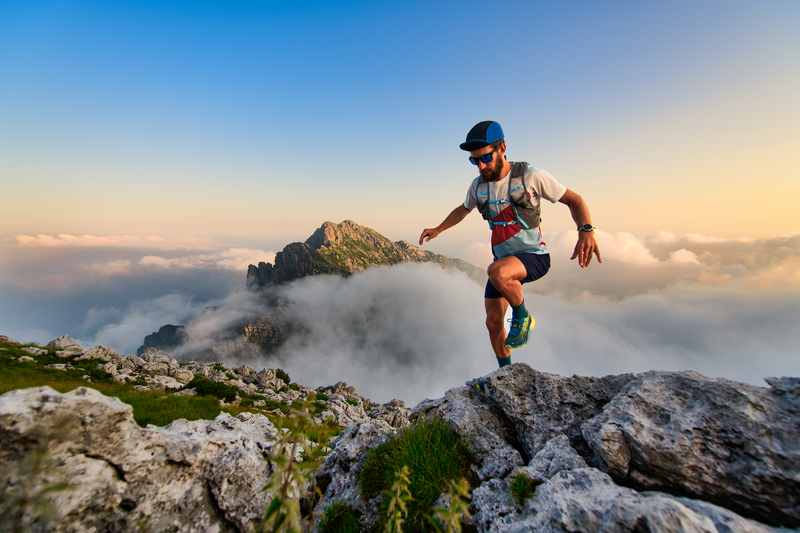
94% of researchers rate our articles as excellent or good
Learn more about the work of our research integrity team to safeguard the quality of each article we publish.
Find out more
REVIEW article
Front. Neurol. , 17 June 2021
Sec. Neurorehabilitation
Volume 12 - 2021 | https://doi.org/10.3389/fneur.2021.667511
Intracerebral hemorrhage (ICH) is the second most common type of stroke and has one of the highest fatality rates of any disease. There are many clinical signs and symptoms after ICH due to brain cell injury and network disruption resulted from the rupture of a tiny artery and activation of inflammatory cells, such as motor dysfunction, sensory impairment, cognitive impairment, and emotional disturbance, etc. Thus, researchers have established many tests to evaluate behavioral changes in rodent ICH models, in order to achieve a better understanding and thus improvements in the prognosis for the clinical treatment of stroke. This review summarizes existing protocols that have been applied to assess neurologic function outcomes in the rodent ICH models such as pain, motor, cognition, and emotion tests. Pain tests include mechanical, hot, and cold pain tests; motor tests include the following 12 types: neurologic deficit scale test, staircase test, rotarod test, cylinder test, grid walk test, forelimb placing test, wire hanging test, modified neurologic severity score, beam walking test, horizontal ladder test, and adhesive removal test; learning and memory tests include Morris water maze, Y-maze, and novel object recognition test; emotion tests include elevated plus maze, sucrose preference test, tail suspension test, open field test, and forced swim test. This review discusses these assessments by examining their rationale, setup, duration, baseline, procedures as well as comparing their pros and cons, thus guiding researchers to select the most appropriate behavioral tests for preclinical ICH research.
Intracerebral hemorrhage (ICH) is a type of intracranial hemorrhage which occurs due to sudden rupture of tiny arteries in the brain parenchyma. As the second most common cause of stroke (1, 2), ICH is responsible for 8–15% of all strokes in high-income countries, with the highest rates in Asia (3). An official Chinese report the National Epidemiological Survey of Stroke in 2012–2013 showed that stroke is the second most common cause of death within the country; among all causes of stroke mortality, the proportion of ICH was 24% (4). Overall, ICH is a major driver of stroke mortality rates, with a 1-month mortality rate of 30–50% (5), and a one-year rate of 54% (6). Hemorrhage primarily occurs in the basal ganglia, although it can also occur in the thalamus, lobes, brainstem, and cerebellum (7, 8). There are many neurologic manifestations of ICH, depending on the location and the size of the hemorrhage, and the extent of subsequent activation of inflammatory cells (9–11). In general, these manifestations can include motor dysfunction, sensory impairment, and cognitive impairment. Additionally, some ICH patients may have recognition deficits, emotional disturbances, and central pain (12). Thus, to better simulate and evaluate all the aspects of brain cell injury and connecting network dysfunction, a battery of assessments have been established to evaluate behavioral changes in ICH models. Currently, a wide variety of behavior tests exist for the ICH rodent model assessment. However, their documentation in research reports and literature reviews have been sporadic (13). Consequently, a systematic and comprehensive review that summarizes all the applications of various behavioral protocols will allow researchers to be able to more efficiently choose suitable behavioral tests and thus establish effective models to illuminate the pathophysiologic mechanisms and assess the potential translation of ICH treatment. This review summarizes the evaluation methods of sensory, motor, emotion, and cognition tests after the operation of the ICH model in rodent animals (Figure 1), thus allowing experimental researchers to select the appropriate detection methods according to different experimental conditions.
Figure 1. Abnormal behavior after intracerebral hemorrhage (ICH) in rodents. For each behavioral testing, we discussed several aspects, such as rationale, setup, duration, baseline, procedures as well as comparing their pros and cons. ART, Adhesive removal test; BW, Beam walking; EPM, Elevated plus maze; FPT, Forelimb placing test; FST, Forced swim test; GWT, Grid walk test; HL, Horizontal ladder; ICH, Intracerebral hemorrhage; MWM, Morris water maze; mNSS, Modified neurological severity score; NDS, Neurological deficit scale; NORT, Novel object recognition test; OFT, Open field test; RT, Rotarod test; SPT, Sucrose preference test; ST, Staircase test; TST, Tail suspension test; WHT, Wire hanging test.
Sensory tests are used to assess pain sensitivity. Sensory tests generally involve mechanical allodynia, thermal hyperalgesia, and cold hyperalgesia test. The mechanical allodynia test can be performed with dynamic plantar aesthesiometer or von Frey filaments; thermal hyperalgesia is often tested by Hargreaves analgesia meter; and cold hyperalgesia can be tested by acetone test, cold plate test, or cold plantar assay. In these tests, the investigator should be blind to the treatment assignment, and animals should be placed in the test boxes no <30 min in advance to allow for habituation (Table 1). ICH may cause changes in the pain threshold, so pain sensory testing can be used as one of the functional outcome parameters.
Mechanical allodynia is often tested by von Frey filaments or dynamic plantar aesthesiometer, which can well-reflect animals' mechanical pain sensitivity (27, 28). The apparatus needed for this test includes a metal mesh floor and several plexiglass chambers (27). The test is usually carried out 1 day prior to surgery, and 1, 3, 5, 7, 10, 14, and 21 days post-surgery (14). Researchers often utilize the up-and-down method designed by S.R. Chaplan in 1994 (29), but there are also several other methods used in this test. At the beginning of the test, the animals are placed in the chamber on a mesh floor and permitted to freely explore for at least 30 min. Then a dynamic plantar aesthesiometer or von Frey filaments are used to prick the plantar surface of animals' hind paws (16).
For the test using von Frey filaments, ascending forces of filaments are used in sequence. The minimum force that causes the rat to remove its paw is defined as the paw withdrawal threshold. The baseline of sham group rats is around 15–20 g (16, 30). In some experiments, filaments weighing 0.07 or 0.4 g are chosen to test the mice's mechanical pain response (17). Each filament was applied 10 times to the plantar surface (with contact for 3 s), and mechanical allodynia was defined as the percentage of withdrawal responses to the 10 stimulation trials. The paw withdrawal percentage is calculated by the following formula: [(number of paw withdrawals/10 trials) × 100%]. The baseline of the sham group is around 10% with the stimulation of 0.07 g filament, and 40% with the stimulation of 0.4 g filament (17). The paw withdrawal time of the ICH animals should be longer in duration than that of the sham group animals.
As for the dynamic plantar aesthesiometer, the forces will change in ascending, graded forces (2 g/s, and cut-off force is 10 g for mice, 50 g for rats) electrically. The minimum force that causes a paw withdrawal response is recorded. The process is repeated three times for each hind paw, and the mean values are taken as the paw withdraw threshold, respectively (15, 23). The baseline force for normal rats is around 35 g (15), while the normal mice is around 0.8 g (16).
The paw withdrawal threshold declines after ICH. Von Frey test is the most widely used method to test mechanical pain threshold. Its advantage is that it is easy to implement and its own set of standard procedures can facilitate a smooth learning process for beginners. However, a von Frey test takes longer than testing with a dynamic plantar aesthesiometer. Its disadvantage is that both assessments can be biased by factors such as the strength of the hand holding the stab pen, the criteria for judging the sting, the spontaneous movements of animals, etc. (31). Humidity, and probably temperature, may affect elastic modulus, and thus bending force. The filaments should be applied smoothly and avoid miss-hitting the foot or slip. The results can be influenced by the animal's behavior such as grooming or spontaneous movements. In some cases, it is difficult to distinguish whether the animals felt pain or just needed to change their posture. The dynamic filaments may be more easily affected by the postural change (32).
The thermal hyperalgesia test requires a shelf with a thick glass plate, a radiant heat stimulator, and plexiglass chambers. The temperature of the plate surface is held constant (14, 16, 18). The test is performed 1 day prior to surgery and on days 1, 3, 5, 7, 10, 14, and 21 post-surgery (14). Before the test, the animals are placed in the chambers for at least 30 min in advance to allow for habituation. The heat stimulator should be focused on the plantar surface of the animals' hind paws under the glass plate (27, 28). The animal will remove its paw when the stimulation reaches the heat threshold. The latency of the withdrawal response (the time between started infrared stimulation to the withdrawal of the hind paw) should be recorded (28). The duration of stimulation should be no longer than 20 s to avoid burn damage (14, 27). The process is repeated three or five times, and the mean values are taken as the threshold values (17, 23, 28). The baseline for normal rats is around 12 s (15) and for mice is around 15 s (18). The latency of the withdrawal response of the ICH animals is shorter than that of the sham group.
The thermal hyperalgesia test is also widely used in pain tests. Its advantage is that when animals remove their hind paws, the device will automatically stop, thus helping to rule out confounding factors such as subjective judgment. Its disadvantage is that other problems might occur because of the free movements of animals as well as their droppings.
The cold hyperalgesia test includes three typical methods: the acetone test, the cold plate test, and the cold plantar assay (18, 23, 26).
The first one requires a 1 ml blunt syringe and a platform with a mesh floor (19, 20). The animals also need to habituate for at least 30 min in the plexiglass chamber. For the test, acetone is sprinkled on the plantar surface of the animal's hind paw (applied volumes vary across articles) (33). The animal response was observed for 20 s and graded on a 4-point scale (0, no response; 1, quick withdrawal, flick or stamp of the paw; 2, prolonged withdrawal or repeated flicking; and 3, repeated flicking of the paw with licking the plantar surface of the paw). Acetone was applied alternately three times to each hind paw at intervals of 30 s (20, 21). The mean values of the three assessments are taken as the threshold values (21). The baseline values of normal animals are around one point (22). The mean value of ICH animals is higher than that of the sham group.
The cold plate test requires a regular cold metal aluminum platform that can maintain at a constant low temperature (4 ± 1°C) (23, 24). Animals are to be situated on the plate and the latency of withdrawal response is recorded. Withdraw latency is defined as lickings, paw movements, or little leaps (23). A 20 s cutoff for mice (30 s for rats) should be implemented to prevent tissue damage (25). The baseline of sham mice is around 15–20 s, and the baseline of rats is around 25~30 s (17, 25). The latency of the withdrawal response for ICH animals is shorter than that of sham group animals.
There is also a novel behavioral assay for measuring cold sensation in mice, the cold plantar assay. This test requires a shelf with a glass plate a 3 ml syringe with the syringe top cut off, and dry ice powder. Then the dry ice is loaded into the syringe and compacted into an icicle. The icicle is placed on the plantar surface of the mouse's hind paw through the glass plate. The paw withdrawal latency is defined as the period of time between the beginning of contact to when the mouse's foot moves away. The baseline latency for normal mice is around 10–15 s. This duration is shorter in ICH animals. The cold plantar assay can complement currently used assays and accurately measure the cold response threshold (26).
Cold hyperalgesia tests are widely used in ICH models. Although the acetone test is easy to administer, it has poor precision since the capacity of most syringes is 1 ml whereas the experimental dosage of acetone needed is usually only 0.025 ml. The rate at which acetone is ejected from the syringe can also adversely affect the tests. In addition, acetone may have adverse health effects for the animals and researchers. In contrast, the cold plate test is more precise and objective, but more expensive. The advantage of the cold plantar assay is that it is easy to utilize and cheap, but it hasn't been widely used as the other two tests.
Presently, motor tests commonly used for ICH detection in basic research generally include the following 12 types: the neurologic deficit scale, corner test, staircase test, rotarod test, cylinder test, grid walk test, forelimb placing test, wire hanging test, modified neurologic severity score, beam walking test, horizontal ladder test, and adhesive removal test (Table 2).
The 24-point neurological deficit scale is often used in the motor function tests. It is the most convenient assessment and doesn't require any apparatus. The animals are tested on days 1, 3, 7, 14, and 21 post-ICH (34, 35). For mice, the researcher should observe 6 aspects, including body symmetry, gait, climbing, circling behavior, front limb symmetry, and compulsory circling. Each aspect is graded from 0 to 4, with the maximum score being 24 points (74). As for rats, the researcher should observe spontaneous ipsilateral circling, hind limb retraction, bilateral forepaw grasp, beam walking ability, forelimb flexion. The first four assessments should be graded from 0 to 3, the last one should be graded from 0 to 2. The maximum score is 14. The detailed scoring protocol has been described previously (75). The baseline for both normal and ICH animals is 0 points. The advantage of the neurological deficit scale scoring system lies in its simplicity of procedure as well as equipment and having a very exact criterion.However, it can be influenced by the animals' autonomous activities and the subjective judgments of the researchers (76).
The corner test is a method used to assess sensory-motor function, which has been proven to be a reliable method for identifying as well as quantifying sensory and postural asymmetry (74, 77). It provides a simple method for the detection of contralateral deficit and ipsilateral steering deviation.
The test was applied to the unilateral nigral striatum injury in rats (78) and to the focal cerebral ischemia in mice (36). The device is tightly attached to two adjacent plastic plates to form a narrow lane of 30 degrees, leaving a small opening to approach, and then the animals are put between the plastic plates facing the corner. When animals approach the corner, both sides of the whiskers are stimulated at the same time, causing the animal to rotate 180 degrees. There is no marked preference for turning direction in healthy animals whereas unilaterally brain-damaged animals may display a consistent preference for the same side. The baseline for normal animals is around 50% (36–39).
The corner test is simple, impartial, and relatively sensitive (36). In addition to identifying sensorimotor disorders, corner testing has been shown to be an objective assessment of long-term functional outcomes in rats and mice (up to 90 days) after stroke (41, 42, 79). There is an advantage that the corner test may be more sensitive than other symmetrical tests because it reflects multiple asymmetries, including forelimb, hind limb, posture, and steering bias (37). However, it is not sensitive for severely injured animals and repeated tests (76).
The staircase test is mainly used to measure and compare the flexibility, motor coordination, and autonomy ability of mice before and after forelimb dysfunction caused by ICH (40). Its main advantage is that it can independently evaluate the ability of mice to skillfully use their limbs after injury.
This test was originally designed to assess the independent use of forelimbs in rats (40) and was later used to assess skilled reaching (80). By observing rats' behavior when they reach for food pellets, bilateral measurements of animal forelimb stretch, mastery of skills, and lateral deviation can be quantified.
The apparatus including a plexiglass box (19 cm wide × 27 cm long × 25 cm high) with an elevated platform in it, and seven stairs descending on each side of the platform (40). Food pellets (45 mg of each pellet) were placed on the stairs (three pellets for each stair). The rat rests on the platform and the food pellets on one side can only be reached by the rat's paw on the same side. The device is designed to encourage animals to get food through confined spaces (40). The rats are generally food-deprived 3 days before the assessment, and trained for 2–3 weeks before this task (5 days per week, two 15-min trials a day with a 4–5 h duration) (64, 81). Normal animals can usually collect pellets quickly (77). The number of pellets collected is recorded. The range of normal values is above nine pellets on each side in both mice and rats (64, 80). This test has been proven to be sensitive to persistent defects in the detection of ischemic brain injury (40, 81). Potential drawbacks of this test are that detailed behavior cannot always be well-quantified (40) and that long-term pre-training is needed (77). The video of detailed procedures for rats is available in reference (82).
The rotarod test is used to assess motor coordination and balance (83, 84). This experiment includes two parts: 3 days of training before surgery and formal tests on days 1, 2, 3, 5, 7, and 14 after surgery. Before starting the rotarod test, the mice are trained for 15 min at a set rotational speed (15 RPM), followed by three trials accelerated from 4 to 40 RPM in 5 min (43, 85). The average baseline latency of the three training days before the operation is obtained (43, 69). On the test day, three trials are run on each animal, and the average retention time of three trials is computed (43). There should be a 15-min rest interval between each test (46). Before surgery, the baseline of rats is around 150 s (86), while the mice are around 250~300 s (45, 87). Animals with hemorrhagic damage tend to fall faster than normal ones (88). The video of detailed procedures for rats is available in reference (89). The video of detailed procedures for mice is available in reference (90). Rotarod test is sensitive and straight forward, but is unable to evaluate the acquisition of motor skill learning (91).
The cylinder test can not only be used as an assay of brain function but can also evaluate rodent's spontaneous forelimb use, which is the main advantage of this method. The device consists of a transparent acrylic glass cylinder with a diameter of 7–10 cm. Two mirrors are placed behind the cylinder to observe the mice from three different angles at the same time. In each trial, the instances of placement of left and right, right, or left forelimbs on the wall are recorded. The average percentage of baseline use of the damaged forelimb is reported to have been tested for two 5-min trials, up to 20 times for each. The animals are evaluated twice prior to surgery for baseline and then at days 1, 3, 7, and 14 days post-injury (47). The results are analyzed with the following formula: (contralateral forelimb movement - ipsilateral forelimb movement)/(contralateral forelimb movement + ipsitralateral forelimb movement + both movement). The baseline for normal animals is 0 (47). The use of contralateral claws is reduced in animals with brain damage. The video of detailed procedures for mice can be seen in reference (92). The video of detailed procedures for rats can be seen in reference (82). The cylinder test is low-costing and easy to perform. It is sensitive to assess chronic deficits (93), but is not under-utilized to assess forelimb deficits (92).
The grid walk test (GWT) is a sensitive measure of sensory-motor coordination (94). The apparatus for this test is consists of an overhead grid that connects two tall walls (15). The opening of the grid for rats is around 2–3 cm (95). The animals should be evaluated at days 1,3,7, and 14 post-injury (48). Normal mice will precisely grasp the wireframe to balance themselves when placed on mesh while the hemorrhagic mice's paws may slip through the open grid, this is defined as a “foot fault” (94). For the test, the animals are placed on the mesh for 3 min while the number of “foot faults” for each limb and their total steps are recorded. The average value of the foot fault test in normal rats is around 20 times (49, 50). A foot fault index [(contralateral faults—ipsilateral faults)/total steps] is calculated. 0 represents no asymmetry; a positive score indicates increased contralateral foot faults and implies impaired contralateral motor function. Since the injury of the unilateral brain will cause contralateral neurologic deficits (77), animals would show increased contralateral foot faults after unilateral ICH. The normal occurrence for slips is < ±5% in the sham mice (51). You can watch the video of detailed procedures for mice as provided by reference (96). The video of detailed procedures for rats is available from reference (97).
The forelimb placing test (FPT) was scored using the vibrissae-elicited forelimb placing test (98). Mice have been known to respond to vibrissae stimulation with foreleg movement (59). Thus, this can be used to assess ICH-induced neurological deficits. Prior to the test, the animals were gently moved up and down to promote muscle relaxation and to eliminate any struggle response (37, 99). Next, they are placed on the edge of the table. When the mice's vibrissae touch the table, the healthy animals will quickly place their ipsilateral forelegs on the table. This forelimb placement experiment can be quantified as the percentage of successful responses in 10 trials. It usually takes 5 min to complete the test. In general, this test was performed on days 1, 3, 12, and 28 after ICH (52, 53). Animals with unilateral brain damage have been found to respond less on the contralateral side while healthy rats will generally have a higher success rate in this task (98). The baseline performance for sham group animals is nearly 100% (54, 55). Overall, the advantage of this test is that it is quick and easy to perform. It can detect mild neurologic impairments (77). The examiners should practice in advance to avoid abrupt moving. The integrity of the mouse whiskers is a prerequisite for this test (77).
The wire-hanging test (WHT) is useful for evaluating locomotor abnormalities (100). It is applied to evaluate grip strength, balance, and endurance in mice on days 1, 3, 7, 14, and 21 post-ICH (56). An iron wire (1 mm in diameter, 55 cm long) is stretched horizontally between two posts, 50 cm above the ground. Mice are placed on the wire and have to use their forelimbs to suspend their body weight. The hind limbs are gently covered with adhesive tape to prevent them from using all four paws. A pillow is placed beneath the mice to prevent falling injuries. The time that each animal remained on the wire is recorded (57). The baseline of the sham group is around 30–40 s (101). The result is represented as the average of three trials per animal (102). Compared to normal mice, gripping and forelimb strength are significantly impaired in ICH mice at all of the time points, and falling latency in the wire-hanging test is shorter than normal or sham animals on days 1, 3, and 7 post-ICH (56). WHT is useful to measure coordination and endurance. The limitation of this test is that it's unsuitable for rats. For their heavier weight means that it is painful for them to support their body weights on a wire and the test has a higher chance of causing fall injuries. Results might be in inconsistent because of the moving of the hind limbs (103).
The modified neurologic severity score (mNSS) contains sensory tests, motor tests, reflex tests, and beam balance tests (104) and is used to assess neurologic deficits and the grade of neurologic damage on the aspects of motor, ground walking, sensory, coordination of movements, reflex, and abnormal movements (105) (Table 3). The tests are performed on days 1, 3, 7, 14, and 30 after ICH by the testers who are blind to the treatment groups using either rats or mice (106). For both mice and rats, the tester should observe 4 aspects, including abnormal movements or absence of reflex, beam balance test, sensory function, and locomotor function. The first, third, and fourth aspects are all graded from 0 to 1 point and the second aspect is graded from 0 to 6 points. The baseline for the normal animals is 0 points. Each animal should be tested twice and the average score is calculated after the test (105). The highest score is 18. The higher the score, the more severe the injury is (104).
Beam walking is always carried out to measure balance and asymmetrical coordination (108). It can be performed at 1, 3, 7, 11, 14, 21, and 28 days post-ICH (59). The wooden beam sets up at 50 cm above the ground for mice and at 1 m above the ground for rats (59–62). Animals should be trained to cross the beam before surgery (107). The animals are graded on a seven-point scale on the performance when they cross the beam. Each testing session consists of three trials to get an average value (63, 65, 109). This test can be used to evaluate the balance and the locomotor activity of the rodents. The disadvantage of this test is that the narrower the balance beam is, the more times the test animal misses, which leads to the lower reliability of the results.
The horizontal ladder test (HLT) is used to evaluate walking ability (110, 111). Rats are mainly used in this test. The horizontal ladder is 30 cm above the ground and is made up of two clear side walls (1 m long and 19 cm high) and several metal rungs (3 mm diameter). The distance between two adjacent rungs is changeable (1–5 cm accordingly) (67, 68). A home cage is placed at the end of the ladder to encourage the animals' moving (112). Rats should be trained for 3 days before surgery and tested at 7, 14, 21, and 28 days post-surgery, and three times per day to get an average value (64–66). Every slight paw slip, deep paw slip, and complete misses are scored as an error (112, 113). The total number of steps and the number of errors of each limb is counted (113). Each limb error rate is calculated as error steps/total steps × 100%. The baseline of normal animals is closed to 0 (68). HLT is sensitive enough to evaluate the relationship between motor impairment and injury volume in ICH (67). It is useful in assessing chronic deficits and can be used repeatedly since the rungs are changeable (112).
The adhesive removal test (ART) is used to assess the sensorimotor neurologic deficits of both forepaws (69, 114). It is assessed on days 1, 3, 7, 14, 21, and 28 post surgery, and needs 3 days' training prior to surgery (69, 70). The equipment needed for this test is very simple, the adhesive tape and a scissor. Using the scissor to cut the tape into small circular or square pieces, the diameter or length is around 4 mm for mice and 6 mm for rats (64, 70, 71). Gently apply the tape to each forepaw in a random order (60, 115). Be sure to keep equal pressure between each trial and each animal (60). The latency of removing the tape is recorded from the time the animal notices the tape until the tape is removed. The animal's forepaw is tested three times to calculate the average value. An interval of 5 min between each trial is necessary (73, 115). The cutoff time is around 120–180 s (60, 64, 115). The animal should acclimatize the experimental cage for 2 min in advance (69, 116). A 3-day-training is required to ensure the animal adapts to the condition of being put on the tape, and to learn to tear the tape off within 10s (73). ART can be used to evaluate primarily the sensory deficits and the asymmetrical biases (79). Special equipment is not needed. The size and stickiness of the tape should be the same for data reproducibility (93).
A variety of mazes are widely used in testing animals' cognitive ability. In basic research of ICH rodent animal models, the Morris water maze, Y-maze, and novel object recognition tests are the most frequently used methods (Table 4).
The Morris water maze (MWM) is usually used to evaluate spatial learning and memory ability after ICH (117). The required device consists of a metal pool (120 cm in diameter; 55 cm in height) which is divided into four quadrants with a platform (10 cm in diameter, 21 cm in height) in one quadrant for the animals to escape. The pool is filled with water 2 cm above the platform. The water temperature is maintained at 26 ± 1°C (58, 118). Noldus EthoVision tracking software is to record the delay, frequencies, and swimming speed of the mice before the discovery of the platform (119). The experiment includes two parts: 5 days of training and a sixth test day. The mice are trained at intervals of 20–30 min for a total of four times during each training day. During training, mice should be placed gently on the instrument facing the wall. On average, the mice found the platform within 90 s and stayed on the platform for 15 s (118, 124). If the platform could not be found within 90 s, the mice are gently guided to the platform for 15 s, and the latency is recorded as 90 s. After each test, mice are wiped with a towel and placed into the heating cage. On the sixth day, the platform is removed, and the animals are tested as usual. The results are the time spent in the incubation period and the target quadrant of the platform (117, 118, 125). In training experiments, shorter latency in reaching the platform can be correlated with better spatial learning and memory ability. Initially, ICH mice took significantly longer than control mice. The escape latency of sham group rats is around 15 s (88). For the sham group mice, the escape latency is around 45s, and target crossings required ~12 s (119). However, this latency should significantly be reduced over the next few days, suggesting that spatial memory is established (126).
MWM is the most widely used method for testing memory (127). Olfactory trails or cues are eliminated in this test (128). The experimental data can accurately assess the animal's sense of spatial position and direction, especially in terms of spatial positioning. However, this method has some shortfalls. Swimming is an acute stressful stimulus for the animals, so neuroendocrine effects may impact experimental results (128). Additionally, the water temperature has a significant impact on animal activities, so it is essential to keep the water at a comfortable temperature. Finally, after the experiment, the experimental animals should be dried immediately to prevent sickness. The video of detailed procedures for mice can be seen in reference (129). The video of detailed procedures for rats can be seen in reference (130).
The Y-maze is also often used to test spatial memory. The device of Y-maze consists of three arms [40 cm long × 15 cm wide × 35 cm high for rats, 30 cm long × 10 cm wide × 17 cm high for mice)], diverging at 120° from the central point (121). The entrance of each arm is closed with a baffle. The test is performed 30 days after the operation (120). During the test, the baffles of two random arms are opened. One of the two is chosen to be the Start arm, and the remaining arm with baffle still on is designated the novel arm (122). The animals are put in the start arm and allowed to explore the opened two arms freely for 5 min. Then they are put back in the home cage to rest. After 2 h, all three baffles are removed and the animal is allowed to explore freely for 5 min. The durations of explorations in three arms and the duration in each arm are recorded (117). The percentage of novel arm entries is calculated around 35% (108, 121). There is another calculation method. First, label the three arms of the maze A, B, and C. Then start recording. Next, examine the recorded number of all arm entries and alternations. Finally, calculate the percent (%) alternation with the following formula:
% Alternation = (Number of Alternations/[Total number of arm entries − 2]) × 100 (131).
The advantage of the Y-maze test is that it is easy to perform and the apparatus itself is simple and convenient. The sensitivity and reproducibility of the test need to be characterized in the ICH models (76). The video of detailed procedures for mice can be seen in reference (132). The video of detailed procedures for rats can be seen in reference (133).
The novel object recognition test (NORT) assesses the animals' memory capability. It requires three objects, numbered A, B, and C, respectively, and an open-field arena (47 cm × 26 cm × 20 cm) that can hold the objects and mouse (56). Object A is the same as object B (green cubes, 4 cm × 4 cm × 3 cm), and they both look different from object C (white ball, 5 cm in diameter) which is the novel object (56). The open-field arena for rats is larger (60 cm × 60 cm × 50 cm) (134). The test includes three periods: habituation on day 1, training on day 2, and testing 1 h after the training. First, the mouse is placed in the empty arena and allowed to explore for 10 min on day 1. On the next day, objects A and B are placed in the open-field arena, and the mouse is put between these two objects, where it is permitted to explore freely for 5 min. Then, the mouse is taken out and placed back in its cage for a rest. The arena should be cleaned with alcohol to eliminate other scents at this time. After 1 h, object B is replaced by object C, and the mouse is returned to the arena for 5 min. The durations of exploration for object A and object C are recorded. The movement of the mouse is recorded by a camera and analyzed later. Sniffing or touching with the nose and/or forepaws within 2 cm around the objects is defined as an exploration event. Sitting or leaning on the objects is not considered to be exploratory behavior. The discrimination index is calculated as the time spent exploring object C divided by the time spent exploring both objects. The baseline of the discrimination index in normal mice or rats is around 70% (57, 123).
NORT is simple to perform and can be completed in a short time (135). However, experimental data is painstaking to obtain since video analysis takes considerable time and the animals' exploratory behavior may be hard to categorize at times (57). Choosing appropriate objects is difficult because the size, shape, material, and height, etc. may affect animals' preference (76). The video of detailed procedures for mice can be seen in reference (136). The video of detailed procedures for rats can be seen in reference (137).
Emotion tests are currently commonly used in basic neurologic research but seldomly used in ICH research. The mood tests are mainly divided into anxiety and depression tests. These texts include the elevated plus-maze, sucrose preference test, tail suspension test, open field test, forced swim test, and so on (Table 5).
The elevated plus maze (EPM) is generally applied to test anxiety (75). The test apparatus is composed of two arms and looks like a cross. One of the arms surrounding walls and is called the enclosed arm, while the arm without walls is called the open arm. The maze for the rats is 50 cm × 10 cm × 50 cm (75). The maze for the mice is 45 cm × 11 cm × 22 cm and located 80 cm above the ground (138).
The test relies on the rodent's exploratory nature. When placed in the middle of an EPM, their nature compels them to examine the open arm. However, rodents' fear of heights would discourage exploration, thus inducing anxiety. The test is performed 30 days post-ICH surgery (75). Two hours before the test, the mice are put into the testing room to adapt to the environment. At the start of the test, the animal is placed at the intersection of two arms with its head facing the open arm. The animal is allowed to explore the maze freely for 5 min (138, 148). The time spent in the open arm and the number of entries into the open arm is recorded (149). For rats, the baseline for entries into the open arm is around three times, and the percentage duration of time spent in the open arm is around 30% of the total testing time (139). For mice, the duration of time spent in the open arm is around 20 s, with ~3 entries (140).
The advantage of the EPM test is that it is easy to conduct and record, and does not need pre-training (150). However, sometimes animals can fall from the open arms and get hurt, and some animals may be reluctant to move onto the arms after surgery. The video of detailed procedures for mice is available from reference (151). The video of detailed procedures for rats is available from reference (152).
The sucrose preference test (SPT) is used to test anhedonia and depression (141). The device includes two bottles, one of which contains 1% sugar solution and the other pure water (141). The test is performed at 18–21 days post-surgery (141). At the beginning of the test, two bottles of water of equal weight are placed in the cage. At the end of the test, the bottles are weighed and water consumption is calculated. Then the sugar solution preference is calculated by the following formula: the sugar solution consumption (g)/[pure water consumption (g) + sugar solution consumption (g)] (153). The baseline of normal rats and mice is around 70% (142) and 90% (56), respectively. We conclude from the references that compared with the control group, the sugar solution preference degree of the depression group is lower (154).
SPT is easy to perform and causes no harm to the animals, It is the best method for evaluating anhedonia in mice (155). However, it lacks standardized apparatus (156). Water loss when taking out and putting in the cage is not easy to control, which will affect the experimental results. The experimental results are closely related to the feeding state of animals and can be affected by environmental inconsistencies (156). Researchers have to control the animals' diet before the test. The video of detailed procedures for mice can be seen in reference (157). The video of detailed procedures for rats is available from reference (158).
The tail suspension test (TST) is applied to test depression (159). The apparatus consists of a hanging box (55 cm × 60 cm × 11.5 cm), polycarbonate tube (4 cm in length, outside diameter 1.6 cm, inner diameter 1.3 cm), and packaging tape. The test is performed on day 21 post-surgery (57). To prepare for the test, a 17 cm tape with a marking at 2 cm is stuck to the animals' tails (2–3 mm of the end of the tail should be left outside the tape). The free end of the tape is hung on the hanging rod. The upside-down position will cause the animals to struggle. When they come to realize that struggling does not change their situation, they will cease to move. The mice are suspended for 7 min, and the stationary time in the last 6 min is recorded (143). After the test, the mice should be put back into their cages, and the researcher should gently pull off the tape from the tail. It is concluded from the references that the immobility time in 6 min of the control group is 150–300 s.
TST is easy to perform (160). The limitation of the test is that it is not suitable for rats because they are heavier. Thus, it is painful for them to rely on their tails to support their bodies' weight (tail fracture is a possibility).
The open-field test (OFT) is often used for locomotor activity and anxiety-like emotion in ICH models (13, 161). The apparatus consists of an open-field box (50 cm × 50 cm × 38 cm), the bottom of which is subdivided into 25 equal squares (nine squares in the center called the inter-zone, surrounded by 16 outer zones), and a computerized tracking system (144). Like the EPM test, the OFT utilizes the exploratory nature of rodent animals. Normal animals will spend time exploring the inner zone, while ICH animals are more likely to stay in the outer zone to feel more at ease. The test is performed on day 30 after surgery (75). On the testing day, the animals are put into the testing room for 2 h in advance to adapt to the testing environment. When the tests begin, the animals are put into the box for 10 min (145). The duration of time spent in the outer and inner zones is recorded (145, 161). The baseline duration spent by normal mice in the outer zone is around 350 s, and the duration spent in the inner zone is around 200 s (144).
OPT is widely applied in rodent behavioral research, but it has limitations because its outcome can be easily affected by many factors, such as time, lighting conditions, and room temperature (76, 144). The video of detailed procedures for mice is available from reference (144).
The forced swim test (FST) is a behavioral test for rodents first described in 1978 by Porsolt et al. (162). This test was developed as a model for predicting the clinical efficacy of antidepressant drugs and is now also widely used to analyze depressive-like behavior (57, 146).
In this test, an animal is placed in a container (50 cm high and 20 cm in diameter for rats, 20 cm high, and 22 cm in diameter for mice) filled with water. The test for rats consists of two sessions 24 h apart. The first session is the pre-test stage (15 min) and the second session is the test stage (5 min). The test for mice consists of one session 6 min long, divided into two sessions; the pre-test (the first 2 min) serves as a habituation period for the test (the last 4 min) (146). Immobility, swimming, and climbing behaviors are recorded (163). References indicate that normal mice have a mean immobility time of 120–140 s, a mean climbing time of 20–40 s, and a mean swimming time of 70–90 s (146). The baseline for normal rats is 125–150 s of immobility time, 75–100 s of climbing time, and 200–225 s of swimming time (147). It has been proven that animals exhibit increased immobility time in the FST after depression and that various antidepressants are able to reduce immobility time by increasing the swimming and/or climbing time (147, 164).
FST is low-costing, fast, and reliable. It is widely used to screen anti-depressants (160). However, it lacks construct validity and specificity (93, 165). Experimental animals will suffer from behavioral desperation when they are forced to swim. Additionally, one crucial limitation of the FST is that re-testing will lead to inaccurate experimental results, so animals can only be tested once in their lifetime. Lastly, similar to other tests, animal behavior in the FST is also influenced by biological factors including preconditioning before the FST, schedule, routes of treatment, dosage, and type of drugs, experimental design and, laboratory environmental factors (166). Detailed video for the mouse (167) and rat (146) procedures are available.
Finally, due to the great variety of animal behavior tests, more effort should be made to ensure the consistency of experimental conditions, and more attention should be paid to the following aspects to reduce the error of experimental results. (1) The test environment should be quiet and appropriate. (2) The animals should be given 2–3 h to habituate to the experimental environment before the test. (3) Animals should have rested for sufficient intervals to eliminate influence from the previous experiment. (4) The experimental equipment is wiped with alcohol before testing to eliminate odor. (5) External stimuli such as lighting, water temperature, water quality, and movement of the experimenter may all influence the animal's behavior. (6) Animals soaked in water should be dried at the end of the experiment to avoid sickness. (7) Double-blind should be taken as far as possible to reduce the influence of subjective factors. (8) Animals with significant limb use bias during pre-training should be excluded from the test.
Acute ICH increases with age and can occur in various brain locations. Subsequent brain damage and network disruption can lead to location-specific clinical signs and symptoms. Therefore, a wide range of behavioral tests should be utilized to assess relevant functional impairment. For example, when the striatum is injured, the sensorimotor function should be assessed, and when the thalamus is injured, the pain and emotional responses in addition to the motor function should be assessed.
Currently, the collagenase-induced and the whole blood animal models are the two best simulations of clinical ICH. They both generate hematoma within the brain parenchyma with distinct pathophysiology. Based on our knowledge, there are no studies focusing on the differences between these two preclinical models of ICH with regard to behavioral aspects. Our unpublished data indicate that, when comparing with the whole blood ICH model, the collagenase-induced ICH model shows greater blood-brain barrier breakdown and more severe neurologic deficits. Comparing with the collagenase-induced striatal ICH model, the collagenase-induced cortical ICH model shows transient and mild neurologic deficits and greater cognitive and emotional impairment (57). It has been suggested that both the collagenase-induced and the whole blood model should be tested in preclinical ICH drug efficacy studies.
There is currently no behavioral test specific to the ICH-induced brain injury. This is different from the Parkinson's disease model that can be assessed with a 6-OHDA-induced behavior test stressed by apomorphine. Based on this fact, we enumerated and discussed the behavioral tests that have been used in preclinical ICH research to provide a clear guide for researchers. These behavioral tests include a full evaluation of pain, motor, cognitive, and emotional dysfunction. The rationale, setup, duration, baseline, procedures, as well as pros and cons of each assessment, are also discussed. One point to note is that the protocol and the baseline used in different laboratories may vary even with the same functional behavioral assessment.
There is a gap between preclinical and clinical research of post-ICH depression. Koivunen et al. reported that about one out of four ICH survivors suffers from long-term depression (168). Because of the high incidence of post-ICH depression, elucidating its pathomechanism and identifying the therapeutic strategies become hot areas of current stroke/ICH research. The application of emotion-related behavioral tests in preclinical ICH research will help with the screening of potential therapeutics for treating post-ICH depression. Through PubMed research, however, we identified only five research papers in which the depression-like behaviors were studied in rodents with ICH (56, 57, 75, 88, 169). Based on the fact that we know very little about post-ICH depression-like behaviors in rodents, more research into this new area is strongly recommended.
Because different brain regions control specific brain functions, the location of brain hematoma determines the type of dysfunction that results. The selection of the behavioral tests for ICH research should keep this in mind. For a striatal ICH model, locomotor function tests can be selected. For a cortical ICH model, the cognitive and emotional tests should be selected, and for a thalamic ICH model with restricted damage to the lateral posterior nucleus, the sensation, cognitive, and emotion-like tests can be selected. Of course, the selection of the specific behavioral test should consider the research objectives, the experimental conditions, and the available lab resources (64).
Several methodologic issues may have hampered the clinical translation of preclinical findings. To provide a feasible and precise assessment of drug efficacy and to elucidate the underly cellular and molecular mechanisms of action, researchers should select the appropriate behavioral tests associated with location-specific ICH-induced brain cell injury and relevant network dysfunction. Additionally, many variables in rodent behavioral tests including age, sex, the specific strain of the animals, and comorbidies such as diabetics and hypertension can all influence the animal's behavioral test performance. For instance, age-related decline in learning, memory, and sensorimotor functions are well-established observations (170–173). In this regard, careful characterization of the baseline behavior should be established to rule out the fundamental differences in test performance, especially if global knockout mice are included. Finally, the blinding strategy should always be followed to reduce the Pygmalion effect or the observer bias, which requires blind allocation of the experimental groups and the blind assessment of the outcome measures (174).
Although histology, cellular and molecular biology, genetics, and electrophysiology are key tools for understanding mechanisms of action of novel therapeutic strategies, behavior represents the functional outcome of ICH and should be used for the final preclinical evaluation. Good lab practice with careful selection and execution of existing behavioral tests as we discussed above may improve the outcome of future translational research.
XC and JianW: conceptualization. XS and HB: writing-original draft preparation. JiarW, LH, and JianW review, edit, and critical revision. All authors literature search, review, commentary, and final approval of the manuscript.
XC was supported by the Key Project of Science and Technology of the Department of Science and Technology of Henan Province (grant number 212102310220).
The authors declare that the research was conducted in the absence of any commercial or financial relationships that could be construed as a potential conflict of interest.
ART, Adhesive removal test; BW, Beam walking; EPM, Elevated plus maze; FPT, Forelimb placing test; FST, Forced swim test; GWT, Grid walk test; HLT, Horizontal ladder test; ICH, Intracerebral hemorrhage; MWM, Morris water maze; mNSS, Modified neurological severity score; NDS, Neurological deficit scale; NORT, Novel object recognition test; OFT, Open field test; PWL, Paw withdraw latency; RPM, Revolutions per minute; SPT, Sucrose preference test; TST, Tail suspension test; WHT, Wire hanging test.
1. de Oliveira MA, Goffi A, Zampieri FG, Turkel-Parrella D, Duggal A, Marotta TR, et al. The critical care management of spontaneous intracranial hemorrhage: a contemporary review. Crit Care. (2016) 20:272. doi: 10.1186/s13054-016-1432-0
2. Zhu H, Wang Z, Yu J, Yang X, He F, Liu Z, et al. Role and mechanisms of cytokines in the secondary brain injury after intracerebral hemorrhage. Prog Neurobiol. (2019) 178:101610. doi: 10.1016/j.pneurobio.2019.03.003
3. Ren H, Han R, Chen X, Liu X, Wan J, Wang L, et al. Potential therapeutic targets for intracerebral hemorrhage-associated inflammation: an update. J Cerebral Blood Flow Metab. (2020) 40:1752–68. doi: 10.1177/0271678x20923551
4. Wu S, Wu B, Liu M, Chen Z, Wang W, Anderson CS, et al. Stroke in China: advances and challenges in epidemiology, prevention, and management. Lancet Neurol. (2019) 18:394–405. doi: 10.1016/s1474-4422(18)30500-3
5. Jiang C, Wang Y, Hu Q, Shou J, Zhu L, Tian N, et al. Immune changes in peripheral blood and hematoma of patients with intracerebral hemorrhage. FASEB J. (2020) 34:2774–91. doi: 10.1096/fj.201902478R
6. Al-Mufti F, Thabet AM, Singh T, El-Ghanem M, Amuluru K, Gandhi CD. Clinical and radiographic predictors of intracerebral hemorrhage outcome. Interv Neurol. (2018) 7:118–36. doi: 10.1159/000484571
7. Hu YZ, Wang JW, Luo BY. Epidemiological and clinical characteristics of 266 cases of intracerebral hemorrhage in Hangzhou, China. J Zhejiang Univ Sci B. (2013) 14:496–504. doi: 10.1631/jzus.B1200332
8. Wang J. Preclinical and clinical research on inflammation after intracerebral hemorrhage. Progr Neurob. (2010) 92:463–77. doi: 10.1016/j.pneurobio.2010.08.001
9. Zhang Z, Zhang Z, Lu H, Yang Q, Wu H, Wang J. Microglial polarization and inflammatory mediators after intracerebral hemorrhage. Mol Neurobiol. (2017) 54:1874–86. doi: 10.1007/s12035-016-9785-6
10. Hua W, Chen X, Wang J, Zang W, Jiang C, Ren H, et al. Mechanisms and potential therapeutic targets for spontaneous intracerebral hemorrhage. Brain Hemorrh. (2020) 1:99–104. doi: 10.1016/j.hest.2020.02.002
11. Lan X, Han X, Li Q, Yang Q-W, Wang J. Modulators of microglial activation and polarization after intracerebral haemorrhage. Nat Rev Neurol. (2017) 13:420–33. doi: 10.1038/nrneurol.2017.69
12. Wang CC, Shih H-C, Shyu BC, Huang ACW. Effects of thalamic hemorrhagic lesions on explicit and implicit learning during the acquisition and retrieval phases in an animal model of central post-stroke pain. Behav Brain Res. (2017) 317:251–62. doi: 10.1016/j.bbr.2016.09.053
13. Chen D, Wang J, Xing Y, Jia P, Zhang Y, Wang J, et al. Behavioral assessment of post-stroke depression and anxiety in rodents. Brain Hemorrh. (2020) 1:105–11. doi: 10.1016/j.hest.2020.02.004
14. Lu HF, Xu CY, Zhang L, Gan L, Chen C, Yan MY, et al. A new central post-stroke pain rat model: autologous blood injected thalamic hemorrhage involved increased expression of P2X4 receptor. Neurosci Lett. (2018) 687:124–30. doi: 10.1016/j.neulet.2018.09.023
15. Lu H-C, Chang W-J, Kuan Y-H, Huang AC-W, Shyu BC. A [14 C]iodoantipyrine study of inter-regional correlations of neural substrates following central post-stroke pain in rats. Mol Pain. (2015) 11:s12990–015–0006. doi: 10.1186/s12990-015-0006-5
16. Singhmar P, Huo X, Li Y, Dougherty PM, Mei F, Cheng X, et al. Orally active Epac inhibitor reverses mechanical allodynia and loss of intraepidermal nerve fibers in a mouse model of chemotherapy-induced peripheral neuropathy. Pain. (2018) 159:884–93. doi: 10.1097/j.pain.0000000000001160
17. Cai W, Wu S, Pan Z, Xiao J, Li F, Cao J, et al. Disrupting interaction of PSD-95 with nNOS attenuates hemorrhage-induced thalamic pain. Neuropharmacology. (2018) 141:238–48. doi: 10.1016/j.neuropharm.2018.09.003
18. Hanada T, Kurihara T, Tokudome M, Tokimura H, Arita K, Miyata A. Development and pharmacological verification of a new mouse model of central post-stroke pain. Neurosci Res. (2014) 78:72–80. doi: 10.1016/j.neures.2013.09.005
19. Bergeson SE, Blanton H, Martinez JM, Curtis DC, Sherfey C, Seegmiller B, et al. Binge ethanol consumption increases inflammatory pain responses and mechanical and cold sensitivity: tigecycline treatment efficacy shows sex differences. Alcoh Clin Exp Res. (2016) 40:2506–15. doi: 10.1111/acer.13252
20. Nieto FR, Cendán CM, Sánchez-Fernández C, Cobos EJ, Entrena JM, Tejada MA, et al. Role of sigma-1 receptors in paclitaxel-Induced neuropathic pain in mice. J Pain. (2012) 13:1107–21. doi: 10.1016/j.jpain.2012.08.006
21. Michot B, Kayser V, Bastian G, Bourgoin S, Hamon M. Differential pharmacological alleviation of oxaliplatin-induced hyperalgesia/allodynia at cephalic versus extra-cephalic level in rodents. Neuropharmacology. (2014) 79:432–43. doi: 10.1016/j.neuropharm.2013.12.011
22. Wang H, Li X, Zhangsun D, Yu G, Su R, Luo S. The alpha9alpha10 nicotinic acetylcholine receptor antagonist alphaO-Conotoxin geXIVA[1,2] alleviates and reverses chemotherapy-Induced neuropathic pain. Mar Drugs. (2019) 17:265. doi: 10.3390/md17050265
23. Tian GH, Tao SS, Chen MT, Li YS, Li YP, Shang HC, et al. Electroacupuncture treatment alleviates central poststroke pain by inhibiting brain neuronal apoptosis and aberrant astrocyte activation. Neural Plast. (2016) 2016:1437148. doi: 10.1155/2016/1437148
24. Cejas PJ, Martinez M, Karmally S, McKillop M, McKillop J, Plunkett JA, et al. Lumbar transplant of neurons genetically modified to secrete brain-derived neurotrophic factor attenuates allodynia and hyperalgesia after sciatic nerve constriction. Pain. (2000) 86:195–210. doi: 10.1016/S0304-3959(00)00245-1
25. Liu L, Ma SH, Xia LJ. The influence of thiamin on the efficacy of pregabalin in rats with spinal nerve ligation (SNL)-induced neuropathic pain. Neurol Res. (2016) 38:717–24. doi: 10.1080/01616412.2016.1188550
26. Brenner DS, Golden JP, Vogt SK, Gereau RW. A simple and inexpensive method for determining cold sensitivity and adaptation in mice. J Visual Exp. (2015) 97:e52640. doi: 10.3791/52640
27. Yang F, Fu H, Lu Y-F, Wang X-L, Yang Y, Yang F, et al. Post-stroke pain hypersensitivity induced by experimental thalamic hemorrhage in rats is region-specific and demonstrates limited efficacy of gabapentin. Neurosci Bull. (2014) 30:887–902. doi: 10.1007/s12264-014-1477-5
28. Kuan YH, Shih HC, Tang SC, Jeng JS, Shyu BC. Targeting P(2)X(7) receptor for the treatment of central post-stroke pain in a rodent model. Neurobiol Dis. (2015) 78:134–45. doi: 10.1016/j.nbd.2015.02.028
29. Chaplan SR, Pogrel JW, Yaksh TL. Role of voltage-dependent calcium channel subtypes in experimental tactile allodynia. J Pharmacol Exp Ther. (1994) 269:1117–23.
30. Singh N, Bansal Y, Bhandari R, Marwaha L, Singh R, Chopra K, et al. Resveratrol protects against ICV collagenase-induced neurobehavioral and biochemical deficits. J Inflamm. (2017) 14:14. doi: 10.1186/s12950-017-0158-3
31. Bove G. Mechanical sensory threshold testing using nylon monofilaments: The pain field's “Tin Standard”. Pain. (2006) 124:13–7. doi: 10.1016/j.pain.2006.06.020
32. Bradman MJ, Ferrini F, Salio C, Merighi A. Practical mechanical threshold estimation in rodents using von Frey hairs/Semmes-Weinstein monofilaments: towards a rational method. J Neurosci Methods. (2015) 255:92–103. doi: 10.1016/j.jneumeth.2015.08.010
33. Manering NA, Reuter T, Ihmsen H, Yeomans DC, Tzabazis A. High-dose remifentanil prevents development of thermal hyperalgesia in a neuropathic pain model. Br J Anaesth. (2013) 110:287–92. doi: 10.1093/bja/aes360
34. Wu H, Wu T, Han X, Wan J, Jiang C, Chen W, et al. Cerebroprotection by the neuronal pGE2 receptor eP2 after intracerebral hemorrhage in middle-aged mice. J Cerebral Blood Flow Metab. (2016) 37:39–51. doi: 10.1177/0271678X15625351
35. Akpan N, Serrano-Saiz E, Zacharia BE, Otten ML, Ducruet AF, Snipas SJ, et al. Intranasal delivery of caspase-9 inhibitor reduces caspase-6-Dependent axon/Neuron loss and improves neurological function after stroke. J Neurosci. (2011) 31:8894–904. doi: 10.1523/JNEUROSCI.0698-11.2011
36. Zhang L, Zhang ZG, Schallert T, Jiang Q, Arniego P, Li Q, et al. A test for detecting long-term sensorimotor dysfunction in the mouse after focal cerebral ischemia. J Neurosci Methods. (2002) 117:207–14. doi: 10.1016/S0165-0270(02)00114-0
37. Hua Y, Schallert T, Keep RF, Wu J, Hoff JT, Xi G. Behavioral tests after intracerebral hemorrhage in the rat. Stroke. (2002) 33:2478–84. doi: 10.1161/01.STR.0000032302.91894.0F
38. Chen J, Zhang C, Jiang H, Li Y, Zhang L, Robin A, et al. Atorvastatin induction of vEGF and bDNF promotes brain plasticity after stroke in mice. J Cerebral Blood Flow Metab. (2005) 25:281–90. doi: 10.1038/sj.jcbfm.9600034
39. Shen F, Jiang L, Han F, Degos V, Chen S, Su H. Increased inflammatory response in old mice is associated with more severe neuronal injury at the acute stage of ischemic stroke. Aging Dis. (2019) 10:12–22.
40. Montoya CP, Campbell-Hope LJ, Pemberton KD, Dunnett SB. The “staircase test”: a measure of independent forelimb reaching and grasping abilities in rats. J Neurosci Methods. (1991) 36:219. doi: 10.1016/0165-0270(91)90048-5
41. Schallert T. Behavioral tests for preclinical intervention assessment. NeuroRX. (2006) 3:497–504. doi: 10.1016/j.nurx.2006.08.001
42. Chang C-F, Wan J, Li Q, Renfroe SC, Heller NM, Wang J. Alternative activation-skewed microglia/macrophages promote hematoma resolution in experimental intracerebral hemorrhage. Neurob Dis. (2017) 103:54–69. doi: 10.1016/j.nbd.2017.03.016
43. Pan C, Liu N, Zhang P, Wu Q, Deng H, Xu F, et al. EGb761 ameliorates neuronal apoptosis and promotes angiogenesis in experimental intracerebral hemorrhage via rSK1/GSK3beta pathway. Mol Neurobiol. (2018) 55:1556–67. doi: 10.1007/s12035-016-0363-8
44. Zhang SZ, Wang QQ, Yang QQ, Gu HY, Yin YQ, Li YD, et al. NG2 glia regulate brain innate immunity via tGF-beta2/TGFBR2 axis. BMC Med. (2019) 17:204. doi: 10.1186/s12916-019-1439-x
45. Xu F, Shen G, Su Z, He Z, Yuan L. Glibenclamide ameliorates the disrupted blood–brain barrier in experimental intracerebral hemorrhage by inhibiting the activation of nLRP3 inflammasome. Brain Behav. (2019) 9:e01254. doi: 10.1002/brb3.1254
46. Lee JH, Chang YS, Ahn SY, Sung SI, Park WS. Dexamethasone does not prevent hydrocephalus after severe intraventricular hemorrhage in newborn rats. PLoS ONE. (2018) 13:e0206306. doi: 10.1371/journal.pone.0206306
47. Taylor RA, Chang C-F, Goods BA, Hammond MD, Grory BM, Ai Y, et al. TGF-β1 modulates microglial phenotype and promotes recovery after intracerebral hemorrhage. J Clin Invest. (2017) 127:280–92. doi: 10.1172/JCI88647
48. López-Valdés HE, Clarkson AN, Ao Y, Charles AC, Carmichael ST, Sofroniew MV, et al. Memantine enhances recovery from stroke. Stroke. (2014) 45:2093–100. doi: 10.1161/STROKEAHA.113.004476
49. Sherin A, Peeyush KT, Jayanarayanan S, Amee KK, Paulose CS. Decreased cholinergic receptor expression in the striatum: motor function deficit in hypoglycemic and diabetic rats. Cell Mol Neurobiol. (2012) 32:83–93. doi: 10.1007/s10571-011-9738-6
50. Soman S, Anju TR, Jayanarayanan S, Antony S, Paulose CS. Impaired motor learning attributed to altered aMPA receptor function in the cerebellum of rats with temporal lobe epilepsy: ameliorating effects of withania somnifera and withanolide a. Epilepsy Behav. (2013) 27:484–91. doi: 10.1016/j.yebeh.2013.01.007
51. Bland ST, Schallert T, Strong R, Aronowski J, Grotta JC, Feeney DM. Early exclusive use of the affected forelimb after moderate transient focal ischemia in rats: functional and anatomic outcome. Stroke. (2000) 31:1144–52. doi: 10.1161/01.STR.31.5.1144
52. Ishida A, Tamakoshi K, Hamakawa M, Shimada H, Nakashima H, Masuda T, et al. Early onset of forced impaired forelimb use causes recovery of forelimb skilled motor function but no effect on gross sensory-motor function after capsular hemorrhage in rats. Behav Brain Res. (2011) 225:126–34. doi: 10.1016/j.bbr.2011.06.036
53. García-Alías G, Barkhuysen S, Buckle M, Fawcett JW. Chondroitinase aBC treatment opens a window of opportunity for task-specific rehabilitation. Nat Neurosci. (2009) 12:1145–51. doi: 10.1038/nn.2377
54. Li Q, Han X, Lan X, Gao Y, Wan J, Durham F, et al. Inhibition of neuronal ferroptosis protects hemorrhagic brain. JCI Insight. (2017) 2:90777. doi: 10.1172/jci.insight.90777
55. Vavers E, Zvejniece L, Svalbe B, Volska K, Makarova E, Liepinsh E, et al. The neuroprotective effects of r-phenibut after focal cerebral ischemia. Pharmacol Res. (2016) 113:796–801. doi: 10.1016/j.phrs.2015.11.013
56. Zhu W, Gao Y, Wan J, Lan X, Han X, Zhu S, et al. Changes in motor function, cognition, and emotion-related behavior after right hemispheric intracerebral hemorrhage in various brain regions of mouse. Brain Behav Immunity. (2018) 69:568–81. doi: 10.1016/j.bbi.2018.02.004
57. Zhu W, Gao Y, Chang C-F, Wan J-R, Zhu S-S, Wang J. Mouse models of intracerebral hemorrhage in ventricle, cortex, and hippocampus by injections of autologous blood or collagenase. PLoS ONE. (2014) 9:e97423. doi: 10.1371/journal.pone.0097423
58. James ML, Wang H, Cantillana V, Lei B, Kernagis DN, Dawson HN, et al. TT-301 inhibits microglial activation and improves outcome after central nervous system injury in adult mice. Anesthesiology. (2012) 116:1299–311. doi: 10.1097/ALN.0b013e318253a02a
59. Krafft PR, McBride DW, Lekic T, Rolland WB, Mansell CE, Ma Q, et al. Correlation between subacute sensorimotor deficits and brain edema in two mouse models of intracerebral hemorrhage. Behav Brain Res. (2014) 264:151–60. doi: 10.1016/j.bbr.2014.01.052
60. Beray-Berthat V, Delifer C, Besson VC, Girgis H, Coqueran B, Plotkine M, et al. Long-term histological and behavioural characterisation of a collagenase-induced model of intracerebral haemorrhage in rats. J Neurosci Methods. (2010) 191:180–90. doi: 10.1016/j.jneumeth.2010.06.025
61. Matsushita H, Hijioka M, Hisatsune A, Isohama Y, Shudo K, Katsuki H. Natural and synthetic retinoids afford therapeutic effects on intracerebral hemorrhage in mice. Eur J Pharmacol. (2012) 683:125–31. doi: 10.1016/j.ejphar.2012.03.023
62. Kinoshita K, Ohtomo R, Takase H, Hamanaka G, Chung KK, Lok J, et al. Different responses after intracerebral hemorrhage between young and early middle-aged mice. Neurosci Lett. (2020) 735:135249. doi: 10.1016/j.neulet.2020.135249
63. Sato C, Tanji K, Shimoyama S, Chiba M, Mikami M, Koeda S, et al. Effects of voluntary and forced exercises on motor function recovery in intracerebral hemorrhage rats. Neuroreport. (2020) 31:189–96. doi: 10.1097/WNR.0000000000001396
64. MacLellan CL, Auriat AM, McGie SC, Yan RH, Huynh HD, De Butte MF, et al. Gauging recovery after hemorrhagic stroke in rats: implications for cytoprotection studies. J Cereb Blood Flow Metab. (2006) 26:1031–42. doi: 10.1038/sj.jcbfm.9600255
65. Tamakoshi K, Ishida A, Takamatsu Y, Hamakawa M, Nakashima H, Shimada H, et al. Motor skills training promotes motor functional recovery and induces synaptogenesis in the motor cortex and striatum after intracerebral hemorrhage in rats. Behav Brain Res. (2014) 260:34–43. doi: 10.1016/j.bbr.2013.11.034
66. Duricki DA, Drndarski S, Bernanos M, Wood T, Bosch K, Chen Q, et al. Stroke recovery in rats after 24-Hour-Delayed intramuscular neurotrophin-3 infusion. Ann Neurol. (2019) 85:32–46. doi: 10.1002/ana.25386
67. Liddle L, Reinders R, South S, Blacker D, Knuckey N, Colbourne F, et al. Poly-arginine-18 peptides do not exacerbate bleeding, or improve functional outcomes following collagenase-induced intracerebral hemorrhage in the rat. PLoS ONE. (2019) 14:e0224870. doi: 10.1371/journal.pone.0224870
68. Caliaperumal J, Wowk S, Jones S, Ma Y, Colbourne F. Bipyridine, an iron chelator, does not lessen intracerebral iron-induced damage or improve outcome after intracerebral hemorrhagic stroke in rats. Transl Stroke Res. (2013) 4:719–28. doi: 10.1007/s12975-013-0272-3
69. Sun J, Wei ZZ, Gu X, Zhang JY, Zhang Y, Li J, et al. Intranasal delivery of hypoxia-preconditioned bone marrow-derived mesenchymal stem cells enhanced regenerative effects after intracerebral hemorrhagic stroke in mice. Exp Neurol. (2015) 272:78–87. doi: 10.1016/j.expneurol.2015.03.011
70. Singh N, Ma B, Leonardo CC, Ahmad AS, Narumiya S, Dore S. Role of pGE(2) eP1 receptor in intracerebral hemorrhage-induced brain injury. Neurotox Res. (2013) 24:549–59. doi: 10.1007/s12640-013-9410-7
71. Lu X, Chen-Roetling J, Regan RF. Systemic hemin therapy attenuates blood–brain barrier disruption after intracerebral hemorrhage. Neurob Dis. (2014) 70:245–51. doi: 10.1016/j.nbd.2014.06.005
72. Chen-Roetling J, Cai Y, Regan RF. Neuroprotective effect of heme oxygenase-2 knockout in the blood injection model of intracerebral hemorrhage. BMC Res Notes. (2014) 7:561. doi: 10.1186/1756-0500-7-561
73. Chen J, Li Y, Wang L, Zhang Z, Lu D, Lu M, et al. Therapeutic benefit of intravenous administration of bone marrow stromal cells after cerebral ischemia in rats. Stroke. (2001) 32:1005–11. doi: 10.1161/01.STR.32.4.1005
74. Li Q, Wan J, Lan X, Han X, Wang Z, Wang J. Neuroprotection of brain-permeable iron chelator vK-28 against intracerebral hemorrhage in mice. J Cerebral Blood Flow Metab. (2017) 37:3110–23. doi: 10.1177/0271678X17709186
75. MacLellan CL, Langdon KD, Churchill KP, Granter-Button S, Corbett D. Assessing cognitive function after intracerebral hemorrhage in rats. Behav Brain Res. (2009) 198:321–8. doi: 10.1016/j.bbr.2008.11.004
76. Ruan J, Yao Y. Behavioral tests in rodent models of stroke. Brain Hemorr. (2020) 1:171–84. doi: 10.1016/j.hest.2020.09.001
77. Schaar KL, Brenneman MM, Savitz SI. Functional assessments in the rodent stroke model. Exp Transl Stroke Med. (2010) 2:13. doi: 10.1186/2040-7378-2-13
78. Schallert T, Upchurch M, Lobaugh N, Farrar SB, Spirduso WW, Gilliam P, et al. Tactile extinction: distinguishing between sensorimotor and motor asymmetries in rats with unilateral nigrostriatal damage. Pharmacol Biochem Behav. (1982) 16:455. doi: 10.1016/0091-3057(82)90452-X
79. Balkaya M, Krober J, Gertz K, Peruzzaro S, Endres M. Characterization of long-term functional outcome in a murine model of mild brain ischemia. J Neurosci Methods. (2013) 213:179–87. doi: 10.1016/j.jneumeth.2012.12.021
80. Baird AL, Meldrum A, Dunnett SB. The staircase test of skilled reaching in mice. Brain Res Bull. (2001) 54:243−50. doi: 10.1016/S0361-9230(00)00457-3
81. Freret T, Valable S, Chazalviel L, Saulnier R, Mackenzie ET, Petit E, et al. Delayed administration of deferoxamine reduces brain damage and promotes functional recovery after transient focal cerebral ischemia in the rat. Europ J Neurosci. (2006) 23:1757–65. doi: 10.1111/j.1460-9568.2006.04699.x
82. Rattka M, Fluri F, Krstić M, Asan E, Volkmann J. A novel approach to assess motor outcome of deep brain stimulation effects in the hemiparkinsonian rat: staircase and cylinder test. J Vis Exp. (2016) 111:e53951. doi: 10.3791/53951
83. Hu Y, Liu N, Zhang P, Pan C, Zhang Y, Tang Y, et al. Preclinical studies of stem cell transplantation in intracerebral hemorrhage: a systemic review and meta-Analysis. Mol Neurob. (2016) 53:5269–77. doi: 10.1007/s12035-015-9441-6
84. Li Q, Han X, Lan X, Hong X, Li Q, Gao Y, et al. Inhibition of tPA-induced hemorrhagic transformation involves adenosine a2b receptor activation after cerebral ischemia. Neurob Dis. (2017) 108:173–82. doi: 10.1016/j.nbd.2017.08.011
85. Lee HJ, Kim KS, Kim EJ, Choi HB, Lee KH, Park IH, et al. Brain transplantation of immortalized human neural stem cells promotes functional recovery in mouse intracerebral hemorrhage stroke model. Stem Cells. (2007) 25:1204–12. doi: 10.1634/stemcells.2006-0409
86. Chen CC, Wang YL, Chang CP. Remarkable cell recovery from cerebral ischemia in rats using an adaptive escalator-based rehabilitation mechanism. PLoS ONE. (2019) 14:e0223820. doi: 10.1371/journal.pone.0223820
87. Jin J, Zhou F, Zhu J, Zeng W, Liu Y. MiR-26a inhibits the inflammatory response of microglia by targeting hMGA2 in intracerebral hemorrhage. J Int Med Res. (2020) 48:300060520929615. doi: 10.1177/0300060520929615
88. Singh N, Bansal Y, Bhandari R, Marwaha L, Singh R, Chopra K, et al. Naringin reverses neurobehavioral and biochemical alterations in intracerebroventricular collagenase-Induced intracerebral hemorrhage in rats. Pharmacology. (2017) 100:172–87. doi: 10.1159/000453580
90. Luong TN, Carlisle HJ, Southwell A, Patterson PH. Assessment of motor balance and coordination in mice using the balance beam. J Vis Exp. (2011) 49:e2376. doi: 10.3791/2376
91. Shiotsuki H, Yoshimi K, Shimo Y, Funayama M, Takamatsu Y, Ikeda K, et al. A rotarod test for evaluation of motor skill learning. J Neurosci Methods. (2010) 189:180–5. doi: 10.1016/j.jneumeth.2010.03.026
92. Roome RB, Vanderluit JL. Paw-dragging: a novel, sensitive analysis of the mouse cylinder test. JoVE. (2015) 98:e52701. doi: 10.3791/52701
93. Nampoothiri SS, Potluri T, Subramanian H, Krishnamurthy RG. Rodent gymnastics: neurobehavioral assays in ischemic stroke. Mol Neurobiol. (2017) 54:6750–61. doi: 10.1007/s12035-016-0195-6
94. Zalewska K, Pietrogrande G, Ong LK, Abdolhoseini M, Kluge M, Johnson SJ, et al. Sustained administration of corticosterone at stress-like levels after stroke suppressed glial reactivity at sites of thalamic secondary neurodegeneration. Brain Behav Immunity. (2018) 69:210–22. doi: 10.1016/j.bbi.2017.11.014
95. Li P, Tang T, Liu T, Zhou J, Cui H, He Z, et al. Systematic analysis of tRNA-Derived small rNAs reveals novel potential therapeutic targets of traditional chinese medicine (Buyang-Huanwu-Decoction) on intracerebral hemorrhage. Int J Biol Sci. (2019) 15:895–908. doi: 10.7150/ijbs.29744
96. Liu P, Song XC, Yang XS, Cao QL, Tang YY, Liu XD, et al. A preclinical model to assess brain recovery after acute stroke in rats. J Vis Exp. (2019) 153:e60166. doi: 10.3791/60166
97. Lin XJ, Wen S, Deng LX, Dai H, Du X, Chen C, et al. Spinal cord lateral hemisection and asymmetric behavioral assessments in adult rats. J Vis Exp. (2020) 157:e57126. doi: 10.3791/57126
98. Hua Y, Nakamura T, Keep RF, Wu J, Schallert T, Hoff JT, et al. Long-term effects of experimental intracerebral hemorrhage: the role of iron. J Neurosurg. (2006) 104:305. doi: 10.3171/jns.2006.104.2.305
99. Okauchi M, Hua Y, Keep RF, Morgenstern LB, Xi G. Effects of deferoxamine on intracerebral hemorrhage-Induced brain injury in aged rats. Stroke. (2009) 40:1858–63. doi: 10.1161/STROKEAHA.108.535765
100. Dadgar M, Pouramir M, Dastan Z, Ghasemi-Kasman M, Ashrafpour M, Moghadamnia AA, et al. Arbutin attenuates behavioral impairment and oxidative stress in an animal model of parkinson's disease. Avic J Phytomed. (2018) 8:533−42.
101. Han X, Lan X, Li Q, Gao Y, Zhu W, Cheng T, et al. Inhibition of prostaglandin e2 receptor eP3 mitigates thrombin-induced brain injury. J Cerebral Blood Flow Metab. (2016) 36:1059–74. doi: 10.1177/0271678X15606462
102. Manaenko A, Lekic T, Sozen T, Tsuchiyama R, Zhang JH, Tang J. Effect of gap junction inhibition on intracerebral hemorrhage-induced brain injury in mice. Neurol Res. (2009) 31:173−8. doi: 10.1179/174313209X393591
103. Hoffman E, Winder SJ. A modified wire hanging apparatus for small animal muscle function testing. PLoS Curr. (2016) 8:ecurrents.md.1e2bec4e78697b7b0ff80ea25a1d38be. doi: 10.1371/currents.md.1e2bec4e78697b7b0ff80ea25a1d38be
104. Song Y, Yang Y, Cui Y, Gao J, Wang K, Cui J. Lipoxin a4 methyl ester reduces early brain injury by inhibition of the nuclear factor kappa b (NF-κB)-Dependent matrix metallopeptidase 9 (MMP-9) pathway in a rat model of intracerebral hemorrhage. Med Sci Monit. (2019) 25:1838–47. doi: 10.12659/MSM.915119
105. Chen C, Yao L, Cui J, Liu B. Fisetin protects against intracerebral hemorrhage-Induced neuroinflammation in aged mice. Cerebrovasc Dis. (2018) 45:154–61. doi: 10.1159/000488117
106. Zhang Q, Shang X, Hao M, Zheng M, Li Y, Liang Z, et al. Effects of human umbilical cord mesenchymal stem cell transplantation combined with minimally invasive hematoma aspiration on intracerebral hemorrhage in rats. Am J Transl Res. (2015) 7:2176–86.
107. Galho AR, Cordeiro MF, Ribeiro SA, Marques MS, Antunes MF, Luz DC, et al. Protective role of free and quercetin-loaded nanoemulsion against damage induced by intracerebral haemorrhage in rats. Nanotechnology. (2016) 27:175101. doi: 10.1088/0957-4484/27/17/175101
108. Hayashi K, Hasegawa Y, Takemoto Y, Cao C, Mukasa A, Kim-Mitsuyama S. Enhanced oxidative stress contributes to worse prognosis and delayed neurofunctional recovery after striatal intracerebral hemorrhage in 5XFAD mice. Eur J Neurosci. (2020) 51:1806–14. doi: 10.1111/ejn.14596
109. Takamatsu Y, Tamakoshi K, Waseda Y, Ishida K. Running exercise enhances motor functional recovery with inhibition of dendritic regression in the motor cortex after collagenase-induced intracerebral hemorrhage in rats. Behav Brain Res. (2016) 300:56–64. doi: 10.1016/j.bbr.2015.12.003
110. Fingas M, Penner M, Silasi G, Colbourne F. Treatment of intracerebral hemorrhage in rats with 12 h, 3 days and 6 days of selective brain hypothermia. Exp Neurol. (2009) 219:156–62. doi: 10.1016/j.expneurol.2009.05.007
111. Tamakoshi K, Maeda M, Nakamura S, Murohashi N. Very early exercise rehabilitation after intracerebral hemorrhage promotes inflammation in the brain. Neurorehabil Neural Repair. (2021) :15459683211006337. doi: 10.1177/15459683211006337
112. Metz GA, Whishaw IQ. Cortical and subcortical lesions impair skilled walking in the ladder rung walking test: a new task to evaluate fore- and hindlimb stepping, placing, and co-ordination. J Neurosci Methods. (2002) 115:169–79. doi: 10.1016/S0165-0270(02)00012-2
113. MacLellan C, Shuaib A, Colbourne F. Failure of delayed and prolonged hypothermia to favorably affect hemorrhagic stroke in rats. Brain Res. (2002) 958:192–200. doi: 10.1016/S0006-8993(02)03702-2
114. Chen-Roetling J, Song W, Schipper HM, Regan CS, Regan RF. Astrocyte overexpression of heme oxygenase-1 improves outcome after intracerebral hemorrhage. Stroke. (2015) 46:1093–8. doi: 10.1161/STROKEAHA.115.008686
115. Alonso G. NG2 proteoglycan-expressing cells of the adult rat brain: possible involvement in the formation of glial scar astrocytes following stab wound. Glia. (2005) 49:318–38. doi: 10.1002/glia.20121
116. Jiang Y, Fan X, Yu Z, Cheng C, Wang XS, Lo EH, et al. Low dose tPA plus annexin a2 combination attenuates tPA delayed treatment-associated hemorrhage and improves recovery in rat embolic focal stroke. Neurosci Lett. (2015) 602:73–8. doi: 10.1016/j.neulet.2015.06.050
117. Gao C, Qian Y, Huang J, Wang D, Su W, Wang P, et al. A three-Day consecutive fingolimod administration improves neurological functions and modulates multiple immune responses of cCI mice. Mol Neurob. (2017) 54:8348–60. doi: 10.1007/s12035-016-0318-0
118. Lei B, Mace B, Bellows ST, Sullivan PM, Vitek MP, Laskowitz DT, et al. Interaction between sex and apolipoprotein e genetic background in a murine model of intracerebral hemorrhage. Transl Stroke Res. (2012) 3:94–101. doi: 10.1007/s12975-012-0176-7
119. Lule S, Wu L, McAllister LM, Edmiston WJ, Chung JY, Levy E, et al. Genetic inhibition of receptor interacting protein kinase-1 reduces cell death and improves functional outcome after intracerebral hemorrhage in mice. Stroke. (2017) 48:2549–56. doi: 10.1161/STROKEAHA.117.017702
120. Washida K, Ihara M, Nishio K, Fujita Y, Maki T, Yamada M, et al. Nonhypotensive dose of telmisartan attenuates cognitive impairment partially due to peroxisome proliferator-Activated receptor-γ activation in mice with chronic cerebral hypoperfusion. Stroke. (2010) 41:1798–806. doi: 10.1161/STROKEAHA.110.583948
121. Akwa Y, Ladurelle N, Covey D, Baulieu E. The synthetic enantiomer of pregnenolone sulfate is very active on memory in rats and mice, even more so than its physiological neurosteroid counterpart: distinct mechanisms? Proc Natl Acad Sci USA. (2001) 98:14033–7. doi: 10.1073/pnas.241503698
122. Lifshitz V, Weiss R, Benromano T, Kfir E, Blumenfeld-Katzir T, Tempel-Brami C, et al. Immunotherapy of cerebrovascular amyloidosis in a transgenic mouse model. Neurob Aging. (2012) 33:432.e1-.e13. doi: 10.1016/j.neurobiolaging.2011.01.006
123. Schuch CP, Jeffers MS, Antonescu S, Nguemeni C, Gomez-Smith M, Pereira LO, et al. Enriched rehabilitation promotes motor recovery in rats exposed to neonatal hypoxia-ischemia. Behav Brain Res. (2016) 304:42–50. doi: 10.1016/j.bbr.2016.02.010
124. Lekic T, Rolland W, Hartman R, Kamper J, Suzuki H, Tang J, et al. Characterization of the brain injury, neurobehavioral profiles, and histopathology in a rat model of cerebellar hemorrhage. Exp Neurol. (2011) 227:96–103. doi: 10.1016/j.expneurol.2010.09.017
125. James ML, Wang H, Venkatraman T, Song P, Lascola CD, Laskowitz DT. Brain natriuretic peptide improves long-term functional recovery after acute cNS injury in mice. J Neurotrauma. (2010) 27:217–28. doi: 10.1089/neu.2009.1022
126. Holscher C. Stress impairs performance in spatial water maze learning tasks. Behav Brain Res. (1999) 100:225–35. doi: 10.1016/S0166-4328(98)00134-X
127. Barnhart CD, Yang D, Lein PJ. Using the morris water maze to assess spatial learning and memory in weanling mice. PLoS ONE. (2015) 10:e0124521. doi: 10.1371/journal.pone.0124521
128. Vorhees CV, Williams MT. Value of water mazes for assessing spatial and egocentric learning and memory in rodent basic research and regulatory studies. Neurotoxicol Teratol. (2014) 45:75–90. doi: 10.1016/j.ntt.2014.07.003
129. Bromley-Brits K, Deng Y, Song W. Morris water maze test for learning and memory deficits in alzheimer's disease model mice. J Vis Exp. (2011) 53:e2920. doi: 10.3791/2920
130. Fernandez-Castaneda A, Gaultier A. Adult oligodendrocyte progenitor cells - multifaceted regulators of the cNS in health and disease. Brain Behav Immun. (2016) 57:1–7. doi: 10.1016/j.bbi.2016.01.005
131. Kraeuter AK, Guest PC, Sarnyai Z. The y-Maze for assessment of spatial working and reference memory in mice. Methods Mol Biol. (2019) 1916:105–11. doi: 10.1007/978-1-4939-8994-2_10
132. Miedel CJ, Patton JM, Miedel AN, Miedel ES, Levenson JM. Assessment of spontaneous alternation, novel object recognition and limb clasping in transgenic mouse models of amyloid-β and tau neuropathology. J Vis Exp. (2017) 123:e55523. doi: 10.3791/55523
133. Gudapati K, Singh A, Clarkson-Townsend D, Feola AJ, Allen RS. Behavioral assessment of visual function via optomotor response and cognitive function via y-Maze in diabetic rats. J Vis Exp. (2020) 164:e61806. doi: 10.3791/61806
134. Yang JW, Wang XR, Ma SM, Yang NN, Li QQ, Liu CZ. Acupuncture attenuates cognitive impairment, oxidative stress and nF-kappaB activation in cerebral multi-infarct rats. Acupunct Med. (2019) 37:283–91. doi: 10.1136/acupmed-2017-011491
135. Antunes M, Biala GJCp. The novel object recognition memory: neurobiology, test procedure, and its modifications. (2012) 13:93–110. doi: 10.1007/s10339-011-0430-z
136. Lueptow LM. Novel object recognition test for the investigation of learning and memory in mice. J Vis Exp. (2017) 126:e55718. doi: 10.3791/55718
137. Buenrostro-Jáuregui M, Rodríguez-Serrano LM, Chávez-Hernández ME, Tapia-de-Jesús A, Mata-Luevanos J, Mata F, et al. Simultaneous monitoring of wireless electrophysiology and memory behavioral test as a tool to study hippocampal neurogenesis. J Vis Exp. (2020) 162:e61494. doi: 10.3791/61494
138. Han Y-X, Tao C, Gao X-R, Wang L-L, Jiang F-H, Wang C, et al. BDNF-Related imbalance of copine 6 and synaptic plasticity markers couples with depression-Like behavior and immune activation in cUMS rats. Front Neurosci. (2018) 12:731. doi: 10.3389/fnins.2018.00731
139. Shimizu T, Minami C, Mitani A. Effect of electrical stimulation of the infralimbic and prelimbic cortices on anxiolytic-like behavior of rats during the elevated plus-maze test, with particular reference to multiunit recording of the behavior-associated neural activity. Behav Brain Res. (2018) 353:168–75. doi: 10.1016/j.bbr.2018.07.005
140. Peng J, Liu Y, Umpierre AD, Xie M, Tian DS, Richardson JR, et al. Microglial p2Y12 receptor regulates ventral hippocampal cA1 neuronal excitability and innate fear in mice. Mol Brain. (2019) 12:71. doi: 10.1186/s13041-019-0492-x
141. Snyder JS, Soumier A, Brewer M, Pickel J, Cameron HA. Adult hippocampal neurogenesis buffers stress responses and depressive behaviour. Nature. (2011) 476:458–61. doi: 10.1038/nature10287
142. Ifergane G, Boyko M, Frank D, Shiyntum HN, Grinshpun J, Kuts R, et al. Biological and behavioral patterns of post-Stroke depression in rats. Can J Neurol Sci. (2018) 45:451–61. doi: 10.1017/cjn.2017.302
143. Tomida S, Mamiya T, Sakamaki H, Miura M, Aosaki T, Masuda M, et al. Usp46 is a quantitative trait gene regulating mouse immobile behavior in the tail suspension and forced swimming tests. Nat Genet. (2009) 41:688–95. doi: 10.1038/ng.344
144. Seibenhener ML, Wooten MC. Use of the open field maze to measure locomotor and anxiety-like behavior in mice. J Visual Exp. (2015) 96:e52434. doi: 10.3791/52434
145. Hartman R, Lekic T, Rojas H, Tang J, Zhang JH. Assessing functional outcomes following intracerebral hemorrhage in rats. Brain Res. (2009) 1280:148–57. doi: 10.1016/j.brainres.2009.05.038
146. Yankelevitch-Yahav R, Franko M, Huly A, Doron R. The forced swim test as a model of depressive-like behavior. JoVE. (2015) 97:e52587. doi: 10.3791/52587
147. Réus GZ, Stringari RB, Ribeiro KF, Cipriano AL, Panizzutti BS, Stertz L, et al. Maternal deprivation induces depressive-like behaviour and alters neurotrophin levels in the rat brain. Neurochem Res. (2011) 36:460–6. doi: 10.1007/s11064-010-0364-3
148. Zubcevic J, Watkins J, Perez PD, Colon-Perez LM, Long MT, Febo M, et al. MEMRI reveals altered activity in brain regions associated with anxiety, locomotion, and cardiovascular reactivity on the elevated plus maze in the wKY vs sHR rats. Brain Imaging Behav. (2018) 12:1318–31. doi: 10.1007/s11682-017-9798-4
149. Saito K, Kasai T, Nagura Y, Ito H, Kanazawa M, Fukudo S. Corticotropin-Releasing hormone receptor 1 antagonist blocks brain–Gut activation induced by colonic distention in rats. Gastroenterology. (2005) 129:1533–43. doi: 10.1053/j.gastro.2005.07.053
150. Carobrez AP, Bertoglio LJ. Ethological and temporal analyses of anxiety-like behavior: the elevated plus-maze model 20 years on. Neurosci Biobehav Rev. (2005) 29:1193–205. doi: 10.1016/j.neubiorev.2005.04.017
151. Komada M, Takao K, Miyakawa T. Elevated plus maze for mice. J Vis Exp. (2008) (22). doi: 10.3791/1088
152. Horii Y, McTaggart I, Kawaguchi M. Testing animal anxiety in rats: effects of open arm ledges and closed arm wall transparency in elevated plus maze test. J Vis Exp. (2018) 136:e56428. doi: 10.3791/56428
153. Zhu W, Cao F-S, Feng J, Chen H-W, Wan J-R, Lu Q, et al. NLRP3 inflammasome activation contributes to long-term behavioral alterations in mice injected with lipopolysaccharide. Neuroscience. (2017) 343:77–84. doi: 10.1016/j.neuroscience.2016.11.037
154. Zhao D, Xu X, Pan L, Zhu W, Fu X, Guo L, et al. Pharmacologic activation of cholinergic alpha7 nicotinic receptors mitigates depressive-like behavior in a mouse model of chronic stress. J Neuroinfl. (2017) 14:234. doi: 10.1186/s12974-017-1007-2
155. Cryan JF, Markou A, Lucki I. Assessing antidepressant activity in rodents: recent developments and future needs. Trends Pharm Sci. (2002) 23:238–45. doi: 10.1016/S0165-6147(02)02017-5
156. Liu MY, Yin CY, Zhu LJ, Zhu XH, Xu C, Luo CX, et al. Sucrose preference test for measurement of stress-induced anhedonia in mice. Nat Protoc. (2018) 13:1686–98. doi: 10.1038/s41596-018-0011-z
157. Burstein O, Doron R. The unpredictable chronic mild stress protocol for inducing anhedonia in mice. J Vis Exp. (2018) (140). doi: 10.3791/58184
158. Zeldetz V, Natanel D, Boyko M, Zlotnik A, Shiyntum HN, Grinshpun J, et al. A new method for inducing a depression-Like behavior in rats. J Vis Exp. (2018) 132:e57137. doi: 10.3791/57137
160. Yan HC, Cao X, Das M, Zhu XH, Gao TM. Behavioral animal models of depression. Neurosci Bull. (2010) 26:327–37. doi: 10.1007/s12264-010-0323-7
161. Sosa PM, de Souza MA, Mello-Carpes PB. Green tea and red tea fromCamellia sinensis partially prevented the motor deficits and striatal oxidative damage induced by hemorrhagic stroke in rats. Neural Plasticity. (2018) 2018:1–8. doi: 10.1155/2018/5158724
162. Porsolt RD, Le Pichon M, Jalfre M. Depression: a new animal model sensitive to antidepressant treatments. Nature. (1977) 266:730–2. doi: 10.1038/266730a0
163. Detke MJ, Lucki I. Detection of serotonergic and noradrenergic antidepressants in the rat forced swimming test: the effects of water depth. Behav Brain Res. (1996) 73:43–6. doi: 10.1016/0166-4328(96)00067-8
164. Abelaira HM, Reus GZ, Quevedo J. Animal models as tools to study the pathophysiology of depression. Rev Brasil Psiquiatria. (2013) 35(Suppl. 2):S112–S20. doi: 10.1590/1516-4446-2013-1098
165. Unal G, Canbeyli R. Psychomotor retardation in depression: a critical measure of the forced swim test. Behav Brain Res. (2019) 372:112047. doi: 10.1016/j.bbr.2019.112047
166. Bogdanova OV, Kanekar S, D'Anci KE, Renshaw PF. Factors influencing behavior in the forced swim test. Physiol Behav. (2013) 118:227–39. doi: 10.1016/j.physbeh.2013.05.012
167. Can A, Dao DT, Arad M, Terrillion CE, Piantadosi SC, Gould TD. The mouse forced swim test. J Vis Exp. (2012) 59:e3638. doi: 10.3791/3638
168. Koivunen RJ, Harno H, Tatlisumak T, Putaala J. Depression, anxiety, and cognitive functioning after intracerebral hemorrhage. Acta Neurol Scand. (2015) 132:179–84. doi: 10.1111/ane.12367
169. Suzuki M, Hayashi A, Sasamata M. Nicardipine, a calcium antagonist, does not aggravate intracerebral haemorrhage in an intracerebral haemorrhage model in rats. J Pharm Pharm. (2005) 57:483–8. doi: 10.1211/0022357055759
170. Camacho E, LoPresti MA, Bruce S, Lin D, Abraham M, Appelboom G, et al. The role of age in intracerebral hemorrhages. J Clin Neurosci. (2015) 22:1867–70. doi: 10.1016/j.jocn.2015.04.020
171. Rådholm K, Arima H, Lindley RI, Wang J, Tzourio C, Robinson T, et al. Older age is a strong predictor for poor outcome in intracerebral haemorrhage: the iNTERACT2 study. Age Ageing. (2015) 44:422–7. doi: 10.1093/ageing/afu198
172. Alexander GE, Ryan L, Bowers D, Foster TC, Bizon JL, Geldmacher DS, et al. Characterizing cognitive aging in humans with links to animal models. Front Aging Neurosci. (2012) 4:21. doi: 10.3389/fnagi.2012.00021
173. Ren H, Han R, Chen X, Liu X, Wan J, Wang L, et al. Potential therapeutic targets for intracerebral hemorrhage-associated inflammation: an update. J Cerebral Blood Flow Metab. (2020) 40:1752–68. doi: 10.1177/0271678X20923551
Keywords: anxiety, behavioral tests, cognition, depression, emotion, intracerebral hemorrhage, motor function, pain
Citation: Shi X, Bai H, Wang J, Wang J, Huang L, He M, Zheng X, Duan Z, Chen D, Zhang J, Chen X and Wang J (2021) Behavioral Assessment of Sensory, Motor, Emotion, and Cognition in Rodent Models of Intracerebral Hemorrhage. Front. Neurol. 12:667511. doi: 10.3389/fneur.2021.667511
Received: 15 February 2021; Accepted: 20 May 2021;
Published: 17 June 2021.
Edited by:
Xinchun Jin, Capital Medical University, ChinaReviewed by:
Sangeetha Sukumari Ramesh, Augusta University, United StatesCopyright © 2021 Shi, Bai, Wang, Wang, Huang, He, Zheng, Duan, Chen, Zhang, Chen and Wang. This is an open-access article distributed under the terms of the Creative Commons Attribution License (CC BY). The use, distribution or reproduction in other forums is permitted, provided the original author(s) and the copyright owner(s) are credited and that the original publication in this journal is cited, in accordance with accepted academic practice. No use, distribution or reproduction is permitted which does not comply with these terms.
*Correspondence: Xuemei Chen, Y2hlbnhtQHp6dS5lZHUuY24=; Jian Wang, amlhbndhbmcyMDIwQG91dGxvb2suY29t
†These authors have contributed equally to this work and share first authorship
Disclaimer: All claims expressed in this article are solely those of the authors and do not necessarily represent those of their affiliated organizations, or those of the publisher, the editors and the reviewers. Any product that may be evaluated in this article or claim that may be made by its manufacturer is not guaranteed or endorsed by the publisher.
Research integrity at Frontiers
Learn more about the work of our research integrity team to safeguard the quality of each article we publish.