- 1Applied Technology for Neuro-Psychology Lab, Istituto Auxologico Italiano, Istituto di Ricovero e Cura a Carattere Scientifico, Milan, Italy
- 2Department of Psychology, E-Campus University, Novedrate, Italy
- 3Department of Psychology, Catholic University of the Sacred Heart, Milan, Italy
Mild cognitive impairment (MCI) refers to a subtle, general cognitive decline with a detrimental impact on elderlies' independent living and quality of life. Without a timely diagnosis, this condition can evolve into dementia over time, hence the crucial need for early detection, prevention, and rehabilitation. For this purpose, current neuropsychological interventions have been integrated with (i) virtual reality, which immerses the user in a controlled, ecological, and safe environment (so far, both virtual reality-based cognitive and motor rehabilitation have revealed promising positive outcomes); and (ii) non-invasive brain stimulation, i.e., transcranial magnetic or electric brain stimulation, which has emerged as a promising cognitive treatment for MCI and Alzheimer's dementia. To date, these two methods have been employed separately; only a few studies (limited to motor rehabilitation) have suggested their integration. The present paper suggests to extend this integration to cognitive rehabilitation as well as to provide a multimodal stimulation that could enhance cognitive training, resulting in a more efficient rehabilitation.
Introduction
To a certain degree, cognitive decline is a physiological change occurring during the aging process that occasionally evolves into a subtle condition known as mild cognitive impairment (MCI) (1). Despite being undiagnosable as proper dementia, at least following a categorical approach, this condition can have a detrimental impact on elderlies' cognitive functioning and worsen their conditions over time, even up to a point where an elderly presents with a frank dementia. However, MCI is also likely to either revert back to normal cognition or stabilize over time (2).
Both MCI patients and their caregivers frequently report concerns about worsening cognition in areas such as everyday memory, language, visuospatial skills, planning, organization, and divided attention (3). The decline in cognitive functioning negatively affects elderlies' independent living and their ability to safely and autonomously carry out instrumental activities of daily life (IADLs), an assessment instrument that measures an individual's ability to perform daily activities (4) such as grocery shopping, managing medications and/or money, and housework. In fact, MCI individuals are less able to perform IADLs than their healthy counterparts (5), with detrimental effects on their wellbeing (3, 6) and an increased risk of developing dementia (7). Since activity restriction underlies the expression of cognitive impairment in daily life, IADLs might enable the detection of early deficits experienced during daily activities beyond those captured by neuropsychological tests (8).
MCI is most commonly referred to as a degenerative etiology (i.e., Alzheimer's disease [AD], frontotemporal dementia, dementia with Lewy bodies), but vascular (i.e., vascular cognitive impairment), psychiatric (e.g., depression), genetic (APOE and TOMM40 genes) and other medical conditions (e.g., uncompensated heart failure, poorly controlled diabetes mellitus, or chronic obstructive pulmonary disease) can also contribute to the determination of cognitive impairment (9, 10). Clinicians classify MCI into broadly differentiated subtypes—amnestic (aMCI) and non-amnestic (naMCI)—based on whether the condition impairs one or multiple cognitive domains (1). aMCI refers to patients who exhibit episodic memory impairments as confirmed by neuropsychological tests and is associated with higher risk of further conversion to AD (11, 12). naMCI refers to patients with neuropsychological deficits in non-memory cognitive domains (12).
Neurobiological studies have revealed that cognitive impairment affecting memory (e.g., episodic memory) and other domains (e.g., executive control, language, or visuospatial abilities) is associated with altered neural activity in prodromal AD (i.e., aMCI): the entorhinal cortex and hippocampus are first affected by histopathological changes, followed by the parahippocampal gyrus, the temporal pole, and the inferior and middle temporal gyri (9, 12–16). While primary cortices seem to be less vulnerable to deterioration, associative areas are the most compromised: among them, the prefrontal cortex (PFC) shows a higher decline (17). Discriminating between normal and pathological neural changes is crucial in order to formulate an accurate diagnosis and a prompt treatment plan (18).
The conversion to dementia usually occurs within 3 years after the diagnosis of MCI, and this rate critically drops in the following years (19). Therefore, a delayed intervention could be ineffective when the cognitive decline is close to the dementia stage (20). Furthermore, the timing of the intervention also affects cost-effectiveness: therefore intervening 2 years prior to standard diagnosis would allow the maximum net benefit of the disease-modifying intervention (19).
Thus, this long “intermediate” phase provides a critical opportunity for therapeutic intervention. Cognitive interventions for MCI usually encompass a variety of approaches, heterogeneous in terms of methods and contents. Among them, cognitive training could be considered as a secondary prevention method, particularly for “at risk” groups. It generally consists of theoretically driven skills and strategies which guide and encourage patients to perform tasks engaging several cognitive domains (21). Previous studies have showed that, on one hand, MCI patients show impairments in everyday memory, language, visuospatial skills, planning, organization, and divided attention affecting daily activities, as also confirmed by worse scores in IADL (7, 12); on the other hand, MCI patients could exhibit different neural impairments, as previously mentioned (12).
Considering that MCI is characterized by both cognitive-behavioral and neural impairments, a successful rehabilitation process should address both of them and could benefit from the integration of technological advancements. A plausible candidate could be virtual reality (VR) due to its psychological and technological features: VR scenarios simulate daily life situations in which the user can feel immersed and interact with an environment updated in real-time, while also receiving dynamic multisensory feedback (21–25). A recent systematic meta-analysis showed that specific VR environments built on principles of neurorehabilitation that potentially enhance learning and recovery seem more efficacious than non-specific VR-based treatments or conventional therapies (26). Non-invasive brain stimulation (NIBS) including transcranial magnetic stimulation (TMS) has been showed to be efficacious for cognitive rehabilitation as well (27).
The widespread of neurodegenerative diseases have increased the demand for the development of new techniques to support the rehabilitation. Since the recovery is complex, there is a growing interest in the development of new technologies for improving outcomes of conventional clinical intervention strategies. The aim of the present paper is two-fold: first, to provide a brief review of current evidence regarding the benefits of non-invasive technologies (VR and TMS) on MCI cognitive rehabilitation. Second, to propose an integrated intervention approach consisting of VR-based cognitive training and neural stimulation by means of TMS. The integration of existing technologies does not replace MCI's standard rehabilitative methods, but rather upgrades them in order to create a novel approach that guarantees an ecological setting and takes action on different aspects of this clinical entity, thereby fostering cognitive improvements. Therefore, the present paper aims to propose a new integrated, multimethod approach acting on both a neural-cognitive and behavioral-cognitive level for MCI by means of VR and TMS.
A New Integrated Approach
This section will be structured as follows: (i) the features of two existing interventions for MCI (i.e., VR and TMS) will be summarized, as well as (ii) the recent literature about their integration in motor rehabilitation of MCI and other clinical applications; (iii) finally, a discussion of a novel approach integrating these methods for MCI cognitive rehabilitation.
Virtual Reality in Rehabilitation
Available methods for MCI rehabilitation consist of cognitive stimulation or cognitive training, usually in the paper-and-pencil format and conducted in an isolated and non-ecological setting (28), consisting, for example, of exercises of categorization, semantic association, classification and mental imagery according to specific goals (memory for proper names, object location, etc.) (28). Recently, new technologies have been increasingly implemented in clinical settings: VR is an immersive technology using 3D computer generated environments. When a user is immersed in virtual reality, he/she experiences the sense of “being there” inside the virtual environment while knowing for sure that he/she is not (29, 30); this allows to recreate lifelike contexts in an ecological, safe and controlled setting (31–34). The sense of presence can be considered as a neuropsychological phenomenon resulting from our biological inheritance and our experience as active agents in our surrounding environment. Fully immersive VR scenarios create a strong sense of Place Illusion and Plausibility Illusion for the user, and result in realistic emotional reactions to the situations encountered in VR, perceptual accuracy, and a strong sense of agency and control over the virtual environment (34–36). These crucial features have fostered the widespread employment of VR in clinical rehabilitation (22, 25, 37, 38). Depending on the degree of immersiveness, VR devices can fall into three categories: non-immersive (e.g., user interacts with the environment with a keyboard and mouse); semi-immersive (e.g., user usually stands in front of a large screen, and gesture and location can be tracked); and fully-immersive [e.g., user wears a head-mounted display (HMD) that involves the entire vision or is immersed in the cave automatic virtual environment (CAVE), a four-walled virtual environment that provides a stronger sense of presence). VR also allows users to interact with virtual objects and to receive multisensory feedback (e.g., visual, auditory, kinesthetic) corresponding to that received in real life through the sensory system (39, 40). Synchronization of the different stimuli corresponding to different sensory streams allows the user to experience the virtual environment as realistic and results in realistic behaviors of users experiencing place illusion (41). Indeed, place illusion is defined as “the illusion of being in a place in spite of the sure knowledge that you are not there” and it differs from the plausibility illusion which is defined as “the illusion that what is apparently happening is really happening, in spite of the sure knowledge that it is not” (42, 43). The behavioral correlate of these illusions is that the user behaves in the virtual environments as he would do in the real world (44). This important feature of VR is what distinguishes it from all other types of media. Moreover, VR allows personalized therapies in a controlled way by modulating difficulty level, environments (e.g., adding or removing cues) and modality of interaction tailored to the patient's needs. The possibility of creating safe, ecological, and standardized settings has supported the employment of VR in neurorehabilitation because it allows cognitive trainings that are relevant for real contexts (22, 45, 46), supported by its potential to promote neuroplasticity (47–50). With respect to MCI, various studies have showed VR's potential to enhance cognitive functions [for reviews, see (36, 38)]. For instance, Optale and colleagues (51) showed that 36 sessions of VR-based memory training in a fully immersive environment (provided by an HMD and enriched by visual and auditory stimuli) improved patients' post-treatment Mini Mental State Examination (MMSE) scores compared to the control group—receiving musical therapy intervention—whose MMSE scores decreased instead. Moreover, memory showed improvements as well, as assessed by digit span forward and verbal story recall (51). Similar promising results were observed in an MCI sample after non-immersive VR sessions consisting of performing tasks and navigating a virtual home and supermarket (52). Patients exhibited significant improvements on the Montreal Cognitive Assessment (MoCA) and IADL after a 3-week VR-based cognitive and physical training: interestingly, after the VR intervention, functional near-infrared spectroscopy (NIRS) revealed decreased brain activation of the prefrontal areas as a result of increased neural efficiency during the training (53). Overall, several studies have highlighted the potential of VR in memory (54–57) and executive functions rehabilitation (58–61). In particular, it has been shown that the multisensory stimulation has a positive impact on both the sense of presence and memory functioning (62). At the same time, by creating complex and ecological environments, VR provides the possibility to train different executive functions (e.g., visual attention, planning, problem solving) along with motor demands, enhancing cognitive functions in daily living (59).
In fact, according to the compensatory model, demented brains show broader activation as a compensatory strategy to preserve intact cognitive functions (63, 64). Therefore, this study expands previous literature about VR efficacy to improve neural efficiency in prefrontal areas (53). Besides cognitive enhancement, VR treatments have beneficial psychological effects: participants reported feeling more enthusiastic, relaxed, energetic and, most importantly, less worried, stressed, and anxious (65). Despite these promising results, however, the heterogeneity of the studies, in terms of VR devices' different degrees of immersivity and the variety of protocols, makes it difficult to clarify the mechanisms underlying VR's effectiveness. A recent meta-analysis (66) suggests three plausible mechanisms. (i) Enjoyment: VR provides fun and engaging experiences with different tasks (e.g., exploration, challenges) that motivate patients to complete them. Conversely, patients perceive conventional rehabilitation methods, consisting of repeated behaviors without immediate feedback, as repetitive and boring. (ii) Physical fidelity: VR offers realistic scenarios that allow users to perform and practice behaviors resembling daily activities, whereas traditional rehabilitation programs focus on non-familiar behaviors. (iii) Cognitive fidelity: VR environments can be built according to specific cognitive tasks and the cognitive load required by the transfer environment. On the contrary, conventional rehabilitation is set in a relatively stimulus-free environment with limited cognitive fidelity.
Transcranial Magnetic Stimulation (TMS) in Rehabilitation
TMS is a form of non-invasive brain stimulation that induces an electrical field through a coil placed on the surface of the scalp over a targeted stimulation site (67). Depending on selected parameters (i.e., frequency, intensity, number of pulses delivered, type of coil, and location of the stimulation), TMS pulses can either excite or inhibit cortical activity and induce long- or short-term neural and behavioral changes (68). Depending on the number of pulses delivered, TMS can be single-pulse (i.e., when only one stimulus at a time is employed), paired-pulse (i.e., when pairs of stimuli separated by an interval are delivered) or repetitive (rTMS) in case of trains of stimuli (69).
TMS has increasingly been applied in several research and clinical fields (70), including in cognitive rehabilitation of MCI and dementia (27, 71–73). One study (27) reviewed the potential of TMS in modulating cognitive functions both in MCI and AD: the majority of the studies employed multiple sessions of high-frequency (>5 Hz) rTMS over the dorsolateral prefrontal cortex (DLPFC). Overall, TMS appeared effective at significantly improving memory and executive functions. Another study (72) reported long-term memory and executive functioning improvements after 10 high-frequency rTMS sessions over the left DLPFC. One study (74) considered the effectiveness of rTMS over the DLPCF at reducing apathy, a symptom frequently reported in MCI patients: a significant improvement in apathy scores resulted following 10 sessions of active TMS compared to sham. Interestingly, authors observed positive outcomes in executive functions as well, as assessed by the Trail Making Test (75). Cognitive benefits resulting from TMS interventions can be explained by the reorganization of the brain networks following the induced changes in cortical excitability. In other words, high-frequency rTMS sessions may determine an improvement in terms of synaptic plasticity, with implications for reorganization of cognitive domains (76, 77).
However, the mechanisms underlying TMS effects are still unclear. One hypothesis is that high-frequency TMS induces intracortical inhibition. In other words, discharging an electrical field causes gamma-aminobutyric acid (GABA) levels to increase, suppressing the activity (78). This temporary neuro-disruption, called a “virtual lesion,” causes a disruption of perceptual, motor, and cognitive processes in the human brain (79). Another hypothesis is that TMS might determine a random neural noise by amplifying the background activity (80). Other authors suggested that TMS could disrupt the temporal relation between neurons implicated in a more extended circuit activated by the task (81). Overall, the effects of TMS are heterogeneous and seemingly dose- and context-dependent. On one hand, the effectiveness of TMS depends on the frequency and duration of the stimulation: its effects are more pronounced as long as both TMS trains and frequency increase. On the other hand, the effects depend on the level of cortical excitability at the moment of the stimulation: the pulse recruits as many neurons are close to the firing threshold (76). Overall, the effectiveness of TMS remains hindered by a number of methodological challenges, including a lack of clear consensus about the optimal stimulation parameters, with variability in the type, frequency, intensity, location, and duration of stimulation.
Virtual Reality and Non-invasive Brain Stimulation
The joint application of NIBS and VR has been previously investigated in different clinical settings to improve the clinical outcomes of conventional therapies. VR provides a controlled, ecological, and appealing setting that could be personalized according to the patient needs, whereas NIBS might alter the neurophysiology underpinning cognitive functions. For this purpose, different studies have suggested that the combination of these technologies could be more synergic than stand-alone treatments. For instance, it has been employed to induce embodiment for an artificial hand (82), to treat spider phobia (83) and in interventions in different populations, such as children with cerebral palsy, post-stroke patients, and healthy people (84). In rehabilitation settings, different studies have investigated the potential of joining VR and transcranial direct current stimulation (tDCS)/TMS for the rehabilitation of the upper limb, one of the most common deficits following a stroke (84–89). Kim and colleagues (90) found that VR wrist exercise after tDCS had greater immediate and sustained corticospinal facilitation effects than exercise without tDCS and tDCS without exercise. Furthermore, this corticospinal facilitation lasted for 20 min after the exercise in the VR+tDCS condition compared to the control groups. Recently, a meta-analysis (88) proved the effectiveness and suitability of NIBS-VR integration for motor rehabilitation of the upper limb. While different studies have proved the efficacy of the joint application of NIBS and VR for motor rehabilitation, to our best knowledge, no studies have investigated the same approach for cognitive rehabilitation.
Virtual Reality and Transcranial Magnetic Stimulation for Cognitive Rehabilitation
This section discusses an integrated intervention approach that encompasses both TMS and a training VR for cognitive rehabilitation of MCI.
VR interventions showed positive outcomes in cognitive and motor functioning in patients with MCI or dementia, as reported by a recent meta-analysis (46). Despite the mechanisms underlying the application of TMS for cognitive rehabilitation being uncertain, heterogeneous, and ambivalent, studies targeting cognitive rehabilitation suggested that aMCI and AD patients benefited from its employment (27). Therefore, a plausible hypothesis is that high-frequency rTMS over the left DLPFC might recruit more neural resources from the prefrontal cortex by inducing an electrophysiologically excitatory effect. This stimulation could also enhance the efficiency of resources to deploy for conflict resolution during multiple stages of cognitive control processing. In other words, rTMS could induce a greater activation and efficacy of the prefrontal cortex (91), an area that is involved in accomplishing the VR tasks. In fact, an eclectic approach to cognitive rehabilitation achieves greater improvements based on the assumption that cognitive deficits are also determined and influenced by physical (e.g., illness, blood pressure, pain, sleep), emotional (e.g., anxiety, annoyances, arousal, mood), social (e.g., relationships, status, social pressure) and motivational (e.g., distractibility, goals, incentives) aspects (92). Specifically, a plausible integrated intervention could include 10 training sessions of 40 min, composed of rTMS (active or sham) and the virtual-based training. Before the first session and at the end of the tenth, aMCI patients will receive a neuropsychological assessment. First, high-frequency rTMS will be delivered over the left DLPCF, a region known to be involved in executive functions and in long-term memory due to its interaction with the medial-temporal network, including the hippocampus (93–95). After each session of rTMS, patients wearing 3-D glasses will be immersed inside a CAVE1, a virtual room-sized cube, at I.R.C.C.S. Istituto Auxologico Italiano (Milan, Italy), in which they will be exposed to two different environments (96). Patients will be first immersed in a virtual supermarket (Figure 1) in which they would be able to move around thanks to an Xbox controller. Tasks will consist of selecting different products on shelves according to precise rules, with increasing difficulty. Every task, according to rules and goals, will require both executive (e.g., planning, problem solving, and divided attention) and memory functioning (e.g., remembering rules). Patients will be then immersed in a virtual city (Figure 2) in which they will be required to perform two tasks. At the beginning, they will be placed in the center of a square and asked to move around in the virtual city, looking for a target object previously identified with the therapist. Then, they will be placed in a random location in the city and asked to retrieve the position of that object. This city task will aim to enhance spatial memory, navigation, and planning strategies.
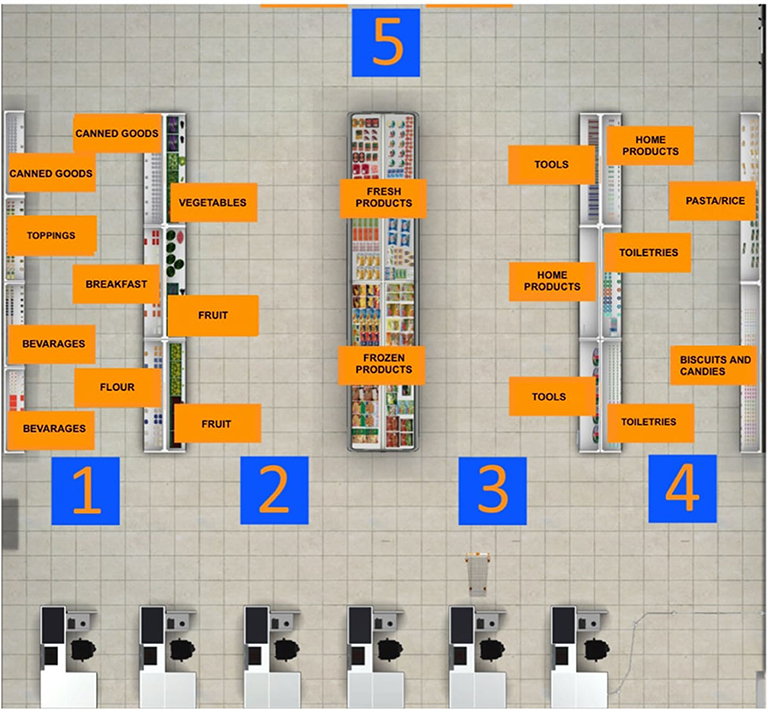
Figure 1. Virtual supermarket task map. Before starting the navigation into the virtual supermarket, this map is projected on one of the four walls of the CAVE. The patient has to memorize the position of every category of products he/she has to buy.
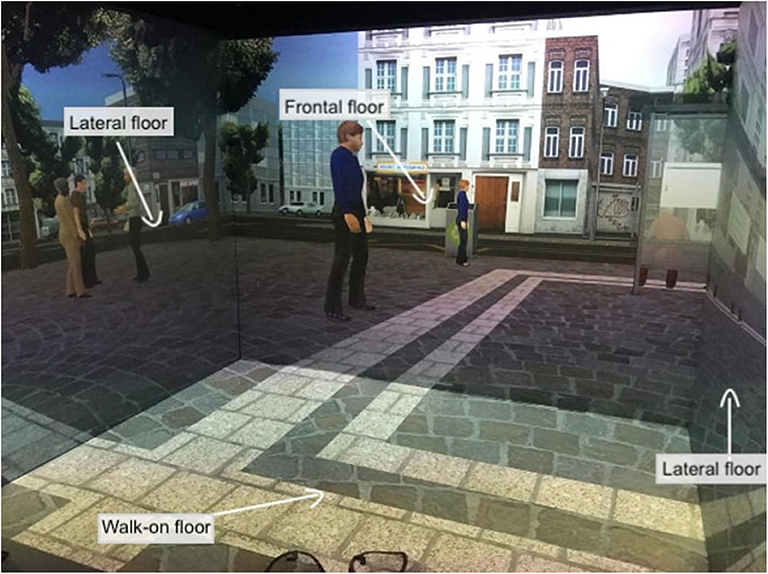
Figure 2. Virtual city task. This figure shows the patient's point of view when immersed in the 3-D CAVE. The projections on the four floors move synchronously with the user's navigation through the controller.
The neuropsychological assessment will target general cognitive functioning through Addenbrooke's Cognitive Examination (ACE-R) (97) and MoCA (98). Executive functioning (planning, initiating, and monitoring) will be assessed with the Trail Making Test (TMT version A and B) (75) and the Stroop test (99). Memory abilities will be evaluated by Digit Span (100) and Babcock (101). Visuo-spatial abilities will be evaluated by Tower of London (ToL) (102). Mood will be assessed by the State-Trait Anxiety Inventory (STAI) (103) and Geriatric Depression Scale (GDS) (104). Lastly, activities of daily living (ADL) (105) and IADL (4) will be collected.
During the entire intervention, physiological measures (e.g., heart rate variability, skin conductance) will be collected.
Discussion and Conclusion
MCI is a transitional subclinical entity creating a fine line between normal and pathological aging. Thus, early interventions are essential to preserving cognitive functioning and, as far as possible, to decelerating its evolution toward dementia. MCI may benefit from an efficacious intervention deeming that the brain might still be able to compensate for its deficiencies and to support the acquisition and retention of the impaired cognitive functions. With the progression of pathological conditions and the spreading of lesions instead, the brain might no longer be able to compensate (106, 107). Thus, a prompt rehabilitation might be helpful in delaying the progression to dementia. Considering that both VR-based training and neuromodulation capitalize on neuroplasticity, they can enhance the therapeutic mechanisms in a complementary way. On one hand, rTMS aims to increase excitability within the lesioned hemisphere and to suppress stimulation to the contralesional hemisphere, namely, reducing inter-hemispheric inhibition from the contralesional side (108–111). Specifically in aMCI patients, the DLPFC is characterized by abnormal functional connectivity, determining several cognitive and emotional impairments (112). Stimulation of the prefrontal cortex is expected to enhance activation and efficiency in this area responsible for both executive functions (e.g., working memory and flexibility) and long-term memory due to its connection with the medial-temporal network (e.g., the hippocampus) (93–95). On the other hand, VR-based intervention will provide patients with lifelike functional tasks (like doing groceries and walking around the city) that involve cognitive domains, physical activity, and emotional–behavioral aspects. Given the potential of VR to provide an ecological and immersive setting, along with immediate feedback, the repetitive practice of these functional tasks would facilitate a complex cognitive processing strengthened by enjoyment and attractiveness, which might facilitate motivation and engagement. Patients would be required to tap into their attentional, mnemonic, planning, flexibility, and navigation abilities to accomplish the virtual tasks. It is plausible to expect that this multi-session, multi-modal intervention would facilitate the transfer of these abilities to real-life daily activities as well. Furthermore, elderlies' enjoyment could promote their engagement and treatment adherence.
The integration of VR and TMS may allow a more sensitive rehabilitation of cognitive symptoms while simultaneously modulating the impaired neural circuits to provide a stronger beneficial effect. It is plausible that the implementation of neural modulation within an ecological virtual environment may allow elderlies to benefit even more than stand-alone intervention. Besides, available interventions for MCI are frequently conducted in isolated and artificial situations, thus allowing evaluation biases.
The sense of presence, i.e., the subjective sense of being there in a virtual environment rather than in the actual physical environment, is central in a VR experience. As the other subjective feeling states, presence depends on a set of predictions about the interoceptive state of the body (113). In this regard, predictive coding theory can be used to describe the relationship between top-down prediction/expectation signals and bottom-up prediction error signals. In immersive environments, the experience of being there is based on the synchronization between expected and actual sensorimotor signals, leveraging a prediction-based model of behavior (114). In this way, immersive environments could allow to foster cognitive modeling/change, by providing realistic life-like multisensory experiences. According to it, we expect that neural and cognitive manipulation through rTMS and VR, respectively, might yield more beneficial outcomes than standard intervention both in paper-and-pencil and computer methods. Similarly to previous results, we expect that this integrated approach would determine improvements in general cognitive, memory, visuospatial, and particularly executive functioning, as well as in IADL and ADL scores for elderlies affected by MCI. In fact, both memory and executive impairments are associated with greater ADL/IADL worsening (115). VR intervention might possibly enhance complex cognitive processing as patients repetitively practiced IADL-based functional tasks. This hypothesis is in accordance with recent literature supporting the advantages of VR in improving global cognition and IADL (53).
Moreover, by collecting physiological indexes, it would be possible to record implicit measures of internal states during the whole experience, evaluating the impact of specific experiences without interfering with them (116). Indeed, biosensors are considered a reliable method for quantitative and objective measurement of the psychophysiological signals the and behavior of participants. The potential of these measures is that they provide additional information that could deepen the understanding of peculiar patterns (116).
Nevertheless, a study based on this approach is not exempt from limitations: for instance, the different stages of aMCI patients' functional levels could provide heterogeneous results. Also, some patients might not be able to complete the intervention due to dizziness or cybersickness [i.e., motion sickness including eye fatigue, nausea, headaches, and sweating (91, 92)] when immersed in virtual environments, although studies have revealed that VR is generally well-tolerated by the elderly (101). TMS could provoke discomfort and headache as well (69). Furthermore, the heterogeneity of neural impairments of MCI and the unclear beneficial effects of TMS might influence the effectiveness of this integrated approach. However, previous studies have showed promising results in the integration of neuromodulation and VR technologies for motor rehabilitation in stroke patients (85, 90). On one hand, TMS enabled shifts in cortical activity from contralesional to ipsilesional motor areas; on the other hand, VR provided repetitive, intensive, and motivating movement tasks with real-time multimodal feedback, applying motor learning principles for stroke neurorehabilitation (49, 117).
Consistent with both empirical evidence and scientific background, we thus expect that the combination of two approaches (TMS + VR) tapping into the same mechanisms will yield deeper and longer clinical outcomes in MCI patients.
Author Contributions
VM and CS-B conceived, defined, and wrote the first draft of the perspective. GR supervised the study. All authors revised the final version of the manuscript.
Funding
This work was supported by the Italian-founded project High-End and Low-End Virtual Reality systems for the rehabilitation of Frailty in the Elderly (PE-2013-02355948).
Conflict of Interest
The authors declare that the research was conducted in the absence of any commercial or financial relationships that could be construed as a potential conflict of interest.
Footnote
1. ^The CAVE is a virtual room-sized cube in which the 3-D visualization of the virtual environments occurs thanks to the combination of four stereoscopic projectors and four screens. Two graphics workstations, mounting Nvidia Quadro K6000 GPU with dedicated Quadro Sync cards, are responsible for the projection surfaces, user tracking and functional logic. A Vicon motion tracking system with four infrared cameras allows the tracking of specific reflective markers positioned on target objects and a correct reading of the simulated spaces and distances with a 1:1 scale ratio, thus enhancing the feeling of being immersed in the virtual scene.
References
1. Petersen RC. Mild cognitive impairment as a diagnostic entity. J Intern Med. (2004) 256:183–94. doi: 10.1111/j.1365-2796.2004.01388.x
2. Koepsell TD, Monsell SE. Reversion from mild cognitive impairment to normal or near-normal cognition; risk factors and prognosis. Neurology. (2012) 79:1591–8. doi: 10.1212/WNL.0b013e31826e26b7
3. Farias ST, Mungas D, Reed BR, Harvey D, Cahn-Weiner D, DeCarli C. MCI is associated with deficits in everyday functioning. Alzheimer Dis Assoc Disord. (2006) 20:217–23. doi: 10.1097/01.wad.0000213849.51495.d9
4. Lawton MP, Brody EM. Assessment of older people: self-maintaining and instrumental activities of daily living. Gerontologist. (1969) 9:179–86. doi: 10.1093/geront/9.3_Part_1.179
5. Nygård L. Instrumental activities of daily living: a stepping-stone towards alzheimer's disease diagnosis in subjects with mild cognitive impairment? Acta Neurol Scand Suppl. (2003) 107:42–6. doi: 10.1034/j.1600-0404.107.s179.8.x
6. Gold DA. An examination of instrumental activities of daily living assessment in older adults and mild cognitive impairment. J Clin Exp Neuropsychol. (2012) 34:11–34. doi: 10.1080/13803395.2011.614598
7. Jekel K, Damian M, Wattmo C, Hausner L, Bullock R, Connelly PJ, et al. Mild cognitive impairment and deficits in instrumental activities of daily living: a systematic review. Alzheimer's Res Ther. (2015) 7:17. doi: 10.1186/s13195-015-0099-0
8. Pérés K, Chrysostome V, Fabrigoule C, Orgogozo JM, Dartigues JF, Barberger-Gateau P. Restriction in complex activities of daily living in mci: Impact Outcome. (2006) 67:461–7. doi: 10.1212/01.wnl.0000228228.70065.f1
9. Petersen RC. Mild cognitive impairment. Contin Lifelong Learn Neurol. (2016) 22, 404–18. doi: 10.1212/CON.0000000000000313
10. Roses AD, Lutz MW, Amrine-Madsen H, Saunders AM, Crenshaw DG, Sundseth SS, et al. A TOMM40 variable-length polymorphism predicts the age of late-onset Alzheimer's disease. Pharmacogenomics J. (2010) 10:375–84. doi: 10.1038/tpj.2009.69
11. Grundman M, Petersen RC, Ferris SH, Thomas RG, Aisen PS, Bennett DA, et al. Mild cognitive impairment can be distinguished from alzheimer disease and normal aging for clinical trials. Arch Neurol. (2004) 61:59–66. doi: 10.1001/archneur.61.1.59
12. Vega JN, Newhouse PA. Mild cognitive impairment : diagnosis, longitudinal course, and emerging treatments. Curr Psychiatry Rep. (2014) 16:490–8. doi: 10.1007/s11920-014-0490-8
13. Pennanen C, Kivipelto M, Tuomainen S, Hartikainen P, Hänninen T, Laakso MP, et al. Hippocampus and entorhinal cortex in mild cognitive impairment and early AD. Neurobiol Aging. (2004) 25:303–10. doi: 10.1016/S0197-4580(03)00084-8
14. Schmidt-Wilcke T, Poljansky S, Hierlmeier S, Hausner J, Ibach B. Memory performance correlates with gray matter density in the ento-/perirhinal cortex and posterior hippocampus in patients with mild cognitive impairment and healthy controls—a voxel based morphometry study. Neuroimage. (2009) 47:1914–20. doi: 10.1016/j.neuroimage.2009.04.092
15. Fennema-Notestine C, Hagler DJ Jr, McEvoy LK, Fleisher AS, Wu EH, Karow DS, et al. Structural MRI biomarkers for preclinical and mild Alzheimer's disease. Hum Brain Mapp. (2009) 30:3238–53. doi: 10.1002/hbm.20744
16. McDonald CR, McEvoy LK, Gharapetian L, Fennema-Notestine C, Hagler DJ, Holland D, et al. Regional rates of neocortical atrophy from normal aging to early Alzheimer disease. Neurology. (2009) 73:457–65. doi: 10.1212/WNL.0b013e3181b16431
17. Raz N, Rodrigue KM. Differential aging of the brain: patterns, cognitive correlates and modifiers. Neurosci Biobehav Rev. (2006) 30:730–48. doi: 10.1016/j.neubiorev.2006.07.001
18. Leal SL, Yassa MA. Perturbations of neural circuitry in aging, mild cognitive impairment, and Alzheimer's disease. Ageing Res Rev. (2013) 12:823–31. doi: 10.1016/j.arr.2013.01.006
19. Sun Z, Van De Giessen M, Lelieveldt BPF, Staring M. Detection of conversion from mild cognitive impairment to Alzheimer's disease using longitudinal brain MRI. Front Neuroinform. (2017) 11:16. doi: 10.3389/fninf.2017.00016
20. Barnett JH, Lewis L, Blackwell AD, Taylor M. Early intervention in Alzheimer's disease: a health economic study of the effects of diagnostic timing. BMC Neurol. (2014) 14:101. doi: 10.1186/1471-2377-14-101
21. Mowszowski L, Batchelor J, Naismith SL. Early intervention for cognitive decline : can cognitive training be used as a selective prevention technique? Int Psychogeriatrics. (2010) 22:537–48. doi: 10.1017/S1041610209991748
22. Massetti T, da Silva TD, Crocetta TB, Guarnieri R, de Freitas BL, Bianchi Lopes P, et al. The clinical utility of virtual reality in neurorehabilitation: a systematic review. J Cent Nerv Syst Dis. (2018) 10:1179573518813541. doi: 10.1177/1179573518813541
23. Faria AL, Cameirão MS, Couras JF, Aguiar JRO, Costa GM, Bermúdez i Badia S. Combined cognitive-motor rehabilitation in virtual reality improves motor outcomes in chronic stroke—a pilot study. Front Psychol. (2018) 9:854. doi: 10.3389/fpsyg.2018.00854
24. Grechuta K, Rubio Ballester B, Espín Munne R, Usabiaga Bernal T, Molina Hervás B, Mohr B, et al. Augmented dyadic therapy boosts recovery of language function in patients with nonfluent aphasia: a randomized controlled trial. Stroke. (2019) 50:1270–4. doi: 10.1161/STROKEAHA.118.023729
25. Garrett B, Taverner T, Gromala D, Tao G, Cordingley E, Sun C. virtual reality clinical research: promises and challenges. JMIR Serious Games. (2018) 6:e10839. doi: 10.2196/preprints.10839
26. Maier M, Rubio Ballester B, Duff A, Duarte Oller E, Verschure PFMJ. Effect of specific over nonspecific VR-based rehabilitation on poststroke motor recovery: a systematic meta-analysis. Neurorehabil Neural Repair. (2019) 33:112–29. doi: 10.1177/1545968318820169
27. Chou YH, Ton That V, Sundman M. A systematic review and meta-analysis of rTMS effects on cognitive enhancement in mild cognitive impairment and Alzheimer's disease. Neurobiol Aging. (2020) 86:1–10. doi: 10.1016/j.neurobiolaging.2019.08.020
28. Wenisch E, Cantegreil-Kallen I, De Rotrou J, Garrigue P, Moulin F, Batouche F, et al. Cognitive stimulation intervention for elders with mild cognitive impairment compared to normal aged subjects: preliminary results. Aging Clin Exp Res. (2007) 19:316–22. doi: 10.1007/BF03324708
30. Slater M. Immersion and the illusion of presence in virtual reality. Br J Psychol. (2018) 109:431–3. doi: 10.1111/bjop.12305
31. Moreno A, Wall KJ, Thangavelu K, Craven L, Ward E, Dissanayaka NN. A systematic review of the use of virtual reality and its effects on cognition in individuals with neurocognitive disorders. Alzheimer's Dement Transl Res Clin Interv. (2019) 5:834–50. doi: 10.1016/j.trci.2019.09.016
32. Chirico A, Cipresso P, Yaden DB, Biassoni F, Riva G, Gaggioli A. Effectiveness of immersive videos in inducing awe : an experimental study. Sci Rep. (2017) 7:1–11. doi: 10.1038/s41598-017-01242-0
33. Ventura S, Brivio E, Riva G, Baños RM. Immersive versus non-immersive experience: exploring the feasibility of memory assessment through 360° technology. Front Psychol. (2019) 10:2509. doi: 10.3389/fpsyg.2019.02509
34. Bohil CJ, Alicea B, Biocca FA. Virtual reality in neuroscience research and therapy. Nat Rev Neurosci. (2011) 12, 752–62. doi: 10.1038/nrn3122
35. Riva G. The neuroscience of body memory: from the self through the space to the others. Cortex. (2018) 104:241–60. doi: 10.1016/j.cortex.2017.07.013
36. Ijsselsteijn W, Riva G. Being There: the experience of presence in mediated environments. In: Riva G, Davide F, IJsselsteijn WA, editors. Being There: Concepts, Effects and Measurement of User Presence in Synthetic Environments. Amsterdam: IOS Press (2003). p. 3–16.
37. Teo WP, Muthalib M, Yamin S, Hendy AM, Bramstedt K, Kotsopoulos E, et al. Does a combination of virtual reality, neuromodulation and neuroimaging provide a comprehensive platform for neurorehabilitation? A narrative review of the literature. Front Hum Neurosci. (2016) 10:284. doi: 10.3389/fnhum.2016.00284
38. Rose FD, Brooks BM, Rizzo AA. Virtual reality in brain damage rehabilitation: review. Cyberpsychol Behav. (2005) 8:241–62. doi: 10.1089/cpb.2005.8.241
39. Riva G, Baños RM, Botella C, Mantovani F, Gaggioli A. Transforming experience: the potential of augmented reality and virtual reality for enhancing personal and clinical change. Front Psychiatry. (2016) 7:164. doi: 10.3389/fpsyt.2016.00164
40. Cipresso P, Giglioli IAC, Raya MA, Riva G. The past, present, and future of virtual and augmented reality research: a network and cluster analysis of the literature. Front Psychol. (2018) 9:2086. doi: 10.3389/fpsyg.2018.02086
41. Sanchez-vives MV, Slater M. From presence towards consciousness. Nat Rev Neurosci. (2005) 6:332. doi: 10.1038/nrn1651
42. Slater M. Place illusion and plausibility can lead to realistic behaviour in immersive virtual environments. Philos Trans R Soc B Biol Sci. (2009) 364:3549–57. doi: 10.1098/rstb.2009.0138
43. Skarbez R, Neyret S, Brooks FP, Slater M, Whitton MC. A psychophysical experiment regarding components of the plausibility illusion. IEEE Trans Vis Comput Graph. (2017) 23:1322–31. doi: 10.1109/TVCG.2017.2657158
44. Slater M, Sanchez-Vives MV. Enhancing our lives with immersive virtual reality. Front Robot AI. (2016) 3:74. doi: 10.3389/frobt.2016.00074
45. Riva G, Wiederhold BK, Mantovani F. Neuroscience of virtual reality: from virtual exposure to embodied medicine. Cyberpsychology Behav Soc Netw. (2019) 22:82–96. doi: 10.1089/cyber.2017.29099.gri
46. Kim O, Pang Y, Kim JH. The effectiveness of virtual reality for people with mild cognitive impairment or dementia: a meta-analysis. BMC Psychiatry. (2019) 19:219. doi: 10.1186/s12888-019-2180-x
47. Henderson A, Korner-Bitensky N, Levin M. Virtual reality in stroke rehabilitation: a systematic review of its effectiveness for upper limb motor recovery. Top Stroke Rehabil. (2007) 14:52–61. doi: 10.1310/tsr1402-52
48. Saposnik G, Teasell R, Mamdani M, Hall J, McIlroy W, Cheung D, et al. Effectiveness of virtual reality using wii gaming technology in stroke rehabilitation: a pilot randomized clinical trial and proof of principle. Stroke. (2010) 41:1477–84. doi: 10.1161/STROKEAHA.110.584979
49. Saposnik G, Levin M. Virtual reality in stroke rehabilitation: a meta-analysis and implications for clinicians. Stroke. (2011) 42:1380–6. doi: 10.1161/STROKEAHA.110.605451
50. Gómez MM. The use of immersive virtual reality in neurorehabilitation and its impact in neuroplasticity (thesis) (2017).
51. Optale G, Busato V, Marin S, Piron L, Priftis K, Gamberini L, et al. Controlling memory impairment in elderly adults using virtual reality memory training: a randomized controlled pilot study. Neurorehabil Neural Repair. (2010) 24:348–57. doi: 10.1177/1545968309353328
52. Man DWK, Chung JCC, Lee GYY. Evaluation of a virtual reality-based memory training programme for Hong Kong Chinese older adults with questionable dementia: a pilot study. Int J Geriatr Psychiatry. (2012) 27:513–20. doi: 10.1002/gps.2746
53. Liao YY, Tseng HY, Lin YJ, Wang CJ, Hsu WC. Using virtual reality-based training to improve cognitive function, instrumental activities of daily living and neural efficiency in older adults with mild cognitive impairment. Eur J Phys Rehabil Med. (2020) 56:47–57. doi: 10.23736/S1973-9087.19.05899-4
54. Cho DR, Lee SH. Effects of virtual reality immersive training with computerized cognitive training on cognitive function and activities of daily living performance in patients with acute stage stroke: a preliminary randomized controlled trial. Medicine (Baltimore). (2019) 98:e14752. doi: 10.1097/MD.0000000000014752
55. De Luca R, Russo M, Naro A, Tomasello P, Leonardi S, Santamaria F, et al. Effects of virtual reality-based training with BTs-Nirvana on functional recovery in stroke patients: preliminary considerations. Int J Neurosci. (2017) 128:791–6. doi: 10.1080/00207454.2017.1403915
56. Faria AL, Andrade A, Soares L, Bermúdez S. Benefits of virtual reality based cognitive rehabilitation through simulated activities of daily living : a randomized controlled trial with stroke patients. J Neuroeng Rehabil. (2016) 13:96–108. doi: 10.1186/s12984-016-0204-z
57. Mathews M, Mitrovic A, Ohlsson S, Holland J, McKinley A. A virtual reality environment for rehabilitation of prospective memory in stroke patients. Procedia Comput Sci. (2016) 96:7–15. doi: 10.1016/j.procs.2016.08.081
58. Faria AL, Pinho MS, Bermúdez I, Badia S. A comparison of two personalization and adaptive cognitive rehabilitation approaches: a randomized controlled trial with chronic stroke patients. J Neuroeng Rehabil. (2020) 17:1–15. doi: 10.1186/s12984-020-00691-5
59. Liao Y, Chen I, Lin Y, Chen Y, Hsu W. Effects of virtual reality-based physical and cognitive training on executive function and dual-task gait performance in older adults with mild cognitive impairment : a randomized control trial. Front Aging Neurosci. (2019) 11:162. doi: 10.3389/fnagi.2019.00162
60. Maggio MG, De Cola MC, Latella D, Maresca G, Finocchiaro C, La Rosa G, et al. What about the role of virtual reality in Parkinson's disease cognitive rehabilitation? Preliminary findings from a randomized clinical trial. J Geriatr Psychiatry Neurol. (2018) 31:312–8. doi: 10.1177/0891988718807973
61. Maggio MG, Torrisi M, Buda A, Luca R De, Cannavò A, Leo A, et al. Effects of robotic neurorehabilitation through Lokomat plus Virtual Reality on cognitive function in patients with Traumatic Brain Injury: a retrospective case-control study. Int J Neurosci. (2019) 130:117–23. doi: 10.1080/00207454.2019.1664519
62. Dinh HQ, Walker N, Hodges LF, Song C, Kobayashi A. Evaluating the importance of multi-sensory input on memory and the sense of presence in virtual environments. In: Proceedings IEEE Virtual Reality (Cat. No. 99CB36316). IEEE (1999). p. 222–8.
63. Lustig C, Shah P, Seidler R, Reuter-Lorenz PA. Aging, training, and the brain: a review and future directions. Neuropsychol Rev. (2009) 19:504–22. doi: 10.1007/s11065-009-9119-9
64. Belleville S, Clément F, Mellah S, Gilbert B, Fontaine F, Gauthier S. Training-related brain plasticity in subjects at risk of developing Alzheimer's disease. Brain. (2011) 134:1623–34. doi: 10.1093/brain/awr037
65. Appel L, Appel E, Bogler O, Wiseman M, Cohen L, Ein N, et al. Older adults with cognitive and/or physical impairments can benefit from immersive virtual reality experiences: a feasibility study. Front Med. (2020) 6:329. doi: 10.3389/fmed.2019.00329
66. Howard MC. A meta-analysis and systematic literature review of virtual reality rehabilitation programs. Comput Human Behav. (2017) 70:317–27. doi: 10.1016/j.chb.2017.01.013
67. Sandrini M, Umiltà C, Rusconi E. The use of transcranial magnetic stimulation in cognitive neuroscience: a new synthesis of methodological issues. Neurosci Biobehav Rev. (2011) 35:516–36. doi: 10.1016/j.neubiorev.2010.06.005
68. Diana M, Raij T, Melis M, Nummenmaa A, Leggio L, Bonci A. Rehabilitating the addicted brain with transcranial magnetic stimulation. Nat Rev Neurosci. (2017) 18:685. doi: 10.1038/nrn.2017.113
69. Rossi S, Hallett M, Rossini PM, Pascual-Leone A, Avanzini G, Bestmann S, et al. Safety, ethical considerations, and application guidelines for the use of transcranial magnetic stimulation in clinical practice and research. Clin Neurophysiol. (2009) 120:2008–39. doi: 10.1016/j.clinph.2009.08.016
70. Brunoni AR, Sampaio-Junior B, Moffa AH, Aparício LV, Gordon P, Klein I, et al. Noninvasive brain stimulation in psychiatric disorders: a primer. Brazilian J Psychiatry. (2019) 41:70–81. doi: 10.1590/1516-4446-2017-0018
71. Nardone R, Tezzon F, Höller Y, Golaszewski S, Trinka E, Brigo F. Transcranial magnetic stimulation (TMS)/repetitive TMS in mild cognitive impairment and Alzheimer's disease. Acta Neurol Scand. (2014) 129:351–66. doi: 10.1111/ane.12223
72. Drumond Marra HL, Myczkowski ML, Maia Memória C, Arnaut D, Leite Ribeiro P, Sardinha Mansur CG, et al. Transcranial magnetic stimulation to address mild cognitive impairment in the elderly: a randomized controlled study. Behav Neurol. (2015) 2015:287843. doi: 10.1155/2015/287843
73. Taylor JL, Hambro BC, Strossman ND, Bhatt P, Hernandez B, Ashford JW, et al. The effects of repetitive transcranial magnetic stimulation in older adults with mild cognitive impairment: a protocol for a randomized, controlled three-arm trial. BMC Neurol. (2019) 19:326. doi: 10.1186/s12883-019-1552-7
74. Padala PR, Padala KP, Lensing SY, Jackson AN, Hunter CR, Parkes CM, et al. Repetitive transcranial magnetic stimulation for apathy in mild cognitive impairment: a double-blind, randomized, sham-controlled, cross-over pilot study. Psychiatry Res. (2018) 261:312–8. doi: 10.1016/j.psychres.2017.12.063
75. Reitan RM. The relation of the Trail Making Test to organic brain damage. J Consult Psychol. (1955) 19:393. doi: 10.1037/h0044509
76. Hoogendam JM, Ramakers GMJ, Di Lazzaro V. Physiology of repetitive transcranial magnetic stimulation of the human brain. Brain Stimul. (2010) 3:95–118. doi: 10.1016/j.brs.2009.10.005
77. Siebner HR, Hartwigsen G, Kassuba T, Rothwell JC. How does transcranial magnetic stimulation modify neuronal activity in the brain? Implications for studies of cognition. Cortex. (2009) 45:1035–42. doi: 10.1016/j.cortex.2009.02.007
78. Mantovani M, Van Velthoven V, Fuellgraf H, Feuerstein TJ, Moser A. Neuronal electrical high frequency stimulation enhances GABA outflow from human neocortical slices. Neurochem Int. (2006) 49:347–50. doi: 10.1016/j.neuint.2006.02.008
79. Pascual-Leone A, Walsh V, Rothwell J. Transcranial magnetic stimulation in cognitive neuroscience – virtual lesion, chronometry, and functional connectivity. Curr Opin Neurobiol. (2000) 10:232–7. doi: 10.1016/S0959-4388(00)00081-7
80. Moliadze V, Zhao Y, Eysel U, Funke K. Effect of transcranial magnetic stimulation on single-unit activity in the cat primary visual cortex. J Physiol. (2003) 553:665–79. doi: 10.1113/jphysiol.2003.050153
81. Pasley BN, Allen EA, Freeman RD. State-dependent variability of neuronal responses to transcranial magnetic stimulation of the visual cortex. Neuron. (2009) 62:291–303. doi: 10.1016/j.neuron.2009.03.012
82. Bassolino M, Franza M, Bello Ruiz J, Pinardi M, Schmidlin T, Stephan MA, et al. Non-invasive brain stimulation of motor cortex induces embodiment when integrated with virtual reality feedback. Eur J Neurosci. (2018) 47:790–9. doi: 10.1111/ejn.13871
83. Notzon S, Deppermann S, Fallgatter A, Diemer J, Kroczek A, Domschke K, et al. Psychophysiological effects of an iTBS modulated virtual reality challenge including participants with spider phobia. Biol Psychol. (2015) 112:66–76. doi: 10.1016/j.biopsycho.2015.10.003
84. Massetti T, Crocetta TB, Silva TD da, Trevizan IL, Arab C, Caromano FA, et al. Application and outcomes of therapy combining transcranial direct current stimulation and virtual reality: a systematic review. Disabil Rehabil Assist Technol. (2017) 12:551–9. doi: 10.1080/17483107.2016.1230152
85. Lee SJ, Chun MH. Combination transcranial direct current stimulation and virtual reality therapy for upper extremity training in patients with sukimbacute stroke. Arch Phys Med Rehabil. (2014) 95:431–8. doi: 10.1016/j.apmr.2013.10.027
86. Viana RT, Laurentino GEC, Souza RJP, Fonseca JB, Silva Filho EM, Dias SN, et al. Effects of the addition of transcranial direct current stimulation to virtual reality therapy after stroke: a pilot randomized controlled trial. NeuroRehabilitation. (2014) 34:437–46. doi: 10.3233/NRE-141065
87. Johnson NN, Carey J, Edelman BJ, Doud A, Grande A, Lakshminarayan K, et al. Combined rTMS and virtual reality brain-computer interface training for motor recovery after stroke. J Neural Eng. (2018) 15:016009. doi: 10.1088/1741-2552/aa8ce3
88. Subramanian SK, Prasanna SS. Virtual reality and noninvasive brain stimulation in stroke: how effective is their combination for upper limb motor improvement? A meta-analysis. PM R. (2018) 10:1261–70. doi: 10.1016/j.pmrj.2018.10.001
89. Kang YJ, Park HK, Kim HJ, Lim T, Ku J, Cho S, et al. Upper extremity rehabilitation of stroke : facilitation of corticospinal excitability using virtual mirror paradigm. J Neuroeng Rehabil. (2012) 9:71. doi: 10.1186/1743-0003-9-71
90. Kim YJ, Ku J, Cho S, Kim HJ, Cho YK, Lim T, et al. Facilitation of corticospinal excitability by virtual reality exercise following anodal transcranial direct current stimulation in healthy volunteers and subacute stroke subjects. J Neuroeng Rehabil. (2014) 11:1–12. doi: 10.1186/1743-0003-11-124
91. Funahashi S, Andreau JM. Prefrontal cortex and neural mechanisms of executive function. J Physiol Paris. (2013) 107:471–82. doi: 10.1016/j.jphysparis.2013.05.001
92. Herrmann D, Parenté R. The multimodal approach to cognitive rehabilitation. Neurorehabilitation. (1994) 4:133–42. doi: 10.3233/NRE-1994-4303
93. Blumenfeld RS, Ranganath C. Dorsolateral prefrontal cortex promotes long-term memory formation through its role in working memory organization. J Neurosci. (2006) 26:916–25. doi: 10.1523/JNEUROSCI.2353-05.2006
94. Ranganath C, Cohen MX, Brozinsky CJ. Working memory maintenance contributes to long-term memory formation: neural and behavioral evidence. J Cogn Neurosci. (2005) 17:994–1010. doi: 10.1162/0898929054475118
95. Yuan B, Chen J, Gong L, Shu H, Liao W, Wang Z, et al. Mediation of episodic memory performance by the executive function network in patients with amnestic mild cognitive impairment: a resting-state functional MRI study. Oncotarget. (2016) 7:64711. doi: 10.18632/oncotarget.11775
96. Pedroli E, Serino S, Cipresso P, Leo G, De Goulene K, Morelli S, et al. An Immersive Cognitive Rehabilitation Program : A Case Study. In: International Conference on NeuroRehabilitation. Pisa: Springer International Publishing (2018).
97. Mioshi E, Dawson K, Mitchell J, Arnold R, Hodges JR. The Addenbrooke's Cognitive Examination revised (ACE-R): a brief cognitive test battery for dementia screening. Int J Geriatr Psychiatry. (2006) 21:1078–85. doi: 10.1002/gps.1610
98. Nasreddine ZS, Phillips NA, Bédirian V, Charbonneau S, Whitehead V, Collin I, et al. The Montreal Cognitive Assessment, MoCA: a brief screening tool for mild cognitive impairment. J Am Geriatr Soc. (2005) 53:695–9. doi: 10.1111/j.1532-5415.2005.53221.x
99. Caffarra P, Vezzadini G, Dieci F, Zonato F, Venneri A. Una versione abbreviata del test di Stroop: Dati normativi nella popolazione Italiana. Nuova Riv di Neurol. (2002) 12:111–5. Available online at: http://hdl.handle.net/11381/2261558
100. Blackburn HL, Benton AL. Revised administration and scoring of the Digit Span Test. J Consult Psychol. (1957) 21:139. doi: 10.1037/h0047235
101. Spinnler H, Tognoni G. Italian Group on the Neuropsychological Study of Ageing: Italian standardization and classification of neuropsychological tests. Ital J Neurol Sci. (1987) 6:1–120.
102. Allamanno N, Della Sala S, Laiacona M, Pasetti C, Spinnler H. Problem solving ability in aging and dementia: normative data on a non-verbal test. Ital J Neurol Sci. (1987) 8:111–9. doi: 10.1007/BF02337583
103. Spielberger CD, Gorsuch RL, Lushene RE. Manual for the State-Trait Anxiety Inventory. Palo Alto, CA: Consulting Psychologists Press (1970).
104. Laudisio A, Antonelli Incalzi R, Gemma A, Marzetti E, Pozzi G, Padua L, et al. Definition of a Geriatric Depression Scale cutoff based upon quality of life: a population-based study. Int J Geriatr Psychiatry. (2018) 33:e58–64. doi: 10.1002/gps.4715
105. Katz S, Ford AB, Moskowitz RW, Jackson BA, Jaffe MW. Studies of illness in the aged: the index of ADL: a standardized measure of biological and psychosocial function. JAMA. (1963) 185:914–9. doi: 10.1001/jama.1963.03060120024016
106. Friston KJ, Price CJ. Degeneracy and redundancy in cognitive anatomy. Trends Cogn Sci. (2003). 7:151–2. doi: 10.1016/S1364-6613(03)00054-8
107. Prvulovic D, Van De Ven V, Sack AT, Maurer K, Linden DEJ. Functional activation imaging in aging and dementia. Psychiatry Res Neuroimaging. (2005) 140:97–113. doi: 10.1016/j.pscychresns.2005.06.006
108. Mansur CG, Fregni F, Boggio PS, Riberto M, Gallucci-Neto J, Santos CM, et al. A sham stimulation-controlled trial of rTMS of the unaffected hemisphere in stroke patients. Neurology. (2005) 64:1802–4. doi: 10.1212/01.WNL.0000161839.38079.92
109. Carey JR, Deng H, Gillick BT, Cassidy JM, Anderson DC, Zhang L, et al. Serial treatments of primed low-frequency rTMS in stroke: characteristics of responders vs. nonresponders. Restor Neurol Neurosci. (2014) 32:323–35. doi: 10.3233/RNN-130358
110. Carey JR, Evans CD, Anderson DC, Bhatt E, Nagpal A, Kimberley TJ, et al. Safety of 6-Hz primed low-frequency rTMS in stroke. Neurorehabil Neural Repair. (2008) 22:185–92. doi: 10.1177/1545968307305458
111. Takeuchi N, Izumi SI. Maladaptive plasticity for motor recovery after stroke: mechanisms and approaches. Neural Plast. (2012) 2012:359728. doi: 10.1155/2012/359728
112. Liang P, Wang Z, Yang Y, Jia X, Li K. Functional disconnection and compensation in mild cognitive impairment: evidence from DLPFC connectivity using resting-state fMRI. PLoS ONE. (2011) 6:e22153. doi: 10.1371/journal.pone.0022153
113. Seth AK, Suzuki K, Critchley HD. An interoceptive predictive coding model of conscious presence. Front Psychol. (2012) 2:395. doi: 10.3389/fpsyg.2011.00395
114. Bernardet U, Väljamäe A, Inderbitzin M, Wierenga S, Mura A, Verschure PFMJ. Quantifying human subjective experience and social interaction using the eXperience Induction Machine. Brain Res Bull. (2011) 85:305–12. doi: 10.1016/j.brainresbull.2010.11.009
115. Marshall GA, Rentz DM, Frey MT, Locascio JJ, Johnson KA, Sperling RA. Executive function and instrumental activities of daily living in mild cognitive impairment and Alzheimer's disease. Alzheimer's Dement. (2011) 7:300–8. doi: 10.1016/j.jalz.2010.04.005
116. Cipresso P, Immekus JC. Back to the future of quantitative psychology and measurement: psychometrics in the twenty-first century. Front Psychol. (2017) 8:2099. doi: 10.3389/fpsyg.2017.02099
Keywords: virtual reality, transcranial magnetic stimulation, mild cognitive impairment, cognitive rehabilitation, cave, dorsolateral prefrontal cortex, non-invasive brain stimulation, executive functions
Citation: Mancuso V, Stramba-Badiale C, Cavedoni S, Pedroli E, Cipresso P and Riva G (2020) Virtual Reality Meets Non-invasive Brain Stimulation: Integrating Two Methods for Cognitive Rehabilitation of Mild Cognitive Impairment. Front. Neurol. 11:566731. doi: 10.3389/fneur.2020.566731
Received: 28 May 2020; Accepted: 24 August 2020;
Published: 30 September 2020.
Edited by:
Marta Matamala-Gomez, University of Milano-Bicocca, ItalyReviewed by:
Klaudia Grechuta, Pompeu Fabra University, SpainSolène Neyret, University of Barcelona, Spain
Copyright © 2020 Mancuso, Stramba-Badiale, Cavedoni, Pedroli, Cipresso and Riva. This is an open-access article distributed under the terms of the Creative Commons Attribution License (CC BY). The use, distribution or reproduction in other forums is permitted, provided the original author(s) and the copyright owner(s) are credited and that the original publication in this journal is cited, in accordance with accepted academic practice. No use, distribution or reproduction is permitted which does not comply with these terms.
*Correspondence: Valentina Mancuso, di5tYW5jdXNvOTUmI3gwMDA0MDtnbWFpbC5jb20=