- 1Department of Neurology, Emory University School of Medicine, Atlanta, GA, United States
- 2Emory Epilepsy Center, Atlanta, GA, United States
- 3Department of Pediatrics, Emory University School of Medicine, Atlanta, GA, United States
- 4Department of Neurology, University of Washington School of Medicine, Seattle, WA, United States
- 5Department of Biomedical Engineering, Georgia Institute of Technology and Emory University, Atlanta, GA, United States
- 6Department of Psychology and Psychiatry, Mayo Clinic, Jacksonville, FL, United States
- 7Department of Neurological Surgery, Mayo Clinic, Jacksonville, FL, United States
Mapping of cortical functions is critical for the best clinical care of patients undergoing epilepsy and tumor surgery, but also to better understand human brain function and connectivity. The purpose of this review is to explore existing and potential means of mapping higher cortical functions, including stimulation mapping, passive mapping, and connectivity analyses. We examine the history of mapping, differences between subdural and stereoelectroencephalographic approaches, and some risks and safety aspects, before examining different types of functional mapping. Much of this review explores the prospects for new mapping approaches to better understand other components of language, memory, spatial skills, executive, and socio-emotional functions. We also touch on brain-machine interfaces, philosophical aspects of aligning tasks to brain circuits, and the study of consciousness. We end by discussing multi-modal testing and virtual reality approaches to mapping higher cortical functions.
Introduction
Mapping of cortical functions in humans has provided substantial insights about the organization of the human forebrain. This review focuses on the mapping of higher cognitive functions, and will not address the mapping of primary sensory or primary motor cortices [recently covered elsewhere: (1)] nor the premotor or cingulate motor regions. We will describe the rationale for mapping in the context of its conceptual and historical development. We will then describe stimulation mapping and how it differs between subdural electrode stimulation and in the setting of stereoelectroenecephalography (SEEG). We will review language mapping and then describe broader applications of mapping to cognition and emotion and the potential for further research and technical development. Wherever relevant we will highlight controversies, key concepts, gaps in knowledge, and areas of present development.
Rationale and Opportunity for Intracranial Monitoring for Understanding Seizure Networks and Conducting Neurocognitive Mapping
When seizures continue despite thoughtful medical management, epilepsy surgery is considered, often in turn resulting in invasive electrophysiology with subdural or depth electrodes. The ultimate goal of such studies is to test hypotheses about the network and location of seizure onset with a view to resective surgery, tissue ablation, or neuromodulation (2). In order to localize areas of hyperexcitability, reproduce seizures and aura, and map cortical function, electrical stimulation can also be performed while intracranial electrodes are in place, with this method arguably having afforded the greatest body of knowledge about cortical function through the last ~80 years. For much of this time in North America, this has involved a large craniotomy and placement of a subdural grid of electrodes on the surface of the brain, sometimes augmented with strip or depth electrodes. In contrast, in Europe, the approach to invasive studies has grown out of a more clinical approach with semiologic analysis, ultimately relying on less invasive depth electrodes with the overall method referred to as SEEG (3). While both methods started out as acute approaches, limited to the operating room, both rapidly developed into continuous extra-operative recording.
SEEG has been used more extensively in Europe for many years (4), but has recently become popular in North America, particularly since the use of minimally invasive procedures has increased (5). These have included the use of laser interstitial thermal therapy [LITT (6)], focused ultrasound (7), and neuromodulatory procedures [e.g., vagal nerve stimulation (VNS), responsive neurostimulation (RNS), and deep brain stimulation (DBS) of the anterior nucleus of the thalamus [DSBS-ANT (8, 9)]. With these less invasive surgical options available, patients and neurosurgeons are reluctant to use a larger procedure for evaluative purposes if the treatment is going to ultimately be more restricted and less invasive in scope. In this manuscript, we will briefly review the history of invasive EEG techniques and explore both the strengths and weaknesses of these procedures. We will discuss the use of such techniques to better understand seizure networks and brain connectivity and to study cognitive and emotional processes, then propose a research agenda that improves clinical practice and furthers our understanding of neural circuitry. The latter goal could include the standardization and dissemination of assessment techniques and the development of new cognitive and emotional testing paradigms that build upon current brain-behavior theoretical models and make use of modern technologies (e.g., augmented and virtual reality, videography, eye-tracking).
History of SEEG and Neuropsychological Mapping
Historical Antecedents of SEEG
In the late eighteenth and early nineteenth century, studies of electricity and its relationship to biology were in their infancy, with debate between Volta and Galvani about intrinsic vs. extrinsic electricity and electrical stimulation of movement (10). This debate was largely synthesized and resolved by Humboldt as Galvani's nephew first stimulated a freshly executed human cadaver in 1802, giving rise to bodily movements, inspiring the public imagination and likely contributing to the creation of the Frankenstein story. Crude localization of function was best inspired by Thomas Willis, but more fine grained cerebral localization principally occurred in the nineteenth century with clinical-pathological correlations and early motor mapping by Fritsch and Hitzig in dogs (11), and a more precise topography described by Ferrier in non-human primates (12–14). These works culminated in the “The Functions of the Brain” in 1876 (15), with later correlations to some clinical observations by Hughlings Jackson (16).
In the clinical realm, this new appreciation of the organization of the cerebral cortex developed alongside the other technical and research advances, such as stereotaxis and the string galvanometer for measuring electrical potentials. Most notably, shortly after David Ferrier's student Robert Caton recorded human brain potentials for the first time (17), Victor Horsley electrically stimulated an encephalocele then, at the behest of Hughlings Jackson, performed the first electrocorticography for epilepsy surgery (18). In 1909, Harvey Cushing reported the use of cortical stimulation in two cases of focal epilepsy (19, 20), with Emil Theodor Krause, in the same year, publishing the first map of the human motor cortex (21). This was followed by a more extensive map by Ottfreid Foerster—a major influence on Penfield's work and his approach to epilepsy surgery. In fact, the literal translation of Foerster's ideas into English and North America was so verbatim that we still use the German alternating current line frequency of 50 Hz in mapping studies till the present day—the original stimulators being simple step-down alternating current transformers.
While cerebral localization had gotten off to a somewhat pseudoscientific start, complete with racialized tropes, the phrenology of Gall and Spurzheim gave way to scientific cortical localization (22), as described above with support from the developing field of neuroanatomy, as well as to anti-localizationalist works in the early twentieth century (e.g., Karl Lashley). In clinical spheres, localization became the hallmark and unique method of neurology, growing from the prescient conjecture of Willis to an eventual clinico-pathological method of Paul Broca and, most productively, Jean Marie Charcot who essentially described the modern screening neurological examination (23). While localization in epilepsy reached a new summit in the works of Hughlings Jackson, epilepsy in the English speaking world did not keep up with the sophistication of the French fusion of Charcot's anatomical and clinical thinking that was directly mapped to the brain and its networks by Jean Talairach, the psychiatrist who pioneered, along with his collaborators in the 1960s, a new stereotaxic method and means of determining anatomical correlations to a standard atlas in functional neurosurgery [e.g., (24)]. While a fruitful research stereotaxic apparatus was invented by the surgeon Victor Horlsey and Robert Henry Clarke, ushering in a new era of neuroscientific discoveries, it was not used in humans until the late 1940s by the founding epileptologist Frederic Gibbs at Harvard, along with Robert Hayne, and was not accompanied by an overall anatomical and localizationalist approach to epilepsy (25). Instead, Talairach, working closely with the neurophysiologist and neurologist Jean Bancaud, developed the means to combine astute localization of seizure semiology, individualized anatomy with functional correlations, and a stereotaxic approach to hypothesis testing, culminating in the 1950s and 1960s into the method that we now know as stereoelectroencephalography (26).
While amplifiers and recording methods, along with the SEEG method were developing, it is important to note that electrical stimulation actually preceded multichannel electrophysiologic recording. Electrodes were thus crafted with stimulation in mind and this was an integral part of both intra- and extra-operative use of both subdural and SEEG-based methods. While stimulation subserves several functions, as mentioned above, we focus in this review on the application of stimulation for electrical stimulation mapping of function (ESM) which can overlap with elicitation of the seizure aura, passive mapping, and connectivity mapping.
Historical Course of Cortical Stimulation Mapping
ESM involves the application of electrical current, typically to the cerebral cortex, in an effort to determine the potential contribution of a given region to a specific cortical function (e.g., sensory, motor, cognitive, linguistic, socio-emotional). While there are a few clear objectives, mapping of function is not always separate from stimulation to elicit after discharges and seizures. For example, stimulation during language testing may be found to reproduce ictal aphasia when a run of after-discharges or focal seizure is elicited in language networks. Similarly, mapping of function may help understand both which cortex is “eloquent,” but also elicit the seizure aura. Eloquence is a problematic concept and essentially equates to an observable function that is considered of high importance. However, under the right conditions and with appropriate testing, much, if not in theory all, of the cerebral cortex can be demonstrated to have a function–this is a major motivation for expanding the paradigms available for cognitive mapping. Nonetheless, eloquent cortex is conventionally considered to subserve key functions such as motor control and core components of language and speech. ESM has most frequently been employed intra- or extra-operatively in the setting of epilepsy and tumor surgery [(26–29)].
Drivers in North America
Wilder Penfield, after studying with Foerster, went on to employ direct electrical stimulation to explore the sensorimotor cortex, experiential phenomena and language functioning (30–32). Prior to that time, in clinical practice, neurosurgeons were reluctant to operate on the language dominant cerebral hemisphere for fear of creating a language disturbance (aphasia), with the exception of procedures involving the occipital lobe or anterior frontal lobe. Therefore, the introduction of ESM for language mapping opened new surgical opportunities for many patients who would have been considered at risk of harm from an open resection procedure. Penfield, along with his colleague Herbert Jasper, created what they termed the “Montreal procedure” during the 1930s, which involved testing the surgical patient while undergoing ESM in an awake state (Penfield and Jasper, 195) (33) (See Figure 1). Their pioneering work led to many discoveries and advancements in our knowledge of neural networks underlying language, and specifically demonstrating that such networks were much more complex and extensive than the classic language network postulated by Broca, Wernicke, Lichtheim, and others (34–37). They also showed that they could elicit both the auras of patients, which can be useful for localizing seizure networks, as well as experiential phenomena such as rich memories of events that included sensory phenomena (e.g., “I am in my grandma's house and I can smell the cookies baking”). With the advent of current regulated bipolar stimulation devices, as well as more adherence to safety limits, less electrical current is applied directly to the cortex, and rarely, if ever, are such contextually rich phenomena seen with cortical surface stimulation.
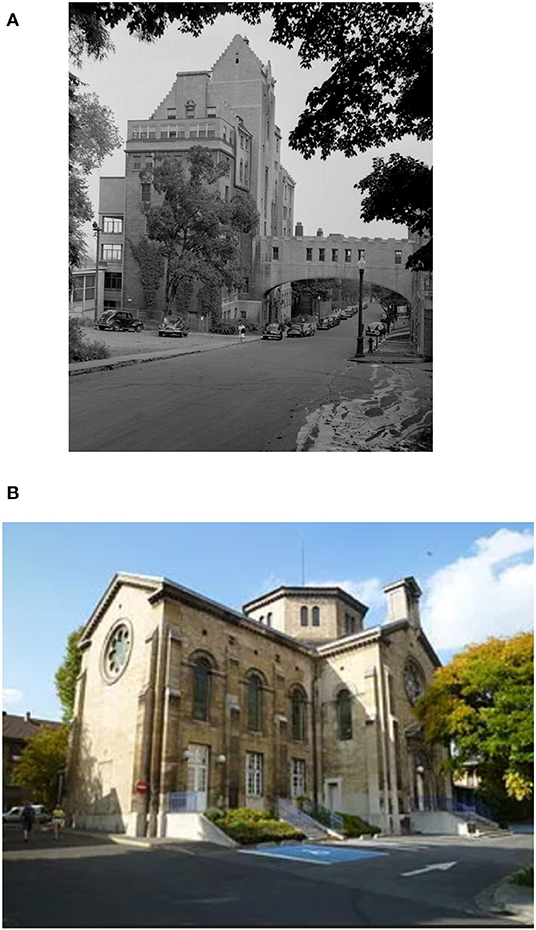
Figure 1. Historical sites central to the development of cognitive mapping: (A) the Montreal Neurological Institute, Montreal, Canada and (B) St. Anne Hospital, Paris, France.
George Ojemann, one of the founding members of the regional epilepsy center at the University of Washington (UW), built upon these ESM experiences, devoting much of his research career to the use of mapping paradigms to better understand cortical function. The UW program was the second regional epilepsy center in the US, following the University of Virginia, with programs funded by a project grant from the National Institutes of Health (NIH). His seminal work in 1989 (38), which included careful language maps of 117 presurgical temporal lobe epilepsy (TLE) patients, clearly demonstrated that critical language sites are much more broadly represented across the cortex than had been conceived. Investigators heavily relied upon visual (picture) naming approaches, which were likely tapping into the widely distributed networks required for one of the more complex actions carried out by humans (i.e., to name an object requires a large swathe of cortex from the primary visual area, unimodal and polymodal association cortices including lexical access, speech monitoring, and production) [see (34, 36, 39)]. There are actually multiple stages to this process, which can be carried out for some stimuli under 1 s, while more complex material may require slightly longer (e.g., 5–8 s for some complex stimuli). The Ojemann work, which included the projects of many trainees and collaborators, led to greater knowledge of reorganization of language and normal atypical variants (e.g., extent, spatial reorganization, contributing factors) and increased knowledge of primary vs. secondary languages and their neural distribution (e.g., primary languages appear to be more focally distributed than secondary languages) (40–45). This work also delved into the study of memory and led to some provocative findings which are being given more credence with the study of focal lesions resulting from our current epoch involving the introduction of minimally invasive surgical techniques. For example, ESM memory paradigms suggested that disruption of lateral cortex could disrupt episodic memory (46, 47), and it has recently been borne out that focal lesions in such subregions can cause lasting damage to memory even when medial TL structures are spared (48). The extent to which such functions could be localized to these lateral regions remains to be determined, and it may be that both medial and lateral aspects of the TL are simply contributing TL larger, interactive networks.
Investigators at the Cleveland Clinic have also made some important contributions to our knowledge of language networks using ESM. In particular, Lüders and colleagues discovered that application of electrical stimulation of the basal temporal lobe region (fusiform gyrus) of the dominant hemisphere at a high intensity produced a significant aphasia in a subset of patients involving disruption of both comprehension and speech output (49–51). This finding sometimes seems underappreciated by the neurological community, and this region is still not routinely assessed in many clinical investigations. Of note, lower intensity stimulation of this region only resulted in naming deficits. This area was dubbed the basal temporal language area.
While Lüders and colleagues initially reported no significant language deficits resulting from resection of this region (51), subsequent studies have indeed reported impairment (52–54). The original work of Penfield also indicated that resection of this region could result in significant confrontation naming problems (32). As electrical stimulation led to comprehension deficits as well as other language symptoms, this may be additional data suggesting a more posterior location of a region that is important for single word comprehension. One group has published a couple of case studies using subdural grid mapping of epilepsy patients which suggest that the posterior basal temporal language area may be most important for relating visual images to phonological content (55). Building upon these findings, a more recent electrophysiological study, which employed cortico-cortical evoked potentials to explore regional connectivity, revealed connectivity between the basal temporal language area and a posterior language area (56). It is also possible that this reflects disruption of the ventral auditory processing pathway, perhaps when including basal temporal white matter.
Benefits and Limitations of ESM
The most obvious benefit of ESM is its potential to spare eloquent functions, which typically include language and sensorimotor function, but really should be considered more broadly to include polymodal, cognitive and emotional processing components. Limitations of ESM primarily involve limits related to the coverage that is possible through grid mapping (29) and the sparse sampling of SEEG. In general, systematic coverage is provided for a limited range of the cerebral cortex. Another key limitation is that not only is the node being stimulated affected, but it seems likely, given conveyance of evoked potentials to other regions during stimulation, that what is observed clinically could be a network effect. Other limitations include a lack of cross-center standardization, and a lack of validated, standardized tasks to cover most cognitive abilities.
Historical Course of Mapping With SEEG
European Contributions
As described above, Jean Talairach designed a robust system which allowed patient-specific mapping of cerebral anatomy to a coordinate space that included functional correlations (4). This impressive registration before the computed tomography and magnetic resonance imaging eras used ventricular position and size derived from pneumoencephalography and angiography to delimit major vessels, particularly arteries, as well as the anterior and posterior commissures. At the same time, Jean Bancaud finished his higher doctoral thesis on the correlation between neuropsychological deficits and EEG in patients with brain tumors. He saw the value of using Talairach space in defining three-dimensional representations of seizures and their propagation, and a new method was born. With the advances in technology and regulations, it became possible to move from intraoperative “acute” to intraoperative prolonged and even ambulatory “chronic” SEEG recordings in France [see (57) for a brief history of epilepsy surgery in France].
While Penfield and Jasper primarily relied on interictal spikes and cortical stimulation for his resections, Talairach and Bancaud were able to obtain ictal recordings, sometimes elicited by pro-convulsant drugs such as pentylenetetrazol, and replicated as components or as a complete sequence to understand the spatiotemporal evolution of spontaneous seizures. While paroxysmal evoked responses were also obtained by both single shock and train stimulation (4, 58, 59), this approach, in contrast to that of Penfield and Jasper, relied on the careful analysis of seizures, rather than resection based on interictal abnormalities.
There were several differences between North American and European approaches to stimulation and mapping. While North American approaches typically demarcated functional mapping from analysis of the patient's epileptic network, this was often not the case in Europe. For example, examination might occur when eliciting the seizure or components thereof, as described above. While 50 Hz stimulation was prominent in North America, the SEEG approach tended to emphasize the importance of tailoring stimulation frequency, amplitude, and train duration based on the location stimulated [e.g., (59)]. From a practical standpoint, low frequency stimulation is used for functional mapping of primary areas, for the study of functional connectivity, and of areas with a lower threshold for after-discharge and seizure (e.g., amygdala and hippocampus); high frequency stimulation, such as 50 Hz, is used for functional mapping of non-primary neocortical areas, and for triggering seizures. SEEG can be utilized to investigate and identify language networks in patients with epilepsy (60). In North America, there was a concern for “clearing” cortex that may be “eloquent” for resection, while the SEEG method tended to precede from the patient's semiology and examine function in relation to seizures. Later academic work in both arenas focused more formally on examining specific cortical functions—work that is far from exhausted today.
While SEEG can be used, unlike cortical surface mapping, to map the functions and connections of fiber bundles [e.g., (61)], much of the work of electrical mapping of the language functions of white matter has been performed intraoperatively, particularly by Duffau and colleagues. This has been achieved by mapping during tumor surgery, involving stimulation of the cortical surface and white matter tracts as they move through the surgical zone in the setting of tumor resection (62, 63). They have combined this approach in an interesting fashion with the use of neuroimaging techniques (e.g., fMRI, DTI) and neuro-dissection techniques in a multimodal manner that has contributed to more localized analyses of function and the inclusion of pathways/connections to the sometimes exclusive focus of past researchers on gray matter/cortex only (64–67). Of note, however, one criticism has been that some of this work has been based on a more crude localization using photographs of the area being stimulated, which are then matched to the available preoperative MRI scans. Therefore, there is likely much more work that can be accomplished in this area as well. These investigators have demonstrated that naming dysfunction differs by the white matter pathway that is stimulated [e.g., semantic errors tend to occur during picture naming when stimulating the inferior frontal occipital fasciculus (IFOF) while phonemic paraphasia results from stimulation of the arcuate fasciculus (67)], and that disturbance of recognition of faces and objects can occur with stimulation of the non-dominant ventral visual processing stream (68, 69). They have also published data showing that seemingly small declines in language processes (including simple speech response speed on naming) can lead to significant decline in functional status (e.g., failure to return to work relates to slowed response rate on these tasks) (70).
Benefits and Limitations of Mapping With SEEG
While the SEEG method itself affords more access to anatomically distant but functionally related areas, with superior access to sulci and deeper cortical and subcortical structures than ESM, the trade-off involves a reliance on a sparser sampling of the cortical regions of interest. A related benefit is that when a patient is implanted thoughtfully, large functional networks can be probed, rather than adjacent fragments of multiple networks as is typically the case with a subdural approach. While use of a 3D-grid approach has been described to overcome sparse sampling (71), this is largely at odds with the overall localizationalist and network thinking of the SEEG method and is generally not recommended—the large number of electrodes in one region, at the expense of covering other hypotheses or parts of the network, may increase hemorrhage risk, although electrode density as a specific risk for hemorrhage has not been studied. Furthermore, skull thickness can be a limiting factor in sampling certain areas (such as anterior temporal region). This is a major consideration in younger children. Finally, SEEG is less invasive than ESM using grids and strips, and this fits very well with the continued trend toward employing minimally invasive surgical procedures.
There are several limitations to mapping with SEEG. A major present obstacle is the learning curve in practicing the SEEG method. There is an uneven level of experience in SEEG mapping within centers which have used SEEG for decades and have well-established methods in place when compared to centers that adopted this method recently. The publication of the French guidelines on SEEG in 2018 is an effort to standardize the practice across centers and offer recommendations for those who are implementing the method (72). The interpretation of positive or negative responses during stimulation can be challenging from a functional standpoint. One of the key concepts that can impact functional mapping in SEEG is charge density given the relatively small surface area stimulated. Theoretically, the stimulation effect is local, however, a distant effect of stimulation is also likely and should be considered when interpreting stimulation results, particularly given that intercontact spacing typically means that white matter is also stimulated [human cortical thickness varies from about 1–4.5 mm, e.g., (73), and 2 mm contacts are typically not <1.5 mm apart, often more] (74, 75). Certain charge densities and frequencies may have an inhibitory effect on certain cortical areas, or may result in inhibition of downstream targets (e.g., with stimulation of the prefrontal cortex), positive clinical phenomenon, or inactivation of a network that extends beyond the area stimulated (e.g., some aphasic effects in language mapping). For all of these reasons, mapping a complex function such as language requires experience and analysis with subdural electrodes and perhaps still more with SEEG, whereas mapping primary motor or sensory function is usually straightforward despite a lower spatial resolution. Therefore, mapping must be used thoughtfully and is best tailored to individual patients and functions. Probing the presence and the effect of a focus in a large interconnected network can be accomplished with SEEG.
Current Status of Cognitive and Emotional Mapping with SEEG
Stimulation Parameters
The parameter space for stimulation can be large, but fundamental neurophysiology significantly reduces this multivariate parameter space. The variables of stimulation are intensity, montage (e.g., bipolar vs. monopolar), duration, waveform, charge balancing, frequency, and train characteristics. While not considered in this review, issues of geometry, contact size and shape, and material are also important considerations. This parameter space is made more tractable by the safety limit in charge per phase with SEEG electrodes that limits amplitude and pulse duration (with 30 μC/cm2/phase based on animal data, electrode metal characteristics and pathological examining of human post-mortem tissue after chronic basal ganglia stimulation) (76). Waveforms have been explored to a greater extent in basal ganglia stimulation and typically a symmetrical charge balanced square wave is used in SEEG, despite other waveforms possibly providing more targeted stimulation at least in microstimulation (77)—this is yet to be studied in detail. Montage is determined by the volume of tissue that one desires to activate and there is a significant body of work pertaining to stimulation frequency for the cerebral cortex in mapping, as mentioned in a prior section [e.g., (26, 78)]. Subdural grid stimulation parameters are similar, except that a higher safety limit is typically accepted (~50 μC/cm2/phase), perhaps given short-circuiting over the pia and through cerebrospinal fluid, based on elemental pathologic analysis of resected temporal cortical tissue after acute stimulation and subsequent temporal lobectomy (79). While these factors all serve to make the stimulation parameter space quite tractable, this should not be confused with therapeutic stimulation parameters, or higher frequency stimulation that may be inhibitory—the parameter space is under-determined, but has gradually become better explored (75).
Risks of Depth Electrode Placement
While SEEG is substantially less morbid and better tolerated than craniotomy with subdural grid placement, injury must be considered. Broadly, it is worth considering three categories of injury: Major (e.g., neurologic deficit that does not entirely resolve), minor (resolving complications), and subtle. We define the latter here as long term effects of electrode insertion in the absence of any complication. Regarding major and minor complications, the most common is hemorrhage. The largest study of complications of SEEG placement reveals a per-patient risk of hemorrhage of about 19%, and ~0.2% risk per electrode for hemorrhage, being symptomatic at a rate of about 0.05% per electrode. In this study it is noted that there is a measurable increase in hemorrhage, on a per patient basis, when the number of electrodes exceeds about 13 (80). Similar rates of symptomatic hemorrhage per electrode (0.04%) were found with a larger SEEG case series focused on broader SEEG practice (81). Overall, this is favorable to the potential harm of subdural grid placement which has a higher incidence of infection, subdural hemorrhage and cerebral edema (82).
Subtle injuries are less clear, given that studies have not yet been designed to prospectively identify these effects. Two fairly recent studies raised questions about the possibility that cognitive function, particularly memory, might be adversely affected by bilateral depth electrodes placed orthogonally through the hippocampi (83, 84). Nevertheless, both studies were retrospective in nature and limited by methodological imprecisions that could not be overcome after the fact (e.g., group differences that could not be controlled for in a retrospective clinical study). In contrast, similar studies have not been suggestive of this decline, and this has included work completed using neurostimulation devices that have been placed longitudinally along the hippocampus in both occipital and temporal lobes. Evaluations of this area have been the subject of several recent commentaries, which go into a great deal of detail, and suggest the need for prospective studies to overcome these limitations (85–87).
Promise of Cognitive Mapping With SEEG
Passive vs. Active Stimulation Mapping
Historically, as we have covered thus far in this manuscript, cognitive mapping for epilepsy presurgical candidates has involved administration of a cognitive paradigm while high frequency electrical stimulation is actively delivered. One drawback to this approach is that it requires the presence of an epileptologist to perform the stimulation and monitor the live recording for after-discharges and seizures, as well as a neuropsychologist or cognitively oriented neurologist. While 50 Hz stimulation is often appropriate for cognitive mapping, outside of the sensorimotor cortex and hippocampal formation, with both a broader range of brain structures accessed and with a greater sophistication in planning stimulation, other frequency, and train parameters can be, and perhaps ought to be chosen. With higher frequency stimulation, seizures are more likely, which abort the attempt to study cognition, or contributes to after discharges which can distort assessment results by disrupting broaders network regions in more formal cognitive assessment. This cannot be entirely avoided with altered stimulation parameters, but the occurrence of focal seizures can be of some assistance in understanding the function of components of the seizure network. Nonetheless, several studies demonstrate the problem of seizures disrupting cognitive mapping. For example, in a study of 122 pediatric patients who received 50 Hz electrical stimulation mapping, sizeable percentages of patients experienced after-discharges (77%) and seizures (35%) (88). In another sample of 57 adults undergoing active mapping, seizures occurred at a similar rate (33%); in a subset of this sample who underwent language assessment, 17% experienced seizures that disrupted mapping attempts (89).
In more recent years, passive mapping of cognition has been explored (See Figure 2). This has been an important advance given that it is theoretically more localizing than stimulation, which can activate a network while exerting local influence, theoretically also providing cognitive evoked potentials or latency information. Conversely, brain regions related to other components of the task may be activated, arguing for a lower spatial specificity in passive mapping. Keeping both of these ideas in mind, it seems reasonable to consider this approach to mapping as complimentary, and potentially capable of acting as a second independent source of information when considering the functional organization of the cerebral cortex. From a practical standpoint, passive mapping is performed by conducting behavioral paradigms during concurrent electrocorticographic recording. In general, electrocorticographic analysis of broadband higher gamma frequencies (70–110 Hz) is typically carried out during administration of a specific cognitive or sensory task (e.g., confrontation naming, verbal fluency) (91–94). Recording of high gamma activity is best done with a sampling rate of ECOG data that is 2- to 3-times higher than the frequency of recorded activity to avoid aliasing of waveforms and a high frequency filter above 300 samples per second (S/s), fitting with most centers recording at 1 kS/s or more. After ECOG data is obtained, time-frequency analysis is performed using the bipolar montage when SEEG electrodes are used (95, 96). Bipolar montage analysis excludes common mode signals, such as muscle activity, whose high-frequency components can mimic high-gamma oscillations (97). Time-frequency analysis of event-related, high-gamma activity helps delineate the network involved in a given cognitive task (picture naming). For instance, high-gamma oscillations recorded at the onset of an auditory naming task is indicative of perceptual processing, while high-gamma activity at the end of the response represents motor processing.
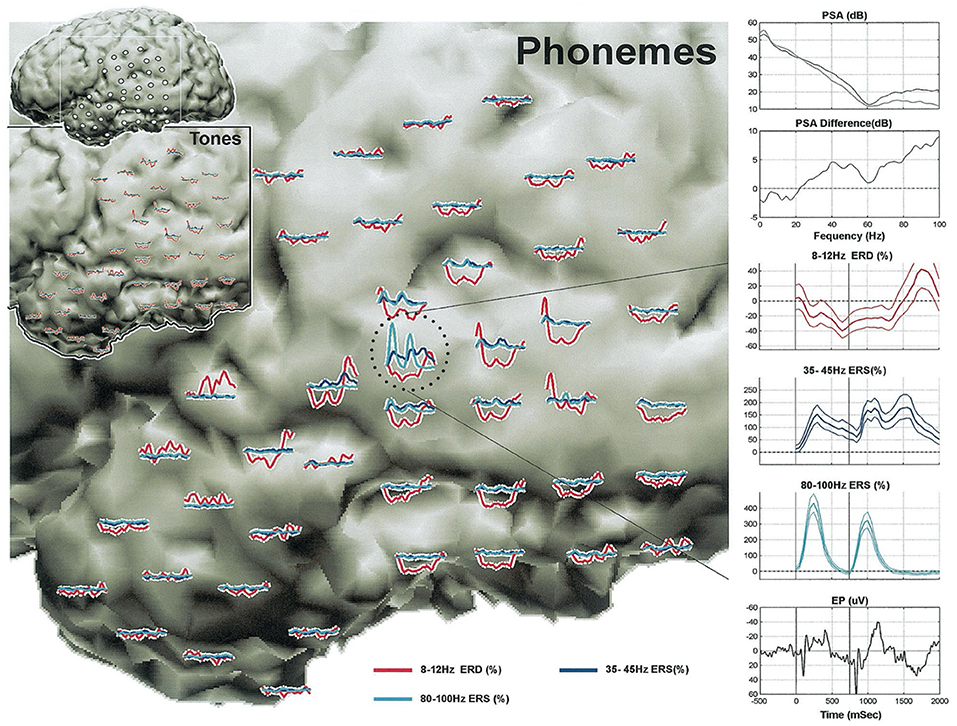
Figure 2. Cortical activity during a receptive language task. From the first study of passive mapping of electrocorticographic activity during a receptive task of distinguishing tones from phonemes by Crone et al. (90). Indices during perception of tones (lower left inset with black border) vs. phonemes (expanded view of left temporal lobe). Plots of event-related power augmentation/suppression are color-coded according to frequency, and correspond to the electrode locations depicted in the upper left corner inset (white frame indicates borders of the expanded views). Detailed plots in the right column are derived from an electrode over the left superior temporal gyrus (circled). PSA, power spectral array; ESD, event related desynchronization; ERS, event related synchronization; EP, evoked potential. From Crone et al. (90).
Studies contrasting active and passive paradigms for language (particularly expressive) have shown reasonably equivalent results at least with respect to critical regions of common overlap [sensitivity (98, 99)]. Indeed, changes in gamma activity during passive language mapping do appear to overlap with language areas identified through electrical stimulation mapping (91, 99–102). In contrast, however, passive mapping paradigms find more regions related to task performance in general, as above, so these techniques do differ with regards to specificity, and this can lead to problems with determining core regions essential to a cognitive task. Although limited in scope, passive mapping appears efficacious when comparing mapping findings to postsurgical results; here, resection of regions that were associated with event-related gamma oscillations in passive mapping was associated with post-operative language deficits (103, 104). Much more work is required in this area, as larger studies are required that cover all behavioral paradigms of interest. Outcome validity studies are also woefully lacking for traditional active mapping paradigms as well.
Passive mapping may have some practical and accuracy advantages over active stimulation. First, passive mapping is more time efficient which lends itself well to children or adults who have difficulty completing more lengthy sessions of active cortical stimulation mapping or who cannot tolerate unpleasant sensations that can sometimes accompany stimulation mapping. It also avoids artificial perturbation of the brain, and thus reduces overall risk for after-discharges and seizures. Passive mapping is suggested to be more sensitive to localized cortical areas (e.g., as defined by language mapping: Broca's, Wernicke's, sensorimotor, basal temporal) than electrical stimulation mapping (102). In contrast, one potential limitation with passive mapping compared to active stimulation mapping, is that passive mapping, like fMRI activations, cannot determine whether regions are essential for a given cognitive task rather than simply being involved in the task (60, 105). If a region is not essential, it can still typically be included in a proposed surgical resection/ablation without harming overall function. Despite the limitations of stimulation mapping, and the potential to disrupt function more broadly than recognized or desired, it does create a “functional lesion” that may more accurately reflect the possible effect of surgical intervention. An interesting research proposition would be to use passive mapping to locate potential regions of eloquent brain tissue, and to follow this up with stimulation mapping of these areas in an attempt to better determine the potential effects of surgery.
Use of Machine Learning to Decode Passively Acquired Electrocorticography
While brain-machine interfaces are generally beyond the scope of this review, there is topical overlap with passive cognitive mapping and research directed toward the decoding of speech. Given the likely problems of under-determining activity in a single brain region from sparse sampling with SEEG, this work has relied on grids, including higher density research grids. While this represents a large body of work that shares some similarities with work on brain-machine interfaces, the most notable current achievement is the transcription of speech while recording from language-dominant frontal opercular region (106), that performs quite well with simple sentences (See Figure 3). This decoding of neural activity in relation to behavior is particularly suited to machine and deep learning, where a classifier can be built or trained based on neural activity and an objective measure of behavior. These approaches will provide practical and theoretical insights into the organization of cortical function, as well as potentially providing a wide-array of brain-machine interfaces.
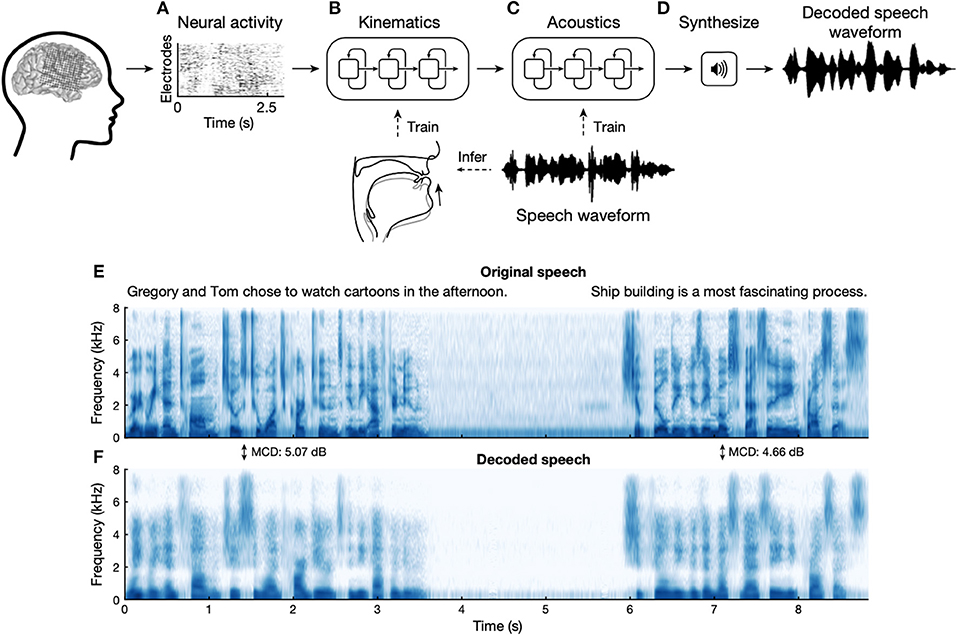
Figure 3. Speech synthesis from recorded electrocorticogram during spoken sentences. (A) The neural decoding process begins by extracting relevant signal features from high-density cortical activity. (B) A neural network decodes kinematic representations of articulation from ECoG signals. (C) An additional algorithm decodes acoustics from the previously decoded kinematics. Acoustics are spectral features extracted from the speech waveform. (D) Decoded signals are then synthesized into an acoustic waveform. (E) Spectrogram shows the frequency content of two sentences spoken by a participant. (F) Spectrogram of synthesized speech from brain signals recorded simultaneously with the speech in (E). From Anumanchipalli et al. (106).
Connectivity, Cognition, and Philosophy
While some notions of localization focus on a one to one mapping of function and brain tissue, it is obvious that functional territories cannot act in isolation. A less extreme form of localizationist thinking might hold that cortical tissue maps well to function, with it taken as implicit that subcortical structures are necessary for input and output. When considering behavior, obviously large networks, typically with multiple cortical waypoints are involved. When considering the abstractions of cognitive psychology, there can be mapping of abstract functions to some brain regions, but the agreement of neurological thinking and the functionalist concepts of cognitive psychology are imperfect (take episodic and semantic memory for examples, where these concepts do not map directly to a brain region or perhaps even a circuit). Overall, this mismatch results in a philosophical approach that grew out of medical materialism, by way of Sellars (107) and Feyerarbend (108), that has come to be known as eliminative materialism (109) where folk psychological abstractions are eliminated in favor of an ontology based around neural mechanisms [see (110), for discussion]. This is critical to mention for three reasons: Firstly, we should keep this in mind when developing new tasks that aim to test particular brain networks. Secondly, this is likely a fruitful general line of research in neuroscience, behavior, and cognitive psychology. Lastly, it marries with a vastly increased interest in neural mechanisms in philosophy and particularly in the philosophy of mind. This latter scenario is beginning to provide productive common ground for more sophisticated understandings of consciousness, cognition and brain function.
Connectivity: DTI, fMRI, CCEPs, and Limitations
As we have thus far argued, an appreciation of connectivity is critical for understanding the correspondence between cortical functions and anatomical networks. There are several ways to determine and define connectivity. It should firstly be emphasized that the gold-standard of brain connectivity remains neuroanatomical tracing. These methods are based around the transport of stereotactically injected dyes, radiolabels or detectable proteins into the brain so that neurons projecting into the area of injection, or axons projecting from this region, can be visualized, often by autoradiography or histological methods and microscopy. Neurotropic viruses such as strains of herpes and rabies have also been used for tracing, which can be polysynaptic. More recently, viral vectors have been the principle means of determining afferent and efferent connections of a given brain region, and by using genetic methods, even of a particular cell type [see (111, 112)]. Needless to say, these methods cannot be performed in humans, meaning that our most detailed knowledge of veridical connectivity comes from non-human primates and inferences from other species. This leaves us with inference and indirect methods to determine connectivity and cortical networks essential for cognition in humans, one of which is based on and related to electrical stimulation mapping.
From the human neuroimaging literature, the indirect methods of determining human “connectivity” is often divided into structural, functional, and effective connectivity (113). Structural connectivity, not to be confused with neuroanatomical tracing, typically refers to diffusion imaging-based methods such as probabilistic and deterministic tractography. There are numerous limitations to this method, when sharp axonal branches or curves cannot be followed, the direction is not determined, nor the length of a given set of axons be determined as identical to that of the larger bundle of fibers that can be detected [see (114)]. Functional connectivity need not represent anatomical connectivity at all: This is where a functional assay is used, and correlated activity is determined by blood oxygen level desaturation (as in functional MRI), or perhaps by intracranial EEG. The problem is that simultaneous activation can result from two structures having a shared input. For example, activation of subcortical nuclei can result in faster activity across the cortex, but it does meaningfully represent “connectivity” between these cortical regions. Effective connectivity is where a connection is implied if a recording site's activity is changed by perturbation of another site. This provides causal information, but typically cannot determine if connectivity is direct or indirect. An example, pertinent to the present review of functional networks is cortico-cortical evoked potentials [CCEPs (115)], which are perhaps the closest we can get to a measure of anatomical connectivity in the human cortex (See Figure 4). While this technique was first described by Brazier (117), by which time it may have already been appreciated by Bancaud and Talairach, it has undergone something of a recent revival. This technique is a boon for studies of human forebrain connectivity, especially as we attempt to divide the cerebral cortex into discrete functional networks. For example, this approach to using low-frequency stimulation, longer than the time of the complete evoked potential and typically 1 Hz, and its resultant CCEPs, has been used to examine connectivity between language areas (118, 119), within the motor cortices (120). This has been of assistance in mapping and in sparing white matter intraoperatively, to preserve connectivity between the anterior and posterior language areas. For example, in one patient a 50% drop in CCEPs amplitude was associated with a long-term language deficit (61).
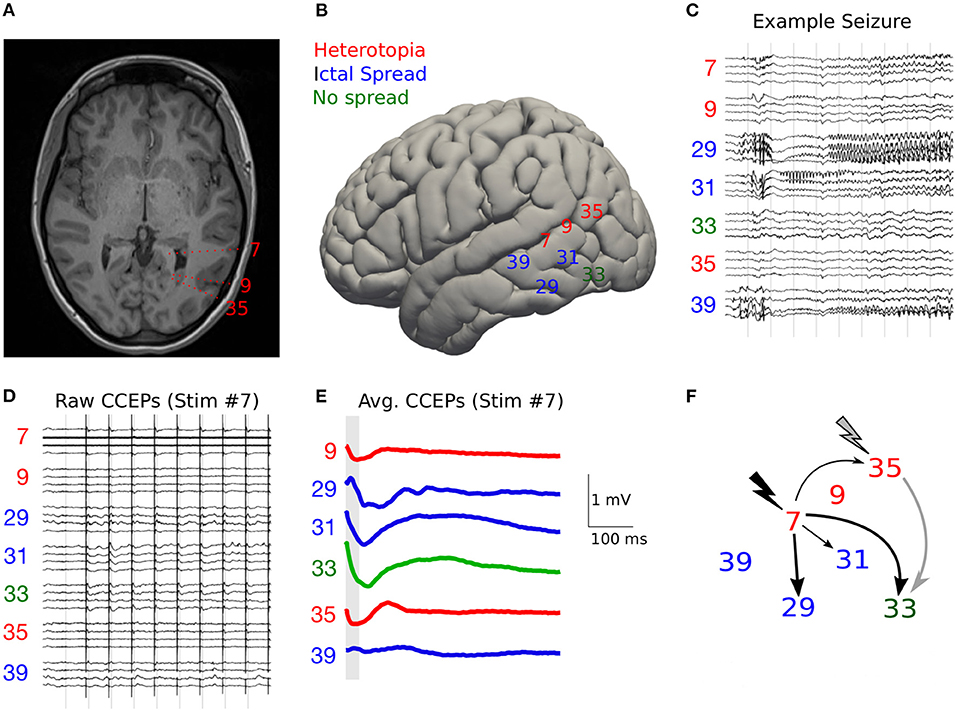
Figure 4. Example of using CCEPs to study effective connectivity. (A) Axial MRI Brian (T1) showing two periventricular nodular heterotopias in the tirgone of the left lateral ventricle and the trajectories of electrodes 7, 9, and 35, with dashes showing the approximate location of the 10 contacts of each recording electrode. (B) The approximate lateral entry points of pertinent left-sided SEEG electrodes are shown as electrode numbers. (C) An example spontaneous left sided seizure onset is shown with gamma activity on electrode 29 contacts 1–3 (posterior hippocampus). (D) Raw cortico-cortical evoked potentials (CCEPS) triggered by 1 Hz bipolar stimulation anterior heterotopion (electrode 7 contacts 3–4). Evoked potentials with peak to trough amplitude >250 μV are evident on electrodes 29, 31, and 33. (E) Averaged CCEPs with 20–50 ms (gray bar) window of interest shown. Only the largest amplitude (root mean squared amplitude (RMSA) CCEP (taken over all 10 contacts) is shown for each electrode. (F) Connectivity map for stimulation to electrode 7 (black) and 35 (gray). Thick arrows represent CCEPs with RMSA >200 mV, thin arrows represent CCEPs with RMSA >100 mV. From Dickey et al. (116).
Functional Mapping of Cognition and Emotion—Anticipating Future Directions
Cognitive Function
While much progress has been made in the mapping of cognitive function, there remains considerable variability in the techniques and paradigms used (29), there are many aspects of cognition that never get assessed, and the determinations of the full networks responsible for a function is often not determined. The focus of cognitive mapping has primarily been language, and to a lesser extent, aspects of memory. This is rightfully so, as the early days of epilepsy surgery were marred by poor outcomes of cases such as Henry Molaison (121), where the severity of the resulting amnestic state overshadowed any other aspect of the case. As we have gotten better at avoiding these catastrophic outcomes, and even lessening the poor cognitive outcomes through improved testing and minimally invasive surgical options, the focus of the field can now broaden to potential deficits which were previously underappreciated and potentially overshadowed. Initial findings suggest that some of these overlooked deficits can have some profound effects on patients (70, 122). Most cognitive mapping efforts have focused on language, in particular visual object naming. Even in this well-hewn area there is little consensus on training approaches as well as best practices for clinical and research efforts. Table 1 lists a number of cognitive and socio-emotional functions for which there is some evidence of structure-function knowledge derived primarily from cognitive mapping procedures.
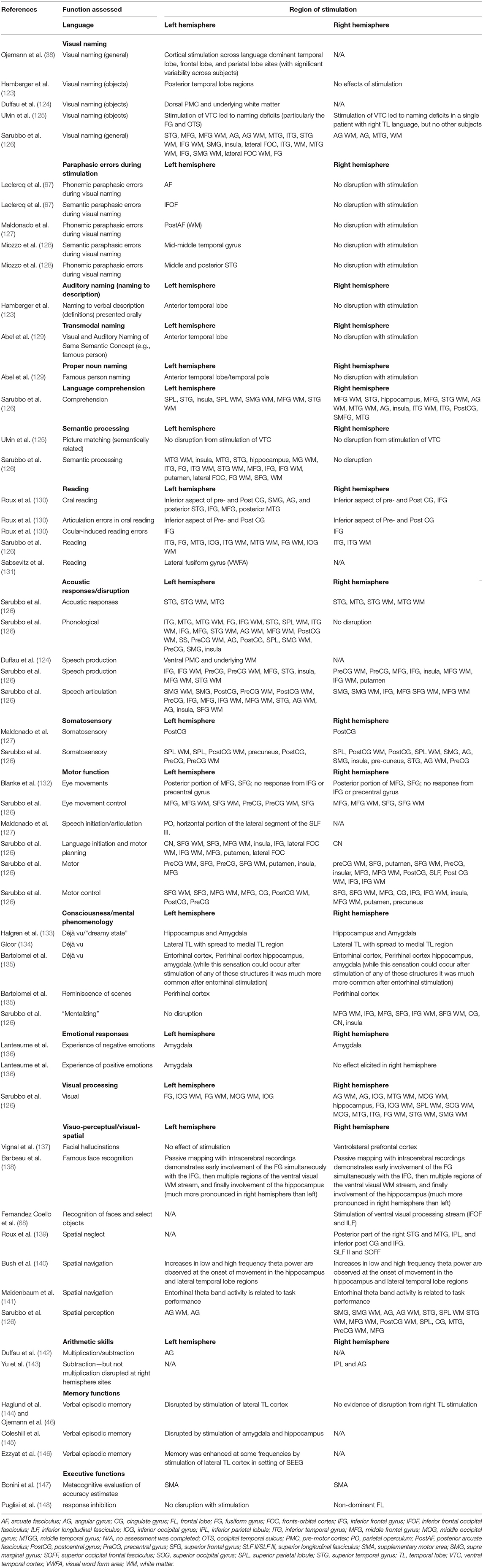
Table 1. Chart of positive neural stimulation sites and specific neuropsychological functions (selected sample of representative studies).
Language
Language mapping often involves administration of relatively simple tasks to test basic, automatic speech functions, such as counting or reciting overlearned phrases. Object naming is considered the “gold standard” for mapping language and allows for a broad sampling of the language network; however, more targeted, multitask testing may be needed to increase sensitivity as naming alone has been shown to miss 31% of temporoparietal and 43% of frontal language sites (149). It is widely accepted that language involves a more distributed network than Broca's and Wernicke's area and that anatomically dissociable regions exist that are specialized for specific linguistic subroutines which interactively support the construct of language (34, 39, 150, 151). Regions spanning the ventral temporal and occipital lobes and fusiform area appear to contribute heavily to recognition (primarily right hemisphere, but some left) of visual objects and faces, while coexisting areas on the left are important for naming (152–156). Naming itself is a complex construct, which can differ by modality of stimulus presentation [e.g., naming sounds vs. naming pictures (157)], object type [i.e., different object types map to different brain region (158, 159)], and level of classification [e.g., proper nouns have been more associated with the temporal pole while common nouns seem more broadly distributed (160)]. Naming can also vary by task parameters such as having a patient name objects/persons based on verbal descriptions (e.g., “the current President of the US”) rather than based on a sensory representation [e.g., naming a washing machine based on the sound it makes or its visual image (161)]. The latter tasks require the subject to determine the semantic content before applying the name, and are therefore slightly more complex. Orthographic or written letter content seems to be managed by a posterior temporal component of this stream [e.g., visual word form area (131, 162, 163)]. Aspects of the mid superior temporal gyrus and sulcus are dedicated to processing phonology or speech sounds, consistent with adjacency to unimodal auditory association cortex, while more posterior areas of the superior temporal gyrus and inferior parietal lobule involved in phonological access and retrieval, and areas in lateral middle and inferior temporal lobe, posterior inferior parietal lobe (angular gyrus) and dorsolateral frontal cortex that are involved in processing semantics [i.e., the meaning of pictures, words, phrases, etc. (29, 150, 164, 165)]. And this is of course all contingent on patient-specific factors like intelligence (44), gender (166), and handedness (167, 168) to name but a few.
Given the complexity and multidimensional nature of language, there is a need to develop and use tasks that differentially engage or drive these linguistic processes so mapping can be better tailored to the functional anatomy of the area being mapped (See Figure 5). For example, (171) have put forward the idea that language networks consist of functionally specialized “cores” and domain general “periphery.” Interactions between language nodes and with other brain networks are thought to subserve different language functions which may differ depending on task parameters. For example, the word “nail” has different meanings depending on the context of use, and will likely engage different subnetworks when presented as body part, an object, or as an action. This conceptualization of language is consistent with fMRI findings, such as those of Tyler et al. (172), which have shown that the same stimulus can activate different brain regions depending on the context of the task (e.g., different regions are activated when presenting a given object and asking the patient to think of the type of object, the general class of object, or the specific name of the object). It could therefore be useful to design tasks with a single set of stimuli that could be used to potentially activate different brain regions depending upon the broader task demands. On the other hand, cognitive psychological approaches to language are often function- and theory-based. Both cognitive theory and underlying brain mechanisms and understanding of the functional organization of the cerebral cortex will be key, related to the idea of eliminative materialism, above. In other words, preceding from neurologically plausible concepts of the organization of language is also critical.
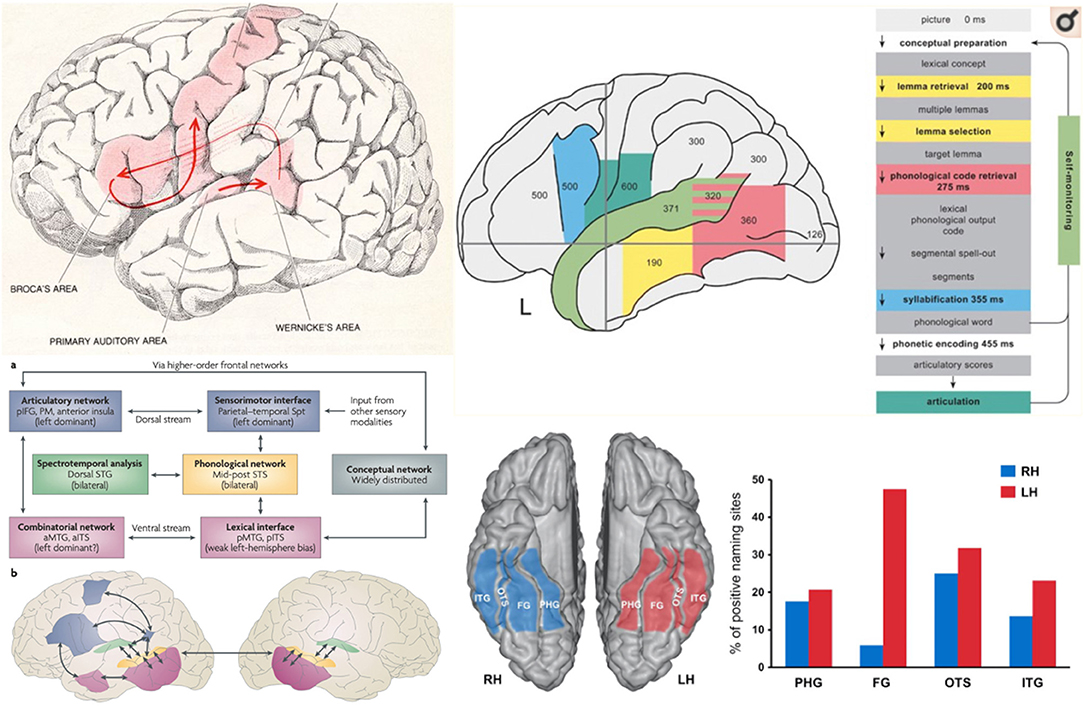
Figure 5. Models and Anatomy of Language Networks reveal the large area of the cerebral cortical involved in language. Top left: Geschwind's (169) illustration of the Broca-Wernicke model of language. Top right: Indefrey's (170) model of cortical activity (showing evoked potential latencies by region in milliseconds) during a confrontation naming task, demonstrating some of the cortical extent of language processing. Bottom left: Hickok and Poeppel (39) dual stream model of the cortical anatomy of auditory language comprehension and response where auditory processing is bilateral and involves bilateral superior temporal sulci and unimodal auditory cortex from which activity is conveyed to either a dorsal stream for, motor and articulatory analysis and phonological representation, vs. a ventral stream for lexical and conceptual representation (aITS, anterior inferior temporal sulcus; aMTG, anterior middle temporal gyrus; pIFG, posterior inferior frontal gyrus; PM, premotor cortex). Bottom right: From Ulvin et al. (125), showing the regions in which stimulation can result in specific naming deficits (“positive naming sites”), identifying the crucial role of the dominant fusiform basal temporal region in naming (ITG, inferior temporal gyrus; FG, fusiform gyrus; OTS, occipitotemporal sulcus; PHG, parahippocampal gyrus).
Beyond Language
To date, much less work has been done on mapping non-language functions—and the notion of non-eloquence in brain areas outside of dominant hemisphere language regions needs to be challenged. The right hemisphere is known to play an important role in visual perception and spatial processing (173, 174), object/face recognition (155, 158, 175–178), socio-emotional processing (7), navigation and learning in a spatial context (179), and attention/neglect (180), and there is great need to develop tasks to assess these functions for the purposes of stimulation mapping.
Visual-Spatial Processing, Construction, Navigation
Some work exists in the area of spatial processing and navigation with regards to ESM/SEEG, but most represent “one-off” case studies and an occasional targeted experiment rather than a planned effort to study these functions with these technologies. However, deficits in many of these functions can lead to varying degrees of disability for the patient. One example is unilateral spatial neglect, which involves an inability to attend to one side of space (most often the left side with right sided lesions). Line bisection and cancellation tasks are commonly used to assess spatial neglect and discrimination behaviorally, and this function has in turn been mapped to the posterior parietal cortex—more specifically the inferior and superior parietal lobules but also portions of the posterior temporal lobe (139). Mental rotation tasks, where the patient has to determine whether two objects are the same or different by mentally rotating them, can also be used to test the non-dominant parietal lobe (181, 182). Functional MRI studies have implicated bilateral superior parietal, frontal, and inferotemporal cortices during mental rotation with greater non-dominant, right parietal activation often seen (183). Facial perception and line orientation have likewise been tied to the non-dominant parietal lobe (specifically the parietal-occipital junction) as well as the non-dominant posteroinferior frontal lobe (184). Impairment on these tasks tend to contribute to social dysfunction and can impair work performance as well, although this is an area that has never been well-studied.
Navigation has also been studied in humans, but the tasks, physiology and circuits have some dissimilarities with the large knowledge from rodent studies. Firstly, studies of neocortical theta are likely irrelevant to the navigation-related activity that is extensively characterized in rodents. Attention has focused on lower frequency components of the intracranial medial temporal lobe EEG, but while non-hippocampal theta has been reported, in relation to virtual maze tasks up to 8 Hz (185), including in relation to possible grid cells of the entorhinal area (141, 186), hippocampal task-related candidate theta activity has been described at 1–4 Hz and around 8 Hz (140, 187–190) also see (191). Furthermore, periods of enriched theta are very brief (188) and are not coordinated along the human septo-hippocampal axis (192). Given the large number of diverse cortical inputs to the hippocampal formation inferred in humans, spatial tasks may only influence as-yet unidentified subregions of the hippocampus. Similarly, rodent work has called attention to the hippocampal formation, given a role in learning of spatial tasks, but spatial learning and perception obviously involves multiple regions of both neo- and allocortex, again ripe for dissection with task development.
Executive Control Processes
Monitoring of executive functions is particularly important during frontal resections, but it is important to note that executive functions involve more distributed cortico-cortico and cortico-subcortical networks, and deficits in this domain can develop with damage outside the frontal lobes (193). Executive functions include processes such as planning, shifting from one mental set to another, updating and monitoring of information, problem solving, metacognition, abstract reasoning, and inhibitory control (194). While little work has been done in this area when it comes to cognitive mapping, tasks to consider could include inhibitory control (e.g., Stroop/color-word interference tests, Go/No Go tests), working memory (e.g., reverse digit sequencing), verbal generative fluency (e.g., saying as many words starting with a particular letter within a short period of time) and mental flexibility (e.g., Oral Trail Making Test requiring alternating recitation of ascending numbers and letters). As an example, researchers used SEEG to demonstrate that the supplementary motor area has a role in evaluating the accuracy of actions (147). Similarly, Puglisi et al. (148) used a simplified Stroop paradigm in a ESM setting, and reported that sparing the identified subcortical sites in the non-dominant frontal lobe region led to preserved executive functions as compared to patients who did not previously receive this mapping.
Learning and Memory
Clinical memory evaluation using ESM and SEEG paradigms has also been minimally explored and is rarely used in clinical practice, although changes in memory comprise some of the worst potential deficits of epilepsy surgical procedures. Estimates of memory decline, which are limited by greater variations in test usage, nevertheless range from 40 to 60% in temporal lobe cases (195–198). These numbers are more modest with minimally invasive surgical procedures but still occur (48, 199). Moreover, we have argued that the current clinical tests of memory are woefully inadequate to fully capture the complexity of memory. Almost all clinically available tasks require the patient to learn solely auditory/verbal or visual content, which they must learn and recall for a very brief period of time. In actuality, human memory is a much more complex construct in which such recall has to be integrated into existing memories (semantic and autobiographical knowledge) and is typically learned in a multimodal fashion (i.e., requires the integration of multisensory and motor input, linguistic/semantic interpretation, etc.) with coding of temporal, spatial, and emotional context. It is possible that we are only testing the most basic of memory subsystems in the process of learning and consolidating information required for effective adaptation to life. Therefore, we need to develop much more advanced measures and test paradigms to allow us to explore these broader aspects of memory. Some of these paradigms can be as simple as combining modalities of learning (e.g., putting a name with a face) and other aspects have only become possible with the advent of technological advances (e.g., virtual reality). Ideally, an array of tasks varying in complexity with dissociable sub-components will allow for the “mental chronometric” process of determining the neural substrates of the basic components (e.g., networks underlying simple encoding of different stimulus modalities) and the broader systems level interactions (e.g., integrating encoded percepts across stimulus modalities, integrating these new memories with existing semantic and autobiographical knowledge bases, processing modulatory feedback from emotional and linguistic systems), which obviously form multiple “scaleable” levels of integrated complexity.
The use of memory paradigms combined with properly designed electrophysiological study (e.g., CCEPs, single unit recordings) could powerfully increase our knowledge of this critical brain function. Recent work with human and non-human primates, for example, is exploring the relationship between the electrophysiology of sleep (e.g., occurrence of sleep spindles) to memory consolidation processes (200–202). Of note, standard clinical neuropsychological batteries only assess patients after time spans under 1 h and never assess patients after periods of sleep (203). This type of work could be carried out in the EMU with intracranially implanted patients, although not without its own set of confounds (e.g., accounting for potential changes in memory related to recent seizure occurrence, changes in antiseizure medications, and a disruptive hospital environment and sleep schedule). Nevertheless, SEEG paradigms with the use of CCEPs is potentially a powerful tool to explore the sub-circuitry of memory processes. George Ojemann carried out a number of memory studies using ESM over the years, and some of his research suggested that the memory system was more complicated than suggested by theoretical models [e.g., episodic memory was disrupted by stimulation of the lateral temporal cortex: (44, 144)]. These findings have more recently been supported by more recent stimulation work through DARPA [i.e., stimulation of lateral TL cortex enhanced memory at some frequencies (146)], and by some initial clinical data involving minimalistic approaches to surgery [lateral TL ablations led to significant verbal memory dysfunction despite preservation of medial TL structures (48)].
Extraoperative single unit recordings can be made when intracranial electrodes are implanted for clinical reasons in patients with refractory epilepsy [see (204)]. While they have provided important insights into memory and cognition, there are a number of limitations. Particularly, it is difficult to continue to record from the same unit for long periods, cell types can be inferred, but not exquisitely defined, and the few neurons that can be recorded, as well as the inability to cover all areas involved in every function (205).
Finally, the possibility of an “electric Wada” seems surprisingly absent from the epilepsy surgery landscape. At least, in the case of patients undergoing invasive monitoring with hippocampal electrodes, it is possible to carry out Wada memory paradigms while disrupting subregions and interconnections of the hippocampus and amygdala or even broader structures (145). This seems particularly relevant in this era of minimally invasive surgical procedures as compared to prior surgical epochs where essentially all patients were undergoing procedures that resected multiple regions of the temporal lobe (including much of the temporal pole, anterior inferior and middle temporal gyrus, fusiform, basal temporal lobe, entorhinal/perirhinal cortex, etc.). The Wada is a blunt test in its own right, with many studies suggesting variability in which brain regions are being affected by the delivery of drug [e.g., sodium amobarbital, brevital (206)]. This was less of a problem with open resective surgery but the Wada test may not adequately reflect the potential outcome of a stereotactic laser amygdalophippocampotomy, which primarily affects the amgdalohippocampal complex. Creating an electric Wada appears to be an area where SEEG will be well-suited, but disruptive stimulation will need to be thoughtfully deployed with very low current intensities and careful tailoring based on the stimulation site: Most patients that would be candidates will have medial temporal onset zones—higher frequency stimulation readily causes seizures when applied to the hippocampus and is often avoided (72).
Social-Emotional Functions
There is increasing recognition of the importance of brain functions beyond cognitive processing, as the preservation of social cognition, emotional processing, and empathy may be equally important in determining quality of life (207), and often represent some of core areas of dysfunction in disorders such as autism and schizophrenia (208, 209). Over the years, socio-emotional tasks (e.g., recognizing emotional state from facial expressions, emotional prosody, self-other distinction, empathy, embodied rotation, and theory of mind tasks) have been shown to depend heavily on the limbic system with significant contributions from the amygdalohippocampal complex, the insula, the cingulate cortex, the anterior temporal lobe (e.g., superior temporal pole), select regions of the broader temporal lobe (e.g., the right temporo-parietal junction), the right dorso-medial prefrontal cortex, the bilateral inferior parietal lobules, and more recently the “default mode network (DMN)” (210–215). The latter is a proposed network derived from functional neuroimaging research that appears to activate whenever an individual is not actively engaged in a task in their environment, and which has been linked to a wide range of cognitive [e.g., forming self-relevant mental constructs, planning for future (216)] and social functions in its own right [e.g., social understanding of others, morality (211, 214)]. These findings relating socio-emotional functions to neural underpinnings were originally formulated from naturally occurring lesion studies in humans and ablation studies in non-human primates (217–221), and have been augmented over the years by data derived from functional neuroimaging studies and to a lesser extent electrophysiological investigation (222, 223). Catani et al. (224) provide a thorough review of the contributors over the past century and a half to these developments, and offer an updated model for the neural substrates underlying emotion, memory, and behavior. These authors reviewed the pioneering work of Papez (225), Yakolev (226), and MacLean (227, 228) among notable contributors, and incorporated the DMN into these earlier models, noting that this network shares neuroanatomical overlap with Papez's circuit. More specifically, the medial aspects of the DMN correspond to the most dorsal aspects of the Papez circuit and are interconnected through the dorsal cingulum. While this is an interesting model, it is worth noting that Papez's circuit was proposed as a mechanism of emotion (225) before the idea of the hippocampus in memory took hold (121), after which Papez's circuit was then recast as a mechanism for memory. Similarly, while ontogeny has some vague relation to the work of MacLean (227), this is a model that has insufficient detail to have explanatory traction in functional localization. Nevertheless, the use of cortical and subcortical mapping paradigms, rarely performed in the thalamus and hypothalamic hamartoma, have not been fully realized as the valuable opportunities that they represent, particularly through the merging of technological advances to create real-life emotional processing situations (e.g., using virtual and augmented reality techniques) that can be coupled with novel data collection methods (e.g., CCEPs, machine learning). In many regards, despite these regions or connections to them being disrupted in many epilepsy surgeries and tumor cases, there has been little clinical attention focused on this likely critical area of function for all of the reasons previously cited.
Theory of mind in particular has emerged as an important facet of social cognition and is one of the few areas that has received some degree of attention in the surgical setting. Critical to this ability—which involves the inference of others' mental/affective states and prediction of behavior(s)—are the posterior inferior frontal gyrus, dorsolateral prefrontal cortex, posterior superior temporal gyrus, and right temporo-parietal junction (207, 229, 230). One such measure that has been adapted to the neurosurgical setting is the Reading the Mind in the Eyes task (229), which was developed for use in the autistic population. This task consists of patients matching one of four affective states to 36 serially presented photographs depicting only the eye region of human faces. Use of this novel task has facilitated mapping of theory of mind, and research has shown that patients do not completely recover this ability following pars opercularis resection (231, 232). Additionally, however, we recently had a teenager decline drastically on this task (normal to impaired) who underwent a left stereotactic laser amygdalohippocampotomy only, and whose dysfunction has persisted over time (233). This highlights the need for further study of the regions critical for such functions as well as individual variability that may occur across patients. Finally, mapping of the insular cortex has also led to some interesting disruptions of potential socio-emotional functions, as well as internal perceptions or interoception, including pain sensation, interoceptive awareness, emotions, self-recognition, empathy, motivation, craving, alterations in breathing, and time perception (234).
Consciousness
An ontology of consciousness is developing, with a key and important example of the necessity of a clear ontology in Antti Revonsuo's Inner Presence (235). This has helped neuroscience move away from imprecise and all-encompassing notions of consciousness, as well as behavioral approaches to consciousness from clinical neurology, where the level of arousal is a major interest. While important recent work has taken this latter approach to study minimally conscious states, often in the setting of diffuse or at least non-focal brain injury [e.g., (236)], other work has brought us close to examining subject-reported inner experience. Of particular note is the extension of Baars “Global Workspace Theory” from the 1980s [see (237)] to neural substrates and cognitive theory. This approach has informed both cognitive and psychological ideas regarding phenomenal consciousness (see Revonsuo) or subjective awareness—a core component of consciousness—such as in the work of Dehaene [e.g., (238)], or in the sphere of SEEG studies, where this has been developed by Naccache et al. (239–246). This work argues, with empirical evidence, that association cortices are fundamental to phenomenal consciousness. Disfunction of these regions in turn leads to a loss of awareness. It is important to note that while phenomenal consciousness may represent a core component of consciousness perhaps its sine qua non, there are other elements that are necessary to normal conscious experience that call for mechanistic explanations, such as metacognition, reflection, selfhood, embodiment, autobiographical narrative, introspection, etc. While all of these seem amenable to well-designed studies that might take advantage of the spatial and temporal resolution of SEEG, sparse sampling needs to be overcome, perhaps by combination with functional imaging methods. When rejecting the behaviorist notion that subjective report is not scientific, and accepting that within certain wide bounds, subjectivity is a core and valid type of data [see, for example (238)]. With a better framework for considering the important constituents of consciousness (e.g., wakefulness, perception, attention, multi-modal representation, mnemonic processing, meta-cognitive processes), the time seems right to begin exploring aspects of these mechanisms. To achieve this, new tasks and experimental paradigms are needed.
Modernization and Standardization of Testing Paradigms and Techniques
It is important to take a highly individualized and functional anatomically informed approach to task selection to optimize mapping/monitoring for any given patient. In addition to greater focus on task development, there is a need to develop effective and innovative methods for administering cognitive testing during ESM more considerate to efficiency and portability of both stimuli and capture methods. Laptop-based systems are widely utilized to display stimuli to patients (e.g., Powerpoint presentations) and allow for extensive stimuli to be stored and displayed electronically but the transition to computers alone does not address the often unstructured and highly variable testing methods used across institutions. Increased consensus in this regard would be valuable in allowing for more comprehensive research endeavors with larger samples across collaborating institutions and further identification of best practices. It would also allow for sharing of common test stimuli, making it possible for more groups to consider more sophisticated mapping paradigms that often take a great deal of preparation time to create. Consensus is becoming increasingly possible through development of specific surgical brain mapping software packages/applications that can be used in a standardized fashion across institutions. One such open-source testing platform is NeuroMapper, which was developed by one of the authors of this manuscript (DSS) (See Figure 6). It is utilized to administer a variety of cognitive paradigms to patients in a highly customized and flexible manner via dual iPads, allowing for highly individualized mapping by using baseline performance to select stimulus sets for mapping. This then allows examiners to quickly and easily code patient responses (e.g., correct/incorrect, types of errors made) and monitor changes in task accuracy and reaction times in real time, as well as to obtain video records of patients responses for later review. Another useful feature of NeuroMapper is its portability, given the relatively reduced size and weight of the iPad vs. more traditional laptop/computer setups.
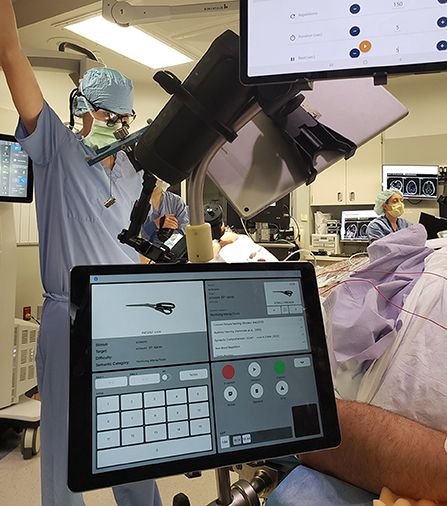
Figure 6. Intraoperative use of the iPad-based Neuromapper tool. Written informed consent was obtained from the individual for the publication of any potentially identifiable images.
We have already suggested many areas of additional test development (e.g., expanded testing of language, adaptation, and creation of tasks of socio-emotional function), but would also like to encourage the exploration of virtual reality and augmented reality techniques to expand the depth and range of constructs that are being examined. These technologies allow for an immersive experience which can be tightly controlled, observed, measured, and manipulated in a manner that allows us to move beyond simple measurement of function yet provides control of the environment while altering task components in a systematic manner. Given the construct of memory, for example, we can continue to test simple episodic recall of a list of words, a story, an object, or a visual scene, but we can also more easily add greater context to the learning, which can pull from semantic (factual information) and autobiographical information (the subject's own personal experiences) and can include both established and novel experiences and information as well. With eye-tracking, motion capture technologies, video-recording, and simultaneous electrophysiological measurement, we can study the patient thoroughly and in a precision manner not previously possible with paper and pencil or even computer-administered tasks. Our own group has created a series of video-vignettes (See Figure 7) with professional actors, which require the patient to learn the content of a brief episodic occurrence in the life of one or more individuals, while learning to recognize those individuals (including face, voice, etc.), the settings in which each event takes place, incidental occurrences in the background, and the interactions between these factors (e.g., “Which person was seen in a given context?” and “What other individuals and objects were present?”). We have created extensive foils that include altering subtle characteristics of each scene and its content to test the limits of recall, including potentially the stylistic manner of information retrieval (e.g., subtasks that can potentially tease out the reliance upon familiarity or recognition or a failure of pattern-separation vs. a failure of language, semantics, or other factors). With current technology and artistic capabilities scenes can be subtly manipulated to allow for the ultimate alternate test forms (e.g., making changes in objects or persons while leaving every other aspect of the scene exactly the same) or to apply eye-tracking mechanisms to determine if changes in repetitive scenes were even noticed based on existing literature on the visual fixation differences for novel vs. old information in patients and controls [a finding observed in human and non-human primates (247, 248)]. These sorts of complex tasks would be ideally suited to a situation in which passive mapping is being used, yet a simplified version (e.g., face-name learning) can be completed using an active stimulation paradigm.
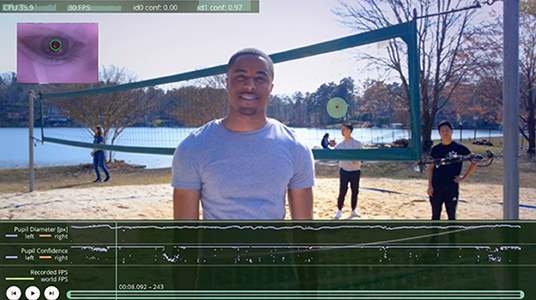
Figure 7. Emory Multimodal Memory Test. A new multimodal tool that is under development for the assessment of multiple domains of cognition and their integration, along with simultaneous recorded eye position and pupil diameter data. Written informed consent was obtained from the individual for the publication of any potentially identifiable images.
Some examples of the use of virtual reality and electrophysiological studies together already exist both with human, rodents, and non-human primates. Several studies have used virtual reality systems combined with implanted electrodes, for example, to study processes such as spatial navigation in rodents and non-human primates (249, 250). These studies have provided a number of interesting findings, facilitating insight into the neural substrates of allocentric (i.e., a more general, global directional sense) and egocentric navigation (a more local system, based on familiar landmarks and their spatial relationships), and other factors that facilitate or hinder navigation. It appears that the hippocampal formation is critical for allocentric navigation (along with parahippocampal and retrosplenial cortex), but that egocentric processes activate neural systems outside of the medial TL (including medial and posterior parietal lobe regions and caudate nucleus).
In humans, a group in France has started piloting the use of a virtual reality headset during awake surgeries in the operating room, using this system to test language but also to explore the ability to understand social gestures (251). There were limitations to what could be accomplished, but they are working on solutions to these issues through ongoing research. Over the last 2 years, virtual tasks have exploded, with ecologically realistic or episodic memory in a shop environment (252, 253), spatial orientation (254), attention (255), and large-scale spatial learning (256). Similarly, standard objects to use in creating these environments (257), and free programming tools (e.g., Unity by Unity Technologies) are making this easier and more standardized (See Figure 8). Combining these rich tasks with intracranial electrophysiology will be difficult, given the complex and unfolding nature of tasks, but much may also be learned. Overall, virtual and augmented reality paradigms will increase our ability to study complex phenomena in a highly controlled manner, and should lead to further insights into the neural substrates of complex behavior of all sorts, which will be helpful for better understanding disease states, navigating neurosurgical procedures to provide optimal benefit with the greatest sparing of function, enhancing our ability to use neuromodulation procedures to treat disease (e.g., seizures, depression) and potentially enhance/restore neurological function, and create the opportunity to develop brain-machine interfaces (259), such as those that have allowed the creation of a bionic prosthetic limb. Of note, all of these “futuristic” advances also call for the need for close collaboration with neuroethicists, discussion between cognitive psychologists and neuroscientists, and potentially input from neurophilosophy, to determine the best path forward in these new frontiers [e.g., (260)].
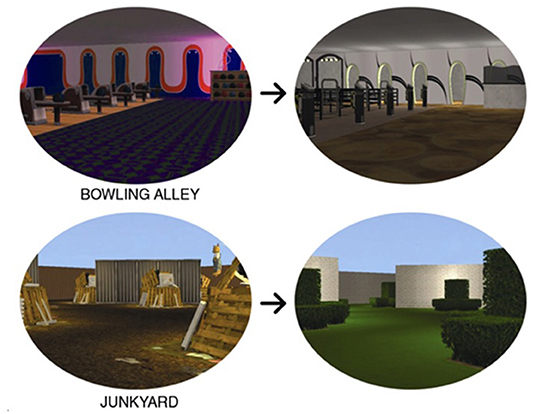
Figure 8. Examining meta-memory with a spatial task. In this virtual reality task from the Cleary Lab (258), subjects can rate feelings of familiarity and deja vu after flying through spatially similar scenes. This is presently being used in the setting of SEEG to examine the anatomy and network activity associated with familiarity. It is also under development with contemporary virtual reality hardware and software.
Conclusions
Cognitive and emotional mapping with SEEG holds great promise both as a clinical tool and research paradigm for significantly improving our understanding of brain structure-function networks. As laid out in this paper, by augmenting a rich history cortical stimulation mapping and SEEG mapping with advances in neuroimaging (e.g., connectivity metrics; precision volumetrics), neuropsychology/neurophilosophy (e.g., updating old models with advances in brain modeling and theory; adding new measures that tap the rich, complexity of thought and memory), technology (e.g., virtual/augmented reality for humans and non-human primates alike), electrophysiological processing and computational modeling (e.g., CCEPs, machine learning algorithms), the field is poised to make rapid advances. Such potential gains could not only improve the care of patients with brain tumors and epilepsy, but potentially allow us to better understand other neurological diseases (e.g., semantic dementia vs. Alzheimer's disease) while developing more targeted, novel treatments. For example, by understanding the neural circuitry of cognition and emotion and their dysfunction, we may be able to develop more specific drug treatments, better position neuromodulatory devices, or even learn to “relink” damaged pathways and circuits. There is still much exciting “building block” work to be done in each of these subfields, and those of us working with these tools and paradigms should be establishing consortiums to share ideas, resources, and data to enable exponential growth in this field over the next couple of decades.
Author's Note
This manuscript reflects a review of the relevant published research related to cognitive and behavioral mapping using passive and active stimulation electrophysiological paradigms. It has not been published in any other source and is the original work of the cited authors. DD and NP created an outline for the article, wrote major components, and invited and supervised co-authors with specific expertise in relevant areas to write subsections of the paper. All authors were given an opportunity to edit the manuscript prior to submission for publication.
Author Contributions
DD and NP planned the paper, created an outline, recruited co-authors to assist with writing specific subsections, wrote several subsections of the paper, and provide editorial oversight. DS, CB, AD, AA, and AK were assigned subsections to write based on their specific expertise, carried out systematic literature searches, and prepared a draft of their assigned section. All co-authors contributed to the editorial process. All authors contributed to the article and approved the submitted version.
Funding
DD's efforts on this paper were supported in part by funding received from the National Institutes of Health/National Institute of Neurological Disorders and Stroke (NIH/NINDS, R01NS088748). He also receives funding from additional grants from the NIH (R01MH118514; R01NS110347), as well as Medtronic, Inc. (A1321808). NPP was supported in part by funding received from the NIH/NINDS (K08NS105929) and CURE Epilepsy.
Conflict of Interest
DD receives ongoing funding from Medtronic, Inc. to run a Core Analysis Lab for neuroimaging and cognitive testing in one of their FDA trials, these funds did not contribute in any form to his role in this paper. They were not involved in this paper in any manner, including study design, data collection, analysis, or interpretation, the writing of this article or the decision to submit it for publication.
The remaining authors declare that the research was conducted in the absence of any commercial or financial relationships that could be construed as a potential conflict of interest.
Acknowledgments
We would like to thank our undergraduate students, Noah Okado and Rogelio Cortez-Cuevas, with their assistance in the preparations of Figures 7, 8, and our neurophilosophy collaborators, Drs. Anne Cleary and Joseph Neisser for contributing ideas related to our use of a deja vu assessment task.
References
1. Arya R, Ervin B, Holloway T, Dudley J, Horn PS, Buroker J, et al. Electrical stimulation sensorimotor mapping with stereo-EEG. Clin Neurophysiol. (2020) 131:1691–701. doi: 10.1016/j.clinph.2020.04.159
2. Chauvel P, Gonzalez-Martinez J, Bulacio J. Presurgical intracranial investigation in epilepsy surgery. Handb Clin Neurol. (2019) 161:45–71. doi: 10.1016/B978-0-444-64142-7.00040-0
3. Cardinale F, Casaceli G, Raneri F, Miller J, Lo Russo G. Implantation of stereoelectroencephalography electrodes: a systematic review. J Clin Neurophysiol. (2016) 33:490–502. doi: 10.1097/WNP.0000000000000249
4. Talairach J, Bancaud J, Bonis A, Szikla G, Tournoux P. Functional stereotactic exploration of epilepsy. Confin Neurol. (1962) 22:328–31. doi: 10.1159/000104378
5. Katz JS, Abel TJ. Stereoelectroencephalography versus subdural electrodes for localization of the epileptogenic zone: what is the evidence? Neurotherapeutics. (2019) 16:59–66. doi: 10.1007/s13311-018-00703-2
6. Willie JT, Laxpati NG, Drane DL, Gowda A, Appin A, Hao C, et al. Real-time magnetic resonance-guided amygdalohippocampotomy for mesial temporal lobe epilepsy. Neurosurgery. (2014) 74:569–84. doi: 10.1227/NEU.0000000000000343
7. Martin AK, Huang J, Hunold A, Meinzer M. Dissociable roles within the social brain for self-other processing: a HD-tDCS study. Cereb Cortex. (2019) 29:3642–54. doi: 10.1093/cercor/bhy238
8. Lee DJ, Lozano CS, Dallapiazza RF, Lozano AM. Current and future directions of deep brain stimulation for neurological and psychiatric disorders. J Neurosurg. (2019) 131:333–42. doi: 10.3171/2019.4.JNS181761
9. Skarpaas TL, Jarosiewicz B, Morrell MJ. Brain-responsive neurostimulation for epilepsy (RNS® System). Epilepsy Res. (2019) 153:68–70. doi: 10.1016/j.eplepsyres.2019.02.003
10. Piccolino M, Bresadola M. Shocking Frogs: Galvani, Volta, and the Electric Origins of Neuroscience. Oxford University Press, Oxford Scholarship Online (2013).
11. Fritsch G, Hitzig E. Über die elektrische erregbarkeit des grosshirns. Arch Anat Physiol Wissen. (1870) 37:300–32.
12. Engelhardt E. Cerebral localization of higher functions: the period between Thomas Willis and Paul Broca. Dementia and Neuropsychologia. (2019) 13:238–43. doi: 10.1590/1980-57642018dn13-020014
13. Ferrier S. Experimental researches in cerebral physiology and pathology. West Riding Lunatic Asylum Med Rep. (1873) 3:30–96. doi: 10.1136/bmj.1.643.457
14. Willis T. De anima brutorum, quae hominis vitalis ac sensitiua est, exercitationes duae. Leyden, IL: Joannis Antonii Huguetan and Soc (1676).
16. Hughlings Jackson J. Selected Writings of John Hughlings Jackson (Taylor J, editor). New York, NY: Basic Books (1958).
18. Horsley V, Clarke RH. The structure and functions of the cerebellum examined by a new method. Brain. (1908) 31:45–124. doi: 10.1093/brain/31.1.45
19. Cushing H. A note upon the faradic stimulation of the postcentral gyrus in conscious patients. Brain. (1909) 32:44–53. doi: 10.1093/brain/32.1.44
20. Isitan C, Yan Q, Spencer DD, Alkawadri R. Brief history of electrical cortical stimulation: a journey in time from Volta to Penfield. Epilepsy Res. (2020) 166:106363. doi: 10.1016/j.eplepsyres.2020.106363
22. Greenblatt SH. Phrenology in the science and culture of the 19th century. Neurosurgery. (1995) 37:790–804. doi: 10.1227/00006123-199510000-00025
23. Charcot JM. Lectures on the diseases of the nervous system, Vol 3. London: New Sydenham Society (1889)
24. Talairach J, Szikla G. Atlas d'anatomie stereotaxique du telencephale. Paris: Masson & Cie (1967).
25. Jensen RL, Stone JL, Hayne RA. Introduction of the human Horsley-Clarke stereotactic frame. Neurosurgery. (1996) 38:563–567. doi: 10.1097/00006123-199603000-00029
26. Bancaud J, Talairach J, Bonis A. La Stéréoencéphalographie dans l'épilepsie. Paris: Masson & Cie (1965).
27. Bancaud J, Talairach J. Méthodologie de l'exploration SEEG et de l'intervention chirurgicale dans l'épilepsie [Methodology of stereo EEG exploration and surgical intervention in epilepsy]. Rev Otoneuroophtalmol. (1973) 45:315–28.
28. Haglund MM, Berger MS, Shamseldin M, Lettich E, Ojemann GA. Cortical localization of temporal lobe language sites in patients with gliomas. Neurosurgery. (1994) 34:567–76. doi: 10.1227/00006123-199404000-00001
29. Hamberger MJ. Cortical language mapping in epilepsy: a critical review. Neuropsychol Rev. (2007) 17:477–89. doi: 10.1007/s11065-007-9046-6
30. Foerster O, Altenburger H. Elektrobiologische Vorgänge an der menschlichen Hirnrinde. Dtsch Z Nervenheilkd. (1935) 135:277–88. doi: 10.1007/BF01732786
31. Penfield W, Jasper H. Epilepsy and the Functional Anatomy of the Human Brain. Boston, MA: Little, Brown and Co (1954). doi: 10.1097/00007611-195407000-00024
32. Penfield W, Roberts L. Speech and Brain-Mechanisms. Princeton, NJ: Princeton University Press (1959).
33. Snyder PJ, Whitaker HA. Neurologic heuristics and artistic whimsy: the cerebral cartography of Wilder Penfield. J Hist Neurosci. (2013) 22:277–91. doi: 10.1080/0964704X.2012.757965
34. Drane DL, Pedersen NP. Knowledge of language function and underlying neural networks gained from complex partial seizures and epilepsy surgery. Brain Lang. (2019) 189:20–33. doi: 10.1016/j.bandl.2018.12.007
35. Dronkers N, Baldo JV. Language: aphasia. In: Squire LR, editor Encyclopedia of Neuroscience. Cambridge, MA: Elsevier Ltd. (2009). p. 343–8.
36. Duffau H, Moritz-Gasser S, Mandonnet E. A re-examination of neural basis of language processing: proposal of a dynamic hodotopical model from data provided by brain stimulation mapping during picture naming. Brain Lang. (2014) 131:1–10. doi: 10.1016/j.bandl.2013.05.011
38. Ojemann G, Ojemann J, Lettich E, Berger M. Cortical language localization in left, dominant hemisphere. An electrical stimulation mapping investigation in 117 patients. J Neurosurg. (1989) 71:316–26. doi: 10.3171/jns.1989.71.3.0316
39. Hickok G, Poeppel D. The cortical organization of speech processing. Nat Rev Neurosci. (2007) 8:393–402. doi: 10.1038/nrn2113
40. Corina DP, Loudermik BC, Detwiler L, Martin RF, Brinkley JF, Ojemann G. Analysis of naming errors during cortical stimulation mapping: implications for models of language representation. Brain Lang. (2010) 115:101–12. doi: 10.1016/j.bandl.2010.04.001
41. Ojemann GA. Individual variability in cortical localization of language. J Neurosurg. (1979) 50:164–9. doi: 10.3171/jns.1979.50.2.0164
42. Ojemann SG, Berger MS, Lettich E, Ojemann GA. Localization of language function in children: results of electrical stimulation mapping. J Neurosurg. (2003) 98:465–70. doi: 10.3171/jns.2003.98.3.0465
43. Drane DL, Roraback-Carson J, Hebb AO, Hersonskey T, Lucas T, Ojemann GA, et al. Cortical stimulation mapping and Wada results demonstrate a normal variant of right hemisphere language organization. Epilepsia. (2012) 53:1790–8. doi: 10.1111/j.1528-1167.2012.03573.x
44. Ojemann GA, Whitaker HA. The bilingual brain. Arch Neurol. (1978) 35:409–12. doi: 10.1001/archneur.1978.00500310011002
45. Ojemann GA, Whitaker H. Language localization and variability. Brain Lang. (1978) 6:239–60. doi: 10.1016/0093-934X(78)90061-5
46. Ojemann GA, Creutzfeldt O, Lettich E, Haglund MM. Neuronal activity in human lateral temporal cortex related to short-term verbal memory, naming and reading. Brain. (1988) 111(Pt 6):1383–403. doi: 10.1093/brain/111.6.1383
47. Ojemann G, Mateer C. Human language cortex: localization of memory, syntax, and sequential motor-phoneme identification systems. Science. (1979) 205:1401–3. doi: 10.1126/science.472757
48. Drane DL. MRI-guided stereotactic laser ablation for epilepsy surgery: promising preliminary results for cognitive outcome. Epilepsy Res. (2018) 142:170–5. doi: 10.1016/j.eplepsyres.2017.09.016
49. Lüders H, Hahn J, Lesser RP, Dinner DS, Morris HH 3rd, Wyllie E, et al. Basal temporal subdural electrodes in the evaluation of patients with intractable epilepsy. Epilepsia. (1989) 30:131–42. doi: 10.1111/j.1528-1157.1989.tb05445.x
50. Lüders H, Lesser RP, Dinner DS, Morris HH, Hahn J. Language disturbances produced by electrical stimulation of the basal temporal region. Ann Neurol. (1985) 18:151.
51. Lüders J, Lesser RP, Hahn J, Dinner DS, Morris HH, Wyllie E, et al. Basal temporal language area. Brain. (1991) 114:743–54. doi: 10.1093/brain/114.2.743
52. Bartha L, Benke T, Bauer G, Trinka E. Interictal language functions in temporal lobe epilepsy. J Neurol Neurosurg Psychiatry. (2005) 76:808–14. doi: 10.1136/jnnp.2004.045385
53. Bartha L, Trinka E, Ortler M, Donnemiller E, Felber S, Bauer G, et al. Linguistic deficits following left selective amygdalohippocampectomy: a prospective study. Epilepsy Behav. (2004) 5:348–57. doi: 10.1016/j.yebeh.2004.02.004
54. Pouratian N, Bookheimer SY, Rubino G, Martin NA, Toga AW. Category-specific naming deficit identified by intraoperative stimulation mapping and postoperative neuropsychological testing. Case Rep J Neurosurg. (2003) 99:170–6. doi: 10.3171/jns.2003.99.1.0170
55. Usui K, Ikeda A, Takayama M, Matsuhashi M, Yamamoto J-I, Satoh T, et al. Conversion of semantic information into phonological representation: a function in left posterior basal temporal area. Brain. (2003) 126:632–41. doi: 10.1093/brain/awg057
56. Araki K, Terada K, Usui K, Usui N, Araki Y, Baba K, et al. Bidirectional neural connectivity between basal temporal and posterior language areas in humans. Clin Neurophysiol. (2015) 126:682–8. doi: 10.1016/j.clinph.2014.07.020
57. Kahane P, Arzimanoglou A, Benabid A-L, Chauvel P. Epilepsy surgery in France. In: Luders HO, editor. Textbook of Epilepsy Surgery. London: Informa Healthcare (2008). p. 46–53.
58. Bancaud J. Apport de l'exploration fonctionnelle par voie stéréotaxique à la chirurgie de l'épilepsie. Neurochirurgie. (1959) 5:55–112.
59. Talairach J, Bancaud J. Stereotaxic approach to epilepsy. Methodology of anatomo- functional stereotaxic investigations. Progr Neurol Surg. (1973) 5:297–354. doi: 10.1159/000394343
60. Trébuchon A, Liégeois-Chauvel C, Gonzalez-Martinez JA, Alario F-X. Contributions of electrophysiology for identifying cortical language systems in patients with epilepsy. Epilepsy Behav. (2020) 112:1525–5050. doi: 10.1016/j.yebeh.2020.107407
61. Yamao Y, Suzuki K, Kunieda T, Matsumoto R, Arakawa Y, Nakae T, et al. Clinical impact of intraoperative CCEP monitoring in evaluating the dorsal language white matter pathway. Hum Brain Mapp. (2017) 38:1977–91. doi: 10.1002/hbm.23498
62. Duffau H, Peggy Gatignol ST, Mandonnet E, Capelle L, Taillandier L. Intraoperative subcortical stimulation mapping of language pathways in a consecutive series of 115 patients with Grade II glioma in the left dominant hemisphere. J Neurosurg. (2008) 109:461–71. doi: 10.3171/JNS/2008/109/9/0461
63. Duffau H. Stimulation mapping of white matter tracts to study brain functional connectivity. Nat Rev Neurol. (2015) 11:255–65. doi: 10.1038/nrneurol.2015.51
64. Herbet G, Moritz-Gasser S, Boiseau M, Duvaux S, Cochereau J, Duffau H. Converging evidence for a cortico-subcortical network mediating lexical retrieval. Brain. (2016) 139:3007–21. doi: 10.1093/brain/aww220
65. Martino J, Brogna C, Robles SG, Vergani F, Duffau H. Anatomic dissection of the inferior fronto-occipital fasciculus revisited in the lights of brain stimulation data. Cortex. (2010) 46:691–9. doi: 10.1016/j.cortex.2009.07.015
66. Yordanova YN, Cochereau J, Duffau H, Herbet G. Combining resting state functional MRI with intraoperative cortical stimulation to map the mentalizing network. NeuroImage. (2019) 186:628–36. doi: 10.1016/j.neuroimage.2018.11.046
67. Leclercq D, Duffau H, Delmaire C, Capelle L, Gatignol P, Ducros M, et al. Comparison of diffusion tensor imaging tractography of language tracts and intraoperative subcortical stimulations. J Neurosurg. (2010) 112:503–11. doi: 10.3171/2009.8.JNS09558
68. Fernandez Coello A, Duvaux S, De Benedictis A, Matsuda R, Duffau H. Involvement of the right inferior longitudinal fascicle in visual hemiagnosia: a brain stimulation mapping study. J Neurosurg. (2013) 118:202–5. doi: 10.3171/2012.10.JNS12527
69. Gil-Robles S, Carvallo A, Jimenez M, Gomez Caicoya A, Martinez R, Ruiz-Ocaña C, et al. Double dissociation between visual recognition and picture naming: a study of the visual language connectivity using tractography and brain stimulation. Neurosurgery. (2013) 72:678–86. doi: 10.1227/NEU.0b013e318282a361
70. Moritz-Gasser S, Herbet G, Maldonado IL, Duffau H. Lexical access speed is significantly correlated with the return to professional activities after awake surgery for low-grade gliomas. J Neuro Oncol. (2012) 107:633–41. doi: 10.1007/s11060-011-0789-9
71. Munyon C, Sweet J, Luders H, Lhatoo S, Miller J. The 3-dimensional grid: a novel approach to stereoelectroencephalography. Neurosurgery. (2015) 11:127–34. doi: 10.1227/NEU.0000000000000649
72. Isnard J, Taussig D, Bartolomei F, Bourdillon P, Catenoix H, Chassoux F, et al. French guidelines on stereoelectroencephalography (SEEG). Clin Neurophysiol. (2018) 48:5–13. doi: 10.1016/j.neucli.2017.11.005
73. von Economo C. The Cytoarchitectonics of the Human Cerebral Cortex. London: Oxford University Press (1929).
74. Borchers S, Himmelbach M, Logothetis N, Karnath HO. Direct electrical stimulation of human cortex - the gold standard for mapping brain functions? Nat Rev Neurosci. (2011) 13:63–70. doi: 10.1038/nrn3140
75. Mohan UR, Watrous AJ, Miller JF, Lega BC, Sperling MR, Worrell GA, et al. The effects of direct brain stimulation in humans depend on frequency, amplitude, white-matter proximity. Brain Stimul. (2020) 13:1183–95. doi: 10.1016/j.brs.2020.05.009
76. Cogan SF, Ludwig KA, Welle CG, Takmakov P. Tissue damage thresholds during therapeutic electrical stimulation. J Neural Eng. (2016) 13:021001. doi: 10.1088/1741-2560/13/2/021001
77. Bari BA, Ollerenshaw DR, Millard DC, Wang Q, Stanley GB. Behavioral and electrophysiological effects of cortical microstimulation parameters. PLoS ONE. (2013) 8:e82170. doi: 10.1371/journal.pone.0082170
78. Bancaud J, Talairach J. Organisation fonctionnelle de l'aire motrice supplémentaire. Enseignements apportés par la stéréo-E.E.G [Functional organization of the supplementary motor area. Data obtained by stereo-E.E.G]. Neurochirurgie. (1967) 13:343-56. French.
79. McCreery DB, Agnew WF, Yuen TG, Bullara L. Charge density and charge per phase as cofactors in neural injury induced by electrical stimulation. IEEE Trans Bio Med Eng. (1990) 37:996–1001. doi: 10.1109/10.102812
80. Jones JC, Alomar S, McGovern RA, Firl D, Fitzgerald Z, Gale J, Gonzalez-Martinez JA. Techniques for placement of stereotactic electroencephalographic depth electrodes: Comparison of implantation and tracking accuracies in a cadaveric human study. Epilepsia. (2018) 59:1667–1675. doi: 10.1111/epi.14538
81. Cardinale F, Rizzi M, Vignati E, Cossu M, Castana L, d'Orio P, et al. Stereoelectroencephalography: retrospective analysis of 742 procedures in a single centre. Brain. (2019) 142:2688–704. doi: 10.1093/brain/awz196
82. Arya R, Mangano FT, Horn PS, Holland KD, Rose DF, Glauser TA. Adverse events related to extraoperative invasive EEG monitoring with subdural grid electrodes: a systematic review and meta-analysis. Epilepsia. (2013) 54:828–39. doi: 10.1111/epi.12073
83. Helmstaedter C, Gielen GH, Witt JA. The immediate and short-term effects of bilateral intrahippocampal depth electrodes on verbal memory. Epilepsia. (2018) 59:e78–84. doi: 10.1111/epi.14019
84. Ljung H, Nordlund A, Strandberg M, Bengzon J, Källén K. Verbal memory decline from hippocampal depth electrodes in temporal lobe surgery for epilepsy. Epilepsia. (2017) 58:2143–52. doi: 10.1111/epi.13931
85. Drane DL, Gross RE. Hippocampal depth electrodes in epilepsy surgery: diagnostic or damaging? Epilepsy Curr. (2018) 18:104–6. doi: 10.5698/1535-7597.18.2.104
86. Drane DL, Pedersen NP, Hewitt KC, Jordan T. Promise and perils of SEEG for cognition and emotion in the epilepsy surgery setting. In: S. U. Schuele, editor. A Practical Approach to Stereo EEG, New York, NY: Springer (2021) 431–438.
87. Meador KJ, Halpern CH, Hermann BP. Cognitive safety of intracranial electrodes for epilepsy. Epilepsia. (2018) 59:1132–7. doi: 10.1111/epi.14197
88. Aungaroon G, Vera AZ, Horn PS, Byars AW, Greiner HM, Tenney JR, et al. After-discharges and seizures during pediatric extra-operative electrical cortical stimulation functional brain mapping: incidence, thresholds, and determinants. Clin Neurophysiol. (2017) 128:2078–86. doi: 10.1016/j.clinph.2017.06.259
89. Bank AM, Schevon CA, Hamberger MJ. Characteristics and clinical impact of stimulation-evoked seizures during extraoperative cortical mapping. Epilepsy Behav. (2014) 34:6–8. doi: 10.1016/j.yebeh.2014.03.004
90. Crone NE, Boatman D, Gordon B, Hao L. Induced electrocorticographic gamma activity during auditory perception. Brazier Award-winning article. Clin Neurophysiol. (2001) 112:565–82. doi: 10.1016/S1388-2457(00)00545-9
91. Babajani-Feremi A, Wheless JW, Papanicolaou JW, Wang Y, Fifer MS, Flinker A, et al. Spatial-temporal functional mapping of language at the bedside with electrocorticography. Neurology. (2016) 87:2604. doi: 10.1212/01.wnl.0000511287.40052.8d
92. Miller KJ, Schalk G, Hermes D, Ojemann JG, Rao RP. Spontaneous decoding of the timing and content of human object perception from cortical surface recordings reveals complementary information in the event-related potential and broadband spectral change. PLoS Comput Biol. (2016) 12:e1004660. doi: 10.1371/journal.pcbi.1004660
93. Su DK, Ojemann JG. Electrocorticographic sensorimotor mapping. Clin Neurophysiol. (2013) 124:1044–8. doi: 10.1016/j.clinph.2013.02.114
94. Van Poppel M, Wheless JW, Clarke DF, McGregor A, McManis MH, Perkins FF, et al. Passive language mapping with magnetoencephalography in pediatric patients with epilepsy. J Neurosurg Pediatrics. (2012) 10:96–102. doi: 10.3171/2012.4.PEDS11301
95. Bagshaw AP, Jacobs J, LeVan P, Dubeau F, Gotman J. Effect of sleep stage on interictal high-frequency oscillations recorded from depth macroelectrodes in patients with focal epilepsy. Epilepsia. (2009) 50:617–28. doi: 10.1111/j.1528-1167.2008.01784.x
96. Burke JF, Sharan AD, Sperling MR, Ramayya AG, Evans JJ, Healey MK, et al. Theta and high-frequency activity mark spontaneous recall of episodic memories. J Neurosci. (2014) 34:11355–65. doi: 10.1523/JNEUROSCI.2654-13.2014
97. Jerbi K, Freyermuth S, Dalal S, Kahane P, Bertrand O, Berthoz A, et al. Saccade related gamma-band activity in intracerebral EEG: dissociating neural from ocular muscle activity. Brain Topogr. (2009) 22:18–23. doi: 10.1007/s10548-009-0078-5
98. Korostenskaja M, Wilson AJ, Rose DF, Brunner P, Schalk G, Leach J, et al. Real-time functional mapping with electrocorticography in pediatric epilepsy: comparison with fMRI and ESM findings. Clin EEG Neurosci. (2014) 45:205–11. doi: 10.1177/1550059413492960
99. Swift JR, Coon WG, Guger C, Brunner P, Bunch M, Lynch T, et al. Passive functional mapping of receptive language areas using electrocorticographic signals. Clin Neurophysiol. (2018) 129:2517–24. doi: 10.1016/j.clinph.2018.09.007
100. Arya R, Wilson JA, Vannest J, Byars AW, Greiner HM, Buroker J, et al. Electrocorticographic language mapping in children by high-gamma synchronization during spontaneous conversation: comparison with conventional electrical cortical stimulation. Epilepsy Res. (2015) 110:78–87. doi: 10.1016/j.eplepsyres.2014.11.013
101. Arya R, Wilson JA, Fujiwara H, Rozhkov L, Leach JL, Byars AW, et al. Presurgical language localization with visual naming associated ECoG high-gamma modulation in pediatric drug-resistant epilepsy. Epilepsia. (2017) 58:663–73. doi: 10.1111/epi.13708
102. Wang Y, Fifer MS, Flinker A, Korzeniewska A, Cervenka MC, Anderson WS, et al. Spatial-temporal functional mapping of language at the bedside with electrocorticography. Neurology. (2016) 86:1181–9. doi: 10.1212/WNL.0000000000002525
103. Cervenka MC, Corines J, Boatman-Reich DF, Eloyan A, Sheng X, Franaszczuk PJ, et al. Electrocorticographic functional mapping identifies human cortex critical for auditory and visual naming. NeuroImage. (2013) 69:267–76. doi: 10.1016/j.neuroimage.2012.12.037
104. Kojima K, Brown EC, Matsuzaki N, Rothermel R, Fuerst D, Shah A, et al. Gamma activity modulated by picture and auditory naming tasks: intracranial recording in patients with focal epilepsy. Clin Neurophysiol. (2013) 124:1737–44. doi: 10.1016/j.clinph.2013.01.030
105. Cuisenier P, Testud B, Minotti L, El Bouzaïdi Tiali S, Martineau L, Job A-S. Relationship between direct cortical stimulation and induced high-frequency activity for language mapping during SEEG recording. J Neurosurg. (2020) 2020:1–11. doi: 10.3171/2020.2.JNS192751
106. Anumanchipalli GK, Chartier J, Chang EF. Speech synthesis from neural decoding of spoken sentences. Nature. (2019) 568:493–8. doi: 10.1038/s41586-019-1119-1
107. Sellars W. Empiricism and the philosophy of mind. In: Feigl H, Scriven M, editor. The Foundations of Science and the Concepts of Psychology and Psychoanalysis: Minnesota Studies in the Philosophy of Science, Vol. 1. Minneapolis, MN: University of Minnesota Press (1956). p. 253–329.
111. Zaborszky L, Wouterlood FG, Lanciego JL, editors. Neuroanatomical Tract Tracing 3: Molecules, Neurons, and Systems. 3rd ed. New York, NY: Springer (2006).
112. Lanciego JL, Wouterlood FG. Neuroanatomical tract-tracing techniques that did go viral. Brain Struct Function. (2020) 225:1193–224. doi: 10.1007/s00429-020-02041-6
113. Park HJ, Friston K. Structural and functional brain networks: from connections to cognition. Science. (2013) 342:1238411. doi: 10.1126/science.1238411
114. Mortazavi F, Oblak AL, Morrison WZ, Schmahmann JD, Stanley HE, Wedeen VJ, Rosene DL. Geometric Navigation of Axons in a Cerebral Pathway: Comparing dMRI with Tract Tracing and Immunohistochemistry. Cereb Cortex. (2018) 28:1219–1232. doi: 10.1093/cercor/bhx034
115. Matsumoto R, Kunieda T, Nair D. Single pulse electrical stimulation to probe functional and pathological connectivity in epilepsy. Seizure. (2017) 44:27–36. doi: 10.1016/j.seizure.2016.11.003
116. Dickey AS, Isbaine F, Fasano RE, Cabaniss BT, Gross RE, Willie JT, et al. Single-pulse electrical stimulation mapping of the connectivity of malformations of cortical development. Presented at the annual meeting of the American Epilepsy Society (AES). Washington, DC (2017).
117. Brazier MA. Evoked responses recorded from the depths of the human brain. Ann NY Acad Sci. (1964) 112:33–59. doi: 10.1111/j.1749-6632.1964.tb26741.x
118. Matsumoto R, Nair DR, LaPresto E, Najm I, Bingaman W, Shibasaki H, Lüders HO. Functional connectivity in the human language system: a cortico-cortical evoked potential study. Brain. (2004) 127:2316–30. doi: 10.1093/brain/awh246
119. Yamao Y, Matsumoto R, Kikuchi T, Yoshida K, Kunieda T, Miyamoto S. Intraoperative Brain Mapping by Cortico-Cortical Evoked Potential. Front Hum Neurosci. (2021) 15:635453. doi: 10.3389/fnhum.2021.635453
120. Matsumoto R, Nair DR, LaPresto E, Bingaman W, Shibasaki H, Lüders HO. Functional connectivity in human cortical motor system: a cortico-cortical evoked potential study. Brain. (2007) 130:181–97. doi: 10.1093/brain/awl257
121. Scoville WB, Milner B. Loss of recent memory after bilateral hippocampal lesions. J Neurol Neurosurg Psychiatr. (1957) 296:1–22. doi: 10.1136/jnnp.20.1.11
122. Rossi M, Nibali MC, Torregrossa F, Bello L, Grasso G. Innovation in neurosurgery: the concept of cognitive mapping. World Neurosurg. (2019) 131:364–70. doi: 10.1016/j.wneu.2019.06.177
123. Hamberger MJ, Goodman RR, Perrine K, Tamny T. Anatomic dissociation of auditory and visual naming in the lateral temporal cortex. Neurology. (2001) 56:56–61. doi: 10.1212/wnl.56.1.56
124. Duffau H, Capelle L, Denvil D, Gatignol P, Sichez N, Lopes M, et al. The role of dominant premotor cortex in language: a study using intraoperative functional mapping in awake patients. Neuroimage. (2003) 20:1903–14. doi: 10.1016/S1053-8119(03)00203-9
125. Ulvin LB, Jonas J, Brissart H, Colnat-Coulbois S, Thiriaux A, Vignal JP, et al. Intracerebral stimulation of left and right ventral temporal cortex during object naming. Brain Lang. (2017) 175:71–6. doi: 10.1016/j.bandl.2017.09.003
126. Sarubbo S, Tate M, De Benedictis A, Merler S, Moritz-Gasser S, Herbet G, et al. A normalized dataset of 1821 cortical and subcortical functional responses collected during direct electrical stimulation in patients undergoing awake brain surgery. Neuroimage. (2020) 28:104892. doi: 10.1016/j.dib.2019.104892
127. Maldonado IL, Moritz-Gasser S, de Champfleur NM, Bertram L, Moulinié G, Duffau H. Surgery for gliomas involving the left inferior parietal lobule: new insights into the functional anatomy provided by stimulation mapping in awake patients. J Neurosurg. (2011) 115:770–9. doi: 10.3171/2011.5.JNS112
128. Miozzo M, Williams AC, McKhann GM 2nd, Hamberger MJ. Topographical gradients of semantics and phonology revealed by temporal lobe stimulation. Hum Brain Mapp. (2017) 38:688–703. doi: 10.1002/hbm.23409
129. Abel TJ, Rhone AE, Nourski KV, Kawasaki H, Oya H, Griffiths TD, et al. Direct physiologic evidence of a heteromodal convergence region for proper naming in human left anterior temporal lobe. J Neurosci. (2015) 35:1513–20. doi: 10.1523/JNEUROSCI.3387-14.2015
130. Roux FE, Lubrano V, Lauwers-Cances V, Trémoulet M, Mascott CR, Démonet JF. Intra-operative mapping of cortical areas involved in reading in mono- and bilingual patients. Brain. (2004) 127(Pt 8):1796–810. doi: 10.1093/brain/awh204
131. Sabsevitz DS, Middlebrooks EH, Tatum W, Grewal SS, Wharen R, Ritaccio AL. Examining the function of the visual word form area with stereo EEG electrical stimulation: a case report of pure alexia. Cortex. (2020) 129:112–8. doi: 10.1016/j.cortex.2020.04.012
132. Blanke O, Spinelli L, Thut G, Michel C, Perrig S, Landis T, et al. Location of the human frontal eye field as defined by electrical cortical stimulation: anatomical, functional and electrophysiological characteristics. Neuroreport. (2000) 11:1907–13. doi: 10.1097/00001756-200006260-00021
133. Halgren E, Walter RD, Cherlow DG, Crandall PH. Mental phenomena evoked by electrical stimulation of the human hippocampal formation and amygdala. Brain. (1978) 101:83–117. doi: 10.1093/brain/101.1.83
134. Gloor P. Experiential phenomena of temporal lobe epilepsy. Facts and hypotheses. Brain. (1990) 113 (Pt 6):1673–94. doi: 10.1093/brain/113.6.1673
135. Bartolomei F, Barbeau E, Gavaret M, Guye M, McGonigal A, Régis J, et al. Cortical stimulation study of the role of rhinal cortex in déjà vu and reminiscence of memories. Neurology. (2004) 63:858–64. doi: 10.1212/01.WNL.0000137037.56916.3F
136. Lanteaume L, Khalfa S, Régis J, Marquis P, Chauvel P, Bartolomei F. Emotion induction after direct intracerebral stimulations of human amygdala. Cereb Cortex. (2007) 17:1307–13. doi: 10.1093/cercor/bhl041
137. Vignal JP, Chauvel P, Halgren E. Localised face processing by the human prefrontal cortex: stimulation-evoked hallucinations of faces. Cogn Neuropsychol. (2000) 17:281–91. doi: 10.1080/026432900380616
138. Barbeau EJ, Taylor MJ, Regis J, Marquis P, Chauvel P, Liégeois-Chauvel C. Spatio temporal dynamics of face recognition. Cerebral Cortex. (2008) 18:997–1009. doi: 10.1093/cercor/bhm140
139. Roux FE, Dufor O, Lauwers-Cances V, Boukhatem L, Brauge D, Draper L, et al. Electrostimulation mapping of spatial neglect. Neurosurgery. (2011) 69:1218–31. doi: 10.1227/NEU.0b013e31822aefd2
140. Bush D, Bisby JA, Bird CM, Gollwitzer S, Rodionov R, Diehl B, et al. Human hippocampal theta power indicates movement onset and distance travelled. Proc Natl Acad Sci USA. (2017) 114:12297–302. doi: 10.1073/pnas.1708716114
141. Maidenbaum S, Miller J, Stein JM, Jacobs J. Grid-like hexadirectional modulation of human entorhinal theta oscillations. Proc Natl Acad Sci USA. (2018) 115:10798–803. doi: 10.1073/pnas.1805007115
142. Duffau H, Denvil D, Lopes M, Gasparini F, Cohen L, Capelle L, et al. Intraoperative mapping of the cortical areas involved in multiplication and subtraction: an electrostimulation study in a patient with a left parietal glioma. J Neurol Neurosurg Psychiatry. (2002) 73:733–8. doi: 10.1136/jnnp.73.6.733
143. Yu X, Chen C, Pu S, Wu C, Li Y, Jiang T, et al. Dissociation of subtraction and multiplication in the right parietal cortex: evidence from intraoperative cortical electrostimulation. Neuropsychologia. (2011) 49:2889–95. doi: 10.1016/j.neuropsychologia.2011.06.015
144. Haglund MM, Ojemann GA, Schwartz TW, Lettich E. Neuronal activity in human lateral temporal cortex during serial retrieval from short-term memory. J Neurosci. (1994) 14:1507–15. doi: 10.1523/JNEUROSCI.14-03-01507.1994
145. Coleshill SG, Binnie CD, Morris RG, Alarcón G, van Emde Boas W, Velis DN, et al. Material-specific recognition memory deficits elicited by unilateral hippocampal electrical stimulation. J Neurosci. (2004) 24:1612–6. doi: 10.1523/JNEUROSCI.4352-03.2004
146. Ezzyat Y, Wanda PA, Levy DF, Kadel A, Aka A, Pedisich I, et al. Closed-loop stimulation of temporal cortex rescues functional networks and improves memory. Nat Commun. (2018) 9:365. doi: 10.1038/s41467-017-02753-0
147. Bonini F, Burle B, Liégeois-Chauvel C, Régis J, Chauvel P, Vidal F. Action monitoring and medial frontal cortex: leading role of supplementary motor area. Science. (2014) 343:888–91. doi: 10.1126/science.1247412
148. Puglisi G, Sciortino T, Rossi M, Leonetti A, Fornia L, Conti Nibali M, et al. Preserving executive functions in nondominant frontal lobe glioma surgery: an intraoperative tool. J Neurosurg. (2018) 131:474–80. doi: 10.3171/2018.4.JNS18393
149. Wellmer J, Weber C, Mende M, von der Groeben F, Urbach H, Clusmann H, et al. Multitask electrical stimulation for cortical language mapping: hints for necessity and economic mode of application. Epilepsia. (2009) 50:2267–75. doi: 10.1111/j.1528-1167.2009.02192.x
150. Binder JR, Desai RH, Graves WW, Conant LL. Where is the semantic system? A critical review and meta-analysis of 120 functional neuroimaging studies. Cereb Cortex. (2009) 19:2767–96. doi: 10.1093/cercor/bhp055
151. Hickok G, Poeppel D. Towards a new functional anatomy of language. Cognition. (2004) 92:1–12. doi: 10.1016/j.cognition.2003.11.001
152. Binder JR, Tong JQ, Pillay SB, Conant LL, Humphries CJ, Raghavan M, et al. Anterior temporal lobe regions essential for picture naming: a voxel-level correlation analysis in left language dominant temporal lobe epilepsy surgery. Epilepsia. (2020) 61:1939–48. doi: 10.1111/epi.16643
153. Damasio AR. Time-locked multiregional retroactivation: a systems-level proposal for the neural substrates of recall and recognition. Cognition. (1989) 33:25–62. doi: 10.1016/0010-0277(89)90005-X
154. Damasio H, Grabowski TJ, Tranel D, Hichwa RD, Damasio A. A neural basis for lexical retrieval. Nature. (1996) 380:499–505. doi: 10.1038/380499a0
155. Drane DL, Ojemann JG, Phatak V, Loring DW, Gross RE, Hebb AO, et al. Famous face identification in temporal lobe epilepsy: Support for a multimodal integration model of semantic memory. Cortex. (2013) 49:1648–67. doi: 10.1016/j.cortex.2012.08.009
156. Hickok G, Poeppel D. Dorsal and ventral streams: a framework for understanding the functional neuroanatomy of language. Cognition. (2004) 92:67–99. doi: 10.1016/j.cognition.2003.10.011
157. Tranel D, Damasio H, Eichhorn GR, Grabowski T, Ponto LL, Hichwa RD. Neural correlates of naming animals from their characteristic sounds. Neuropsychologia. (2003) 41:847–54. doi: 10.1016/S0028-3932(02)00223-3
158. Drane DL, Ojemann GA, Aylward EH, Ojemann JG, Johnson LC, Silbergeld DL, et al. Category-specific naming and recognition deficits in temporal lobe epilepsy surgical patients. Neuropsychologia. (2008) 46:1242–55. doi: 10.1016/j.neuropsychologia.2007.11.034
159. Gomez J, Pestilli F, Witthoft N, Golarai G, Liberman A, Poltoratski S, et al. Functionally defined white matter reveals segregated pathways in human ventral temporal cortex associated with category-specific processing. Neuron. (2015) 85:216–27. doi: 10.1016/j.neuron.2014.12.027
160. Tranel D. The left temporal pole is important for retrieving words for unique concrete entities. Aphasiology. (2009) 23:867. doi: 10.1080/02687030802586498
161. Hamberger MJ, Miozzo M, Schevon CA, Morrison C, Carlson C, Mehta AD, et al. Functional differences among stimulation-identified cortical naming sites in the temporal region. Epilepsy Behav. (2016) 60:124–9. doi: 10.1016/j.yebeh.2016.04.021
162. Cohen L, Dehaene S, Naccache L, Lehéricy S, Dehaene-Lambertz G, Hénaff MA, et al. The visual word form area: spatial and temporal characterization of an initial stage of reading in normal subjects and posterior split-brain patients. Brain. (2000) 123(Pt 2):291–307. doi: 10.1093/brain/123.2.291
163. Henry C, Gaillard R, Volle E, Chiras J, Ferrieux S, Dehaene S, et al. Brain activations during letter-by-letter reading: a follow-up study. Neuropsychologia. (2005) 43:1983–9. doi: 10.1016/j.neuropsychologia.2005.04.007
164. Binder JR. Current controversies on Wernicke's area and its role in language. Curr Neurol Neurosci Rep. (2017) 17:58. doi: 10.1007/s11910-017-0764-8
165. Perrine K. Future directions for functional mapping. Epilepsia. (1994) 35:S90–102. doi: 10.1111/j.1528-1157.1994.tb05991.x
166. Mateer C, Polen S, Ojemann GA. Sexual variation in cortical localization of naming as determined by stimulation mapping. Commentary on McGlone 1980. Behav Brain Sci. (1982) 5:310–1. doi: 10.1017/S0140525X00012188
167. Devinsky O, Perrine K, Hirsch J, McMullen W, Pacia S, Doyle W. Relation of cortical language distribution and cognitive function in surgical epilepsy patients. Epilepsia. (2000) 41:400–4. doi: 10.1111/j.1528-1157.2000.tb00180.x
168. Schwartz TH, Devinsky O, Doyle W, Perrine K. Preoperative predictors of anterior temporal language areas. J Neurosurg. (1998) 89:962–70. doi: 10.3171/jns.1998.89.6.0962
169. Geschwind N. The organization of language. Science. (1979) 170:940–4. doi: 10.1126/science.170.3961.940
170. Indefrey P. The spatial and temporal signatures of word production components: a critical update. Front Psychol. (2011) 2:255. doi: 10.3389/fpsyg.2011.00255
171. Fedorenko E, Thompson-Schill SL. Reworking the language network. Trends Cogn Sci. (2014) 18:120–6. doi: 10.1016/j.tics.2013.12.006
172. Tyler LK, Stamatakis EA, Bright P, Acres K, Abdallah S, Rodd JM, et al. Processing objects at different levels of specificity. J Cogn Neurosci. (2004) 16:351–62. doi: 10.1162/089892904322926692
173. Roth EC, Hellige JB. Spatial processing and hemispheric asymmetry. Contributions of the transient/magnocellular visual system. J Cogn Neurosci. (1998) 10:472–84. doi: 10.1162/089892998562889
174. Takamiya N, Maekawa T, Yamasaki T, Ogata K, Yamada E, Tanaka M, et al. Different hemispheric specialization for face/word recognition: a high-density ERP study with hemifield visual stimulation. Brain Behav. (2020) 10:e01649. doi: 10.1002/brb3.1649
175. Levine SC, Banich MT, Koch-Weser MP. Face recognition: a general or specific right hemisphere capacity? Brain Cogn. (1988) 8:303–25. doi: 10.1016/0278-2626(88)90057-7
176. Jonas J, Descoins M, Koessler L, Colnat-Coulbois S, Sauvée M, Guye M, et al. Focal electrical intracerebral stimulation of a face-sensitive area causes transient prosopagnosia. Neuroscience. (2012) 222:281–8 doi: 10.1016/j.neuroscience.2012.07.021
177. Jonas J, Rossion B, Krieg J, Koessler L, Colnat-Coulbois S, Vespignani H, et al. Intracerebral electrical stimulation of a face-selective area in the right inferior occipital cortex impairs individual face discrimination. Neuroimage. (2014) 99:487–97. doi: 10.1016/j.neuroimage.2014.06.017
178. Jonas J, Jacques C, Liu-Shuang J, Brissart H, Colnat-Coulbois S, Maillard L, et al. A face-selective ventral occipito-temporal map of the human brain with intracerebral potentials. Proc Natl Acad Sci USA. (2016) 113:E4088–97. doi: 10.1073/pnas.1522033113
179. Burgess N, Maguire EA, O'Keefe J. The human hippocampus and spatial and episodic memory. Neuron. (2002) 35:625–41. doi: 10.1016/S0896-6273(02)00830-9
180. Karnath H-O, Rorden C. The anatomy of spatial neglect. Neuropsychologia. (2012) 50:1010–7. doi: 10.1016/j.neuropsychologia.2011.06.027
181. Corballis MC. Mental rotation and the right hemisphere. Brain Lang. (1997) 57:100–21. doi: 10.1006/brln.1997.1835
182. Parsons TD, Larson P, Kratz K, Thiebaux M, Bluestein B, Buckwalter JG, et al. Sex differences in mental rotation and spatial rotation in a virtual environment. Neuropsychologia. (2004) 42:555–62. doi: 10.1016/j.neuropsychologia.2003.08.014
183. Hawes Z, Sokolowski HM, Ononye CB, Ansari D. Neural underpinnings of numerical and spatial cognition: an fMRI meta-analysis of brain regions associated with symbolic number, arithmetic, mental rotation. Neurosci Biobehav Rev. (2019) 103:316–36. doi: 10.1016/j.neubiorev.2019.05.007
184. Fried I, Mateer C, Ojemann GA, Wohns R, Fedio P. Organization of visuospatial functions in human cortex: evidence from electrical stimulation. Brain. (1982) 105:349–71. doi: 10.1093/brain/105.2.349
185. Kahana MJ, Sekuler R, Caplan JB, Kirschen M, Madsen JR. Human theta oscillations exhibit task dependence during virtual maze navigation. Nature. (1999) 399:781–4. doi: 10.1038/21645
186. Chen D, Kunz L, Wang W, Zhang H, Wang W-X, Schulze-Bonhage A, et al. Hexadirectional modulation of theta power in human entorhinal cortex during spatial navigation. Curr Biol. (2018) 28:3310–4. doi: 10.1016/j.cub.2018.08.029
187. Ekstrom AD, Caplan JB, Ho E, Shattuck K, Fried I, Kahana MJ. Human hippocampal theta activity during virtual navigation. Hippocampus. (2005) 15:881–9. doi: 10.1002/hipo.20109
188. Aghajan Z, Schuette P, Fields TA, Tran ME, Siddiqui SM, Hasulak NR, et al. Theta oscillations in the human medial temporal lobe during real-world ambulatory movement. Curr Biol. (2017) 27:3743–51.e3. doi: 10.1016/j.cub.2017.10.062
189. Miller J, Watrous AJ, Tsitsiklis M, Lee SA, Sheth SA, Schevon CA, et al. Lateralized hippocampal oscillations underlie distinct aspects of human spatial memory and navigation. Nat Commun. (2018) 9:2423. doi: 10.1038/s41467-018-04847-9
190. Goyal A, Miller J, Qasim SE, Watrous AJ, Zhang H, Stein JM, et al. Functionally distinct high and low theta oscillations in the human hippocampus. Nat Commun. (2020) 11:2469. doi: 10.1038/s41467-020-15670-6
191. Jacobs J. Hippocampal theta oscillations are slower in humans than in rodents: implications for models of spatial navigation and memory. Philos Trans R Soc B Biol Sci. (2014) 369:20130304. doi: 10.1098/rstb.2013.0304
192. Mormann F, Osterhage H, Andrzejak RG, Weber B, Fernández G, Fell J, et al. Independent delta/theta rhythms in the human hippocampus and entorhinal cortex. Front Hum Neurosci. (2008) 2:3. doi: 10.3389/neuro.09.003.2008
193. Koziol LF, Budding DE, editors. Subcortical Structures and Cognition: Implications for Neuropsychological Assessment. Newark, NJ: Springer (2009).
194. Henri-Bhargava A, Stuss DT, Freedman M. Clinical assessment of prefrontal lobe functions. Continuum. (2018) 24:704–26. doi: 10.1212/CON.0000000000000609
195. Baxendale S, Thompson PJ, Sander JW. Neuropsychological outcomes in epilepsy surgery patients with unilateral hippocampal sclerosis and good preoperative memory function. Epilepsia. (2013) 54:e131–4. doi: 10.1111/epi.12319
196. Gleissner U, Helmstaedter C, Schramm J, Elger CE. Memory outcome after selective amygdalohippocampectomy in patients with temporal lobe epilepsy: one-year follow-up. Epilepsia. (2004) 45:960–2. doi: 10.1111/j.0013-9580.2004.42203.x
197. Helmstaedter C, Petzold I, Bien CG. The cognitive consequence of resecting nonlesional tissues in epilepsy surgery–results from MRI- and histopathology-negative patients with temporal lobe epilepsy. Epilepsia. (2011) 52:1402–8. doi: 10.1111/j.1528-1167.2011.03157.x
198. Seidenberg M, Hermann B, Wyler AR, Davies K, Dohan FC Jr, Leveroni C. Neuropsychological outcome following anterior temporal lobectomy in patients with and without the syndrome of mesial temporal lobe epilepsy. Neuropsychology. (1998) 12:303–16. doi: 10.1037/0894-4105.12.2.303
199. Gross RE, Stern MA, Willie JT, Fasano RE, Saindane AM, Soares BP, et al. Stereotactic laser amygdalohippocampotomy for mesial temporal lobe epilepsy. Ann Neurol. (2018) 83:575–87. doi: 10.1002/ana.25180
200. Cairney SA, Guttesen A, El Marj N, Staresina BP. Memory consolidation is linked to spindle-mediated information processing during sleep. Curr Biol. (2018) 28:948–54. doi: 10.1016/j.cub.2018.01.087
201. Jiang X, Gonzalez-Martinez J, Halgren E. Coordination of human hippocampal sharpwave ripples during NREM sleep with cortical theta bursts, spindles, downstates, and upstates. J Neurosci. (2019) 39:8744–61. doi: 10.1523/JNEUROSCI.2857-18.2019
202. Lambert I, Tramoni-Negre E, Lagarde S, Roehri N, Giusiano B, Trebuchon-Da Fonseca A, et al. Hippocampal interictal spikes during sleep impact long-term memory consolidation. Ann Neurol. (2020) 87:976–87. doi: 10.1002/ana.25744
203. Drane DL. Neuropsychological evaluation of the epilepsy surgical candidate. In: Barr WB, Morrison C, editors. Handbook on the Neuropsychology of Epilepsy. New York, NY: Springer (2015) 87–121.
204. Chari A, Thornton RC, Tisdall MM, Scott RC. Microelectrode recordings in human epilepsy: a case for clinical translation. Brain Commun. (2020) 2:fcaa082. doi: 10.1093/braincomms/fcaa082
205. Quian Quiroga R. Plugging in to human memory: advantages, challenges, and insights from human single-neuron recordings. Cell. (2019) 179:1015–32. doi: 10.1016/j.cell.2019.10.016
206. Coubes P, Baldy-Moulinier M, Zanca M, Boire JY, Child R, Bourbotte G, et al. Monitoring sodium methohexital distribution with [99mTc]HMPAO with single photon emission computed tomography during Wada test. Epilepsia. (1995) 36:1041–9. doi: 10.1111/j.1528-1157.1995.tb00964.x
207. Herbet G, Moritz-Gasser S. Beyond language: mapping cognition and emotion. Neurosurg Clin. (2019) 30:75–83. doi: 10.1016/j.nec.2018.08.004
208. Ameis SH, Catani M. Altered white matter connectivity as a neural substrate for social impairment in Autism Spectrum Disorder. Cortex. (2015) 62:158–81. doi: 10.1016/j.cortex.2014.10.014
209. Savla GN, Vella L, Armstrong CC, Penn DL, Twamley EW. Deficits in domains of social cognition in schizophrenia: a meta-analysis of the empirical evidence. Schizophrenia Bull. (2013) 39:979–92. doi: 10.1093/schbul/sbs080
210. Blair RJ. The amygdala and ventromedial prefrontal cortex in morality and psychopathy. Trends Cogn Sci. (2007) 11:387–92. doi: 10.1016/j.tics.2007.07.003
211. Li W, Mai X, Liu C. The default mode network and social understanding of others: what do brain connectivity studies tell us. Front Hum Neurosci. (2014) 8:74. doi: 10.3389/fnhum.2014.00074
212. Raichle ME, MacLeod AM, Snyder AZ, Powers WJ, Gusnard DA, Shulman GL. A default mode of brain function. Proc Natl Acad Sci USA. (2001) 98:676–82. doi: 10.1073/pnas.98.2.676
213. Raichle ME, Snyder AZ. A default mode of brain function: a brief history of an evolving idea. NeuroImage. (2007) 37:1083–99. doi: 10.1016/j.neuroimage.2007.02.041
214. Schilbach L, Eickhoff SB, Rotarska-Jagiela A, Fink GR, Vogeley K. Minds at rest? Social cognition as the default mode of cognizing and its putative relationship to the “default system” of the brain. Consciou Cogn. (2008) 17:457–67. doi: 10.1016/j.concog.2008.03.013
215. Martin E, Jeanmonod D, Morel A, Zadicario E, Werner B. High-intensity focused ultrasound for noninvasive functional neurosurgery. Ann Neurol. (2009) 66:858–61. doi: 10.1002/ana.21801
216. Buckner RL, Andrews-Hanna JR, Schacter DL. The brain's default network: anatomy, function, and relevance to disease. Ann NY Acad Sci. (2008) 1124:1–38. doi: 10.1196/annals.1440.011
217. Aggleton JP, Passingham RE. Syndrome produced by lesions of the amygdala in monkeys (Macacamulatta). J Comp Physiol Psychol. (1981) 95:961–77. doi: 10.1037/h0077848
218. Brown S, Schäfer EA. An investigation into the functions of the occipital and temporal lobes of the monkey's brain. Philos Trans R Soc Lond. (1888) 179B:303–27. doi: 10.1098/rstb.1888.0011
219. Klüver H, Bucy PC. “Psychic blindness” and other symptoms following bilateral temporal lobectomy in Rhesus monkeys. Am J Physiol. (1937) 119:352–3.
220. Klüver H, Bucy PC. Preliminary analysis of functions of the temporal lobes in monkeys. Arch Neurol Psychiatry. (1939) 42:979–1000. doi: 10.1001/archneurpsyc.1939.02270240017001
221. Marlowe WB, Mancall EL, Thomas JJ. Complete Klüver-Bucy syndrome in man. Cortex. (1975) 11:53–9. doi: 10.1016/S0010-9452(75)80020-7
222. Cox CL, Uddin LQ, Di Martino A, Castellanos FX, Milham MP, Kelly C. The balance between feeling and knowing: affective and cognitive empathy are reflected in the brain's intrinsic functional dynamics. Soc Cogn Affect Neurosci. (2012) 7:727–37. doi: 10.1093/scan/nsr051
223. Molnar-Szakacs I, Uddin LQ. Self-processing and the default mode networks: interactions with the mirror neuron system. Front Human Neurosci. (2013) 7:571. doi: 10.3389/fnhum.2013.00571
224. Catani M, Dell'acqua F, Thiebaut de Schotten M. A revised limbic system model for memory, emotion and behaviour. Neurosci Biobehav Rev. (2013) 37:1724–37. doi: 10.1016/j.neubiorev.2013.07.001
225. Papez JW. A proposed mechanism of emotion. Arch Neurol Psychiatry. (1937) 38:725–43. doi: 10.1001/archneurpsyc.1937.02260220069003
226. Yakovlev PJ. Motility, behavior and the brain; stereodynamic organization and neural coordinates of behavior. J Nervous Mental Dis. (1948) 107:313–35. doi: 10.1097/00005053-194810740-00001
227. MacLean PD. Psychosomatic disease and the ‘visceral brain’: recent developments bearing on the Papez theory of emotion. Psychosom Med. (1949) 11:338–53. doi: 10.1097/00006842-194911000-00003
228. MacLean PD. Some psychiatric implications of physiological studies on frontotemporal portion of limbic system (visceral brain). Electroencephalogr Clin Neurophysiol. (1952) 4:407–18. doi: 10.1016/0013-4694(52)90073-4
229. Baron-Cohen S, Wheelwright S, Hill J, Raste Y, Plumb I. The “Reading the Mind in the Eyes” Test revised version: a study with normal adults, and adults with Asperger syndrome or high-functioning autism. J Child Psychol Psychiatry Allied Discipl. (2001) 42:241–51. doi: 10.1111/1469-7610.00715
230. Döhnel K, Schuwerk T, Meinhardt J, Sodian B, Hajak G, Sommer M. Functional activity of the right temporo-parietal junction and of the medial prefrontal cortex associated with true and false belief reasoning. NeuroImage. (2012) 60:1652-61. doi: 10.1016/j.neuroimage.2012.01.073
231. Herbet G, Lafargue G, Bonnetblanc F, Moritz-Gasser S, Duffau H. Is the right frontal cortex really crucial in the mentalizing network? A longitudinal study in patients with a slow-growing lesion. Cortex. (2013) 49:2711–27. doi: 10.1016/j.cortex.2013.08.003
232. Herbet G, Lafargue G, Bonnetblanc F, Moritz-Gasser S, Menjot de Champfleur N, Duffau H. Inferring a dual-stream model of mentalizing from associative white matter fibres disconnection. Brain. (2014) 137:944–59. doi: 10.1093/brain/awt370
233. Ono KE, Bearden DJ, Adams E, Doescher J, Koh S, Eksioglu Y, et al. Cognitive and behavioral outcome of stereotactic laser amydalohippocampotomy in a pediatric setting. Epilepsy Behav Rep. (2020) 14:100370. doi: 10.1016/j.ebr.2020.100370
234. Hofman MA, Falk D, editors. Progress in Brain Research, Vol. 195. Amsterdam: Elsevier (2012). p. 123–63.
235. Revonsuo A. Inner Presence: Consciousness as Biological Phenomenon. Cambridge, MA: MIT Press (2006).
236. Giacino JT, Fins JJ, Laureys S, Schiff ND. Disorders of consciousness after acquired brain injury: the state of the science. Nat Rev Neurol. (2014) 10:99–114. doi: 10.1038/nrneurol.2013.279
239. Arthuis M, Valton L, Regis J, Chauvel P, Wendling F, Naccache L, et al. Impaired consciousness during temporal lobe seizures is related to increased long-distance cortical-subcortical synchronization. Brain. (2009) 132:2091–101. doi: 10.1093/brain/awp086
240. Gaillard R, Dehaene S, Adam C, Clemenceau S, Hasboun D, Baulac M, et al. Converging intracranial markers of conscious access. PLoS Biol. (2009) 7:e61. doi: 10.1371/journal.pbio.1000061
241. Naccache L, Marti S, Sitt JD, Trübutschek D, Berkovitch L. Why the P3b is still a plausible correlate of conscious access? A commentary on Silverstein et al., 2015. Cortex. (2016) 85:126–8. doi: 10.1016/j.cortex.2016.04.003
242. Naccache L. Why and how access consciousness can account for phenomenal consciousness. Philos Trans R Soc Lond B Biol Sci. (2018) 373:20170357 doi: 10.1098/rstb/2017.0357
243. Bartolomei F, Naccache L. The global workspace (GW) theory of consciousness and epilepsy. Behav Neurol. (2011) 24:67–74. doi: 10.3233/BEN-2011-0313
244. Bartolomei F, McGonigal A, Naccache L. Alteration of global workspace in focal epilepsy: the global workspace alteration theory. Epilepsy Behav. (2014) 30:17–23. doi: 10.1016/j.yebeh.2013.09.012
245. Bonini F, Lambert I, Wendling F, McGonigal A, Bartolomei F. Altered synchrony and loss of consciousness during frontal lobe seizures. Clin Neurophysiol. (2016) 127:1170–5. doi: 10.1016/j.clinph.2015.04.050
246. Lambert I, Arthius M, McGonigal A, Wendling F, Bartolomei F. Alteration of global workspace during loss of consciousness: a study of parietal seizures. Epilepsia. (2012) 53:2014–110. doi: 10.1111/j.1528-1167.2012.03690.x
247. Lagun D, Manzanares C, Zola SM, Buffalo EA, Agichtein E. Detecting cognitive impairment by eye movement analysis using automatic classification algorithms. J Neurosci Methods. (2011) 201:196–203. doi: 10.1016/j.jneumeth.2011.06.027
248. Zola SM, Manzanares CM, Clopton P, Lah JJ, Levey AI. A behavioral task predicts conversion to mild cognitive impairment and Alzheimer's disease. Am J Alzheimer's Dis Other Dement. (2013) 28:179–84. doi: 10.1177/1533317512470484
249. Aronov D, Tank DW. Engagement of neural circuits underlying 2D spatial navigation in a rodent virtual reality system. Neuron. (2014) 84:442–56. doi: 10.1016/j.neuron.2014.08.042
250. Lester AW, Moffat SD, Wiener JM, Barnes CA, Wolbers T. The aging navigational system. Neuron. (2017) 95:1019–35. doi: 10.1016/j.neuron.2017.06.037
251. Bernard F, Lemée JM, Aubin G, Ter Minassian A, Menei P. Using a virtual reality social network during awake craniotomy to map social cognition: prospective trial. J Med Internet Res. (2018) 20:e10332. doi: 10.2196/10332
252. Corriveau Lecavalier N, Ouellet É, Boller B, Belleville S. Use of immersive virtual reality to assess episodic memory: a validation study in older adults. Neuropsychol Rehabil. (2020) 30:462–80. doi: 10.1080/09602011.2018.1477684
253. Ouellet É, Boller B, Corriveau-Lecavalier N, Cloutier S, Belleville S. The virtual shop: a new immersive virtual reality environment and scenario for the assessment of everyday memory. J Neurosci Methods. (2018) 303:126–35. doi: 10.1016/j.jneumeth.2018.03.010
254. da Costa R, Pompeu JE, de Mello DD, Moretto E, Rodrigues FZ, Dos Santos MD, et al. Two new virtual reality tasks for the assessment of spatial orientation: preliminary results of tolerability, sense of presence and usability. Dement Neuropsychol. (2018) 12:196–204. doi: 10.1590/1980-57642018dn12-020013
255. Climent G, Rodríguez C, García T, Areces D, Mejías M, Aierbe A, et al. New virtual reality tool (Nesplora Aquarium) for assessing attention and working memory in adults: a normative study. Appl Neuropsychol Adult. (2019) 1–13. doi: 10.1080/23279095.2019.1646745
256. König SU, Clay V, Nolte D, Duesberg L, Kuske N, König P. Learning of spatial properties of a large-scale virtual city with an interactive map. Front Hum Neurosci. (2019) 13:240. doi: 10.3389/fnhum.2019.00240
257. Peeters D. A standardized set of 3-D objects for virtual reality research and applications. Behav Res Methods. (2018) 50:1047–54. doi: 10.3758/s13428-017-0925-3
258. Cleary AM, Brown AS, Sawyer BD, Nomi JS, Ajoku AC, Ryals AJ. Familiarity from the configuration of objects in 3-dimensional space and its relation to déjà vu: a virtual reality investigation. Conscious Cogn. (2012) 21:969–75. doi: 10.1016/j.concog.2011.12.010
259. Caldwell DJ, Ojemann JG, Rao R. Direct electrical stimulation in electrocorticographic brain-computer interfaces: enabling technologies for input to cortex. Front Neurosci. (2019) 13:804. doi: 10.3389/fnins.2019.00804
Keywords: stimulation mapping, language, passive mapping, cerebral cortex, connectivity, socioemotional, memory, SEEG
Citation: Drane DL, Pedersen NP, Sabsevitz DS, Block C, Dickey AS, Alwaki A and Kheder A (2021) Cognitive and Emotional Mapping With SEEG. Front. Neurol. 12:627981. doi: 10.3389/fneur.2021.627981
Received: 10 November 2020; Accepted: 04 March 2021;
Published: 12 April 2021.
Edited by:
Patrick Chauvel, University of Pittsburgh Medical Center, United StatesReviewed by:
Liankun Ren, Capital Medical University, ChinaSilvia Kochen, Hospital EL Cruce, Argentina
Aileen McGonigal, Aix-Marseille Université, France
Louis Maillard, Université de Lorraine, France
Copyright © 2021 Drane, Pedersen, Sabsevitz, Block, Dickey, Alwaki and Kheder. This is an open-access article distributed under the terms of the Creative Commons Attribution License (CC BY). The use, distribution or reproduction in other forums is permitted, provided the original author(s) and the copyright owner(s) are credited and that the original publication in this journal is cited, in accordance with accepted academic practice. No use, distribution or reproduction is permitted which does not comply with these terms.
*Correspondence: Daniel L. Drane, ZGRyYW5lJiN4MDAwNDA7ZW1vcnkuZWR1; Nigel P. Pedersen, bnBlZGVycyYjeDAwMDQwO2Vtb3J5LmVkdQ==