auditorischen Systems nach Cochlea-Implantat-Versorgung. Greifswald: Universität Greifswald (2018).
42. Verhaert N, Hofmann M, Wouters J. Transient and steady state auditory responses with direct acoustic cochlear stimulation. Ear Hear. (2015) 36:320–9. doi: 10.1097/AUD.0000000000000117
43. Skarzynski H, Olszewski L, Skarzynski PH, Lorens A, Piotrowska A, Porowski M, et al. Direct round window stimulation with the Med-El Vibrant Soundbridge: 5 years of experience using a technique without interposed fascia. Eur Arch Otorhinolaryngol. (2014) 271:477–82. doi: 10.1007/s00405-013-2432-1
44. Schraven S, Großmann W, Rak K, Shehata-Dieler W, Hagen R. Long-term stability of the active middle-ear implant with floating-mass transducer technology: a single-center study. Otol Neurotol. (2016) 37:252–66. doi: 10.1097/MAO.0000000000000943
45. Brkic FF, Riss D, Auinger A, Zoerner B, Arnoldner C, Baumgartner W-D, et al. Long-term outcome of hearing rehabilitation with an active middle ear implant. Laryngoscope. (2019) 129:477–81. doi: 10.1002/lary.27513
46. Zahnert T, Mlynski R, Löwenheim H, Beutner D, Hagen R, Ernst A, et al. Long-term outcomes of vibroplasty coupler implantations to treat mixed/conductive hearing loss. Audiol Neurotol. (2018) 23:316–25. doi: 10.1159/000495560
47. Spiegel JL, Kutsch L, Jakob M, Weiss BG, Canis M, Ihler F. Long-term stability and functional outcome of an active middle ear implant regarding different coupling sites. Otol Neurotol. (2020) 41:60–7. doi: 10.1097/MAO.0000000000002418
48. Jerger J, Hall J. Effects of age and sex on auditory brainstem response. Arch Otolaryngol Head Neck Surg. (1980) 106:387–91. doi: 10.1001/archotol.1980.00790310011003
49. Debruyne F. Influence of age and hearing loss on the latency shifts of the auditory brainstem response as a result of increased stimulus rate. Int J Audiol. (1986) 25:101–6. doi: 10.3109/00206098609078375
50. Rahne T, Plontke SK. Apparative Therapie bei kombiniertem Hörverlust: ein audiologischer Vergleich aktueller Hörsysteme. HNO. (2016) 64:91–100. doi: 10.1007/s00106-015-0087-5
51. Rahne T. Physikalisch-audiologische Grundlagen implantierbarer Hörsysteme: Über Energieübertragung, Ankopplung und Ausgangsleistung [Physical audiological principles of implantable hearing systems : about power transmission, coupling and power output]. HNO. (2021) 69:475–82. doi: 10.1007/s00106-019-00776-1
Keywords: active middle ear implant, coupling efficiency, objective measures, auditory brainstem response, latency
Citation: Fröhlich L, Müller A, Kropp MH, Mir-Salim P, Dziemba O, Oberhoffner T, Plontke SK and Rahne T (2022) Active Middle Ear Implant Evoked Auditory Brainstem Response Intensity-Latency Characteristics. Front. Neurol. 12:739906. doi: 10.3389/fneur.2021.739906
Received: 12 July 2021; Accepted: 16 December 2021;
Published: 20 January 2022.
Edited by:
Maurizio Barbara, Sapienza University of Rome, ItalyReviewed by:
Francesco Comacchio, Regional Specialized Vertigo Center Veneto Region, ItalyVedat Topsakal, Vrije University Brussel, Belgium
Copyright © 2022 Fröhlich, Müller, Kropp, Mir-Salim, Dziemba, Oberhoffner, Plontke and Rahne. This is an open-access article distributed under the terms of the Creative Commons Attribution License (CC BY). The use, distribution or reproduction in other forums is permitted, provided the original author(s) and the copyright owner(s) are credited and that the original publication in this journal is cited, in accordance with accepted academic practice. No use, distribution or reproduction is permitted which does not comply with these terms.
*Correspondence: Laura Fröhlich, bGF1cmEuZnJvZWhsaWNoJiN4MDAwNDA7dWstaGFsbGUuZGU=