- 1Department of Molecular, Cellular, & Developmental Biology, University of Michigan, Ann Arbor, MI, United States
- 2Department of Ophthalmology & Visual Sciences, University of Michigan, Ann Arbor, MI, United States
- 3Department of Psychology, BIO5 Research Institute, University of Arizona, Tucson, AZ, United States
- 4Department of Neurology, McKnight Brain Research Institute, University of Arizona, Tucson, AZ, United States
A growing number of studies document circadian phase-shifting after exposure to millisecond light flashes. When strung together by intervening periods of darkness, these stimuli evoke pacemaker responses rivaling or outmatching those created by steady luminance, suggesting that the circadian system's relationship to light can be contextualized outside the principle of simple dose-dependence. In the current review, we present a brief chronology of this work. We then develop a conceptual model around it that attempts to relate the circadian effects of flashes to a natural integrative process the pacemaker uses to intermittently sample the photic information available at dawn and dusk. Presumably, these snapshots are employed as building blocks in the construction of a coherent representation of twilight the pacemaker consults to orient the next day's physiology (in that way, flash-resetting of pacemaker rhythms might be less an example of a circadian visual illusion and more an example of the kinds of gestalt inferences that the image-forming system routinely makes when identifying objects within the visual field; i.e., closure). We conclude our review with a discussion on the role of cones in the pacemaker's twilight predictions, providing new electrophysiological data suggesting that classical photoreceptors—but not melanopsin—are necessary for millisecond, intermediate-intensity flash responses in ipRGCs (intrinsically photosensitive retinal ganglion cells). Future investigations are necessary to confirm this “Cone Sentinel Model” of circadian flash-integration and twilight-prediction, and to further define the contribution of cones vs. rods in transducing pacemaker flash signals.
Introduction
The retina integrates light signals detected across a tripartite network of photoreceptors to convey time-of-day information related to the Earth's rotation and solar cycle directly to the brain's circadian pacemaker, the suprachiasmatic nucleus (SCN) (1–5). Grounded within the crossroads of this light-detection system is a subset of intrinsically photosensitive retinal ganglion cells (ipRGCs) containing the vitamin A-based photopigment, melanopsin (6–14). ipRGCs are recurrently configured in the eye. By virtue of melanopsin expression, these cells are themselves photo-excitable and can operate as independent relays to the SCN, but—nevertheless—also receive synaptic connections from rods and cones (15–24), and participate in a centrifugal feedback pathway involving several types of retinal amacrine interneurons (25, 26). As an emergent unit, the whole of the retinal circuitry that comprises the (non-image forming) input to the SCN is extremely flexible in its reading of ambient light across various intensities, spectra, and patterns of contrast. While it is appreciated that all photoreceptor classes make contributions to irradiance detection and are activated across an overlapping range of wavelengths (27–31), there is particular specialization with regards to each's role in signaling contact with steady (i.e., non-flickering) vs. intermittent light.
For example, ipRGCs provide a sustained signal to the SCN throughout the duration of exposure to a discrete light stimulus lasting up to several hours (32–38); cones, on the other hand, amplify signaling only at the beginning of the exposure owing to their transient response within the first <1 s of light-onset (39–44). This functional dissociation is evident in electrophysiological retinal recordings of ipRGCs (8, 17, 19), single-unit recordings of SCN neurons (33, 41), as well as behavioral comparisons between rodents with selective loss of cones vs. wildtype animals. In the latter case, cone-deficient mice exhibit full-magnitude phase shifts to 15-min but not 1-min light administration (480 nm) (40). Conversely, cone-activating light fails to phase-shift the rodent pacemaker as a continuous 15-min stimulus but does so when presented intermittently along 15 separated 1-min steps over an hour (42). These aggregated data suggest a wider truth about the circadian pacemaker's timekeeping estimates. They are based on two superimposed changes in incident light: (1) the slow intensity variation of sunlight that marks the day's movement through the morning and afternoon and that which separates the day from the night (~10–12 h; weighted toward melanopsin function); and (2) the higher-frequency changes in irradiance and spectrum that punctuate twilight interludes at dawn/dusk (~30–60 min; weighted toward cone function).
The pacemaker's phase responses to the same light stimulus (e.g., a 15-min pulse) change systematically across the subjective night along a sigmoidal-like wave (45). In the vast majority of species that have been surveyed, light administration in the first half of the night will produce phase delays in behavioral-physiological rhythms commensurate with the difference in timing between the photic stimulation and the timing of dusk in the solar cycle or lights-out within an indoor light-dark schedule [e.g., in humans, lab rodents and Drosophila, introduction of the light stimulus 2 h after subjective sunset will delay rhythms by up to 2 h; (46–48)]. The reciprocal is observed in the second half of the night, where light administration will advance rhythms in proportion to how much earlier the light was seen with respect to expected sunrise [e.g., stimulation 2 h before sunrise or lights-on will fast-forward the onset of diurnal physiology and behavior by up to 2 h; (46–48)]. When describing the circadian pacemaker's phase response curve (PRC) to light, many commentators note the technical shape of the PRC in passing or the relationship it might bear to a biological phenomenon of interest. Few point out the bigger picture: the circadian PRC to light is arguably the most demonstrable example of the brain's prediction coding.
Predictive processing is a mature field of inquiry in psychology and cognitive neuroscience (49–52), where diverse methodologies have established the brain as a prospection device that interprets sensory information with the express purpose of generating expectations—and thereby obtaining a level of preparedness—for the immediately relevant future (49–61). Early studies of prediction coding or “sensory anticipation” were primarily motivated by experiments that attempted to resolve fundamental questions about how the visual field manages to remain stable with the constant image-displacement introduced by physical activity, head and eye movements, and blinking (62, 63). At about the same time as these models of primary vision were conceived, species-generalizable PRCs-to-light had been developed across several experimental organisms occupying different ecological and temporal niches within the biosphere (45, 64, 65). Ironically, study of the non-image forming visual system had produced a wealth of empirical data (not to mention the resounding image of the PRC itself) attesting to the brain's prediction-making capabilities and its raison d'être in reducing the ongoing discrepancies occurring between expectation and actual experience. Yet, it was in the field of perceptual vision research that inference, prediction, and information-seeking became topics of intense scrutiny and now look to embody cutting-edge algorithms for machine vision and artificial intelligence [e.g., (66)].
Organisms were pressured to evolve a circadian timekeeping system that could make predictions about the environment because, ultimately, an inability to do so meant life or death vis-à-vis finding food, staying temperature-regulated, and avoiding predators. While direct responses to light independent of such a timekeeping mechanism (e.g., masking) would effectively restrict animals to a nocturnal or diurnal niche (67), they would not be sufficient for preparing and optimizing vast, interconnected areas of organismal physiology for times-of-day when—for example—food might be most readily available and digested or sleep might be most biologically restorative. Regarding entrainment, we have lost sight of these stakes and the inferences that came along with them—namely, that photodetection mechanisms in the service of the circadian pacemaker are likely to be highly flexible in the light information they use to localize sunset or sunrise. Evolutionary pressure not only coaxed the advent of an entrainable clock but also created a race to the bottom for sunlight detection. Organisms who won-out were able to use the least amount of light information in the service of entrainment and could interpret that information accurately whether it resulted from consistent or erratic contact with sunlight. Successful entrainment did not require a prolonged “sitting” audience with midday or twilight and, for some animals, could be achieved (well-enough) within their natural habitats by a few minutes' exposure once or twice a day (68, 69).
Dynamic Light and the Circadian Pacemaker
Research has established the lower floors of circadian photoentrainment in laboratory models such as Drosophila and mice (70–72). Data suggest that most animals can synchronize and maintain a stable phase relationship to a 12-h light-dark schedule with an irradiance of <1 nW/cm2 or with skeleton photoperiods consisting of ~11-h intervals of darkness bookended by a pair of 30-min light pulses simulating dusk and dawn (70–75). Despite the appreciation that entrainment requires little in the way of photic energy, there is still a lingering assumption among chronobiologists that light-induced phase shifting demands a relatively large energy investment to trigger complete resetting of endogenous rhythmicity. This perspective is best couched by the reciprocity hypothesis, which asserts that the size of any phase-shift is directly proportional to the time-integrated illuminance the pacemaker registers from a light signal (76, 77). However, extant data suggest that light's association with circadian timekeeping is more complicated.
Emission technology in the 20th and early 21st centuries rarely offered control systems equipped to deliver light in a rapid, intermittent, and multidimensional fashion, where all physical exposure variables could be manipulated at once in quick successive steps. Within the technology milieu, however, were movie/photography studio devices that could produce microsecond and millisecond xenon flashes at fixed frequencies. These devices are still used today in order to illuminate and visualize color in dark scenes (e.g., Metz Mecablitz and DynaLite units). Over the past 60 years, several investigators have also used them to examine the phase-shifting effects of flashes in organisms as diverse as Drosophila, hamsters, rats, mice, and humans (78–86). The findings collated from these studies have established that the metazoan circadian pacemaker responds to intermittent millisecond flashes with phase shifts comparable to those that would have been generated with continuous, uninterrupted light administration, provided that the stimuli are delivered at regular intervals every few seconds or each minute. What's more, each circadian hour of the subjective night is equally amenable to flash stimulation; xenon-flash PRCs have been compiled for the eclosion rhythm of Drosophila pseudoobscura (79) and the flight activity of the Schneider's roundleaf bat (Hipposideros speoris) (80, 81), and this patterned stimulation has proven effective in both the delay and advance zones of C57BL/6 mice (82), the most common mouse strain bred in biomedical science.
Employing electrophysiology amplifiers and LED Ganzfeld lamps, researchers have summarized a few other observations germane to the pacemaker's reaction to (sub)millisecond light. First, the energy-efficiency with which flashes phase-shift the clock are maximized by shortening exposure, with optimization accruing all the way down to at least 10 μs (87–89). Ergo, it is likely that the circadian system responds to instantaneous light contact, habituates immediately thereafter, and then cycles through a rapid re-sensitization process. Second, flashes that reset the clock do so with a combinatorial logic that integrates the responses of these flashes with shorter and longer episodes of light (87). This means that flashes do not require delivery in some invariant or artificial sequence (e.g., with a fixed pulse duration, metered along a specific frequency) to impact the circadian system's timekeeping (90, 91). Third, the action spectra for flash resetting of circadian rhythms follows the action spectra that's been documented for visible light. Analogous to broad-spectrum xenon flashes, narrowband blue and green LED flashes can operate as stand-ins for continuous blue/green light exposure (92). Finally, the lower energy bounds for photic induction of circadian resetting reside within the micro-to-nanojoule range (92).
These observations lend support to a model where the pacemaker creates wholistic representations of twilight—thereby predicting the timing of the next day's dawn and dusk—by intermittently sampling bits of photic information that strike the retina as an organism navigates its environment. Presumably, sampling is done in rapid succession by capturing snapshots of incident light, integrating these snapshots across seconds/minutes, and favoring this integration process for the parts of the day when the sun's movement in the sky will invoke the greatest rates of change in ambient illumination intensity and spectral composition (i.e., the 30–60 min of twilight perceived when the sun is ascending or descending the horizon). Under this scenario, the pacemaker's intermittent reading frame is (1) optimized with photic information in its most dynamic state, and (2) withstands stochastic changes in light quantity and quality transiently introduced by clouds, wind and atmospheric turbidity (e.g., light scattering from wind-borne particles, haze), and by the behavior of the organism itself as it moves back-and-forth underground or underneath a discontinuous awning of trees and green vegetation (93).
It is worth noting that this model of circadian photoreception recapitulates an important gestalt principle of the image-forming visual system referred to as closure, which describes the brain's ability to perceive objects as a whole in their completeness even when the objects appear in the visual field lacking one or more constituent parts (94). The image-forming brain is not a stickler for the discrepancies that arise in detecting and identifying figures when they are obstructed, appear at an alternative angle, or when constituent parts may be physically absent. It compensates for the lack of information, interpolates what is missing, makes (mostly) correct deductions about the object or person in front of it, and actively “re-creates” the image of it. Compression algorithms are applied as soon as light contacts the retina, continue their processing as the signal traverses the thalamus and visual cortex, and culminate as the signal breaches the visual streams (95–97). Gestalt principles of visual perception detail how the image-forming system creates structure—and structure within space—by default. Analogous “gestalt” principles might be valuable toward explaining how the pacemaker creates automatic representations of time using twilight as a palette and compression algorithms requiring operation only within the circumscribed circuitry binding the retina and SCN.
Flashes, Cones, and Circadian Predictions About Twilight
Throughout millennia, the pacemaker has been conditioned to track light transitions enveloping sunrise and sunset. The aggregated literature on flash-induction of circadian resetting suggests that this timekeeping mechanism occurs intermittently, using but a quantum of contact with light, to set in motion an integrative process that results in the synthetic construction of a ~60-min episode of twilight from just milliseconds of photic information; integration might occur throughout all stages of signal transduction across the retina and retinohypothalamic tract (RHT), SCN, and SCN outputs. With an engram of this temporarily stored in the SCN circuitry, the representation is then consulted to orient the synchronization of the SCN's output signal to the rest of the brain and periphery, thus phase-locking the next day's physiology and behavior. The literature that has burgeoned from Van den Pol and Heller's original observations (82)—that the circadian pacemaker orients to sequenced intermittent light exposures and not just individual flashes of oversaturating light (78, 80)—was facilitated by co-opting studio equipment but has since been enabled by the advent of semiconductor LEDs. These luminaires provide spectral/temporal photoemission control at the microsecond-level and offer the prospect of engineering dynamic patterns of flash exposure that quickly (and repeatedly) transition from one set of physical-exposure variables to another (98, 99). Despite this technology breakthrough, which is now ushering a fundamental shift in lighting practices around the world, many investigators still regard flash induction of circadian resetting as a lab curiosity—an example of a “circadian visual illusion” worth noting but not necessarily formalizing within studies of circadian photoreception (100). Perhaps what is needed is a better mechanistic characterization of the phenomenon and identification of the relative importance of each photoreceptor class to these types of physiological responses.
Prior to any experiment, one might hypothesize that classic photoreceptor cells would be important conduits for transducing flashes that will feed the pacemaker's twilight predictions. Both rods and cones contribute to RHT responses at light onset and provide short-latency inputs to ipRGCs. If the relative contribution of rods vs. cones were weighed, however, more data than not would suggest that cones are the more relevant photoreceptor class for flash conveyance. Cones with overlapping ranges of wavelength sensitivity: (1) Are the chief contributors to retinal responses driven by short-duration light, generating transient signals that account for most of the RHT activity evoked by a series of brief light pulses (8, 17–19, 33, 40–43); (2) Are disproportionately responsible for the upstream ability of the SCN to track one or more sudden fluctuations in light intensity and spectral contrast (e.g., akin to the salient changes in ambient illumination that characterize dawn and dusk) (33, 42, 44); (3) Differ from melanopsin or rods in that cones cannot drive sustained RHT activity during continuous, uninterrupted light exposure; accordingly, they are neither necessary nor sufficient for photoentrainment of behavioral activity rhythms to recurring solar or electric light-dark cycles (where light is presented in a relatively unwavering fashion for 10–12 straight hours) (27, 29, 33–37, 42); and (4) Are better than melanopsin or rods at adjusting their sensitivity to background illuminance (101–103), thus enabling them to operate as twilight detectors irrespective of how bright or long the photoperiod feeding into dusk. In short, cones are not “circadian-alignment” tools à la melanopsin or rods, which signal the enduring presence of light with fidelity thereby marking the day from the night and providing an estimation of daylength. Rather, they are critical sentinels for detecting the flickering kinds of light that signal the initiation of a sunset or sunrise has migrated to a time later or earlier than anticipated. In this role, they might or might not work together with rods, which already have established roles supporting photoentrainment and quantitative assessments of irradiance alongside melanopsin (10, 11, 27, 29, 42, 104).
Retinally degenerate and knockout mice offer powerful platforms for gauging the relative contribution of each photoreceptor class to a clock light-response of interest. As a proof-of-concept test of our suggestion that classic photoreceptors mediate the circadian effects of millisecond light flashes, one of the authors (KYW) recorded ex vivo ipRGC responses from Opn4Cre/Cre melanopsin-knockout (105) vs. Pde6brd1/rd1rod/cone-degenerate mice. The stimuli were 150-s trains of 2-ms flashes (full-field; 470 nm, 14.0 log photons cm−2 s−1 or roughly 300 lux) with various interstimulus intervals (ISI, 2.5–10 s; Figure 1). These experiments were motivated by previous observations suggesting that: (1) Melanopsin responds better to 20 1-s flashes with certain ISIs than to a continuous 20-s light step, indicating temporal summation (106); and (2) Flashes 50-ms in duration could, occasionally, evoke a melanopsin response in ipRGCs (34), indicating that melanopsin might respond to short as well as prolonged illumination. Given these properties, and the lack of any preexisting data on temporal summation of shorter flashes (i.e., <1 s) by melanopsin, it was not immediately obvious that there would be a clear dichotomy between an ipRGC's outer retinal photoreceptor-driven and melanopsin-mediated responses to millisecond flash stimulation. Such a dichotomy did emerge, however. Upon flash exposure, wildtype mouse ipRGCs with fully-intact rod/cone input showed increases in ipRGC spiking that scaled inversely with ISI (Figure 1B, left panel). Melanopsin-deficient ipRGCs (Opn4Cre/Cre) exhibited similar ISI-dependent patterns of flash response, but ipRGCs in retinas largely devoid of rods and cones (Pde6brd1/rd1) mounted virtually no response (Figure 1B, middle and right panels, respectively). Subsequent head-to-head analysis of wildtype and Opn4Cre/Cre ipRGC light-evoked spiking indicated that the decay in the spike rate over the stimulation window was not statistically different for any of the ISI conditions, suggesting that melanopsin did not contribute to any temporal summation of the 2-ms flashes (Figure 1C).
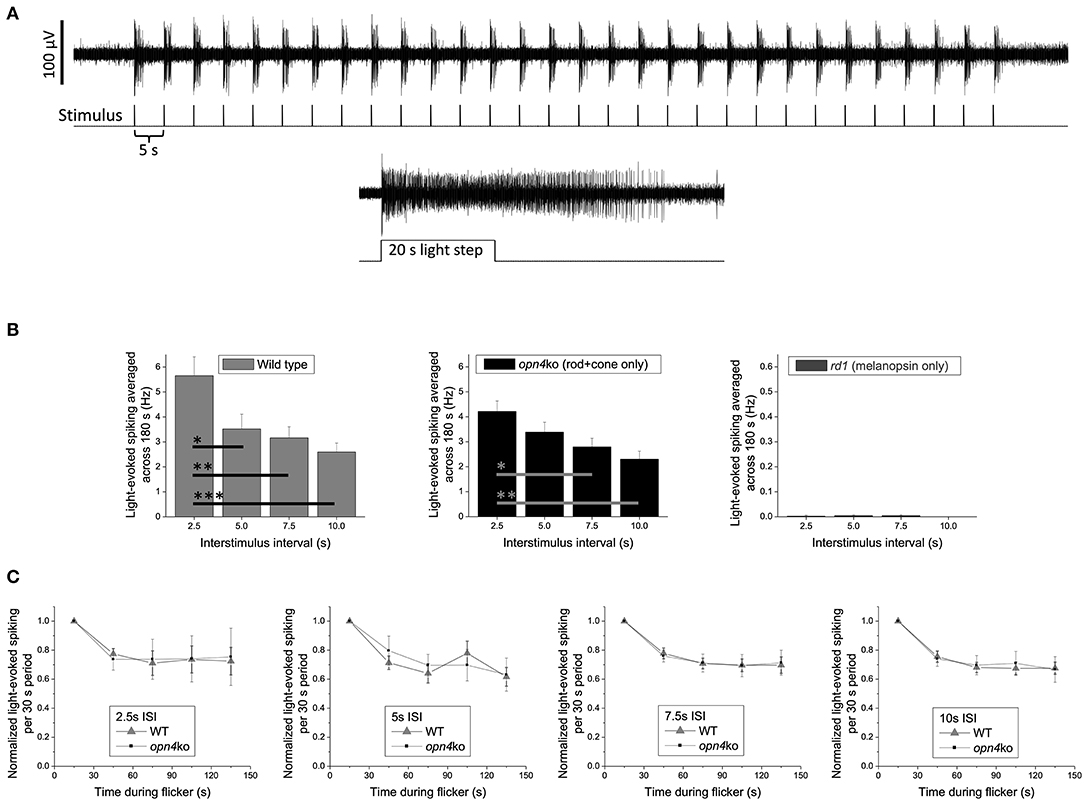
Figure 1. Spiking responses of mouse ipRGCs to a 150 s train of 2 ms flashes (2.5–10 s intervals) are mediated almost exclusively by rod/cone input. (A) Example response of a wild-type ipRGC to 30 flashes with a 5 s interstimulus interval (upper recording). This cell was identified as an ipRGC by its sustained response to a subsequent prolonged light step (lower recording), which evokes transient responses in all non-intrinsically photosensitive RGCs [Wong 2012; (35)]. Spike recording methods were identical to those described in the “Multielectrode-array recording” section in Wong 2012 except that all stimuli, including the 20 s light step, were 14.0 log photons cm−2 s−1 (~300 lux) full-field light produced by an LED with peak emission at 470 nm. (B) The light-induced elevation in spike rate averaged across the flicker plus 30 s of post-flicker darkness (to include responses outlasting the flicker) for C57BL/6 wild-type mice (n = 26 ipRGCs; left plot), Opn4Cre/Cre melanopsin-knockout mice originally created by Ecker et al. (105) (n = 24 ipRGCs; middle plot), and Pde6brd1/rd1 rod/cone-degenerate mice (n = 43 ipRGCs; right plot); note the expanded y-axis in the right plot. All mice in each group were about 7 months old and included both sexes. Though flashes delivered with a 2.5 s interstimulus interval appear to cause a greater spike rate increase in wild-type vs. melanopsin-knockout mice, this difference is not statistically significant (p = 0.156). The wild-type vs. melanopsin-knockout differences for the other three intervals are also statistically insignificant (p-values between 0.689 and 0.818), suggesting that melanopsin does not enhance the flicker responses. Asterisks represent p-values calculated using one-way ANOVA with post-hoc Tukey test: *, p < 0.05; **, p < 0.01; ***, p < 0.001. (C) For all four interstimulus intervals, the gradual decay in light-evoked spike rate during the 150 s flicker is comparable between wild-type and melanopsin-knockout mice, suggesting that melanopsin does not contribute to any temporal summation of successive flash responses. Error bars are S.E.M.
Like all data collected from retinal-degenerate mice, these results need to be interpreted with caution. These models do not allow us to visualize what would occur within an intact system where all photoreceptor classes influence the activity of one another, nor control for the possibility of compensatory reorganization of the circuit loops in which these photoreceptors operate [e.g., (107)]. There is also the caveat that melanopsin might have responded to flashes had they been delivered with higher-intensity stimulation protocols [moderate regimens were tested here, instead, because they are more physiologically relevant for nocturnal rodents and better conform to the flash intensities that have been studied in humans; (91)]. All that said, the clarity of the data and the conclusion they offer remain striking: rods and/or cones enable ipRGC detection and integration of light flashes independent of melanopsin, whose contribution—if any—is predicated on initial processing by the outer retinal photoreceptors. The results are consistent with the suppositions made in the current review and with the idea that cones (with or without the input of rods) will prove to be key regulators of flash-induced resetting of pacemaker rhythms. Future experiments using mice with intact retinal circuitry (e.g., Opn1mwR) or with selective loss in rod vs. cone photosensitivity [e.g., Gnat1−/− and Gnat2cpfl3 mice (108–110)] will be necessary to isolate the relative contributions of cones to this phenomenon and to determine whether the retina processes staccatos of narrowband light with any circadian phase-dependence. Using principles of silent substitution, flash stimuli differing in the amount of cone-and-rod excitation might also be probed for their circadian effects in retinally-intact humans compared to humans with congenital achromatopsia (i.e., people without a functional cone system; prevalence one in 30,000–50,000) (111).
Conclusion
The way we illuminate our world is changing, moving away from unidimensional forms of illumination provided by gas-discharge fluorescent lamps to highly customizable solid-state lighting with semiconductor LEDs. Data suggest that this watershed is material to understanding the circadian pacemaker's phase-responses to electric light exposure, which are likely rooted within a natural process where the pacemaker “flash-samples” the photic information available at twilight to create predictions about the timing of the next day's dusk and dawn. While the mechanisms subserving flash photoreception and the SCN's twilight predictions require further study, it is becoming clear that classical photoreceptors, including cones, operate as sensors in this process. The “Cone Sentinel Model” we articulate here [and hinted at by Zeitzer; (91)] raises many considerations for how different combinations of narrowband LED stimulation can be strung together in phototherapy protocols to improve mental and physical health (112). The gestalt inferences made in this model will be challenging to demonstrate experimentally. However, prudent first steps might include the design of studies examining the differential phase-shifting effects of flashes patterned after twilight progressions—vs. more randomly generated sequences—in parallel to those examining the neurophysiological responses arising from flash regimens highly-optimized (or ill-suited) for driving cone input to ipRGCs.
Data Availability Statement
The original contributions generated for this study are included in the article/supplementary material, further inquiries can be directed to Kwoon Y. Wong, a3dvb25AdW1pY2guZWR1.
Ethics Statement
The animal study was reviewed and approved by the University of Michigan Institutional Animal Care and Use Committee (IACUC).
Author Contributions
F-XF conceptualized the Cone Sentinel model, which was further refined by KYW. KYW developed and carried out the retinal electrophysiology experiments. All authors contributed to writing the manuscript.
Funding
F-XF was supported by Project No. 1360 from the Velux Stiftung (Switzerland). KYW acknowledges support from a Brain Research Foundation Fay/Frank Seed Grant and an Alliance for Vision Research grant.
Conflict of Interest
The authors declare that the research was conducted in the absence of any commercial or financial relationships that could be construed as a potential conflict of interest.
References
1. Lucas RJ, Lall GS, Allen AE, Brown TM. How rod, cone, and melanopsin photoreceptors come together to enlighten the mammalian circadian clock. Prog Brain Res. (2012) 199:1–18. doi: 10.1016/B978-0-444-59427-3.00001-0
2. Freedman MS, Lucas RJ, Soni B, von Schantz M, Muñoz M, David-Gray Z, et al. Regulation of mammalian circadian behavior by non-rod, non-cone, ocular photoreceptors. Science. (1999) 284:502–4. doi: 10.1126/science.284.5413.502
3. Hattar S, Lucas RJ, Mrosovsky N, Thompson S, Douglas RH, Hankins MW, et al. Melanopsin and rod-cone photoreceptive systems account for all major accessory visual functions in mice. Nature. (2003) 424:76–81. doi: 10.1038/nature01761
4. Güler AD, Ecker JL, Lall GS, Haq S, Altimus CM, Liao HW, et al. Melanopsin cells are the principal conduits for rod-cone input to non-image-forming vision. Nature. (2008) 453:102–5. doi: 10.1038/nature06829
5. Güler AD, Altimus CM, Ecker JL, Hattar S. Multiple photoreceptors contribute to nonimage-forming visual functions predominantly through melanopsin-containing retinal ganglion cells. Cold Spring Harb Symp Quant Biol. (2007) 72:509–15. doi: 10.1101/sqb.2007.72.074
6. Fu Y, Zhong H, Wang MH, Luo DG, Liao HW, Maeda H, et al. Intrinsically photosensitive retinal ganglion cells detect light with a vitamin A-based photopigment, melanopsin. Proc Natl Acad Sci USA. (2005) 102:10339–44. doi: 10.1073/pnas.0501866102
7. Provencio I, Jiang G, De Grip WJ, Hayes WP, Rollag MD. Melanopsin: an opsin in melanophores, brain, and eye. Proc Natl Acad Sci USA. (1998) 95:340–5. doi: 10.1073/pnas.95.1.340
8. Berson DM, Dunn FA, Takao M. Phototransduction by retinal ganglion cells that set the circadian clock. Science. (2002) 295:1070–3. doi: 10.1126/science.1067262
9. Gooley JJ, Lu J, Chou TC, Scammell TE, Saper CB. Melanopsin in cells of origin of the retinohypothalamic tract. Nat Neurosci. (2001) 4:1165. doi: 10.1038/nn768
10. Ruby NF, Brennan TJ, Xie X, Cao V, Franken P, Heller HC, et al. Role of melanopsin in circadian responses to light. Science. (2002) 298:2211–3. doi: 10.1126/science.1076701
11. Panda S, Sato TK, Castrucci AM, Rollag MD, DeGrip WJ, Hogenesch JB, et al. Melanopsin (Opn4) requirement for normal light-induced circadian phase shifting. Science. (2002) 298:2213–6. doi: 10.1126/science.1076848
12. Sollars PJ, Smeraski CA, Kaufman JD, Ogilvie MD, Provencio I, Pickard GE. Melanopsin and non-melanopsin expressing retinal ganglion cells innervate the hypothalamic suprachiasmatic nucleus. Vis Neurosci. (2003) 20:601–10. doi: 10.1017/S0952523803206027
13. Hattar S, Kumar M, Park A, Tong P, Tung J, Yau KW, et al. Central projections of melanopsin-expressing retinal ganglion cells in the mouse. J Comp Neurol. (2006) 497:326–49. doi: 10.1002/cne.20970
14. Hannibal J, Fahrenkrug J. Target areas innervated by PACAP-immunoreactive retinal ganglion cells. Cell Tissue Res. (2004) 316:99–113. doi: 10.1007/s00441-004-0858-x
15. Zhao X, Stafford BK, Godin AL, King WM, Wong KY. Photoresponse diversity among the five types of intrinsically photosensitive retinal ganglion cells. J Physiol. (2014) 592:1619–36. doi: 10.1113/jphysiol.2013.262782
16. Ekesten B, Gouras P. Cone and rod inputs to murine retinal ganglion cells: evidence of cone opsin specific channels. Vis Neurosci. (2005) 22:893–903. doi: 10.1017/S0952523805226172
17. Dacey DM, Liao HW, Peterson BB, Robinson FR, Smith VC, Pokorny J, et al. Melanopsin-expressing ganglion cells in primate retina signal colour and irradiance and project to the LGN. Nature. (2005) 433:749–54. doi: 10.1038/nature03387
18. Wong KY, Dunn FA, Graham DM, Berson DM. Synaptic influences on rat ganglion-cell photoreceptors. J Physiol. (2007) 582:279–96. doi: 10.1113/jphysiol.2007.133751
19. Schmidt TM, Taniguchi K, Kofuji P. Intrinsic and extrinsic light responses in melanopsin-expressing ganglion cells during mouse development. J Neurophysiol. (2008) 100:371–84. doi: 10.1152/jn.00062.2008
20. Dumitrescu ON, Pucci FG, Wong KY, Berson DM. Ectopic retinal ON bipolar cell synapses in the OFF inner plexiform layer: contacts with dopaminergic amacrine cells and melanopsin ganglion cells. J Comp Neurol. (2009) 517:226–44. doi: 10.1002/cne.22158
21. Hoshi H, Liu WL, Massey SC, Mills SL. ON inputs to the OFF layer: bipolar cells that break the stratification rules of the retina. J Neurosci. (2009) 29:8875–83. doi: 10.1523/JNEUROSCI.0912-09.2009
22. Schmidt TM, Kofuji P. Differential cone pathway influence on intrinsically photosensitive retinal ganglion cell subtypes. J Neurosci. (2010) 30:16262–71. doi: 10.1523/JNEUROSCI.3656-10.2010
23. Weng S, Estevez ME, Berson DM. Mouse ganglion-cell photoreceptors are driven by the most sensitive rod pathway and by both types of cones. PLoS ONE. (2013) 8:e66480. doi: 10.1371/journal.pone.0066480
24. Belenky MA, Smeraski CA, Provencio I, Sollars PJ, Pickard GE. Melanopsin retinal ganglion cells receive bipolar and amacrine cell synapses. J Comp Neurol. (2003) 460:380–93. doi: 10.1002/cne.10652
25. Zhang DQ, Wong KY, Sollars PJ, Berson DM, Pickard GE, McMahon DG. Intraretinal signaling by ganglion cell photoreceptors to dopaminergic amacrine neurons. Proc Natl Acad Sci USA. (2008) 105:14181–6. doi: 10.1073/pnas.0803893105
26. Reifler AN, Chervenak AP, Dolikian ME, Benenati BA, Li BY, Wachter RD, et al. All spiking, sustained ON displaced amacrine cells receive gap-junction input from melanopsin ganglion cells. Curr Biol. (2015) 25:2763–73. doi: 10.1016/j.cub.2015.09.018
27. Altimus CM, Güler AD, Alam NM, Arman AC, Prusky GT, Sampath AP, et al. Rod photoreceptors drive circadian photoentrainment across a wide range of light intensities. Nat Neurosci. (2010) 13:1107–12. doi: 10.1038/nn.2617
28. van Oosterhout F, Fisher SP, van Diepen HC, Watson TS, Houben T, VanderLeest HT, et al. Ultraviolet light provides a major input to non-image-forming light detection in mice. Curr Biol. (2012) 22:1397–402. doi: 10.1016/j.cub.2012.05.032
29. van Diepen HC, Ramkisoensing A, Peirson SN, Foster RG, Meijer JH. Irradiance encoding in the suprachiasmatic nuclei by rod and cone photoreceptors. FASEB J. (2013) 27:4204–12. doi: 10.1096/fj.13-233098
30. Walmsley L, Hanna L, Mouland J, Martial F, West A, Smedley AR, et al. Colour as a signal for entraining the mammalian circadian clock. PLoS Biol. (2015) 13:e1002127. doi: 10.1371/journal.pbio.1002127
31. Mouland JW, Martial F, Watson A, Lucas RJ, Brown TM. Cones support alignment to an inconsistent world by suppressing mouse circadian responses to the blue colors associated with twilight. Curr Biol. (2019) 29:4260–7. doi: 10.1016/j.cub.2019.10.028
32. Hannibal J, Vrang N, Card JP, Fahrenkrug J. Light-dependent induction of cFos during subjective day and night in PACAP-containing ganglion cells of the retinohypothalamic tract. J Biol Rhythms. (2001) 16:457–70. doi: 10.1177/074873001129002132
33. Mure LS, Rieux C, Hattar S, Cooper HM. Melanopsin-dependent nonvisual responses: evidence for photopigment bistability in vivo. J Biol Rhythms. (2007) 22:411–24. doi: 10.1177/0748730407306043
34. Do MT, Kang SH, Xue T, Zhong H, Liao HW, Bergles DE, et al. Photon capture and signalling by melanopsin retinal ganglion cells. Nature. (2009) 457:281–7. doi: 10.1038/nature07682
35. Wong KY. A retinal ganglion cell that can signal irradiance continuously for 10 hours. J Neurosci. (2012) 32:11478–85. doi: 10.1523/JNEUROSCI.1423-12.2012
36. Emanuel AJ, Do MT. Melanopsin tristability for sustained and broadband phototransduction. Neuron. (2015) 85:1043–55. doi: 10.1016/j.neuron.2015.02.011
37. Zhao X, Pack W, Khan NW, Wong KY. Prolonged inner retinal photoreception depends on the visual retinoid cycle. J Neurosci. (2016) 36:4209–17. doi: 10.1523/JNEUROSCI.2629-14.2016
38. Harrison KR, Reifler AN, Chervenak AP, Wong KY. Prolonged melanopsin-based photoresponses depend in part on RPE65 and cellular retinaldehyde-binding protein (CRALBP). Curr Eye Res. (2020) 7:1–9. doi: 10.1080/02713683.2020.1815793
39. Aggelopoulos NC, Meissl H. Responses of neurones of the rat suprachiasmatic nucleus to retinal illumination under photopic and scotopic conditions. J Physiol. (2000) 523:211–22. doi: 10.1111/j.1469-7793.2000.t01-1-00211.x
40. Dkhissi-Benyahya O, Gronfier C, De Vanssay W, Flamant F, Cooper HM. Modeling the role of mid-wavelength cones in circadian responses to light. Neuron. (2007) 53:677–87. doi: 10.1016/j.neuron.2007.02.005
41. Drouyer E, Rieux C, Hut RA, Cooper HM. Responses of suprachiasmatic nucleus neurons to light and dark adaptation: relative contributions of melanopsin and rod-cone inputs. J Neurosci. (2007) 27:9623–31. doi: 10.1523/JNEUROSCI.1391-07.2007
42. Lall GS, Revell VL, Momiji H, Al Enezi J, Altimus CM, Güler AD, et al. Distinct contributions of rod, cone, and melanopsin photoreceptors to encoding irradiance. Neuron. (2010) 66:417–28. doi: 10.1016/j.neuron.2010.04.037
43. Dollet A, Albrecht U, Cooper HM, Dkhissi-Benyahya O. Cones are required for normal temporal responses to light of phase shifts and clock gene expression. Chronobiol Int. (2010) 27:768–81. doi: 10.3109/07420521003695704
44. Brown TM, Wynne J, Piggins HD, Lucas RJ. Multiple hypothalamic cell populations encoding distinct visual information. J Physiol. (2011) 589:1173–94. doi: 10.1113/jphysiol.2010.199877
45. Johnson CH. Forty years of PRCs–what have we learned? Chronobiol Int. (1999) 16:711–43. doi: 10.3109/07420529909016940
46. Hall JC, Rosbash M. Genes and biological rhythms. Trends Genet. (1987) 3:185–91. doi: 10.1016/0168-9525(87)90231-9
47. Schwartz WJ, Zimmerman P. Circadian timekeeping in BALB/c and C57BL/6 inbred mouse strains. J Neurosci. (1990) 10:3685–94. doi: 10.1523/JNEUROSCI.10-11-03685.1990
48. Khalsa SB, Jewett ME, Cajochen C, Czeisler CA. A phase response curve to single bright light pulses in human subjects. J Physiol. (2003) 549:945–52. doi: 10.1113/jphysiol.2003.040477
49. Clark A. Whatever next? Predictive brains, situated agents, and the future of cognitive science. Behav Brain Sci. (2013) 36:181–204. doi: 10.1017/S0140525X12000477
50. Friston K. A theory of cortical responses. Philos Trans R Soc Lond B Biol Sci. (2005) 360:815–36. doi: 10.1098/rstb.2005.1622
51. Schacter DL, Addis DR, Buckner RL. Episodic simulation of future events: concepts, data, and applications. Ann N Y Acad Sci. (2008) 1124:39–60. doi: 10.1196/annals.1440.001
52. Molinari M, Masciullo M. The implementation of predictions during sequencing. Front Cell Neurosci. (2019) 13:439. doi: 10.3389/fncel.2019.00439
53. Alink A, Schwiedrzik CM, Kohler A, Singer W, Muckli L. Stimulus predictability reduces responses in primary visual cortex. J Neurosci. (2010) 30:2960–6. doi: 10.1523/JNEUROSCI.3730-10.2010
54. Vetter P, Edwards G, Muckli L. Transfer of predictive signals across saccades. Front Psychol. (2012) 3:176. doi: 10.3389/fpsyg.2012.00176
55. Sigman M, Cecchi GA, Gilbert CD, Magnasco MO. On a common circle: natural scenes and Gestalt rules. Proc Natl Acad Sci USA. (2001) 98:1935–40. doi: 10.1073/pnas.98.4.1935
56. Elder JH, Goldberg RM. Ecological statistics of Gestalt laws for the perceptual organization of contours. J Vis. (2002) 2:324–53. doi: 10.1167/2.4.5
57. Coggan DD, Giannakopoulou A, Ali S, Goz B, Watson DM, Hartley T, et al. A data-driven approach to stimulus selection reveals an image-based representation of objects in high-level visual areas. Hum Brain Mapp. (2019) 40:4716–31. doi: 10.1002/hbm.24732
58. Criado-Boado F, Alonso-Pablos D, Blanco MJ, Porto Y, Rodríguez-Paz A, Cabrejas E, et al. Coevolution of visual behaviour, the material world and social complexity, depicted by the eye-tracking of archaeological objects in humans. Sci Rep. (2019) 9:3985. doi: 10.1038/s41598-019-39661-w
59. Iacaruso MF, Gasler IT, Hofer SB. Synaptic organization of visual space in primary visual cortex. Nature. (2017) 547:449–52. doi: 10.1038/nature23019
60. Desolneux A, Moisan L, Morel JM. Computational gestalts and perception thresholds. J Physiol Paris. (2003) 97:311–24. doi: 10.1016/j.jphysparis.2003.09.006
61. Kourtzi Z, Welchman AE. Adaptive shape coding for perceptual decisions in the human brain. J Vis. (2015) 15:2. doi: 10.1167/15.7.2
62. Merriam EP, Colby CL. Active vision in parietal and extrastriate cortex. Neuroscientist. (2005) 11:484–93. doi: 10.1177/1073858405276871
63. Bubic A, von Cramon DY, Schubotz RI. Prediction, cognition and the brain. Front Hum Neurosci. (2010) 4:25. doi: 10.3389/fnhum.2010.00025
64. Hastings JW, Sweeney BM. A persistent diurnal rhythm of luminescence in Gonyaulax polyedra. Biol Bull. (1958) 115:440–58. doi: 10.2307/1539108
65. De Coursey PJ. Daily light sensitivity rhythm in a rodent. Science. (1960) 131:33–5. doi: 10.1126/science.131.3392.33
66. Kim B, Reif E, Wattenberg M, Bengio S, Mozer MC. Neural networks trained on natural scenes exhibit gestalt closure. arXiv:[Preprint] arXiv:1903.01069v4.
67. Redlin U, Mrosovsky N. Masking by light in hamsters with SCN lesions. J Comp Physiol A. (1999) 184:439–48. doi: 10.1007/s003590050343
68. Hut RA, van Oort BE, Daan S. Natural entrainment without dawn and dusk: the case of the European ground squirrel (Spermophilus citellus). J Biol Rhythms. (1999) 14:290–9. doi: 10.1177/074873099129000704
69. Flôres DE, Jannetti MG, Valentinuzzi VS, Oda GA. Entrainment of circadian rhythms to irregular light/dark cycles: a subterranean perspective. Sci Rep. (2016) 6:34264. doi: 10.1038/srep34264
70. Ebihara S, Tsuji K. Entrainment of the circadian activity rhythm to the light cycle: effective light intensity for a Zeitgeber in the retinal degenerate C3H mouse and the normal C57BL mouse. Physiol Behav. (1980) 24:523–7. doi: 10.1016/0031-9384(80)90246-2
71. Hirsh J, Riemensperger T, Coulom H, Iché M, Coupar J, Birman S. Roles of dopamine in circadian rhythmicity and extreme light sensitivity of circadian entrainment. Curr Biol. (2010) 20:209–14. doi: 10.1016/j.cub.2009.11.037
72. Butler MP, Silver R. Divergent photic thresholds in the non-image-forming visual system: entrainment, masking and pupillary light reflex. Proc Biol Sci. (2011) 278:745–50. doi: 10.1098/rspb.2010.1509
73. Pittendrigh CS, Daan S. A functional analysis of circadian pacemakers in nocturnal rodents. IV. Entrainment: pacemaker as clock. J Comp Physiol. (1976) 106:291–331. doi: 10.1007/BF01417859
74. Stephan FK. Circadian rhythms in the rat: constant darkness, entrainment to T cycles and to skeleton photoperiods. Physiol Behav. (1983) 30:451–62. doi: 10.1016/0031-9384(83)90152-X
75. Olde Engberink AHO, Huisman J, Michel S, Meijer JH. Brief light exposure at dawn and dusk can encode day-length in the neuronal network of the mammalian circadian pacemaker. FASEB J. (2020) 34:13685–95. doi: 10.1096/fj.202001133RR
76. Takahashi JS, DeCoursey PJ, Bauman L, Menaker M. Spectral sensitivity of a novel photoreceptive system mediating entrainment of mammalian circadian rhythms. Nature. (1984) 308:186–8. doi: 10.1038/308186a0
77. Nelson DE, Takahashi JS. Sensitivity and integration in a visual pathway for circadian entrainment in the hamster (Mesocricetus auratus). J Physiol. (1991) 439:115–45. doi: 10.1113/jphysiol.1991.sp018660
78. Bruce VG, Weight F, Pittendrigh CS. Resetting the sporulation rhythm in Pilobolus with short light flashes of high intensity. Science. (1960) 131:728–30. doi: 10.1126/science.131.3402.728
79. Pittendrigh CS. Circadian rhythms and the circadian organization of living systems. Cold Spring Harb Symp Quant Biol. (1960) 25:159–84. doi: 10.1101/SQB.1960.025.01.015
80. Joshi D, Chandrashekaran MK. Bright light flashes of 0.5 milliseconds reset the circadian clock of a microchiropteran bat. J Exp Zool. (1984) 230:325–8. doi: 10.1002/jez.1402300219
81. Joshi D, Chandrashekaran MK. Light flashes of different durations (0.063-3.33 msec) phase shift the circadian flight activity of a bat. J Exp Zool. (1985) 233:187–92. doi: 10.1002/jez.1402330204
82. Van Den Pol AN, Cao V, Heller HC. Circadian system of mice integrates brief light stimuli. Am J Physiol. (1998) 275:R654–7. doi: 10.1152/ajpregu.1998.275.2.R654
83. Vidal L, Morin LP. Absence of normal photic integration in the circadian visual system: response to millisecond light flashes. J Neurosci. (2007) 27:3375–82. doi: 10.1523/JNEUROSCI.5496-06.2007
84. Zeitzer JM, Ruby NF, Fisicaro RA, Heller HC. Response of the human circadian system to millisecond flashes of light. PLoS ONE. (2011) 6:e22078. doi: 10.1371/journal.pone.0022078
85. Zeitzer JM, Fisicaro RA, Ruby NF, Heller HC. Millisecond flashes of light phase delay the human circadian clock during sleep. J Biol Rhythms. (2014) 29:370–6. doi: 10.1177/0748730414546532
86. Kaladchibachi S, Negelspach DC, Fernandez F. Circadian phase-shifting by light: beyond photons. Neurobiol Sleep Circadian Rhythms. (2018) 5:8–14. doi: 10.1016/j.nbscr.2018.03.003
87. Arvanitogiannis A, Amir S. Resetting the rat circadian clock by ultra-short light flashes. Neurosci Lett. (1999) 261:159–62. doi: 10.1016/S0304-3940(99)00021-X
88. Kaladchibachi S, Negelspach DC, Zeitzer JM, Fernandez F. Optimization of circadian responses with shorter and shorter millisecond flashes. Biol Lett. (2019) 15:20190371. doi: 10.1098/rsbl.2019.0371
89. Joyce DS, Spitschan M, Zeitzer JM. Integration of brief light flashes varying in intensity and duration by the human circadian system. BioRxiv [Preprint]. doi: 10.1101/759134
90. Vartanian GV, Zhao X, Wong KY. Using flickering light to enhance nonimage-forming visual stimulation in humans. Invest Ophthalmol Vis Sci. (2015) 56:4680–8. doi: 10.1167/iovs.15-16468
91. Najjar RP, Zeitzer JM. Temporal integration of light flashes by the human circadian system. J Clin Invest. (2016) 126:938–47. doi: 10.1172/JCI82306
92. Negelspach DC, Kaladchibachi S, Fernandez F. The circadian activity rhythm is reset by nanowatt pulses of ultraviolet light. Proc Biol Sci. (2018) 285:20181288. doi: 10.1098/rspb.2018.1288
93. Woelders T, Wams EJ, Gordijn MCM, Beersma DGM, Hut RA. Integration of color and intensity increases time signal stability for the human circadian system when sunlight is obscured by clouds. Sci Rep. (2018) 8:15214. doi: 10.1038/s41598-018-33606-5
94. Wagemans J, Elder JH, Kubovy M, Palmer SE, Peterson MA, Singh M, et al. A century of Gestalt psychology in visual perception: I. Perceptual grouping and figure-ground organization. Psychol Bull. (2012) 138:1172–217. doi: 10.1037/a0029333
95. Kveraga K, Ghuman AS, Bar M. Top-down predictions in the cognitive brain. Brain Cogn. (2007) 65:145–68. doi: 10.1016/j.bandc.2007.06.007
96. Gilbert CD, Sigman M. Brain states: top-down influences in sensory processing. Neuron. (2007) 54:677–96. doi: 10.1016/j.neuron.2007.05.019
97. Harrison LM, Stephan KE, Rees G, Friston KJ. Extra-classical receptive field effects measured in striate cortex with fMRI. Neuroimage. (2007) 34:1199–208. doi: 10.1016/j.neuroimage.2006.10.017
98. Katona TM, Pattison PM, Paolini S. Status of solid state lighting product development and future trends for general illumination. Annu Rev Chem Biomol Eng. (2016) 7:263–81. doi: 10.1146/annurev-chembioeng-080615-034625
99. Pattison PM, Tsao JY, Brainard GC, Bugbee B. LEDs for photons, physiology and food. Nature. (2018) 563:493–500. doi: 10.1038/s41586-018-0706-x
100. Dobb R, Martial F, Elijah D, Storchi R, Brown TM, Lucas RJ. The impact of temporal modulations in irradiance under light adapted conditions on the mouse suprachiasmatic nuclei (SCN). Sci Rep. (2017) 7:10582. doi: 10.1038/s41598-017-11184-2
101. Normann RA, Perlman I. The effects of background illumination on the photoresponses of red and green cones. J Physiol. (1979) 286:491–507. doi: 10.1113/jphysiol.1979.sp012633
102. Burkhardt DA. Light adaptation and photopigment bleaching in cone photoreceptors in situ in the retina of the turtle. J Neurosci. (1994) 14:1091–105. doi: 10.1523/JNEUROSCI.14-03-01091.1994
103. Nathan J, Reh R, Ankoudinova I, Ankoudinova G, Chang B, Heckenlively J, et al. Scotopic and photopic visual thresholds and spatial and temporal discrimination evaluated by behavior of mice in a water maze. Photochem Photobiol. (2006) 82:1489–94. doi: 10.1111/j.1751-1097.2006.tb09804.x
104. Demontis GC, Bisti S, Cervetto L. Light sensitivity, adaptation and saturation in mammalian rods. Prog Brain Res. (1993) 95:15–24. doi: 10.1016/S0079-6123(08)60353-2
105. Ecker JL, Dumitrescu ON, Wong KY, Alam NM, Chen SK, LeGates T, et al. Melanopsin-expressing retinal ganglion-cell photoreceptors: cellular diversity and role in pattern vision. Neuron. (2010) 67:49–60. doi: 10.1016/j.neuron.2010.05.023
106. Walch OJ, Zhang LS, Reifler AN, Dolikian ME, Forger DB, Wong KY. Characterizing and modeling the intrinsic light response of rat ganglion-cell photoreceptors. J Neurophysiol. (2015) 114:2955–66. doi: 10.1152/jn.00544.2015
107. Lupi D, Cooper HM, Froehlich A, Standford L, McCall MA, Foster RG. Transgenic ablation of rod photoreceptors alters the circadian phenotype of mice. Neuroscience. (1999) 89:363–74. doi: 10.1016/S0306-4522(98)00353-4
108. Calvert PD, Krasnoperova NV, Lyubarsky AL, Isayama T, Nicoló M, Kosaras B, et al. Phototransduction in transgenic mice after targeted deletion of the rod transducin alpha-subunit. Proc Natl Acad Sci USA. (2000) 97:13913–8. doi: 10.1073/pnas.250478897
109. Chang B, Dacey MS, Hawes NL, Hitchcock PF, Milam AH, Atmaca-Sonmez P, et al. Cone photoreceptor function loss-3, a novel mouse model of achromatopsia due to a mutation in Gnat2. Invest Ophthalmol Vis Sci. (2006) 47:5017–21. doi: 10.1167/iovs.05-1468
110. Schroeder MM, Harrison KR, Jaeckel ER, Berger HN, Zhao X, Flannery MP, et al. The roles of rods, cones, and melanopsin in photoresponses of M4 intrinsically photosensitive retinal ganglion cells (ipRGCs) and optokinetic visual behavior. Front Cell Neurosci. (2018) 12:203. doi: 10.3389/fncel.2018.00203
111. Genead MA, Fishman GA, Rha J, Dubis AM, Bonci DM, Dubra A, et al. Photoreceptor structure and function in patients with congenital achromatopsia. Invest Ophthalmol Vis Sci. (2011) 52:7298–308. doi: 10.1167/iovs.11-7762
Keywords: light, circadian, rhythms, photostimulation, flash, retina, photoreceptors, ipRGC
Citation: Wong KY and Fernandez F-X (2021) Circadian Responses to Light-Flash Exposure: Conceptualization and New Data Guiding Future Directions. Front. Neurol. 12:627550. doi: 10.3389/fneur.2021.627550
Received: 09 November 2020; Accepted: 21 January 2021;
Published: 11 February 2021.
Edited by:
Victoria Susan Pelak, University of Colorado, United StatesReviewed by:
Chiara La Morgia, Istituto di Ricovero e Cura a Carattere Scientifico (IRCCS) Institute of Neurological Sciences of Bologna (ISNB), ItalyChristophe P. Ribelayga, University of Texas Health Science Center at Houston, United States
Kenkichi Baba, Morehouse School of Medicine, United States
Copyright © 2021 Wong and Fernandez. This is an open-access article distributed under the terms of the Creative Commons Attribution License (CC BY). The use, distribution or reproduction in other forums is permitted, provided the original author(s) and the copyright owner(s) are credited and that the original publication in this journal is cited, in accordance with accepted academic practice. No use, distribution or reproduction is permitted which does not comply with these terms.
*Correspondence: Kwoon Y. Wong, a3dvb25AdW1pY2guZWR1; Fabian-Xosé Fernandez, RmFiaWFuRkBlbWFpbC5hcml6b25hLmVkdQ==