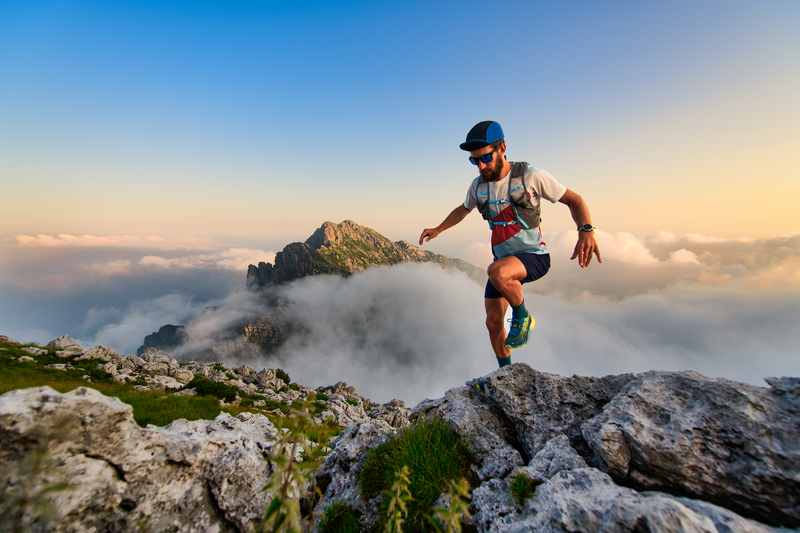
94% of researchers rate our articles as excellent or good
Learn more about the work of our research integrity team to safeguard the quality of each article we publish.
Find out more
REVIEW article
Front. Neurol. , 08 September 2021
Sec. Experimental Therapeutics
Volume 12 - 2021 | https://doi.org/10.3389/fneur.2021.680210
Paralysis or paraplegia caused by transient or permanent spinal cord ischemia–reperfusion injury (SCIRI) remains one of the most devastating post-operative complications after thoracoabdominal aortic surgery, even though perioperative strategies and surgical techniques continue to improve. Uncovering the molecular and cellular pathophysiological processes in SCIRI has become a top priority. Recently, the expression, function, and mechanism of non-coding RNAs (ncRNAs) in various diseases have drawn wide attention. Non-coding RNAs contain a variety of biological functions but do not code for proteins. Previous studies have shown that ncRNAs play a critical role in SCIRI. However, the character of ncRNAs in attenuating SCIRI has not been systematically summarized. This review article will be the first time to assemble the knowledge of ncRNAs regulating apoptosis, inflammation, autophagy, and oxidative stress to attenuate SCIRI. A better understanding of the functional significance of ncRNAs following SCIRI could help us to identify novel therapeutic targets and develop potential therapeutic strategies. All the current research about the function of nRNAs in SCIRI will be summarized one by one in this review.
Up to 45% of the reported cases of spinal cord ischemia–reperfusion injury (SCIRI) were attributed to an iatrogenic cause (1). SCIRI remains one of the major concerns for aortic surgery as a result of postoperative paraplegia, with the overall incidences of 0.5–1.5% for coarctation repair, 10% for thoracic aneurysm repair, and around 20% for thoracoabdominal aorta repair (2). Despite the dramatic progress in surgical techniques and perioperative strategies, SCIRI, which can cause some extremely devastating postoperative complications, including paralysis and paraplegia, still cannot be prevented completely. Furthermore, the incidence of postoperative neurological deficit and permanent paraplegia induced by SCIRI remains as high as 9–16% (3, 4) and 0.3–6.5%, respectively (5).
Although occurring less frequently than cerebral ischemic diseases, SCIRI results in high mortality and disability rate and reduced quality of life. In contrast to cerebral ischemic diseases, which have well-established guidelines, no consensus guideline exists for the treatment of spinal cord ischemia (6). At present, lumbar cerebrospinal fluid drainage and epidural hypothermia protocols have been widely accepted as preventative measures for SCIRI (7). Monitoring of somatosensory and motor-evoked potential during the operation has also been demonstrated to help reduce the incidence of ischemic events (8). Moreover, several studies verify that those patients whose intra-operative blood pressure was maintained above 55 mmHg had a lower rate of neurologic deficits (9, 10). Unfortunately, there are scarce clinical management experience and data of spinal cord ischemia until now. Therefore, it is urgently necessary to develop definitive and effective therapeutic approaches for SCIRI.
Since the post-genome sequencing era, there is increasing evidence to prove that non-protein-coding regions account for more than 98% of the human genome, 80% of which are transcribed into RNAs (11). For a long time, the non-coding RNAs (ncRNAs) are mistaken for the “noise” of transcription. Recently, the expression, function, and mechanism of ncRNAs have attracted wide attention. To develop more effective and promising therapeutic agents for treating SCIRI, we need to have a better understanding of how various ncRNAs mediate the pathophysiological mechanisms of SCIRI.
ncRNAs are functional molecules that originate in the genome but cannot be translated to proteins. According to molecular size, shape, and function, ncRNAs are divided into different types (Figure 1), including ribosomal RNAs (rRNAs), transfer RNAs (tRNAs), short interfering RNAs (siRNAs), piwi-interacting RNAs (piRNAs), microRNAs (miRNAs), circular RNAs (circRNAs), and long non-coding RNAs (lncRNAs) (12, 13).
Figure 1. Diagram about the RNA family. According to molecular size, shape, function, and localization, ncRNAs are divided into different types. Most of the RNAs exert regulatory functions. Only ~2% of RNAs are coding protein. circRNA, circular RNA; lncRNA, long non-coding RNA; mRNA, messenger RNA; miRNA, microRNA; piRNA, piwi-interacting RNA; rRNA, ribosome RNA; rasiRNA, repeat-associated small interfering RNA; scaRNA, small cajal body-specific RNA; siRNA, small interfering RNA; snRNA, small nuclear RNA; snoRNA, small nucleolar RNA; tRNA, transfer RNA; tiRNA, transfer RNA-derived stress-induced small RNA.
Although rRNAs and tRNAs have been introduced as translation-related RNAs for several decades, other ncRNAs were previously regarded as transcriptional “murmur,” owing to their unclear physiologic functions. However, recent studies have shown that ncRNAs are a product of pervasive transcription (13, 14) and perform many biological functions, such as transcriptional and translational regulation, epigenetic modifications, and protein and RNA scaffolding, thereby adding a new layer of genomic regulation (15–18). Therefore, a more in-depth study of ncRNAs could help us to better understand the pathophysiology of humans (19).
miRNAs are a class of highly conserved non-coding RNAs with a length of about 17 to 22 nucleotides. miRNAs can bind to the 3′-untranslated region (3′-UTR) of target messenger RNA (mRNA) through complementary base pairing. This interaction allows the inhibition of their target mRNA and the negative regulation of gene expression after transcription (20). Each miRNA can bind to various target genes, while one same gene can also be conducted with several miRNAs (21). It has been demonstrated that miRNAs are ubiquitous in the human body, which have been associated with inflammation, tumor, and various diseases (22).
piRNAs are regarded as another large class of ncRNAs, with a length of 24 to 31 nucleotides and a lack of sequence conservation between species (23). Although their functional roles and method of biogenesis remain poorly understood, piRNAs can maintain genome integrity and prevent mutations through controlling the post-transcriptional silencing of retrotransposons (24).
lncRNAs are another type of RNA with a length longer than 200 nucleotides. So far, more than 3,000 lncRNAs have been identified, of which one-third are involved in various biological processes, such as cell growth, differentiation, apoptosis, and proliferation (25–28). The mechanism of lncRNA regulation at the transcriptional and post-transcriptional levels is, respectively, through the regulation and modification of chromosomes, resulting in changes in gene expression, and participation in RNA degradation as a source of competition for endogenous RNA and miRNA. Given their display of a wide variety of biological functions, lncRNAs have recently become a research hotspot (29).
circRNAs are a subclass of lncRNAs which have a covalently closed cyclic structure and lack a polyadenylated tail. Compared with linear RNA, circRNAs are more stable and resistant to RNA exonuclease by reason of their covalently closed loop structure (30). Recent studies have suggested that circRNAs are involved in regulating gene expression by acting as an RNA binding sponge, miRNA protein sponge, and translational regulator (31, 32). Therefore, circRNAs have great potential to become another promising and effective therapeutic target.
SCIRI usually occurs during thoracoabdominal aortic surgery and cardiac surgery, which can cause some unpredictable and extremely devastating complications, including paralysis and paraplegia. Two distinct phases of SCIRI influence the occurrence of disability. The first phase is ischemia, which occurs during a period of circulatory arrest or aortic cross-clamping. The second one is reperfusion, which results in intracellular calcium overload, the release of oxygen-free radicals, inflammatory response, and neuronal apoptosis (33, 34).
In contrast to cerebral ischemic diseases, which have well-established guidelines, no consensus guideline exists for the treatment of SCIRI (6). The current strategies in our clinical setting (including hypothermia, distal aortic perfusion, and cerebrospinal fluid drainage) fail to effectively mediate spinal cord protection against ischemia–reperfusion injury (IRI) due to their inherent limitation (35). Therefore, a more effective strategy should be developed to attenuate SCIRI. Mounting evidence suggested that many ncRNAs played critical roles in the pathophysiology of SCIRI (Figure 2). Hence, to design more innovative and effective therapeutic agents for treating SCIRI, we should better understand the function of various ncRNAs mediating the pathophysiological mechanisms underlying SCIRI.
Figure 2. Many miRNAs contribute to the pathophysiology of spinal cord ischemia-reperfusion injury. Mounting evidence has suggested that various miRNAs contribute to the pathophysiology of spinal cord ischemia-reperfusion injury by mediating apoptosis, autophagy, inflammation, relieving central cord edema, and ischemic preconditioning. miRNA, microRNA.
Considered as a major breakthrough, miRNAs are regarded as therapeutic targets for SCIRI for at least two reasons: (1) a single miRNA can regulate various target genes and affect the whole gene network and (2) miRNAs can be efficiently targeted by several tools to elevate the levels of miRNAs with beneficial functions or reduce the levels of pathogenic miRNAs (36) (Table 1).
Apoptosis can lead to tissue loss in ischemic lesions. Various research have confirmed the character of miRNAs in ameliorating neuronal apoptosis after spinal cord ischemia. He and his colleagues reported that the overexpression of miRNA-21 exerted neuroprotective effects in a rodent model of SCIRI, possibly via the downregulated expressions of Fas ligand, programmed cell death 4, and caspase-3 (38). Su and his team demonstrated that naive astrocytes were transformed into A2s in vivo by ischemia. The in vitro silencing of miR-21 can transform neurotoxic reactive astrocyte A1 into A2 and promote synapsis formation and nerites growth by targeting Gpc6 and GDNF through the STAT3 signaling pathway after oxygen-glucose deprivation (OGD) (39). Li and his co-workers showed that miRNA-124-3p in exosomes derived from bone marrow mesenchymal stem cell ameliorates nerve injury and tissue impairment and impedes cell apoptosis in a SCIRI rat model by reducing the expression of Ern1 and augmenting the polarization of M2 macrophages (47).
Another study found that, in SCIRI rats and hypoxic nerve cells, miR-136 decreased, and tissue inhibitor of metalloproteinase-3 (TIMP3) increased. Cells (AGE1.NH and PC12 cell lines) transfected with miR-136 mimic protected neurocytes against hypoxia, while cells co-transfected with pcDNA- TIMP3 abrogated the results induced by the overexpression of miR-136 (50). miR-199a-5p was also identified as apoptosis-related miRNA, which decreased in the rat spinal cord following IRI and targeted endothelin-converting enzyme 1 (ECE1). Upregulating miR-199a-5p might protect the spinal cord against IRI by negatively regulating ECE1 (11).
B-cell lymphoma-2 (Bcl2), a well-known anti-apoptotic protein, was confirmed to be negatively targeted and regulated by miR-204 in neuronal cells exposed to hypoxia. In the neuronal lines (AGE1.HN and PC12) induced by hypoxia, the expression of Bcl2 in neurocytes was lower than in the control group, while miR-204 expression was higher. Bcl-2 expression was enhanced by miR-204 inhibitor and inhibited by miR-204 mimic (53). A similar study showed that hypoxia significantly increased the apoptosis rate and downregulated the expression of RAP2C. Moreover, miR-204 could bind to the 3′-UTR of RAP2C. miR-204 inhibitors elevated the activity and expression of RAP2C in the neuronal lines (AGE1.HN and PC12) subjected to hypoxia, while miR-204 mimics downregulated the activity and expression of RAP2C. The miR-204 inhibitor and the overexpression of RAP2C could attenuate apoptosis in the cells treated with hypoxia (54).
Foxd3 upregulated miR-214 which can inhibit the expression of Kcnk2, thus resulting in reduced viability and increased apoptosis of PC12 cells under hypoxia/reoxygenation conditions. However, the overexpression of Kcnk2 or knockdown of Foxd3 enhanced the cell viability and inhibited the apoptosis of the hypoxia/reoxygenation-treated PC12 cells (56).
Hsp20 is a member of the small heat-shock protein family performing many physiologic functions, including anti-apoptosis effects (64–66), the regulation of protein aggregation, and the maintenance of the cellular cytoskeleton. Inhibiting miR-320 could protect the spinal cord against IRI in vivo by dramatically upregulating the expression of phospho-Hsp20 (58).
Recent studies have demonstrated that inflammation involves the pathological mechanism of IRI (67, 68). Proinflammatory cytokines have been implicated in damage to blood–spinal cord barrier (BSCB) integrity (69, 70). The BSCB consists of continuous capillary endothelial cells, surrounded by astrocytes, pericytes as well as perivascular microglia (71). During SCIRI, the microglia are widely introduced around the injury site as the primary responding immune cell. The activated microglia can aggravate ischemic nerve damage via activating phagocytes or secreting inflammatory cytokines (72).
In the OGD-stimulated microglial cells, Na et al. found that dexmedetomidine (DEX) led to the inactivation of microglial cells by upregulating the let-7a-1-3p and let-7a-2-3p expression. High-mobility group box-1 (HMGB1) was targeted and negatively mediated by let-7a-1-3p and let-7a-2-3p. An intraperitoneal injection of DEX improved the motion function of mice in a SCIRI model, mediated by the let-7a-1/2-3p/HMGB1 pathway (37).
Recently, miR-22-3p has been found to be downregulated in rat models of SCIRI. Increased miR-22-3p can facilitate the M2 polarization of macrophages, relieve inflammation, and reduce the degree of severity of SCIRI by repressing IRF5 (40). In contrast, another study found that miR-22-3p was persistently overexpressed in both 24- and 48-h groups by bioinformatics analysis and qRT-PCT (41).
An intrathecal infusion of the miR-27a mimics attenuated inflammatory damage to the BSCB in a rat model after SCIRI by negatively regulating the Toll-like receptor adaptor molecule 2 of the Toll-like receptor 4 (TLR4) signaling pathway and inhibiting the NF-κB/IL-1β pathway, whereas pretreatment with miR-27a anti-miR oligonucleotide significantly aggravated the inflammatory injuries (43).
Sirtuin 1 (SIRT1) acts as an essential role in inflammation submitted to IRI (73). Wang et al. showed that the depletion of miR-30c protected against inflammation and cell apoptosis by increasing the SIRT1 level in OGD-treated PC12 cells and SCIRI-treated rats (44). Another study found that the miR-125b expression was markedly downregulated at 12 h after SCIRI. Treatment with miR-125b mimic before ischemia decreased the expression of tumor protein 53-induced nuclear protein 1, p53, cytokines IL-1β, TNF-α, and cleaved caspase 3, thereby protecting the neurons against neuroinflammation, whereas the miR-125b inhibitor did not have the effects as mentioned above (48). Moreover, pretreatment with miR-129-5p mimic by intrathecal administration protected the neurons and BSCB against inflammatory responses after transient SCIRI by downregulating HMGB1 expression and inhibiting Toll-like receptor-3 (TLR3)-cytokine pathway activation, but rHMGB1 and TLR3 agonist Poly (I:C) reversed these effects (49).
miR-186-5p was found to be downregulated in the SCIRI rat model. Pre-treatment with the miR-186-5p mimic improved the neurological function, attenuated BSCB leakage and spinal cord edema, and decreased IL-15, IL-6, IL-1β, TNF-α, Wnt5a, TLR3, and chemokine ligand 13 expressions (52).
Intriguingly, in OGD-AGE1.HN and SY-SH-5Y cells, the inflammation and cell apoptosis were enhanced by miR-221 knockdown and attenuated by miR-221 overexpression via negatively regulating TNF-α induced protein 2 (57). Chen et al. found that the serum TNF-α expression was significantly elevated in patients who suffered from SCIRI. TNF-α treatment caused cell apoptosis in vitro (AGE1.HN and SY-SH-5Y), whereas this effect was reversed by hydrogen sulfide (H2S). H2S suppressed tumor necrosis factor receptor type 1-associated DEATH domain protein expression via promoted miR-485-5p expression (62). miR-448 was another miRNA reported as mediating SIRT1 to improve neurological function and inhibit the apoptosis of nerve cells. Downregulated miR-448 could contribute to relieving SCIRI through upregulating SIRT1 in SCIRI tissue and hypoxia-treated cells (61).
Autophagy, as the name implies, is that process wherein autophagosomes deliver the cargo they engulfed in the cytoplasm to lysosomes (74, 75). Autophagosomes can engulf cellular material in bulk and remove damaged organelles and unwanted cytosolic proteins (76).
Li and his team found that miR-30c downregulated the expression of Beclin-1 in SY-SH-5Y cells subjected to OGD, and H2S protected the spinal cord and induced autophagy via downregulating miR-30c expression and upregulating Beclin-1 and LC3II expression in the rat model of SCIRI. However, the administration of 3-methyladenine (3-MA), an inhibitor for autophagy, could reverse the spinal cord-protective effect of H2S (45). Yan et al. found that miR-204 was also an autophagy-related gene. The transient ischemia of spinal cords enhanced the ratio of LC3-II/LC3-I and reduced Bcl-2. AntagomiR-204 significantly reduced the level of caspase-3 and dramatically upregulated the expressions of Beclin-1, LC3-II/ LC3-I ratio, and Bcl-2 in spinal cords after reperfusion. 3-MA diminished the protective effect on the hind-limb motor function of antagomiR-204 (55). The miR-372 is another autophagy-related miRNA. Interference of this particular miRNA could reduce nerve cell apoptosis in SCIRI via increasing Beclin-1-mediated autophagy (60).
Recently, it has been found that mitophagy is a selective autophagic process for the mitochondria. In response to metabolic demand, mitophagy can regulate mitochondrial numbers and eliminate damaged mitochondria (77). In a SCIRI rat model, treatment with antagomiR-124 enhanced the expressions of mitochondrial Becline-1, LC3-II, and inhibitory member of the apoptosis-stimulating proteins of the p53 family in spinal cords. Inhibiting miRNA-124 may exert neuroprotection on the spinal cord by inducing mitophagy and anti-apoptotic effects. However, the administration of 3-MA could abrogate the neuroprotective effects of antagomiR-124 (46).
Reactive oxygen species (ROS) are another major mediator of the neurologic injury submitted to SCIRI. The sole purpose of NADPH oxidase (NOX) is to produce ROS (78). To date, there are seven known NOX isoforms, of which NOX4 is strongly associated with cerebral ischemia. Because of the organ-specific effect of NOX4 in neurons and blood–brain barrier endothelial cells, NOX4 is highly sensitive to cerebral ischemic damage (79).
Zhao and his colleagues found that pretreatment with miRNA-25-enriched exosomes by intrathecal injection diminished NOX4 expression in SCIRI rats, elevated superoxide dismutase activity, and decreased the levels of TNF-α, IL-1b, and malondialdehyde content in spinal cords, thus giving better neuroprotective effects (42).
Ischemic preconditioning (IPC) has protective effects against IRI in different organs. However, the mechanisms underlying the protective effects of IPC are still poorly understood. Recently, Ueno et al. demonstrated that miRNA-762 and miR-3072-5p were downregulated in the rat after remote IPC compared with those in sham-operated control and probably bound to the 3′-UTR of vascular endothelial growth factor (VEGF) mRNA. Anti-miR-762 and anti-miR-3072-5p can induce CD34-positive BM cells to produce a higher level of plasma VEGF to protect spinal cord neurons against ischemia (63).
The integrity of BSCB is crucial to the spinal cord. On the contrary, the breakdown of BSCB allows circulating immune cells and exogenous pathogens to infiltrate the central nervous system, which can lead to disastrous consequences, such as central sensitization, neuronal loss, and glial remodeling (80, 81).
Major facilitator superfamily domain-containing 2a (Mfsd2a) is implicated in the establishment of a functional blood–brain barrier and the maintenance of its integrity, and Mfsd2a−/− mice present with blood–brain barrier leakage due to increased transcellular trafficking across the endothelial cytoplasm (82). miR-155 ablation slowed the progression of central cord edema and reduced the incidence of paralysis. miR-155 was found to negatively regulate Mfsd2a in vitro (51). Li et al. reported that pretreatment with miR-320a mimic by intrathecal infusion attenuated IRI-induced lower-limb motor function deficits and BSCB dysfunction in SCIRI rats through downregulating the expression of AQP1 (59).
Recent evidence has indicated that many lncRNAs are differentially expressed in SCIRI and may act as a significant part of the pathophysiology of SCIRI. Zhou et al. analyzed the expression pattern of lncRNAs in the SCIRI rat model using high-throughput RNA sequencing, suggesting that 6,707 mRNAs and 1,455 lncRNAs were differentially expressed, including 761 lncRNAs with increased expression and 694 with decreased expression (83). Furthermore, another study profiled the expression pattern of lncRNAs using a similar method and indicated that 7,980 lncRNAs (234 upregulated and 7,746 downregulated) and 3,428 mRNAs were differentially expressed (84). A bioinformatics analysis showed that those differentially expressed lncRNAs and mRNAs were associated with many biological pathways and processes, which might be crucial to provide new insight into the identification of potential therapeutic targets for SCIRI (83).
lncRNA CasC7 is about 9.3 kb in size, whose full name is cancer susceptibility candidate 7 (85). van Heesch and his team revealed that CasC7 exists in the nucleus and cytoplasm. When CasC7 exists in the cytoplasm, it will react with the large cytoplasmic polyribosome complex and participate in translation regulation (86).
It has been demonstrated that hydrogen sulfide (NaSH) preprocessing can decrease the spinal cord infarct zone in a SCIRI rat model and reduce the apoptosis rate in the SH5Y-SY cells treated with OGD/R. CasC7 expression was diminished and miR-30c was enhanced in vitro and in vivo, respectively. NaSH preprocessing can augment the level of CasC7 and inhibit the expression of miR-30c in SH5Y-SY cells treated with OGD/R. Furthermore, knockdown of CasC7 results in increased expression of Mir-30c and promotes apoptosis in this cell model (87).
Therefore, lncRNA CasC7 could upregulate the level of autophagy, protect against cell apoptosis and inflammation via inhibiting miR-30c, and increase SIRT1 expression in vivo and in vitro (44, 45, 87).
MALAT1, which exists in various species, is the first lncRNA identified to promote the proliferation and metastasis of various cancers through alternative splicing and gene expression (88–90). MALAT1 is an abundant, quite conservative, and stable lncRNA (~7 kb) (91). MALAT1 is expressed in the skeletal muscle, vascular endothelial cells, and cardiomyocytes, which can be regarded to be involved in pathological myogenesis and angiogenesis (92–95).
Qiao et al. confirmed that MALAT1 exerted a neuroprotective effect in neurocyte lines under hypoxic conditions and the rat model of SCIRI by regulating miR-204. In vivo and in vitro experiments indicated that the expression of MALAT1 and Bcl-2 was reduced, while the expression of miR-204 was enhanced. Furthermore, overexpression of MALAT1 induced anti-apoptosis, and knockdown induced pro-apoptosis. However, the anti-apoptosis and pro-apoptosis effects can be reversed by synchronous overexpression and knockdown of miR-204, respectively. Moreover, SCIRI rats treated with MALAT1 exhibited higher levels of Bcl-2 expression, lower miR-204 expression, and lower Motor Deficit Index scores (96).
Therefore, overexpression of MALAT1 could decrease the expressions of miR-204, increase the expressions of Bcl-2, Beclin-1, the LC3-II/ LC3-I ratio, and RAP2C to attenuate apoptosis in the cells treated with hypoxia, and exert spinal cord protection against IRI (53–55, 96).
TUG1 is firstly identified in a genomic screen, which takes part in the development of the retina. In response to taurine, developing retinal cells can enhance the expression of TUG1 (97). The abnormal expression of TUG1 is strongly associated with different kinds of tumors (98, 99), myocardial ischemia (100), and neurodegenerative diseases (101).
The TLR4-mediated spinal microglial activation and NF-κB/IL-1β signaling pathway were involved in SCIRI, which initiated neuro-inflammation and neuro-apoptosis. Inhibiting TLR4 reduced the release of inflammatory cytokines after SCIRI (69). TLR4 interactor with leucine-rich repeats (TRIL), a receptor accessory protein of TLR4, significantly regulated the activation of TLR4 and the release of its downstream inflammatory cytokine IL-1b (102).
The mRNA levels of TUG1 and the expression of NF-κB/IL-1b signaling pathway were upregulated after SCIRI in rats. Activated microglia aggravated the neurological impairment and BSCB leakage after IRI. However, the knockdown of TUG1 can inhibit the inflammatory damage of IRI through decreasing the TRIL expression. Contrarily, the overexpression of TRIL can reverse the restraint of inflammatory effect. This study suggested that the regulation of TUG1 and TRIL may be a promising treatment strategy for SCIRI (103).
Despite the dramatic progress in surgical techniques and perioperative strategies of thoracoabdominal aortic aneurysm repair, the prevention of SCIRI remains a challenge for cardiovascular surgeons. An increasing number of studies indicated that ncRNAs play critical parts in neurological dysfunction after SCIRI and may pave the way to develop more novel molecular therapeutic options.
This review article is the first time to assemble the knowledge of ncRNAs regulating apoptosis, inflammation, autophagy, oxidative stress, or other processes to attenuate SCIRI. In fact, numerous research focused on the relationship between miRNAs and SCIRI in the last decades, while the functions of other ncRNAs in SCIRI need to be fully investigated in the future. lncRNAs, the emerging star ncRNAs, should become the focus of research in the field of SCIRI for the next decade.
The translation of ncRNA research into clinical practice is a fascinating step. Even though miRNAs have “off-target” effects, they are ideal candidates for pre-clinical experimental research being highly conserved among species. Contrarily, in the ncRNA field, lncRNA, and circRNA are relatively new players. The poor conservation of nucleotide sequence between species and the presence of several transcripts are two major obstacles for scientists to clarify the specific signaling mechanism of lncRNA. With the ability to resist RNA exonucleases, circRNAs have great potential to become a biomarker for disease diagnosis.
At present, the ncRNA-based therapy aims to restore dysregulated expression levels of ncRNA to their basic level. Animal model experiments in vivo revealed that an intrathecal injection of mimic and exosome can effectively upregulate ncRNA. siRNA, shRNA, oligonucleotide, and inhibitor can downregulate ncRNA under the same operation. The exciting possibilities and enthusiasm of regulating ncRNAs to intervene in an SCIRI animal model can be tempered by some harsh realities. These therapeutic strategies are still far from our clinical setting. The disadvantages associated with regulating ncRNAs to treat SCIRI can be listed as follows: First, modifying cellular processes and molecular pathways to prevent SCIRI may not be as simple as expected around the bedside. Inferences drawn from experimental animal models may not successfully carry over to human systems. Second, modulating the expression of miRNAs may have undesirable side effects and unexpected consequences, which may have not been found in a short-term animal model. The regulation of ncRNA can promote a protumor environment (104–108).
Even though an increasing number of research results emphasized the promising therapeutic effects of ncRNAs, the wide clinic application of ncRNA-targeting molecules in SCIRI still has a long way to go.
XL, PZhou, SZ, and PZhu contributed to the conception and design of this review. XL, JL, and JY searched the literature and wrote the manuscript. XL, HQ, and XZ created the figure and table, and participated in drafting the manuscript. PZhou, SZ, and PZhu revised the manuscript. All the authors read and approved the final manuscript.
This research was supported by grants from the National Natural Science Foundation of China (82170274 and 82100410), Guangzhou Science and Technology Planning Project (Grant No. 201804010067 and 2017ZC0064), and the President Foundation of Nanfang Hospital, Southern Medical University (Grant No. 2019c030 and 2017B022).
The authors declare that the research was conducted in the absence of any commercial or financial relationships that could be construed as a potential conflict of interest.
All claims expressed in this article are solely those of the authors and do not necessarily represent those of their affiliated organizations, or those of the publisher, the editors and the reviewers. Any product that may be evaluated in this article, or claim that may be made by its manufacturer, is not guaranteed or endorsed by the publisher.
12/15-LOX, 12/15-lipoxygenase; 3-MA, 3-methyladenine; 3′-UTR, 3′-untranslated region; Bcl2, B-cell lymphoma-2; BSCB, blood–spinal cord barrier; circRNAs, circular RNAs; CSF, cerebrospinal fluid; dEX, dexmedetomidine; ECE1, endothelin-converting enzyme 1; FosDT, Fos downstream transcript; GLT-1, glutamate transporter-1; HMGB1, high-mobility group box-1; H2S, hydrogen sulfide; iASPP, inhibitory member of the apoptosis-stimulating proteins of p53 family; IPC, ischemic preconditioning; IRI, ischemia–reperfusion injury; lncRNAs, long non-coding RNAs; lncRNA CasC7, long non-coding RNA cancer susceptibility candidate 7; MALAT1, metastasis-associated lung adenocarcinoma transcript 1; MCAO, middle cerebral artery occlusion; Mfsd2a, major facilitator superfamily domain-containing 2a; miRNAs, microRNAs; mRNA, messenger RNA; NaSH, hydrogen sulfide; ncRNAs, non-coding RNAs; NOX, NADPH oxidase; OGD, oxygen-glucose deprivation; OGD/R, oxygen-glucose deprivation/reoxygenation; piRNAs, piwi-interacting RNAs; ROS, reactive oxygen species; rRNAs, ribosomal RNAs; SCIRI, spinal cord ischemia–reperfusion injury; siRNAs, short interfering RNAs; SIRT1, sirtuin 1; SNHG14, small nucleolar RNA host gene 14; TIMP3, tissue inhibitor of metalloproteinases-3; TLR3, Toll-like receptor-3; TLR4, Toll-like receptor-4; TNF, tumor necrosis factor; TNF-α, tumor necrosis factor-α; TRIL, TLR4 interactor with leucine-rich repeats; tRNAs, transfer RNAs; TUG1, taurine-upregulated gene 1; VEGF, vascular endothelial growth factor.
1. Robertson CE, Brown RD Jr, Wijdicks EF, Rabinstein AA. Recovery after spinal cord infarcts: long-term outcome in 115 patients. Neurology. (2012) 78:114–21. doi: 10.1212/WNL.0b013e31823efc93
2. Acher C, Wynn M. Outcomes in open repair of the thoracic and thoracoabdominal aorta. J Vasc Surg. (2010) 52 (4 Suppl):3S−9S. doi: 10.1016/j.jvs.2010.06.137
3. Bisdas T, Panuccio G, Sugimoto M, Torsello G, Austermann M. Risk factors for spinal cord ischemia after endovascular repair of thoracoabdominal aortic aneurysms. J Vasc Surg. (2015) 61:1408–16. doi: 10.1016/j.jvs.2015.01.044
4. Verhoeven EL, Katsargyris A, Bekkema F, Oikonomou K, Zeebregts CJ, Ritter W, et al. Editor's choice - ten-year experience with endovascular repair of thoracoabdominal aortic aneurysms: results from 166 consecutive patients. Eur J Vasc Endovasc Surg. (2015) 49:524–31. doi: 10.1016/j.ejvs.2014.11.018
5. Estrera AL, Miller CC 3rd, Chen EP, Meada R, Torres RH, Porat EE, et al. Descending thoracic aortic aneurysm repair: 12-year experience using distal aortic perfusion and cerebrospinal fluid drainage. Ann Thorac Surg. (2005) 80:1290–6; discussion 1296. doi: 10.1016/j.athoracsur.2005.02.021
6. Powers WJ, Rabinstein AA, Ackerson T, Adeoye OM, Bambakidis NC, Becker K, et al. 2018 guidelines for the early management of patients with acute ischemic stroke: a guideline for healthcare professionals from the American heart association/american stroke association. Stroke. (2018) 49:e46–110. doi: 10.1161/STR.0000000000000158
7. Hnath JC, Mehta M, Taggert JB, Sternbach Y, Roddy SP, Kreienberg PB, et al. Strategies to improve spinal cord ischemia in endovascular thoracic aortic repair: Outcomes of a prospective cerebrospinal fluid drainage protocol. J Vasc Surg. (2008) 48:836–40. doi: 10.1016/j.jvs.2008.05.073
8. Shine TS, Harrison BA, De Ruyter ML, Crook JE, Heckman M, Daube JR, et al. Motor and somatosensory evoked potentials: their role in predicting spinal cord ischemia in patients undergoing thoracoabdominal aortic aneurysm repair with regional lumbar epidural cooling. Anesthesiology. (2008) 108:580–7. doi: 10.1097/ALN.0b013e318168d921
9. Lynch DR, Dawson TM, Raps EC, Galetta SL. Risk factors for the neurologic complications associated with aortic aneurysms. Arch Neurol. (1992) 49:284–8. doi: 10.1001/archneur.1992.00530270098025
10. Sandhu HK, Evans JD, Tanaka A, Atay S, Afifi RO, Charlton-Ouw KM, et al. Fluctuations in spinal cord perfusion pressure: a harbinger of delayed paraplegia after thoracoabdominal aortic repair. Semin Thorac Cardiovasc Surg. (2017) 29:451–9. doi: 10.1053/j.semtcvs.2017.05.007
11. Bao N, Fang B, Lv H, Jiang Y, Chen F, Wang Z, et al. Upregulation of miR-199a-5p protects spinal cord against ischemia/reperfusion-induced injury via downregulation of ECE1 in rat. Cell Mol Neurobiol. (2018) 38:1293–303. doi: 10.1007/s10571-018-0597-2
12. Dong Y, Xu W, Liu C, Liu P, Li P, Wang K. Reactive oxygen species related noncoding RNAs as regulators of cardiovascular diseases. Int J Biol Sci. (2019) 15:680–7. doi: 10.7150/ijbs.30464
13. Wade JT, Grainger DC. Pervasive transcription: illuminating the dark matter of bacterial transcriptomes. Nat Rev Microbiol. (2014) 12:647–53. doi: 10.1038/nrmicro3316
14. Consortium EP, Birney E, Stamatoyannopoulos JA, Dutta A, Guigo R, Gingeras TR, et al. Identification and analysis of functional elements in 1% of the human genome by the ENCODE pilot project. Nature. (2007) 447:799–816. doi: 10.1038/nature05874
15. Schmitz SU, Grote P, Herrmann BG. Mechanisms of long noncoding RNA function in development and disease. Cell Mol Life Sci. (2016) 73:2491–509. doi: 10.1007/s00018-016-2174-5
16. Peschansky VJ, Wahlestedt C. Non-coding RNAs as direct and indirect modulators of epigenetic regulation. Epigenetics. (2014) 9:3–12. doi: 10.4161/epi.27473
17. Rinn JL, Chang HY. Genome regulation by long noncoding RNAs. Annu Rev Biochem. (2012) 81:145–66. doi: 10.1146/annurev-biochem-051410-092902
18. Qureshi IA, Mehler MF. Emerging roles of non-coding RNAs in brain evolution, development, plasticity and disease. Nat Rev Neurosci. (2012) 13:528–41. doi: 10.1038/nrn3234
19. Denham J, Gray A, Scott-Hamilton J, Hagstrom AD. Sprint interval training decreases circulating MicroRNAs important for muscle development. Int J Sports Med. (2018) 39:67–72. doi: 10.1055/s-0043-120763
20. Kim VN. MicroRNA biogenesis: coordinated cropping and dicing. Nat Rev Mol Cell Biol. (2005) 6:376–85. doi: 10.1038/nrm1644
21. Zhang S, Chen N. Regulatory role of MicroRNAs in muscle atrophy during exercise intervention. Int J Mol Sci. (2018) 19:405. doi: 10.3390/ijms19020405
22. Duenas A, Exposito A, Aranega A, Franco D. The role of non-coding RNA in congenital heart diseases. J Cardiovasc Dev Dis. (2019) 6:15. doi: 10.3390/jcdd6020015
23. O'Donnell KA, Boeke JD. Mighty piwis defend the germline against genome intruders. Cell. (2007) 129:37–44. doi: 10.1016/j.cell.2007.03.028
24. Chandran R, Mehta SL, Vemuganti R. Non-coding RNAs and neuroprotection after acute CNS injuries. Neurochem Int. (2017) 111:12–22. doi: 10.1016/j.neuint.2017.01.015
25. Yamamoto T, Saitoh N. Non-coding RNAs and chromatin domains. Curr Opin Cell Biol. (2019) 58:26–33. doi: 10.1016/j.ceb.2018.12.005
26. Rynkeviciene R, Simiene J, Strainiene E, Stankevicius V, Usinskiene J, Miseikyte Kaubriene E, et al. Non-Coding RNAs in glioma. Cancers. (2018) 11:17. doi: 10.3390/cancers11010017
27. Saeedi Borujeni MJ, Esfandiary E, Baradaran A, Valiani A, Ghanadian M, Codoner-Franch P, et al. Molecular aspects of pancreatic beta-cell dysfunction: oxidative stress, microRNA, and long noncoding RNA. J Cell Physiol. (2019) 234:8411–25. doi: 10.1002/jcp.27755
28. Zhao CX, Zhu W, Ba ZQ, Xu HJ, Liu WD, Zhu B, et al. The regulatory network of nasopharyngeal carcinoma metastasis with a focus on EBV, lncRNAs and miRNAs. Am J Cancer Res. (2018) 8:2185–209.
29. Gomes CPC, de Gonzalo-Calvo D, Toro R, Fernandes T, Theisen D, Wang DZ, et al. Non-coding RNAs and exercise: pathophysiological role and clinical application in the cardiovascular system. Clin Sci. (2018) 132:925–42. doi: 10.1042/CS20171463
30. Rong D, Sun H, Li Z, Liu S, Dong C, Fu K, et al. An emerging function of circRNA-miRNAs-mRNA axis in human diseases. Oncotarget. (2017) 8:73271–81. doi: 10.18632/oncotarget.19154
31. Zhang Z, Yang T, Xiao J. Circular RNAs: promising biomarkers for human diseases. EBioMedicine. (2018) 34:267–74. doi: 10.1016/j.ebiom.2018.07.036
32. Sapp RM, Shill DD, Roth SM, Hagberg JM. Circulating microRNAs in acute and chronic exercise: more than mere biomarkers. J Appl Physiol. (2017) 122:702–17. doi: 10.1152/japplphysiol.00982.2016
33. Yu D, Li M, Ni B, Kong J, Zhang Z. Induction of neuronal mitophagy in acute spinal cord injury in rats. Neurotox Res. (2013) 24:512–22. doi: 10.1007/s12640-013-9397-0
34. Bell MT, Puskas F, Agoston VA, Cleveland JC Jr, Freeman KA, Gamboni F, et al. Toll-like receptor 4-dependent microglial activation mediates spinal cord ischemia-reperfusion injury. Circulation. (2013) 128 (11 Suppl. 1):S152–6. doi: 10.1161/CIRCULATIONAHA.112.000024
35. Caton MT, Huff JS. Spinal cord ischemia. In: StatPearls. Treasure Island, FL: StatPearls (2020).
36. Peek AS, Behlke MA. Design of active small interfering RNAs. Curr Opin Mol Ther. (2007) 9:110–8.
37. Na HST, Nuo M, Meng QT, Xia ZY. The pathway of Let-7a-1/2-3p and HMGB1 mediated dexmedetomidine inhibiting microglia activation in spinal cord ischemia-reperfusion injury mice. J Mol Neurosci. (2019) 69:106–14. doi: 10.1007/s12031-019-01338-4
38. He F, Ren Y, Shi E, Liu K, Yan L, Jiang X. Overexpression of microRNA-21 protects spinal cords against transient ischemia. J Thorac Cardiovasc Surg. (2016) 152:1602–8. doi: 10.1016/j.jtcvs.2016.07.065
39. Su Y, Chen Z, Du H, Liu R, Wang W, Li H, et al. Silencing miR-21 induces polarization of astrocytes to the A2 phenotype and improves the formation of synapses by targeting glypican 6 via the signal transducer and activator of transcription-3 pathway after acute ischemic spinal cord injury. FASEB J. (2019) 33:10859–71. doi: 10.1096/fj.201900743R
40. Fang H, Yang M, Pan Q, Jin HL, Li HF, Wang RR, et al. MicroRNA-22-3p alleviates spinal cord ischemia/reperfusion injury by modulating M2 macrophage polarization via IRF5. J Neurochem. (2020) 156:106–120. doi: 10.1111/jnc.15042
41. Liu ZG, Li Y, Jiao JH, Long H, Xin ZY, Yang XY. MicroRNA regulatory pattern in spinal cord ischemia-reperfusion injury. Neural Regen Res. (2020) 15:2123–30. doi: 10.4103/1673-5374.280323
42. Zhao L, Jiang X, Shi J, Gao S, Zhu Y, Gu T, et al. Exosomes derived from bone marrow mesenchymal stem cells overexpressing microRNA-25 protect spinal cords against transient ischemia. J Thorac Cardiovasc Surg. (2019) 157:508–17. doi: 10.1016/j.jtcvs.2018.07.095
43. Li XQ, Lv HW, Wang ZL, Tan WF, Fang B, Ma H. MiR-27a ameliorates inflammatory damage to the blood-spinal cord barrier after spinal cord ischemia: reperfusion injury in rats by downregulating TICAM-2 of the TLR4 signaling pathway. J Neuroinflammation. (2015) 12:25. doi: 10.1186/s12974-015-0246-3
44. Wang X, Su X, Gong F, Yin J, Sun Q, Lv Z, et al. MicroRNA-30c abrogation protects against spinal cord ischemia reperfusion injury through modulating SIRT1. Eur J Pharmacol. (2019) 851:80–7. doi: 10.1016/j.ejphar.2019.02.027
45. Li L, Jiang HK, Li YP, Guo YP. Hydrogen sulfide protects spinal cord and induces autophagy via miR-30c in a rat model of spinal cord ischemia-reperfusion injury. J Biomed Sci. (2015) 22:50. doi: 10.1186/s12929-015-0135-1
46. Liu K, Yan L, Jiang X, Yu Y, Liu H, Gu T, et al. Acquired inhibition of microRNA-124 protects against spinal cord ischemia-reperfusion injury partially through a mitophagy-dependent pathway. J Thorac Cardiovasc Surg. (2017) 154:1498–508. doi: 10.1016/j.jtcvs.2017.05.046
47. Li R, Zhao K, Ruan Q, Meng C, Yin F. Bone marrow mesenchymal stem cell-derived exosomal microRNA-124-3p attenuates neurological damage in spinal cord ischemia-reperfusion injury by downregulating Ern1 and promoting M2 macrophage polarization. Arthritis Res Ther. (2020) 22:75. doi: 10.1186/s13075-020-2146-x
48. Li XQ, Yu Q, Tan WF, Zhang ZL, Ma H. MicroRNA-125b mimic inhibits ischemia reperfusion-induced neuroinflammation and aberrant p53 apoptotic signalling activation through targeting TP53INP1. Brain Behav Immun. (2018) 74:154–5. doi: 10.1016/j.bbi.2018.09.002
49. Li XQ, Chen FS, Tan WF, Fang B, Zhang ZL, Ma H. Elevated microRNA-129-5p level ameliorates neuroinflammation and blood-spinal cord barrier damage after ischemia-reperfusion by inhibiting HMGB1 and the TLR3-cytokine pathway. J Neuroinflammation. (2017) 14:205. doi: 10.1186/s12974-017-0977-4
50. Jin R, Xu S, Lin X, Shen M. MiR-136 controls neurocytes apoptosis by regulating tissue inhibitor of metalloproteinases-3 in spinal cord ischemic injury. Biomed Pharmacother. (2017) 94:47–54. doi: 10.1016/j.biopha.2017.07.053
51. Awad H, Bratasz A, Nuovo G, Burry R, Meng X, Kelani H, et al. MiR-155 deletion reduces ischemia-induced paralysis in an aortic aneurysm repair mouse model: utility of immunohistochemistry and histopathology in understanding etiology of spinal cord paralysis. Ann Diagn Pathol. (2018) 36:12–20. doi: 10.1016/j.anndiagpath.2018.06.002
52. Chen F, Li X, Li Z, Qiang Z, Ma H. Altered expression of MiR-186-5p and its target genes after spinal cord ischemia-reperfusion injury in rats. Neurosci Lett. (2020) 718:134669. doi: 10.1016/j.neulet.2019.134669
53. Wang X, Li J, Wu D, Bu X, Qiao Y. Hypoxia promotes apoptosis of neuronal cells through hypoxia-inducible factor-1alpha-microRNA-204-B-cell lymphoma-2 pathway. Exp Biol Med. (2016) 241:177–83. doi: 10.1177/1535370215600548
54. Qiao Y, Peng C, Li J, Wu D, Wang X. Spinal cord ischemia-reperfusion causes damage of neurocyte by inhibiting RAP2C. Neurol Res. (2017) 39:877–84. doi: 10.1080/01616412.2017.1352120
55. Yan L, Shi E, Jiang X, Shi J, Gao S, Liu H. Inhibition of MicroRNA-204 conducts neuroprotection against spinal cord ischemia. Ann Thorac Surg. (2019) 107:76–83. doi: 10.1016/j.athoracsur.2018.07.082
56. Li R, Zhao K, Ruan Q, Meng C, Yin F. The transcription factor Foxd3 induces spinal cord ischemia-reperfusion injury by potentiating microRNA-214-dependent inhibition of Kcnk2. Exp Mol Med. (2020) 52:118–29. doi: 10.1038/s12276-019-0370-8
57. Zhao D, Deng SC, Ma Y, Hao YH, Jia ZH. miR-221 alleviates the inflammatory response and cell apoptosis of neuronal cell through targeting TNFAIP2 in spinal cord ischemia-reperfusion. Neuroreport. (2018) 29:655–60. doi: 10.1097/WNR.0000000000001013
58. He F, Shi E, Yan L, Li J, Jiang X. Inhibition of micro-ribonucleic acid-320 attenuates neurologic injuries after spinal cord ischemia. J Thorac Cardiovasc Surg. (2015) 150:398–406. doi: 10.1016/j.jtcvs.2015.03.066
59. Li XQ, Fang B, Tan WF, Wang ZL, Sun XJ, Zhang ZL, et al. miR-320a affects spinal cord edema through negatively regulating aquaporin-1 of blood-spinal cord barrier during bimodal stage after ischemia reperfusion injury in rats. BMC Neurosci. (2016) 17:10. doi: 10.1186/s12868-016-0243-1
60. Li X, Lou X, Xu S, Wang Q, Shen M, Miao J. Knockdown of miR-372 inhibits nerve cell apoptosis induced by spinal cord ischemia/reperfusion injury via enhancing autophagy by up-regulating beclin-1. J Mol Neurosci. (2018) 66:437–44. doi: 10.1007/s12031-018-1179-y
61. Wang Y, Pang QJ, Liu JT, Wu HH, Tao DY. Down-regulated miR-448 relieves spinal cord ischemia/reperfusion injury by up-regulating SIRT1. Braz J Med Biol Res. (2018) 51:e7319. doi: 10.1590/1414-431x20177319
62. Chen Z, Zhang Z, Zhang D, Li H, Sun Z. Hydrogen sulfide protects against TNF-alpha induced neuronal cell apoptosis through miR-485-5p/TRADD signaling. Biochem Biophys Res Commun. (2016) 478:1304–9. doi: 10.1016/j.bbrc.2016.08.116
63. Ueno K, Samura M, Nakamura T, Tanaka Y, Takeuchi Y, Kawamura D, et al. Increased plasma VEGF levels following ischemic preconditioning are associated with downregulation of miRNA-762 and miR-3072-5p. Sci Rep. (2016) 6:36758. doi: 10.1038/srep36758
64. Zeng L, Tan J, Hu Z, Lu W, Yang B. Hsp20 protects neuroblastoma cells from ischemia/reperfusion injury by inhibition of apoptosis via a mechanism that involves the mitochondrial pathways. Curr Neurovasc Res. (2010) 7:281–7. doi: 10.2174/156720210793180783
65. Bartelt-Kirbach B, Golenhofen N. Reaction of small heat-shock proteins to different kinds of cellular stress in cultured rat hippocampal neurons. Cell Stress Chaperones. (2014) 19:145–53. doi: 10.1007/s12192-013-0452-9
66. Fan GC, Ren X, Qian J, Yuan Q, Nicolaou P, Wang Y, et al. Novel cardioprotective role of a small heat-shock protein, Hsp20, against ischemia/reperfusion injury. Circulation. (2005) 111:1792–9. doi: 10.1161/01.CIR.0000160851.41872.C6
67. Matsumoto S, Matsumoto M, Yamashita A, Ohtake K, Ishida K, Morimoto Y, et al. The temporal profile of the reaction of microglia, astrocytes, and macrophages in the delayed onset paraplegia after transient spinal cord ischemia in rabbits. Anesth Analg. (2003) 96:1777–84. doi: 10.1213/01.ANE.0000064204.67561.73
68. Lu K, Cho CL, Liang CL, Chen SD, Liliang PC, Wang SY, et al. Inhibition of the MEK/ERK pathway reduces microglial activation and interleukin-1-beta expression in spinal cord ischemia/reperfusion injury in rats. J Thorac Cardiovasc Surg. (2007) 133:934–41. doi: 10.1016/j.jtcvs.2006.11.038
69. Li XQ, Wang J, Fang B, Tan WF, Ma H. Intrathecal antagonism of microglial TLR4 reduces inflammatory damage to blood-spinal cord barrier following ischemia/reperfusion injury in rats. Mol Brain. (2014) 7:28. doi: 10.1186/1756-6606-7-28
70. Li XQ, Lv HW, Tan WF, Fang B, Wang H, Ma H. Role of the TLR4 pathway in blood-spinal cord barrier dysfunction during the bimodal stage after ischemia/reperfusion injury in rats. J Neuroinflammation. (2014) 11:62. doi: 10.1186/1742-2094-11-62
71. Ni B, Cao Z, Liu Y. Glycyrrhizin protects spinal cord and reduces inflammation in spinal cord ischemia-reperfusion injury. Int J Neurosci. (2013) 123:745–51. doi: 10.3109/00207454.2013.796551
72. Anwar MA, Al Shehabi TS, Eid AH. Inflammogenesis of secondary spinal cord injury. Front Cell Neurosci. (2016) 10:98. doi: 10.3389/fncel.2016.00098
73. Pantazi E, Zaouali MA, Bejaoui M, Folch-Puy E, Ben Abdennebi H, Rosello-Catafau J. Role of sirtuins in ischemia-reperfusion injury. World J Gastroenterol. (2013) 19:7594–602. doi: 10.3748/wjg.v19.i43.7594
74. Dower CM, Wills CA, Frisch SM, Wang HG. Mechanisms and context underlying the role of autophagy in cancer metastasis. Autophagy. (2018) 14:1110–28. doi: 10.1080/15548627.2018.1450020
75. Kaur J, Debnath J. Autophagy at the crossroads of catabolism and anabolism. Nat Rev Mol Cell Biol. (2015) 16:461–72. doi: 10.1038/nrm4024
76. Mizushima N. Autophagy: process and function. Genes Dev. (2007) 21:2861–73. doi: 10.1101/gad.1599207
77. Balsam LB. Spinal cord ischemia-reperfusion injury: MicroRNAs and mitophagy at a crossroads. J Thorac Cardiovasc Surg. (2017) 154:1509–10. doi: 10.1016/j.jtcvs.2017.06.010
78. Altenhofer S, Kleikers PW, Radermacher KA, Scheurer P, Rob Hermans JJ, Schiffers P, et al. The NOX toolbox: validating the role of NADPH oxidases in physiology and disease. Cell Mol Life Sci. (2012) 69:2327–43. doi: 10.1007/s00018-012-1010-9
79. Casas AI, Geuss E, Kleikers PWM, Mencl S, Herrmann AM, Buendia I, et al. NOX4-dependent neuronal autotoxicity and BBB breakdown explain the superior sensitivity of the brain to ischemic damage. Proc Natl Acad Sci USA. (2017) 114:12315–20. doi: 10.1073/pnas.1705034114
80. Echeverry S, Shi XQ, Rivest S, Zhang J. Peripheral nerve injury alters blood-spinal cord barrier functional and molecular integrity through a selective inflammatory pathway. J Neurosci. (2011) 31:10819–28. doi: 10.1523/JNEUROSCI.1642-11.2011
81. Fang B, Li XM, Sun XJ, Bao NR, Ren XY, Lv HW, et al. Ischemic preconditioning protects against spinal cord ischemia-reperfusion injury in rabbits by attenuating blood spinal cord barrier disruption. Int J Mol Sci. (2013) 14:10343–54. doi: 10.3390/ijms140510343
82. Ben-Zvi A, Lacoste B, Kur E, Andreone BJ, Mayshar Y, Yan H, et al. Mfsd2a is critical for the formation and function of the blood-brain barrier. Nature. (2014) 509:507–11. doi: 10.1038/nature13324
83. Zhou Z, Han B, Jin H, Chen A, Zhu L. Changes in long non-coding RNA transcriptomic profiles after ischemia-reperfusion injury in rat spinal cord. PeerJ. (2020) 8:e8293. doi: 10.7717/peerj.8293
84. Wang Q, Li ZX, Li YJ, He ZG, Chen YL, Feng MH, et al. Identification of lncRNA and mRNA expression profiles in rat spinal cords at various timepoints following cardiac ischemia/reperfusion. Int J Mol Med. (2019) 43:2361–75. doi: 10.3892/ijmm.2019.4151
85. Lennox KA, Behlke MA. Cellular localization of long non-coding RNAs affects silencing by RNAi more than by antisense oligonucleotides. Nucleic Acids Res. (2016) 44:863–77. doi: 10.1093/nar/gkv1206
86. van Heesch S, van Iterson M, Jacobi J, Boymans S, Essers PB, de Bruijn E, et al. Extensive localization of long noncoding RNAs to the cytosol and mono- and polyribosomal complexes. Genome Biol. (2014) 15:R6. doi: 10.1186/gb-2014-15-1-r6
87. Liu Y, Pan L, Jiang A, Yin M. Hydrogen sulfide upregulated lncRNA CasC7 to reduce neuronal cell apoptosis in spinal cord ischemia-reperfusion injury rat. Biomed Pharmacother. (2018) 98:856–862. doi: 10.1016/j.biopha.2017.12.079
88. Guffanti A, Iacono M, Pelucchi P, Kim N, Solda G, Croft LJ, et al. A transcriptional sketch of a primary human breast cancer by 454 deep sequencing. BMC Genomics. (2009) 10:163. doi: 10.1186/1471-2164-10-163
89. Fan Y, Shen B, Tan M, Mu X, Qin Y, Zhang F, et al. TGF-beta-induced upregulation of malat1 promotes bladder cancer metastasis by associating with suz12. Clin Cancer Res. (2014) 20:1531–41. doi: 10.1158/1078-0432.CCR-13-1455
90. Ji P, Diederichs S, Wang W, Boing S, Metzger R, Schneider PM, et al. MALAT-1, a novel noncoding RNA, and thymosin beta4 predict metastasis and survival in early-stage non-small cell lung cancer. Oncogene. (2003) 22:8031–41. doi: 10.1038/sj.onc.1206928
91. Ip JY, Nakagawa S. Long non-coding RNAs in nuclear bodies. Dev Growth Differ. (2012) 54:44–54. doi: 10.1111/j.1440-169X.2011.01303.x
92. Michalik KM, You X, Manavski Y, Doddaballapur A, Zornig M, Braun T, et al. Long noncoding RNA MALAT1 regulates endothelial cell function and vessel growth. Circ Res. (2014) 114:1389–97. doi: 10.1161/CIRCRESAHA.114.303265
93. Tang Y, Jin X, Xiang Y, Chen Y, Shen CX, Zhang YC, et al. The lncRNA MALAT1 protects the endothelium against ox-LDL-induced dysfunction via upregulating the expression of the miR-22-3p target genes CXCR2 and AKT. FEBS Lett. (2015) 589 (20 Pt. B):3189–96. doi: 10.1016/j.febslet.2015.08.046
94. Watts R, Johnsen VL, Shearer J, Hittel DS. Myostatin-induced inhibition of the long noncoding RNA Malat1 is associated with decreased myogenesis. Am J Physiol Cell Physiol. (2013) 304:C995–1001. doi: 10.1152/ajpcell.00392.2012
95. Zhao J, Li L, Peng L. MAPK1 up-regulates the expression of MALAT1 to promote the proliferation of cardiomyocytes through PI3K/AKT signaling pathway. Int J Clin Exp Pathol. (2015) 8:15947–53.
96. Qiao Y, Peng C, Li J, Wu D, Wang X. LncRNA MALAT1 is neuroprotective in a rat model of spinal cord ischemia-reperfusion injury through miR-204 regulation. Curr Neurovasc Res. (2018) 15:211–9. doi: 10.2174/1567202615666180712153150
97. Young TL, Matsuda T, Cepko CL. The noncoding RNA taurine upregulated gene 1 is required for differentiation of the murine retina. Curr Biol. (2005) 15:501–12. doi: 10.1016/j.cub.2005.02.027
98. Sun J, Hu J, Wang G, Yang Z, Zhao C, Zhang X, et al. LncRNA TUG1 promoted KIAA1199 expression via miR-600 to accelerate cell metastasis and epithelial-mesenchymal transition in colorectal cancer. J Exp Clin Cancer Res. (2018) 37:106. doi: 10.1186/s13046-018-0771-x
99. Li TH, Zhang JJ, Liu SX, Chen Y. Long non-coding RNA taurine-upregulated gene 1 predicts unfavorable prognosis, promotes cells proliferation, and inhibits cells apoptosis in epithelial ovarian cancer. Medicine. (2018) 97:e0575. doi: 10.1097/MD.0000000000010575
100. Wu Z, Zhao S, Li C, Liu C. LncRNA TUG1 serves an important role in hypoxia-induced myocardial cell injury by regulating the miR1455pBinp3 axis. Mol Med Rep. (2018) 17:2422–30. doi: 10.3892/mmr.2017.8116
101. Wu P, Zuo X, Deng H, Liu X, Liu L, Ji A. Roles of long noncoding RNAs in brain development, functional diversification and neurodegenerative diseases. Brain Res Bull. (2013) 97:69–80. doi: 10.1016/j.brainresbull.2013.06.001
102. Pietretti D, Spaink HP, Falco A, Forlenza M, Wiegertjes GF. Accessory molecules for Toll-like receptors in Teleost fish. Identification of TLR4 interactor with leucine-rich repeats (TRIL). Mol Immunol. (2013) 56:745–56. doi: 10.1016/j.molimm.2013.07.012
103. Jia H, Ma H, Li Z, Chen F, Fang B, Cao X, et al. Downregulation of LncRNA TUG1 inhibited TLR4 signaling pathway-mediated inflammatory damage after spinal cord ischemia reperfusion in rats via suppressing TRIL expression. J Neuropathol Exp Neurol. (2019) 78:268–82. doi: 10.1093/jnen/nly126
104. Wang JP, Tang YY, Fan CM, Guo C, Zhou YH, Li Z, et al. The role of exosomal non-coding RNAs in cancer metastasis. Oncotarget. (2018) 9:12487–502. doi: 10.18632/oncotarget.23552
105. Wang X, Luo G, Zhang K, Cao J, Huang C, Jiang T, et al. Hypoxic tumor-derived exosomal miR-301a mediates M2 macrophage polarization via PTEN/PI3Kgamma to promote pancreatic cancer metastasis. Cancer Res. (2018) 78:4586–98. doi: 10.1158/0008-5472.CAN-17-3841
106. Lin XJ, Fang JH, Yang XJ, Zhang C, Yuan Y, Zheng L, et al. Hepatocellular carcinoma cell-secreted exosomal MicroRNA-210 promotes angiogenesis in vitro and in vivo. Mol Ther Nucleic Acids. (2018) 11:243–52. doi: 10.1016/j.omtn.2018.02.014
107. Kwizera EA, O'Connor R, Vinduska V, Williams M, Butch ER, Snyder SE, et al. Molecular detection and analysis of exosomes using surface-enhanced raman scattering gold nanorods and a miniaturized device. Theranostics. (2018) 8:2722–38. doi: 10.7150/thno.21358
Keywords: non-coding RNAs, spinal cord ischemia-reperfusion injury, microRNA, long non-coding RNA, cerebral ischemic diseases
Citation: Ling X, Lu J, Yang J, Qin H, Zhao X, Zhou P, Zheng S and Zhu P (2021) Non-Coding RNAs: Emerging Therapeutic Targets in Spinal Cord Ischemia–Reperfusion Injury. Front. Neurol. 12:680210. doi: 10.3389/fneur.2021.680210
Received: 10 April 2021; Accepted: 09 August 2021;
Published: 08 September 2021.
Edited by:
Ulises Gomez-Pinedo, Instituto de Investigación Sanitaria del Hospital Clínico San Carlos, SpainReviewed by:
Rodrigo Ramos-Zúñiga, University of Guadalajara, MexicoCopyright © 2021 Ling, Lu, Yang, Qin, Zhao, Zhou, Zheng and Zhu. This is an open-access article distributed under the terms of the Creative Commons Attribution License (CC BY). The use, distribution or reproduction in other forums is permitted, provided the original author(s) and the copyright owner(s) are credited and that the original publication in this journal is cited, in accordance with accepted academic practice. No use, distribution or reproduction is permitted which does not comply with these terms.
*Correspondence: Peng Zhu, ZG9jdGZmQHNtdS5lZHUuY24=; Shaoyi Zheng, emhzeUBzbXUuZWR1LmNu; Pengyu Zhou, cHl6aG91QHNtdS5lZHUuY24=
†These authors have contributed equally to this work and share first authorship
Disclaimer: All claims expressed in this article are solely those of the authors and do not necessarily represent those of their affiliated organizations, or those of the publisher, the editors and the reviewers. Any product that may be evaluated in this article or claim that may be made by its manufacturer is not guaranteed or endorsed by the publisher.
Research integrity at Frontiers
Learn more about the work of our research integrity team to safeguard the quality of each article we publish.