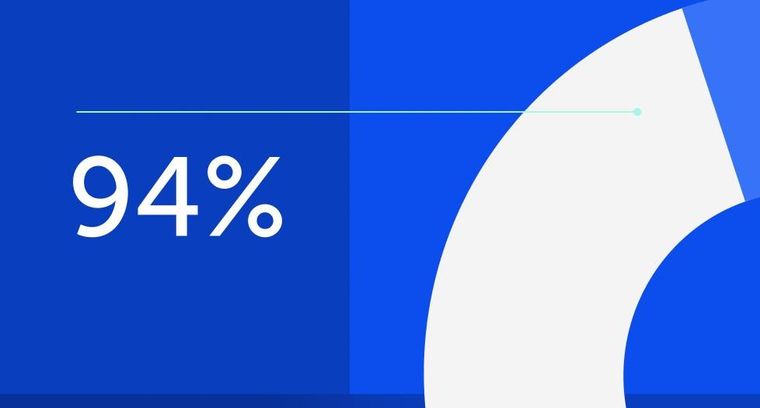
94% of researchers rate our articles as excellent or good
Learn more about the work of our research integrity team to safeguard the quality of each article we publish.
Find out more
MINI REVIEW article
Front. Neurol., 10 February 2021
Sec. Dementia and Neurodegenerative Diseases
Volume 12 - 2021 | https://doi.org/10.3389/fneur.2021.610330
This article is part of the Research TopicTau Pathology in Neurological DisordersView all 18 articles
Adult hippocampal neurogenesis (AHN) has been widely confirmed in mammalian brains. A growing body of evidence points to the fact that AHN sustains hippocampal-dependent functions such as learning and memory. Impaired AHN has been reported in post-mortem human brain hippocampus of Alzheimer's disease (AD) and is considered to contribute to defects in learning and memory. Neurofibrillary tangles (NFTs) and amyloid plaques are the two key neuropathological hallmarks of AD. NFTs are composed of abnormal tau proteins accumulating in many brain areas during the progression of the disease, including in the hippocampus. The physiological role of tau and impact of tau pathology on AHN is still poorly understood. Modifications in AHN have also been reported in some tau transgenic and tau-deleted mouse models. We present here a brief review of advances in the relationship between development of tau pathology and AHN in AD and what insights have been gained from studies in tau mouse models.
AD has two neuropathological hallmarks, amyloid plaques, and NFTs. Amyloid plaques are composed of amyloid ß peptides (1) derived from successive cleavages of amyloid precursor protein (APP) (2). NFTs are constituted of microtubule-associated protein tau (MAPT) (3). In a family of neurodegenerative diseases called tauopathies including AD, tau undergoes hyperphosphorylation and aggregation to develop pathological forms of tau species such as oligomers or highly insoluble filaments that form NFTs. The levels of NFTs are highly correlated with cognitive decline (4). Tauopathies include frontotemporal lobar degeneration (FTLD) with tau positive inclusions with or without gene mutation in MAPT, Pick disease, progressive supranuclear palsy, corticobasal degeneration, and others (5). In AD brains, tau deposition occurs in a stereotypical manner, with the hippocampus, limbic structures, brain stem, and the basal nucleus of Meynert being most affected at the early stages (6). The hippocampus is a crucial brain structure for the acquisition of new memories and retrieval of older memories. Afferent pathways to the dentate gyrus (DG) are affected by NFTs developing in the entorhinal cortex (6), and NFTs develop in the granule cell layer (GCL) (7, 8) in the DG in AD and in some tau transgenic mouse models (Figures 1A–D). Tau pathology in the DG might play a role in memory impairment. Whereas, abnormalities in AHN have been extensively investigated in AD mouse models based on APP or PSEN1/2 familial AD mutations (13, 14), the impact of tau pathology on AHN remains largely unclear in AD and other tauopathies. We provide here a brief overview of recent advances on the relationship between development of tau pathology and AHN in AD and what insights have been gained from studies in tau transgenic mouse models.
Figure 1. (A–C) Representative photos of tau pathology detected in the DG of post-mortem brain section of a 65-year-old male AD patient (Braak VI). Tau pathology was detected by anti-total tau B19 antibody (9) (A), anti-phospho Ser396/404 tau PHF1 antibody (10) (B), or by Gallyas silver staining (11) (C). (D) Representative photo of tau pathology detected by PHF1 in the DG of 12-month-old tau Tg30 mice (12). ML, molecular layer; GCL, granule cell layer; SGZ, subgranular zone. (E) Specific markers for five different stages of AHN in the dentate gyrus of the hippocampus. GFAP, glial fibrillary acidic protein; BLBP, brain lipid-binding protein; SOX2, SRY (sex determining region Y)-box 2; DCX, doublecortin; 3R tau, tau with 3 repeats of microtubule-binding sequences; NeuN, neuronal nuclei; 4R tau, tau with 4 repeats of microtubule-binding sequences. (F) Schematic representation of the human 6 isoforms of tau protein. Exon 2, 3, and 10 (E2, E3, and E10, respectively) are alternatively spliced. Alternative splicing leads to 0, 1 or 2 inserts near amino terminus (0N, 1N, or 2N, respectively) and 3 or 4 repeats (3R or 4R, respectively) of microtubule-binding sequences near carboxyl terminus. The shortest 0N3R isoform is predominantly detected in immature neurons of fetal brains and of adult hippocampus. While only 4R isoforms are principally expressed in adult mouse brains, all the 6 isoforms are expressed in adult human brains. (G) Immunostaining of immature neurons by anti-3R tau RD3 antibody (Merck Millipore #05-803) in the dentate gyrus of the hippocampus in a 12-month-old wild-type mouse. (H) Functional involvement of tau at different stages of AHN. Hematoxylin counterstaining for (A–D,G). Detailed protocol on histological analyses is available in (12). Scale bars: 25 μm.
Since its discovery in mammalian brain in 1965 (15), AHN has been documented in many species (16–20). In placental mammals and marsupials, adult neurogenesis is mainly limited to two areas: the subventricular zone (SVZ) along the lateral ventricles and the subgranular zone (SGZ) of the dentate gyrus (DG). AHN is necessary for spatial memory and specific learning tasks and is related to mood regulation (21, 22).
Neural stem cells found in the SGZ of the hippocampus generate new neurons for the DG (23). The identity of adult neural stem cells remains still controversial. Growing evidence suggests that they have an astrocytic phenotype (24, 25) or they may be radial glial cells, able to give rise asymmetrically either to a glial cell or a neuron (26). There are five principal developmental stages of AHN starting from the radial glia-like cells, progenitor cells, neuroblast cells, immature neurons, and finally mature neurons as granular cells (27). These stages can be identified by specific markers such as GFAP, BLBP, SOX2, Nestin, Doublecortin (DCX), tau with three-repeats (3R) or four-repeats (4R) of microtubule-binding repeat domains (RD), NeuN, and Calbindin (Figure 1E) (23, 28). Newborn cells can be experimentally traced using exogenous cell tracers such as thymidine analogs that are incorporated into dividing cells during DNA synthesis (29). Newborn neurons can be also identified by other mitotic markers such as Ki67 in combination with neuronal markers (30). Studies have provided compelling evidence for the persistence of AHN in humans and non-human primates (31, 32). There are some contradictory findings pointing to hardly detectable levels of AHN in human brains due to a sharp decrease in childhood (33, 34). A breakthrough was made when a study provided evidence for the birth of ~700 newborn neurons a day per one adult human hippocampus by measuring the concentration of nuclear bomb test-derived 14C in genome DNA (35). By a similar approach, striatum has also been recently identified as a neurogenic zone in the adult human brain (36). Annual turnover rates are estimated as 1.75% of neurons in the hippocampus and 2.7% in the striatum in the human adult brain (35, 36). Although observed in other species than human (37, 38), the role of adult striatal neurogenesis remains largely elusive (39).
Stress, aging, and disease have a negative impact on AHN (40). On the contrary, AHN can be enhanced in rodents by lifestyle factors such as environmental enrichment (EE) (41), physical activity (e.g., running) (42, 43), anti-depressants (44), or electroconvulsive seizures (45).
Tau is a cytosolic protein predominantly expressed in neurons. Tau has physiological roles, the most studied being the regulation of the axonal transport and of the cytoskeleton by maintaining the stability of microtubules (46). Human MAPT gene is located on chromosome 17 and contains 16 exons. Exons 2, 3, and 10 are alternatively spliced to give rise to six different isoforms in the adult human central nervous system (Figure 1F) (47). Alternative splicing of exon 10 results in generating either tau with 3R or 4R microtubule-binding sequences in the half carboxyl domain. 3R and 4R tau isoforms include sequences of exon 2, exons 2 and 3, or none of them in their amino domain. Tau regulates axonal microtubule assembly but has also other functions (48) by interacting with many partners in addition to microtubules (49, 50). Among other functions, tau is implicated in pathways regulating synaptic plasticity, cell signaling, and DNA integrity (51). Tau is also secreted via several pathways (52), a process that is thought to play a role in the “Prion-like” propagation of tau pathology (53) but that is not well-understood in physiological conditions. This multifunctional aspect of tau might be involved in the regulation of AHN.
While six isoforms are expressed in adult human brain, only 4R isoforms are predominantly detected in the mature neurons of mouse brains. During brain development, only the 0N3R isoform (fetal isoform) is expressed in human and rodent brains (54, 55). Owing to the lack of one microtubule-binding domain, 3R tau isoforms have less affinity for microtubules and consequently less efficiency to promote microtubule assembly compared to 4R isoforms (56). Expression of 3R tau isoforms is thus related to plasticity in neuronal development in neonatal stage and in neurogenesis for dynamic process formation, neurite elongation, and neuronal polarity (57–59). 3R tau isoform lacking exon 2 and 3 is also expressed in the adult brain in the immature neurons in the SGZ (60) and can be used as a specific marker to detect newborn neurons and newly generated axons in the adult mouse hippocampus (28, 61). The number of cells expressing 3R tau isoforms in the SGZ decreases with age in mice, but they are still detectable at 12 months (Figure 1G) (12). Tau in immature neurons in the SGZ shows a higher phosphorylation seemingly through activated GSK-3 (62), reducing its affinity for microtubules and providing these cells with a more dynamic microtubule network during dendritic and axonal outgrowth. In these immature neurons, tau is abundant in the somatodendritic domain (as during development) and appears to be at least partly in a microtubule-unbound form (63). Increased tau phosphorylation is associated with increased proliferation of newborn neurons (62).
Emerging evidence suggests that overall AHN (e.g., generation of fully functional new neurons) is reduced in AD (64). The detection of AHN markers by immunohistochemistry on post-mortem brain tissues has recently confirmed the existence of AHN in aged healthy subjects and a significant reduction of DCX-positive immature neurons in AD brains (65, 66). AHN drops sharply even at the early stage of cognitive decline in the patients with mild cognitive impairment (66). These studies imply that the reduction of AHN may directly compromise cognitive functions (67). Importantly, SOX2-positive neural stem cells were increased in some cognitively normal subjects but with extensive AD neuropathological lesions (68), implying that increased AHN may rescue cognitive deficits caused by AD lesions. Numerous genetic factors and variants implicated in AD (Apolipoprotein E, PSEN1, APP) have been identified with a modulating role on AHN in human AD patients (69). This observation is supported by the generalized decrease in newborn neuron generation observed in various AD transgenic mouse models overexpressing FAD-related mutant APP and/or PS1 (13) or overexpressing APP intracellular C-terminal domain fragments (AICD) (70).
Studies of AHN in different tau transgenic mouse models have suggested that tau has critical roles in proliferation, neuronal differentiation/maturation, dendritic/axonal outgrowth, neuronal plasticity and synaptic maturation in DG. Tau is also involved in selective cell death of newborn granule neurons in case of acute stress (71) (Figure 1H). However there remain controversies in distinct tau models (Table 1). Whereas tau knockout mice are viable and macroscopically normal (72, 73, 92), behavioral studies have unraveled that they exhibit abnormalities such as hyperactivity (93) and deficits in short-time memory in an age-dependent manner (94). Deletion of endogenous tau also leads to delayed neuronal maturation in primary cultured neurons (73) and transcriptional repression of neuronal genes in the hippocampus (95). A significant reduction of DCX- and NeuroD- positive neuroblast cells in tau knockout mice was observed (62). On the contrary, Criado-Marrero et al. have recently reported that BrdU-positive newborn cells and DCX-positive immature neurons were increased in the DG and SVZ of tau knockout mice at 14 months (75). Yet, other two independent studies have reported that DCX-labeled neuroblast cell number was not altered in the DG of adult tau knockout mice (71, 74). Moreover, tau has critical roles in both stress-induced suppression of AHN and stimulatory effect of EE. Unlike wild-type mice, tau knockout mice are insensitive to the modulation of AHN by stress or EE (71).
Human non-mutant tau seems to have several roles in AHN such as suppressing proliferation and promoting neuronal differentiation. KOKI mice expressing human 2N4R tau isoform in the absence of murine tau (76) had an increase in DCX-positive immature neurons, hippocampal volume and cell number in DG and an improved cognitive function (77). Nevertheless, other studies suggest negative effect of human non-mutant tau on AHN in mouse brains. hTau mice expressing the 6 isoforms of non-mutant wild-type human tau (78) in the absence of murine tau had reduced DCX-positive immature neurons at 2 and 6 months (79). Hippocampal injection of soluble non-mutant 2N4R human tau led to morphological changes of newborn granule neurons without changing the total number of DCX-positive neuroblast cells (80). Adeno-associated virus-mediated specific overexpression of human tau in DG interneurons induced deficits in AHN by suppressing GABAergic transmission (81). Another recent study has reported an impact of glial tau accumulation on AHN. Lentiviral-mediated 1N3R tau accumulation in hilar astrocytes in mouse led to reduction of AHN accompanied by impaired spatial memory performances (82).
Abnormalities in AHN have been observed in FTLD-mutant tau transgenic mouse models. In THY-Tau22 and Tg30 mice that express a human 1N4R tau mutated at G272V and P301S under a Thy1.2-promoter, Gallyas-positive NFTs are detectable from 6 months in hippocampus (83, 85). An increase in AHN was observed with the DCX and BrdU markers in 6-month-old THY-Tau22 mouse (84). Nonetheless, Tg30 mice exhibited an impaired AHN at 12 months, an age in which some of the granule cells in DG have a severe somatodendritic tau pathology (Figure 1D) (12). By crossing Tg30 with tau knockout mice (72), we generated Tg30/tauKO mice that express only human mutant tau in the absence of murine tau (86, 87). The reduction of AHN observed in Tg30 mice at 12 months was rescued in the Tg30/tauKO mouse model as measured by DCX-positive cell number (12). Another independent study reported that TauVLW mice carrying G272V, P301L, and R406W mutant tau (88) also had decreased DCX-positive immature neurons (89). Interestingly, EE significantly increased the number of DCX-positive immature neurons in wild-type littermates but not in TauVLW mice (89). To our knowledge, this is the first and only report showing that tau pathology may inhibit the response to a positive factor enhancing AHN.
The overall controversies may derive from the variation in the age and from the heterogeneities of tau species in distinct models. TauRDDKPP mice expressing anti-aggregant tau RD showed increased number of DCX-positive cells in DG and a larger volume of hippocampus unlike tauRDDK mice expressing pro-aggregant mutant tau RD (91). The latter findings support the idea that distinct tau species seem to have different effects on neurogenesis.
There are conflicting reports as to whether AHN persists in late age in humans. Controversies may be partially due to the limited availability of adequately preserved post-mortem human brain samples. The technical and methodological issues can further add variability in detecting specific markers of neural stem and progenitor cells in human autopsy tissues. Some of the conflicting results are also presumably related to the heterogeneities in individual life stories: age, sex, lifestyle, physical activities, with or without previous disease histories, and medical status at the end of life. There is a great variability in the post-mortem delays and processing methods of human post-mortem brain tissues. In general, fixation is known to play a critical role in antigen preservation since some epitopes are more prone to denaturation during the fixation. For example, the immature neuron marker DCX undergoes rapid degradation during the post-mortem period (96). Some difficulties could be overcome by tightly documenting the brain samples and their processing, optimizing the methodologies (65), and standardization of detailed protocols (97).
Although tau seems involved in modulating AHN, there are controversies among the different tau mouse models about the effect of tau ablation or overexpression. As for human samples, controversial reports may derive from distinct protocols and various parameters such as genetic background, age, gender, and tau species. Distinct time point of analysis could lead to data variation (98). A remarkable sex difference was observed in AHN of rodent brains (75, 99). Furthermore, data variability may be caused by the sensitivities of antibodies used for detection (29). Besides, the methods of analysis and quantification have significant impact on the results. One of the most commonly used approaches is to measure total proliferating cell number using optical fractionator, an unbiased stereological method, on serial sections of the whole hippocampus (100). Since the distributions of the proliferating cells are not homogeneous and are often in the form of clusters in the SGZ of hippocampus (30), measuring setup needs to be carefully optimized (101).
The mechanisms behind AHN impairment in AD are still poorly understood. Numbers of independent studies have shown that amyloid pathology, APP, and PSEN1/2 are involved in modulating AHN in AD transgenic mouse brains (13). Since tau pathology led to defects in AHN in several tauopathy mouse models (12, 89, 91), we support the idea that tau pathology impairs AHN independently from amyloid pathology. In this context, it would be highly informative to study AHN in the post-mortem human brains of primary tauopathies devoid of amyloid pathology (e.g., FTLD with tau pathology, etc.). Yet, more studies are necessary to better understand both physiological and pathological roles of tau in AHN.
Given that increased AHN is associated with preservation of cognitive functions in non-demented individuals with AD lesions (68), stimulation of AHN should be beneficial. However, tau pathology presumably plays a negative role in AHN: EE led to increased AHN in wild-type mice but not in tauVLW transgenic mice (89). Taking into consideration that an ablation of murine tau rescued AHN impairment in Tg30 mice (12) and stress-induced suppression of neurogenesis (74), reduction of tau may be beneficial for AHN. Indeed, there is compelling evidence showing the efficacy of tau reduction via anti-sense oligonucleotides (ASOs) to prolong life expectancy, reduce tau pathology, and rescue behavioral deficits in tau transgenic mice (102). Cautions need to be taken as complete ablation of tau leads to deficits in cognitive function in an age-dependent manner (94). Tau is a multifunctional protein and the net benefit of long-term reduction of tau still remains unclear (48). There are numbers of factors that can boost AHN such as EE, physical activities, or pharmacological agents (44). Testing these in tau transgenic models of tauopathies in combination with modulation of tau expression may open a new window for future therapies.
SH, J-PB, and KA wrote the main manuscript. All the authors participated in constructing the concept and writing the manuscript, contributed to manuscript revision, and read and approved the submitted version.
This study was supported by grants from the Belgian Fonds de la Recherche Scientifique Médicale (T.0027.19) to J-PB and KL, the Fund Aline (King Baudoin Foundation) to J-PB, the Belgian Fondation Recherche Alzheimer/Stichting Alzheimer Onderzoek to J-PB and KL, and the Génicot Fund (ULB) to J-PB and KL.
The authors declare that the research was conducted in the absence of any commercial or financial relationships that could be construed as a potential conflict of interest.
We sincerely thank Dr. Peter Davies for the generous gift of anti-PHF1 antibody and Dr. Luc Buée for Tg30 mice.
1. Wong CW, Quaranta V, Glenner GG. Neuritic plaques and cerebrovascular amyloid in Alzheimer disease are antigenically related. Proc Natl Acad Sci USA. (1985) 82:8729–32. doi: 10.1073/pnas.82.24.8729
2. Glenner GG, Wong CW. Alzheimer's disease: initial report of the purification and characterization of a novel cerebrovascular amyloid protein. Biochem Biophys Res Commun. (1984) 120:885–90. doi: 10.1016/S0006-291X(84)80190-4
3. Brion JP, Passareiro H, Nunez J, Flament-Durand J. Mise en évidence immunologique de la protéine tau au niveau des lésions de dégénérescence neurofibrillaire de la maladie d'Alzheimer. Arch Biol. (1985) 95:229–35.
4. Nelson PT, Alafuzoff I, Bigio EH, Bouras C, Braak H, Cairns NJ, et al. Correlation of Alzheimer disease neuropathologic changes with cognitive status: a review of the literature. J Neuropathol Exp Neurol. (2012) 71:362–81. doi: 10.1097/NEN.0b013e31825018f7
5. Buee L, Bussiere T, Buee-Scherrer V, Delacourte A, Hof PR. Tau protein isoforms, phosphorylation and role in neurodegenerative disorders. Brain Res Brain Res Rev. (2000) 33:95–130. doi: 10.1016/S0165-0173(00)00019-9
6. Braak H, Braak E. Neuropathological stageing of Alzheimer-related changes. Acta Neuropathol. (1991) 82:239–59. doi: 10.1007/BF00308809
7. Dickson DW, Yen SH, Horoupian DS. Pick body-like inclusions in the dentate fascia of the hippocampus in Alzheimer's disease. Acta Neuropathol. (1986) 71:38–45. doi: 10.1007/BF00687960
8. Wakabayashi K, Hansen LA, Vincent I, Mallory M, Masliah E. Neurofibrillary tangles in the dentate granule cells of patients with Alzheimer's disease, Lewy body disease and progressive supranuclear palsy. Acta Neuropathol. (1997) 93:7–12. doi: 10.1007/s004010050576
9. Brion JP, Hanger DP, Bruce MT, Couck AM, Flament-Durand J, Anderton BH. Tau in Alzheimer neurofibrillary tangles. N- and C-terminal regions are differentially associated with paired helical filaments and the location of a putative abnormal phosphorylation site. Biochem J. (1991) 273:127–33. doi: 10.1042/bj2730127
10. Otvos LJr, Feiner L, Lang E, Szendrei GI, Goedert M, Lee VM. Monoclonal antibody PHF-1 recognizes tau protein phosphorylated at serine residues 396 and 404. J Neurosci Res. (1994) 39:669–73. doi: 10.1002/jnr.490390607
11. Kuninaka N, Kawaguchi M, Ogawa M, Sato A, Arima K, Murayama S, et al. Simplification of the modified Gallyas method. Neuropathology. (2015) 35:10–5. doi: 10.1111/neup.12144
12. Houben S, Leroy K, Ando K, Yilmaz Z, Widomski C, Buee L, et al. Genetic ablation of tau in postnatal neurons rescues decreased adult hippocampal neurogenesis in a tauopathy model. Neurobiol Dis. (2019) 127:131–41. doi: 10.1016/j.nbd.2019.02.021
13. Mu Y, Gage FH. Adult hippocampal neurogenesis and its role in Alzheimer's disease. Mol Neurodegener. (2011) 6:85. doi: 10.1186/1750-1326-6-85
14. Hollands C, Tobin MK, Hsu M, Musaraca K, Yu TS, Mishra R, et al. Depletion of adult neurogenesis exacerbates cognitive deficits in Alzheimer's disease by compromising hippocampal inhibition. Mol Neurodegener. (2017) 12:64. doi: 10.1186/s13024-017-0207-7
15. Altman J, Das GD. Autoradiographic and histological evidence of postnatal hippocampal neurogenesis in rats. J Comp Neurol. (1965) 124:319–35. doi: 10.1002/cne.901240303
16. West MJ. Stereological studies of the hippocampus: a comparison of the hippocampal subdivisions of diverse species including hedgehogs, laboratory rodents, wild mice and men. Prog Brain Res. (1990) 83:13–36. doi: 10.1016/S0079-6123(08)61238-8
17. Barnea A, Nottebohm F. Seasonal recruitment of hippocampal neurons in adult free-ranging black-capped chickadees. Proc Natl Acad Sci USA. (1994) 91:11217–21. doi: 10.1073/pnas.91.23.11217
18. Cai Y, Xiong K, Chu Y, Luo DW, Luo XG, Yuan XY, et al. Doublecortin expression in adult cat and primate cerebral cortex relates to immature neurons that develop into GABAergic subgroups. Exp Neurol. (2009) 216:342–56. doi: 10.1016/j.expneurol.2008.12.008
19. Wullimann MF. Secondary neurogenesis and telencephalic organization in zebrafish and mice: a brief review. Integr Zool. (2009) 4:123–33. doi: 10.1111/j.1749-4877.2008.00140.x
20. Kempermann G. New neurons for 'survival of the fittest'. Nat Rev Neurosci. (2012) 13:727–36. doi: 10.1038/nrn3319
21. Stone SS, Teixeira CM, Devito LM, Zaslavsky K, Josselyn SA, Lozano AM, et al. Stimulation of entorhinal cortex promotes adult neurogenesis and facilitates spatial memory. J Neurosci. (2011) 31:13469–84. doi: 10.1523/JNEUROSCI.3100-11.2011
23. Ming GL, Song H. Adult neurogenesis in the mammalian brain: significant answers and significant questions. Neuron. (2011) 70:687–702. doi: 10.1016/j.neuron.2011.05.001
24. Doetsch F, Caille I, Lim DA, Garcia-Verdugo JM, Alvarez-Buylla A. Subventricular zone astrocytes are neural stem cells in the adult mammalian brain. Cell. (1999) 97:703–16. doi: 10.1016/S0092-8674(00)80783-7
25. Alvarez-Buylla A, Garcia-Verdugo JM, Tramontin AD. A unified hypothesis on the lineage of neural stem cells. Nat Rev Neurosci. (2001) 2:287–93. doi: 10.1038/35067582
26. Kriegstein A, Alvarez-Buylla A. The glial nature of embryonic and adult neural stem cells. Annu Rev Neurosci. (2009) 32:149–84. doi: 10.1146/annurev.neuro.051508.135600
27. Kozareva DA, Cryan JF, Nolan YM. Born this way: hippocampal neurogenesis across the lifespan. Aging Cell. (2019) 18:e13007. doi: 10.1111/acel.13007
28. Fuster-Matanzo A, Llorens-Martin M, Jurado-Arjona J, Avila J, Hernandez F. Tau protein and adult hippocampal neurogenesis. Front Neurosci. (2012) 6:104. doi: 10.3389/fnins.2012.00104
29. Leuner B, Glasper ER, Gould E. Thymidine analog methods for studies of adult neurogenesis are not equally sensitive. J Comp Neurol. (2009) 517:123–33. doi: 10.1002/cne.22107
30. Wojtowicz JM, Kee N. BrdU assay for neurogenesis in rodents. Nat Protoc. (2006) 1:1399–405. doi: 10.1038/nprot.2006.224
31. Eriksson PS, Perfilieva E, Bjork-Eriksson T, Alborn AM, Nordborg C, Peterson DA, et al. Neurogenesis in the adult human hippocampus. Nat Med. (1998) 4:1313–7. doi: 10.1038/3305
32. Boldrini M, Fulmore CA, Tartt AN, Simeon LR, Pavlova I, Poposka V, et al. Human hippocampal neurogenesis persists throughout aging. Cell Stem Cell. (2018) 22:589–99 e585. doi: 10.1016/j.stem.2018.03.015
33. Cipriani S, Ferrer I, Aronica E, Kovacs GG, Verney C, Nardelli J, et al. Hippocampal radial glial subtypes and their neurogenic potential in human fetuses and healthy and Alzheimer's disease adults. Cereb Cortex. (2018) 28:2458–78. doi: 10.1093/cercor/bhy096
34. Sorrells SF, Paredes MF, Cebrian-Silla A, Sandoval K, Qi D, Kelley KW, et al. Human hippocampal neurogenesis drops sharply in children to undetectable levels in adults. Nature. (2018) 555:377–81. doi: 10.1038/nature25975
35. Spalding KL, Bergmann O, Alkass K, Bernard S, Salehpour M, Huttner HB, et al. Dynamics of hippocampal neurogenesis in adult humans. Cell. (2013) 153:1219–27. doi: 10.1016/j.cell.2013.05.002
36. Ernst A, Alkass K, Bernard S, Salehpour M, Perl S, Tisdale J, et al. Neurogenesis in the striatum of the adult human brain. Cell. (2014) 156:1072–83. doi: 10.1016/j.cell.2014.01.044
37. Dayer AG, Cleaver KM, Abouantoun T, Cameron HA. New GABAergic interneurons in the adult neocortex and striatum are generated from different precursors. J Cell Biol. (2005) 168:415–27. doi: 10.1083/jcb.200407053
38. Luzzati F, De Marchis S, Fasolo A, Peretto P. Neurogenesis in the caudate nucleus of the adult rabbit. J Neurosci. (2006) 26:609–21. doi: 10.1523/JNEUROSCI.4371-05.2006
39. Inta D, Cameron HA, Gass P. New neurons in the adult striatum: from rodents to humans. Trends Neurosci. (2015) 38:517–23. doi: 10.1016/j.tins.2015.07.005
40. Lazarov O, Mattson MP, Peterson DA, Pimplikar SW, Van Praag H. When neurogenesis encounters aging and disease. Trends Neurosci. (2010) 33:569–79. doi: 10.1016/j.tins.2010.09.003
41. Kempermann G, Gast D, Gage FH. Neuroplasticity in old age: sustained fivefold induction of hippocampal neurogenesis by long-term environmental enrichment. Ann Neurol. (2002) 52:135–43. doi: 10.1002/ana.10262
42. Van Praag H, Kempermann G, Gage FH. Running increases cell proliferation and neurogenesis in the adult mouse dentate gyrus. Nat Neurosci. (1999) 2:266–70. doi: 10.1038/6368
43. Dostes S, Dubreucq S, Ladeveze E, Marsicano G, Abrous DN, Chaouloff F, et al. Running per se stimulates the dendritic arbor of newborn dentate granule cells in mouse hippocampus in a duration-dependent manner. Hippocampus. (2016) 26:282–8. doi: 10.1002/hipo.22551
44. Malberg JE, Eisch AJ, Nestler EJ, Duman RS. Chronic antidepressant treatment increases neurogenesis in adult rat hippocampus. J Neurosci. (2000) 20:9104–10. doi: 10.1523/JNEUROSCI.20-24-09104.2000
45. Schloesser RJ, Orvoen S, Jimenez DV, Hardy NF, Maynard KR, Sukumar M, et al. Antidepressant-like effects of electroconvulsive seizures require adult neurogenesis in a neuroendocrine model of depression. Brain Stimul. (2015) 8:862–7. doi: 10.1016/j.brs.2015.05.011
46. Wang Y, Mandelkow E. Tau in physiology and pathology. Nat Rev Neurosci. (2016) 17:22–35. doi: 10.1038/nrn.2015.1
47. Goedert M, Spillantini MG, Jakes R, Rutherford D, Crowther RA. Multiple isoforms of human microtubule-associated protein tau: sequences and localization in neurofibrillary tangles of Alzheimer's disease. Neuron. (1989) 3:519–26. doi: 10.1016/0896-6273(89)90210-9
48. Sotiropoulos I, Galas MC, Silva JM, Skoulakis E, Wegmann S, Maina MB, et al. Atypical, non-standard functions of the microtubule associated Tau protein. Acta Neuropathol Commun. (2017) 5:91. doi: 10.1186/s40478-017-0489-6
49. Brandt R, Trushina NI, Bakota L. Much more than a cytoskeletal protein: physiological and pathological functions of the non-microtubule binding Region of Tau. Front Neurol. (2020) 11:590059. doi: 10.3389/fneur.2020.590059
50. Sinsky J, Majerova P, Kovac A, Kotlyar M, Jurisica I, Hanes J. Physiological Tau interactome in brain and its link to tauopathies. J Proteome Res. (2020) 19:2429–42. doi: 10.1021/acs.jproteome.0c00137
51. Guo T, Noble W, Hanger DP. Roles of tau protein in health and disease. Acta Neuropathol. (2017) 133:665–704. doi: 10.1007/s00401-017-1707-9
52. Merezhko M, Uronen RL, Huttunen HJ. The cell biology of tau secretion. Front Mol Neurosci. (2020) 13:569818. doi: 10.3389/fnmol.2020.569818
53. Mudher A, Colin M, Dujardin S, Medina M, Dewachter I, Alavi Naini SM, et al. What is the evidence that tau pathology spreads through prion-like propagation? Acta Neuropathol Commun. (2017) 5:99. doi: 10.1186/s40478-017-0488-7
54. Kosik KS, Orecchio LD, Bakalis S, Neve RL. Developmentally regulated expression of specific tau sequences. Neuron. (1989) 2:1389–97. doi: 10.1016/0896-6273(89)90077-9
55. Brion JP, Smith C, Couck AM, Gallo JM, Anderton BH. goe. J Neurochem. (1993) 61:2071–80. doi: 10.1111/j.1471-4159.1993.tb07444.x
56. Goedert M, Jakes R. Expression of separate isoforms of human tau protein: correlation with the tau pattern in brain and effects on tubulin polymerization. EMBO J. (1990) 9:4225–30. doi: 10.1002/j.1460-2075.1990.tb07870.x
57. Caceres A, Kosik KS. Inhibition of neurite polarity by tau antisense oligonucleotides in primary cerebellar neurons. Nature. (1990) 343:461–3. doi: 10.1038/343461a0
58. Shea TB, Beermann ML, Nixon RA, Fischer I. Microtubule-associated protein tau is required for axonal neurite elaboration by neuroblastoma cells. J Neurosci Res. (1992) 32:363–74. doi: 10.1002/jnr.490320308
59. Goode BL, Feinstein SC. Identification of a novel microtubule binding and assembly domain in the developmentally regulated inter-repeat region of tau. J Cell Biol. (1994) 124:769–82. doi: 10.1083/jcb.124.5.769
60. Bullmann T, De Silva R, Holzer M, Mori H, Arendt T. Expression of embryonic tau protein isoforms persist during adult neurogenesis in the hippocampus. Hippocampus. (2007) 17:98–102. doi: 10.1002/hipo.20255
61. Llorens-Martin M, Teixeira CM, Fuster-Matanzo A, Jurado-Arjona J, Borrell V, Soriano E, et al. Tau isoform with three microtubule binding domains is a marker of new axons generated from the subgranular zone in the hippocampal dentate gyrus: implications for Alzheimer's disease. J Alzheimers Dis. (2012) 29:921–30. doi: 10.3233/JAD-2012-112057
62. Hong XP, Peng CX, Wei W, Tian Q, Liu YH, Yao XQ, et al. Essential role of tau phosphorylation in adult hippocampal neurogenesis. Hippocampus. (2010) 20:1339–49. doi: 10.1002/hipo.20712
63. Fuster-Matanzo A, De Barreda EG, Dawson HN, Vitek MP, Avila J, Hernandez F. Function of tau protein in adult newborn neurons. FEBS Lett. (2009) 583:3063–8. doi: 10.1016/j.febslet.2009.08.017
64. Gatt A, Lee H, Williams G, Thuret S, Ballard C. Expression of neurogenic markers in Alzheimer's disease: a systematic review and metatranscriptional analysis. Neurobiol Aging. (2019) 76:166–80. doi: 10.1016/j.neurobiolaging.2018.12.016
65. Moreno-Jimenez EP, Flor-Garcia M, Terreros-Roncal J, Rabano A, Cafini F, Pallas-Bazarra N, et al. Adult hippocampal neurogenesis is abundant in neurologically healthy subjects and drops sharply in patients with Alzheimer's disease. Nat Med. (2019) 25:554–60. doi: 10.1038/s41591-019-0375-9
66. Tobin MK, Musaraca K, Disouky A, Shetti A, Bheri A, Honer WG, et al. Human hippocampal neurogenesis persists in aged adults and Alzheimer's disease patients. Cell Stem Cell. (2019) 24:974–82 e973. doi: 10.1016/j.stem.2019.05.003
67. Choi SH, Tanzi RE. Is Alzheimer's disease a neurogenesis disorder? Cell Stem Cell. (2019) 25:7–8. doi: 10.1016/j.stem.2019.06.001
68. Briley D, Ghirardi V, Woltjer R, Renck A, Zolochevska O, Taglialatela G, et al. Preserved neurogenesis in non-demented individuals with AD neuropathology. Sci Rep. (2016) 6:27812. doi: 10.1038/srep27812
69. Van Der Lee SJ, Wolters FJ, Ikram MK, Hofman A, Ikram MA, Amin N, et al. The effect of APOE and other common genetic variants on the onset of Alzheimer's disease and dementia: a community-based cohort study. Lancet Neurol. (2018) 17:434–44. doi: 10.1016/S1474-4422(18)30053-X
70. Jiang M, Vanan S, Tu HT, Zhang W, Zhang ZW, Chia SY, et al. Amyloid precursor protein intracellular domain-dependent regulation of FOXO3a inhibits adult hippocampal neurogenesis. Neurobiol Aging. (2020) 95:250–63. doi: 10.1016/j.neurobiolaging.2020.07.031
71. Pallas-Bazarra N, Jurado-Arjona J, Navarrete M, Esteban JA, Hernandez F, Avila J, et al. Novel function of Tau in regulating the effects of external stimuli on adult hippocampal neurogenesis. EMBO J. (2016) 35:1417–36. doi: 10.15252/embj.201593518
72. Tucker KL, Meyer M, Barde YA. Neurotrophins are required for nerve growth during development. Nat Neurosci. (2001) 4:29–37. doi: 10.1038/82868
73. Dawson HN, Ferreira A, Eyster MV, Ghoshal N, Binder LI, Vitek MP. Inhibition of neuronal maturation in primary hippocampal neurons from tau deficient mice. J Cell Sci. (2001) 114:1179–87.
74. Dioli C, Patricio P, Trindade R, Pinto LG, Silva JM, Morais M, et al. Tau-dependent suppression of adult neurogenesis in the stressed hippocampus. Mol Psychiatry. (2017) 22:1110–8. doi: 10.1038/mp.2017.103
75. Criado-Marrero M, Sabbagh JJ, Jones MR, Chaput D, Dickey CA, Blair LJ. Hippocampal neurogenesis is enhanced in adult tau deficient mice. Cells. (2020) 9:210. doi: 10.3390/cells9010210
76. Terwel D, Lasrado R, Snauwaert J, Vandeweert E, Van Haesendonck C, Borghgraef P, et al. Changed conformation of mutant Tau-P301L underlies the moribund tauopathy, absent in progressive, nonlethal axonopathy of Tau-4R/2N transgenic mice. J Biol Chem. (2005) 280:3963–73. doi: 10.1074/jbc.M409876200
77. Sennvik K, Boekhoorn K, Lasrado R, Terwel D, Verhaeghe S, Korr H, et al. Tau-4R suppresses proliferation and promotes neuronal differentiation in the hippocampus of tau knockin/knockout mice. Faseb J. (2007) 21:2149–61. doi: 10.1096/fj.06-7735com
78. Andorfer C, Kress Y, Espinoza M, De Silva R, Tucker KL, Barde YA, et al. Hyperphosphorylation and aggregation of tau in mice expressing normal human tau isoforms. J Neurochem. (2003) 86:582–90. doi: 10.1046/j.1471-4159.2003.01879.x
79. Komuro Y, Xu G, Bhaskar K, Lamb BT. Human tau expression reduces adult neurogenesis in a mouse model of tauopathy. Neurobiol Aging. (2015) 36:2034–42. doi: 10.1016/j.neurobiolaging.2015.03.002
80. Bolos M, Pallas-Bazarra N, Terreros-Roncal J, Perea JR, Jurado-Arjona J, Avila J, et al. Soluble Tau has devastating effects on the structural plasticity of hippocampal granule neurons. Transl Psychiatry. (2017) 7:1267. doi: 10.1038/s41398-017-0013-6
81. Zheng J, Li HL, Tian N, Liu F, Wang L, Yin Y, et al. Interneuron accumulation of phosphorylated tau impairs adult hippocampal neurogenesis by suppressing GABAergic transmission. Cell Stem Cell. (2020) 26:331–345 e336. doi: 10.1016/j.stem.2020.01.021
82. Richetin K, Steullet P, Pachoud M, Perbet R, Parietti E, Maheswaran M, et al. Tau accumulation in astrocytes of the dentate gyrus induces neuronal dysfunction and memory deficits in Alzheimer's disease. Nat Neurosci. (2020) 23:1567–79. doi: 10.1038/s41593-020-00728-x
83. Schindowski K, Bretteville A, Leroy K, Begard S, Brion JP, Hamdane M, et al. Alzheimer's disease-like tau neuropathology leads to memory deficits and loss of functional synapses in a novel mutated tau transgenic mouse without any motor deficits. Am J Pathol. (2006) 169:599–616. doi: 10.2353/ajpath.2006.060002
84. Schindowski K, Belarbi K, Bretteville A, Ando K, Buee L. Neurogenesis and cell cycle-reactivated neuronal death during pathogenic tau aggregation. Genes Brain Behav. (2008) 7 (Suppl. 1):92–100. doi: 10.1111/j.1601-183X.2007.00377.x
85. Leroy K, Bretteville A, Schindowski K, Gilissen E, Authelet M, De Decker R, et al. Early axonopathy preceding neurofibrillary tangles in mutant tau transgenic mice. Am J Pathol. (2007) 171:976–92. doi: 10.2353/ajpath.2007.070345
86. Ando K, Leroy K, Heraud C, Kabova A, Yilmaz Z, Authelet M, et al. Deletion of murine tau gene increases tau aggregation in a human mutant tau transgenic mouse model. Biochem Soc Trans. (2010) 38:1001–5. doi: 10.1042/BST0381001
87. Ando K, Leroy K, Heraud C, Yilmaz Z, Authelet M, Suain V, et al. Accelerated human mutant tau aggregation by knocking out murine tau in a transgenic mouse model. Am J Pathol. (2011) 178:803–16. doi: 10.1016/j.ajpath.2010.10.034
88. Lim F, Hernandez F, Lucas JJ, Gomez-Ramos P, Moran MA, Avila J. FTDP-17 mutations in tau transgenic mice provoke lysosomal abnormalities and Tau filaments in forebrain. Mol Cell Neurosci. (2001) 18:702–14. doi: 10.1006/mcne.2001.1051
89. Terreros-Roncal J, Flor-Garcia M, Moreno-Jimenez EP, Pallas-Bazarra N, Rabano A, Sah N, et al. Activity-dependent reconnection of adult-born dentate granule cells in a mouse model of frontotemporal dementia. J Neurosci. (2019) 39:5794–815. doi: 10.1523/JNEUROSCI.2724-18.2019
90. Mocanu MM, Nissen A, Eckermann K, Khlistunova I, Biernat J, Drexler D, et al. The potential for beta-structure in the repeat domain of tau protein determines aggregation, synaptic decay, neuronal loss, and coassembly with endogenous Tau in inducible mouse models of tauopathy. J Neurosci. (2008) 28:737–48. doi: 10.1523/JNEUROSCI.2824-07.2008
91. Joseph M, Anglada-Huguet M, Paesler K, Mandelkow E, Mandelkow EM. Anti-aggregant tau mutant promotes neurogenesis. Mol Neurodegener. (2017) 12:88. doi: 10.1186/s13024-017-0230-8
92. Harada A, Oguchi K, Okabe S, Kuno J, Terada S, Ohshima T, et al. Altered microtubule organization in small-calibre axons of mice lacking tau protein. Nature. (1994) 369:488–91. doi: 10.1038/369488a0
93. Ikegami S, Harada A, Hirokawa N. Muscle weakness, hyperactivity, and impairment in fear conditioning in tau-deficient mice. Neurosci Lett. (2000) 279:129–32. doi: 10.1016/S0304-3940(99)00964-7
94. Biundo F, Del Prete D, Zhang H, Arancio O, D'adamio L. A role for tau in learning, memory and synaptic plasticity. Sci Rep. (2018) 8:3184. doi: 10.1038/s41598-018-21596-3
95. De Barreda EG, Dawson HN, Vitek MP, Avila J. Tau deficiency leads to the upregulation of BAF-57, a protein involved in neuron-specific gene repression. FEBS Lett. (2010) 584:2265–70. doi: 10.1016/j.febslet.2010.03.032
96. Boekhoorn K, Joels M, Lucassen PJ. Increased proliferation reflects glial and vascular-associated changes, but not neurogenesis in the presenile Alzheimer hippocampus. Neurobiol Dis. (2006) 24:1–14. doi: 10.1016/j.nbd.2006.04.017
97. Zhao X, Van Praag H. Steps towards standardized quantification of adult neurogenesis. Nat Commun. (2020) 11:4275. doi: 10.1038/s41467-020-18046-y
98. Chen Q, Nakajima A, Choi SH, Xiong X, Sisodia SS, Tang YP. Adult neurogenesis is functionally associated with AD-like neurodegeneration. Neurobiol Dis. (2008) 29:316–26. doi: 10.1016/j.nbd.2007.09.005
99. Yagi S, Splinter JEJ, Tai D, Wong S, Wen Y, Galea L, et al. Sex differences in maturation and attrition of adult neurogenesis in the hippocampus. eNeuro. (2020) 7. doi: 10.1523/ENEURO.0468-19.2020
100. West MJ, Slomianka L, Gundersen HJ. Unbiased stereological estimation of the total number of neurons in thesubdivisions of the rat hippocampus using the optical fractionator. Anat Rec. (1991) 231:482–97. doi: 10.1002/ar.1092310411
101. Noori HR, Fornal CA. The appropriateness of unbiased optical fractionators to assess cell proliferation in the adult hippocampus. Front Neurosci. (2011) 5:140. doi: 10.3389/fnins.2011.00140
Keywords: neurogenesis, tauopathy, Alzheimer's disease, dentate gyrus, tau
Citation: Houben S, Homa M, Yilmaz Z, Leroy K, Brion J-P and Ando K (2021) Tau Pathology and Adult Hippocampal Neurogenesis: What Tau Mouse Models Tell us? Front. Neurol. 12:610330. doi: 10.3389/fneur.2021.610330
Received: 25 September 2020; Accepted: 06 January 2021;
Published: 10 February 2021.
Edited by:
Sonia Do Carmo, McGill University, CanadaReviewed by:
Chaur-Jong Hu, Taipei Medical University, TaiwanCopyright © 2021 Houben, Homa, Yilmaz, Leroy, Brion and Ando. This is an open-access article distributed under the terms of the Creative Commons Attribution License (CC BY). The use, distribution or reproduction in other forums is permitted, provided the original author(s) and the copyright owner(s) are credited and that the original publication in this journal is cited, in accordance with accepted academic practice. No use, distribution or reproduction is permitted which does not comply with these terms.
*Correspondence: Kunie Ando, S3VuaWUuQW5kb0B1bGIuYWMuYmU=
Disclaimer: All claims expressed in this article are solely those of the authors and do not necessarily represent those of their affiliated organizations, or those of the publisher, the editors and the reviewers. Any product that may be evaluated in this article or claim that may be made by its manufacturer is not guaranteed or endorsed by the publisher.
Research integrity at Frontiers
Learn more about the work of our research integrity team to safeguard the quality of each article we publish.