- 1The Ritchie Centre, Hudson Institute of Medical Research, Clayton, VIC, Australia
- 2Department of Obstetrics and Gynecology, Monash University, Clayton, VIC, Australia
- 3Neonatal Research Unit, Centre for the Studies of Asphyxia and Resuscitation, Royal Alexandra Hospital, Edmonton, AB, Canada
- 4Department of Pediatrics, University of Alberta, Edmonton, AB, Canada
Many preterm neonates require mechanical ventilation which increases the risk of cerebral inflammation and white matter injury in the immature brain. In this review, we discuss the links between ventilation and brain injury with a focus on the immediate period after birth, incorporating respiratory support in the delivery room and subsequent mechanical ventilation in the neonatal intensive care unit. This review collates insight from large animal models in which acute injurious ventilation and prolonged periods of ventilation have been used to create clinically relevant brain injury patterns. These models are valuable resources in investigating the pathophysiology of ventilation-induced brain injury and have important translational implications. We discuss the challenges of reconciling lung and brain maturation in commonly used large animal models. A comprehensive understanding of ventilation-induced brain injury is necessary to guide the way we care for preterm neonates, with the goal to improve their neurodevelopmental outcomes.
Introduction
Respiratory support is a necessary life-saving intervention which has been associated with brain injury, especially in preterm neonates. Preterm birth, defined as birth prior to 37 completed weeks of gestation, is a major cause of perinatal mortality and morbidity (1, 2). Almost 1 million preterm infants who survive the neonatal period suffer adverse neurodevelopmental outcomes (1) which, in addition to an individual burden, imposes enormous financial and social costs to their families and society. Many complications associated with prematurity are due to an interruption of normal organ development that would otherwise proceed to term in utero. For this reason, the distinction of babies by gestational age (GA) at birth—extremely preterm (<28 weeks), very preterm (28–<32 weeks), and moderate to late preterm (32–<37 weeks)—helps to identify infant populations which are most at risk of complications related to preterm birth (3). Notably, the lungs of very and extremely preterm infants are often too immature to provide adequate respiratory function required to sustain extrauterine life.
The lower the GA of the infant at birth, the less mature the lungs are, and the higher the requirement for respiratory support. An estimated 2.4 million babies are born very and extremely preterm worldwide each year (3) and ~60–95% of these infants will require respiratory support during their neonatal period (2, 4–7). At the same time, the brains of these infants who require respiratory support are at a vulnerable stage of development and prone to injury. It is this combination of high requirements for respiratory support and the heightened vulnerability of their immature brains that increases the risk of ventilation-induced brain injury (VIBI) in extremely preterm infants.
Importantly, VIBI is likely to ensue as early as when respiratory support commences in the delivery room. Depending on GA, ~34–85% of preterm infants require intubation and positive pressure ventilation (PPV) to establish lung aeration immediately after birth (2, 8–10). These statistics exclude non-invasive forms of ventilation, meaning the total percentage of preterm infants who need respiratory support immediately after birth is substantially higher. Despite this high requirement, the limitations of equipment used in delivery suites mean that a significant proportion of babies receive inappropriate pressures or tidal volumes (VT) (11, 12), which can initiate pathways leading to VIBI (13). Subsequent to this, the duration of ventilatory support in the neonatal intensive care unit (NICU) is proportional to the risk of neurodevelopmental impairment and disorders (5, 14).
Respiratory support exacerbates key pathways of preterm brain injury: (1) cerebral inflammation and (2) cerebral hemodynamic instability (13, 15, 16), meaning ventilated preterm infants are in the unfortunate position of double jeopardy and are at an increased risk of brain injury. The nature of VIBI is not fully understood because it is difficult to determine clinically if brain injury is attributed solely or predominantly to ventilation. It is in this background that large animal models have played a vital role in improving our understanding of the pathogenesis of VIBI and to aid development of therapies.
In this review we will explore the issue of VIBI, how large animal models have been utilized to investigate VIBI, and the value of these models to develop much-needed therapies.
Preterm Birth, The Requirement For Respiratory Support, And How This May Be Injurious
Prematurity is the key contributor to the need for respiratory support in newborns. The majority of extremely preterm newborns will require respiratory support due to inadequate alveolarization, insufficient surfactant production, and impaired lung liquid clearance, together with reduced respiratory drive, weak chest muscles and flexible ribs (16, 17).
Our improved understanding of respiratory transition and lung function from fetal to newborn life has led to significant advances in neonatal respiratory care, many of which aim to reduce the risk of chronic lung diseases and adverse neonatal outcomes. Despite this, a significant proportion of preterm infants still develop long-term pulmonary and neurodevelopmental morbidities due to ventilation-induced injury. Various methods of respiratory support (e.g., nasal continuous positive airway pressure, PPV via face mask or endotracheal tube) have been linked to cerebral inflammation and neuropathologies in preterm infants, including cystic periventricular leukomalacia, diffuse white matter injury and intraventricular hemorrhage (IVH) (14, 18–21). It is essential to clarify and address the effect ventilation has on the preterm infant.
Positive Pressure Ventilation in the Delivery Room
Most infants can independently transition from a fetus to a newborn, but many preterm infants will require assistance for this physiologically challenging process. Neonatal transition involves cardiovascular adaptations and, more importantly, respiratory adaptations since the newborn is no longer supported by the placenta for oxygenation (17). Infants who cannot spontaneously breathe at birth will require PPV which is usually first delivered non-invasively via a facemask, and infants who are still unable to initiate stable respiration are intubated (22, 23). Extremely preterm infants may be electively intubated in the delivery room in some centers although it has been suggested that individualized intubation strategies after establishing respiratory failure may be better to reduce morbidities (24, 25), given that the process of intubation may itself be injurious and is associated with neurodevelopmental impairments (26).
A significant proportion of very and extremely infants require intubation in the delivery room. Despite decreasing percentages of infants requiring intubation in the delivery room over the past decades (7, 10), a staggering 31.6–77.7% of very low birth weight (VLBW) and/or extremely preterm infants continue to require this invasive intervention (2, 7, 9, 10). Early PPV in the delivery room has been associated with the development of severe IVH (20, 21). VLBW infants, mostly born extremely preterm, who received PPV in the delivery room had a nearly 3-fold increased likelihood of severe IVH (grades III and IV) than infants who did not receive PPV (20). However, it could be that the infants who require higher levels of intervention are sicker and more vulnerable to brain injury to begin with, hence it is challenging to accurately determine the extent to which advanced resuscitation is causal in the progression of brain injury in these infants.
Importantly, despite the high requirement of PPV in the delivery room, it is likely the least controlled respiratory support a neonate will ever receive, and this has proven to be inadvertently injurious to the immature brain (11, 13, 21). Current neonatal resuscitation guidelines in the delivery room rely on visual assessment of chest rise to deliver an adequate VT during PPV where pressure monitoring is unavailable (23, 27, 28). Besides being subjective, the ability to observe changes in chest wall movement is reduced when a preterm infant is covered to maintain body temperature during delivery room resuscitation, stabilization, and transportation (27). It is challenging even for experienced clinicians to accurately estimate the VT delivered (11, 28) and a noticeably expanded chest wall from PPV may itself be a sign of lung overdistension. Excessively high VT causes volutrauma—a major cause of lung inflammation and injury (16, 29–32). Together, these factors contribute to a suboptimal ventilation situation that leads to injury of the lung and, consequently, the brain. Indeed, the use of excessive VT has dire consequences on the immature brain. Preterm infants <29 weeks GA who received unintentional high VT ventilation (>6 ml/kg, where median normal VT is 4.2–5.8 ml/kg) in the delivery room had a nearly 4-fold higher incidence of IVH than infants who received normal VT (<6 ml/kg; 51% vs. 13%) (21, 33).
Other mechanisms by which PPV leads to lung injury are barotrauma (e.g., high airway pressure), atelectrauma (e.g., repeated opening and closing of collapsed airways), and biotrauma (30–32, 34). Systemic inflammation secondary to lung injury can also initiate cerebral inflammation which is a major cause of brain injury. Inappropriate ventilation pressures and volumes can also trigger the hemodynamic pathway of injury to cause hemorrhagic brain injury (13).
Mechanical Ventilation in the Neonatal Intensive Care Unit
Preterm infants often continue to require respiratory support after transfer to the NICU. In Australia and New Zealand, up to 95.0% of very and extremely preterm babies (<32 weeks GA) and 91.3% of moderate to late preterm infants (32–36 weeks GA) needed assisted ventilation in the NICU, with each baby receiving on average 8.8 days of assisted ventilation (2). A cohort study in South Korea reported that 38.5% of VLBW preterm infants received >7 days of mechanical ventilation (35). Importantly, the trends for long-term respiratory support in preterm infants do not seem to be decreasing (2, 36).
Prolonged periods of mechanical ventilation increases the risks of IVH (4, 22), periventricular leukomalacia or white matter injury (4, 6, 19, 35, 37), cerebral palsy (14), and attention deficit hyperactivity disorder (14) in preterm infants. In a retrospective analysis of extremely low birth weight infants, most of whom were extremely preterm, only 24% of infants who were ventilated for ≥60 days and 7% of those ventilated for ≥90 days survived without neurodevelopmental impairments (5). All infants who had been ventilated for ≥120 days and survived suffered some form of neurodevelopmental impairment (5).
Compared to the initiation of PPV in the delivery room, PPV in the NICU is much more controlled with sophisticated equipment and vigilant monitoring of ventilation parameters (38). The precise cause of VIBI in this setting has not been thoroughly investigated, with additional confounding factors such as analgesia and anesthetics (39–41), oxygenation (19), and a plethora of other NICU interventions for a range of primary and/or secondary complications that need to be considered. However, it is known that the duration of ventilation is an important determinant of neurodevelopmental morbidities (5, 19, 42).
Attempts to shift management encouraging earlier extubation or less invasive ventilation strategies have not translated to improved neurological outcomes in preterm infants (43). Furthermore, limiting the duration of mechanical ventilation to reduce complications is not always feasible with preterm infants. Therefore, it is imperative to devise treatments for unavoidable brain injury from prolonged respiratory support.
Using Animal Models to Investigate Ventilation-Induced Brain Injury
Clinical observations discussed above underpin the need to understand mechanisms through which respiratory support causes brain injury, to allow focused clinical strategies or new therapies aimed at improving outcomes. However, such investigations are not necessarily achievable in preterm infants since respiratory support cannot be studied in isolation. Herein lies the value of using animals for comprehensive characterization of VIBI through imaging, physiological, immunohistochemical, and molecular techniques. Animals can also be used to model various conditions such as growth restriction and chorioamnionitis to more closely interrogate VIBI under conditions of compromised pregnancies.
Studies using large animals, most often sheep, to model ventilation-induced injury can be categorized by experimental technique (fetal [head-out or in utero ventilation] or neonatal ventilation) and period/duration of ventilation (acute or chronic) (Figure 1). These different experimental techniques enable replication of specific scenarios of preterm respiratory support, including the initial resuscitation in the delivery room and prolonged care in the NICU. However, with all models, an understanding of the strengths and limitations is essential for appropriate interpretation of the findings and potential replication in clinical trials.
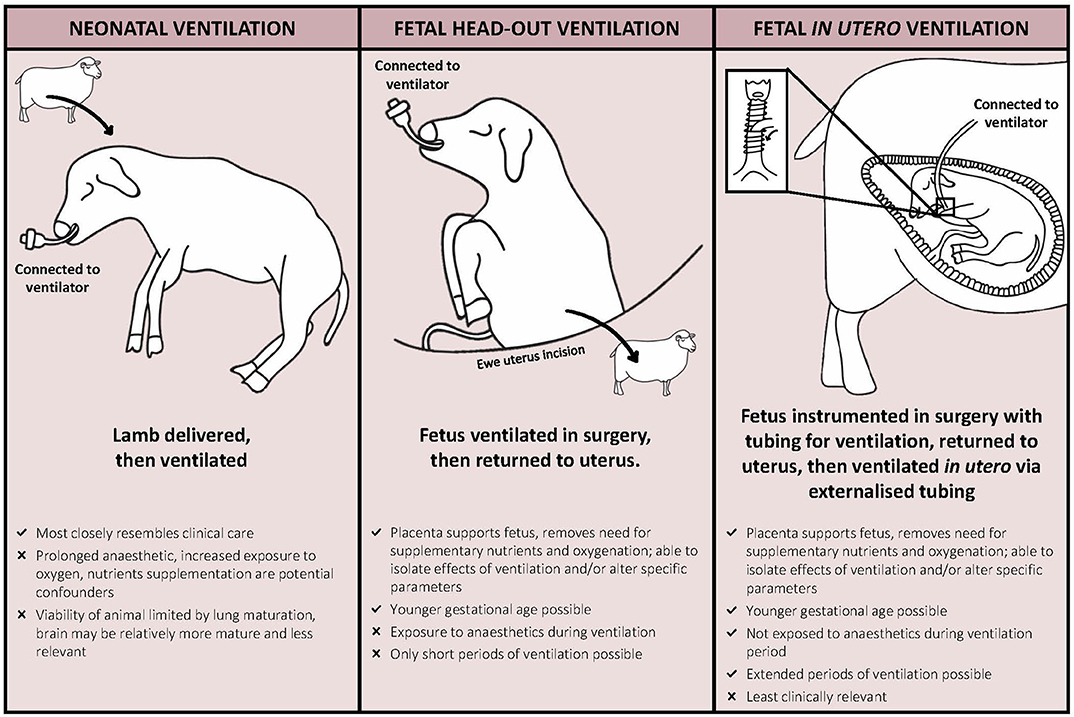
Figure 1. Experimental models of ventilation-induced injury in sheep outlining the major advantages and disadvantages of each model.
Balance Between Lung and Brain Development in Large Animal Models
An inevitable limitation to using large animals to model neonatal conditions is the difference in developmental milestones of major organ systems and physiology compared to humans. The animal's age cannot be chosen based on gestation duration alone since an animal at 0.65 gestation is not necessarily developmentally equivalent to a human at 0.65 gestation. Instead, the crucial factor is the stage of development of the organ of interest. This proves challenging for models of VIBI as the developmental milestones of both the brain and lungs must be considered. Detailed comparisons of species-specific lung development and anatomical features have previously been compiled (44–46) and comparisons for brain development are summarized in Table 1. This presents a conundrum: how do we balance desired stage of brain development with lung maturation?
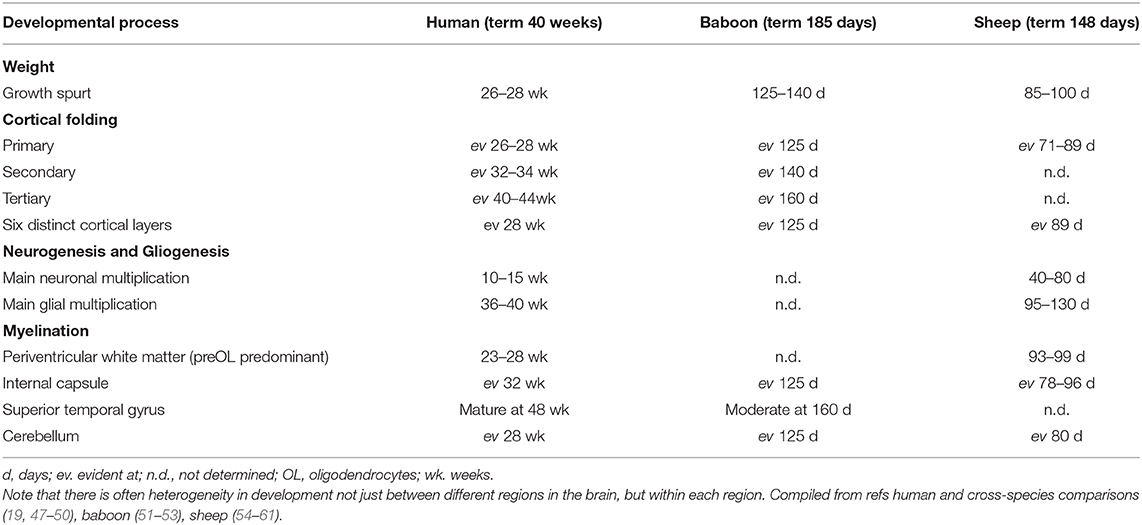
Table 1. Comparative gestational ages for key brain development processes in the human, baboon, and sheep.
Non-human primate studies have used baboons (Papio papio; Papio cynocephalus) delivered at 125 days (term is 185 days; 0.68 gestation) which have similar lung development to an infant born at 26 weeks preterm age (44). At this stage, brain development is comparable to that of a 26–28 week-old extremely preterm human infant (51). While these developmental stages are congruent, there are significant technical challenges as well as practical, financial and ethical concerns associated with the use of non-human primates (62). Thus, even though they are the closest animal models to humans and offer vital insights to developmental studies (62), non-human primate models of VIBI are relatively less commonly pursued.
Piglets (Sus scrofa) have been proposed to be suitable for studying neurodevelopment and cerebral consequences of early life insults (63). A piglet at 91–94 days gestation (term is 115 days; 0.8 gestation) is physiologically similar to a 23–25 week extremely preterm infant in terms of lung development and the requirement for respiratory support for survival (64). Neurodevelopment of the piglet at this GA is slightly more mature, comparable instead to a moderate to late preterm human infant (63, 65). Besides this developmental mismatch, there are significant challenges in performing fetal surgery and chronic instrumentation in pigs due to relatively large litters and the large size of the sow. Hence, piglets have not been widely used in VIBI studies that require these techniques. The suitability of piglets in postnatal ventilation studies for VIBI has not been extensively explored.
Similarly, brain maturation in sheep (Ovies aries) advances more rapidly in late gestation than development of the lungs, relative to humans. Previous studies in preterm lambs that have investigated VIBI were performed at the earliest GA at which the lambs were viable with respiratory support [125 days where term is 148 days; 0.85 gestation; structural lung development comparable to a 26–28 week human infant (46, 66)]. However, a limitation is that the fetal sheep brain development at 125 days gestation is comparable to a late preterm or term human fetus on the basis of white matter maturation (60, 61). Studies using lambs at this gestation have investigated the effect of respiratory support for up to 4 weeks on chronic lung injury (67), but have only looked at VIBI up to 24 h of ventilation (68).
Modeling of Acute VIBI
Animal studies to date have focused on VIBI downstream of lung injury resulting from volutrauma in the delivery room. The rationale behind these studies is that clinical findings have shown that variable VT during delivery room resuscitation can be outside recommended limits (11, 12, 69).
Observed VT during mask ventilation can range from 0 to 31 ml/kg (11, 12, 69) and VT during endotracheal tube ventilation has been reported to be 3.9–9.6 ml/kg (12). The upper ranges of these VT are higher than the recommended 4–8 ml/kg for very and extremely preterm infants (11). This is critical as we have known for decades that as few as six manual inflations of high VT (35–40 ml/kg) are enough to induce injury in immature, surfactant-deficient lungs of preterm lambs (70). Sheep studies that have investigated acute VIBI have similarly found that brief periods of high VT ventilation resulted in detectable brain injury as early as 90 min after ventilation onset (13, 15, 16, 71–73). Importantly, even if recommended VT is delivered, the act of respiratory support in itself can activate an immune response in immature respiratory units (30, 31, 34).
To isolate this initial period of injurious respiratory support, akin to poorly regulated VT in the delivery room, sheep studies have employed an acute high VT ventilation strategy: 15 min injurious ventilation with stepwise increments of VT to achieve a high target VT of 10–15 ml/kg, which is 2–3 times the normal VT of lambs for that GA (125 days of term 148 days; ~5–7 ml/kg) (13, 15, 16, 71–73). Thereafter, lambs are sustained on appropriate respiratory support (neonatal model) or returned to the uterus (head-out model) to allow for the inflammation and injury pathways to manifest into gross lung/brain injury. These studies have provided valuable information on the pathology and mechanisms of acute VIBI (discussed in section Understanding VIBI From Injurious Respiratory Support in the Delivery Room).
Chronic Models of VIBI
The majority of animal models used to model VIBI are acute, focusing on the initial hours after birth. This is mainly due to inherent problems with maintaining animals for long periods of time. In particular, the problem with maintaining respiratory support for long periods of time in newborn animal models is the inability to control for specific factors, due to the need to introduce increasing levels of neonatal intensive care—akin to that of looking after a chronically ventilated preterm infant. To get around this problem, animal models have utilized respiratory support via a head-out approach or entirely in utero (Figure 1). Using these techniques, the intact placental circulation manages nutrition and gas exchange of the fetus, allowing subtle mechanisms of respiratory support to be examined. In the head-out approach, the fetal head and chest are exteriorized, the fetus is intubated and ventilation with various strategies altering delivered volume, pressures, respiratory frequencies, or oxygen content, and then returned to the uterus (74–78). In utero ventilation (IUV) studies require the fetus to be exteriorized and instrumented with ventilation tubes and equipment required for monitoring prior to being returned to the uterus. After a recovery period for the ewe and fetus, the fetus is ventilated via the externalized ventilation tubes for various times, although to date the longest has been 12 h (79–81). IUV has been used in fetal sheep to study cardiopulmonary physiology (82, 83), lung mechanics (84), and ventilation-induced lung injury (79, 81, 85). While cerebral physiological responses to IUV have been investigated previously (86), histopathology of brain injury after IUV has not been reported.
It is obvious that these in utero models are not designed with the intention to replicate clinical situations given that prolonged neonatal studies are more reflective of current clinical care. Instead, they provide the opportunity to manipulate specific ventilatory parameters in isolation so that we can better understand the contribution of a sole variable to lung and brain injury. Importantly, the IUV model allows ventilation of a fetus at a younger gestation than would be viable postnatally. This is advantageous, especially in ovine models, as the stage of brain development will be more comparable to that of extremely preterm infants.
Understanding VIBI From Injurious Respiratory Support in the Delivery Room
Animal Studies That Investigate Pathology of VIBI
Studies in preterm lambs have characterized acute white matter changes following 15 min of injurious high VT ventilation (13, 15, 16, 71–73, 87, 88). High VT ventilation causes a robust pulmonary inflammatory response which increases systemic and cerebral inflammation, characterized by elevated IL-6 and IL-8 messenger ribonucleic acid (mRNA) levels in the periventricular and subcortical white matter of the brain in ventilated preterm lambs (15, 73, 87). Increased microglial activation and aggregation, and a higher incidence of vascular protein extravasation (indicative of a compromised blood-brain barrier) and cerebral hemorrhage in the same regions were also observed (15, 73, 88). Injurious ventilation did not alter expression of myelin basic protein (MBP; oligodendrocyte marker) in the internal capsule or neuronal nuclei (NeuN; neuron marker) in the thalamus (89) and did not increase inflammation or injury in gray matter (90).
Importantly, pathology resultant from injurious ventilation can be visualized using non-invasive imaging such as magnetic resonance imaging (MRI) (72, 77, 91) and correlated with histopathology (89). Magnetic resonance spectroscopy (MRS) detected acute changes in brain metabolite peak-area ratios (Lactate/Creatine and Lactate/Choline) in preterm lambs that received high VT although macroscopic injury was absent in structural MR images (T1, T2) (72). Alterations in MRS-detected metabolite levels relate to neuronal damage and potentially predict subsequent neurodevelopmental impairments (92, 93). Notably, these MRS changes were observed within 90 min of ventilation onset (72). Recent findings suggest that MRS-detectable changes persist 24 h after injurious ventilation (77). Diffusion tensor imaging (DTI) perhaps offers the most sensitive measures of early brain injury. DTI detected decreased diffusivity measures in the frontal white matter (axial, radial, and mean) and internal capsule (axial) in preterm lambs 24 h after injurious ventilation (77). These parameters have been suggested to correlate with myelination deficits (77).
Mechanistic Insight From Animal Studies
Several explanations have been put forward to link ventilation and brain injury. Studies in ventilated preterm lambs have identified two major pathways of acute VIBI: cerebral inflammation and hemodynamic instability (13, 15, 16, 77). Both pathways are proposed to be downstream effects of the pulmonary consequences following ventilation (13, 15, 16). Incidentally, these key VIBI pathways mirror those of preterm brain injury—suggesting compounded risk of injury in preterm infants. These mechanisms have been reviewed previously (13).
Briefly, the inflammatory pathway of VIBI involves upregulation of pro-inflammatory cytokines (e.g., IL-6, IL-8) and activation of microglia and astrocytes within the developing white matter of the brain (94). Injurious ventilation initiates a profound pulmonary inflammatory response caused by volutrauma, barotrauma, atelectrauma, and/or biotrauma (30–32, 34). This inflammatory cascade is associated with systemic inflammation and subsequent localized inflammation and injury in the white matter involving glia cells (13, 15, 16). Activated microglia and astrocytes are thought to mediate the destruction of cells in the oligodendrocyte lineage, contributing to hypomyelination and diffuse white matter injury that can underlie long-term neurological sequelae such as cerebral palsy (94, 95).
The hemodynamic pathway of injury refers to significant alterations, caused by PPV and atypical to hemodynamic changes during the transition at birth, to pulmonary blood flow and consequently cardiac output and cerebral blood flow (CBF) (15, 16). During PPV, applying a high pressure into the airways decreases pulmonary capillary transmural pressure, causing compression of intra-alveolar capillaries, hence increasing capillary resistance and decreasing pulmonary blood flow (13, 16). This reduces pulmonary venous return, left ventricular output, and accordingly alters CBF (13, 15, 16). Arterial blood pressure variability within a physiological range is not usually a problem because it is compensated by pressure-flow autoregulation to sustain a stable CBF. This involves constriction and dilation of arteries to alter cerebral vasculature resistance in response to changing perfusion pressures (96). The autoregulatory plateau, bounded by lower and upper limits of arterial pressure, has been postulated to be narrower in preterm infants with decreasing GA (96–98). Moreover, it has been suggested that preterm delivery or treatments reflective of clinical care of the preterm infant, including mechanical ventilation, affects cerebral autoregulation (99). Prolonged CBF fluctuations for more than 10 to 20 s has been defined as cerebral hemodynamic instability (100). The initiation of ventilation in preterm lambs caused CBF instability in the initial 15 min, even when a gentle strategy was used (71); the variability in CBF amplified when an injurious high VT strategy was used (15). Clinically, 91% of babies with respiratory distress syndrome who had fluctuating CBF after 12 h of life subsequently had an IVH (101), highlighting the critical importance of preventing fluctuations in hemodynamics immediately after birth.
The relative contribution of each pathway toward the progression of white matter injury discussed in section Animal Studies That Investigate Pathology of VIBI is unknown although a recent study suggests that the hemodynamic pathway has an additive effect on the inflammatory pathway on injury progression, but the inflammatory pathway seems to dominate (77).
Understanding VIBI From Ventilation in the Neonatal Intensive Care Unit
Ventilation studies in preterm baboons and lambs suggest that the brain injury underlying neurodevelopmental impairments in chronically ventilated preterm infants involves subtle diffuse white and gray matter lesions, often without intraventricular or germinal matrix hemorrhage and overt lesions or infarcts (19, 51, 102–104). This indicates a potentially distinct mechanism of injury to acute VIBI sustained in delivery room settings.
Preterm baboons have been used extensively to study the impact of prolonged mechanical ventilation (2–4 weeks) on the lungs (105–107) and, more recently, the brain (103, 107, 108). In these studies, preterm baboons (125 days of term 185 days; 0.68 gestation) are cared for with similar interventions to that of preterm infants in the NICU, including mechanical ventilation using a gentle strategy to maintain VT at 4–6 ml/kg with adequate chest motion (51, 106). While not investigating injury from ventilation per se, the brain injury observed in these animals is not from any direct insult or influenced by potentiating conditions associated with preterm birth or an adverse uterine environment. The subtle neuropathologies from preterm birth and subsequent intensive care alone closely resemble what is observed clinically (51, 102, 103, 109). After 14 days of ventilator support, preterm baboon brains had delayed gyrification (102, 104), reduced brain weight (102–104), reduced white and gray matter volumes (103, 104), increased white and gray matter injury (51), increased astrogliosis in the forebrain (103), increased ramified microglia (103), and a reduction of oligodendrocytes (103, 104) compared to gestation-matched controls. These histopathological indices correlated with microstructural and macrostructural changes detected by ex vivo MRI (109).
Additionally, the effects of shorter durations of controlled NICU respiratory support have been investigated in sheep. Preterm lambs (125 days of term 148 days; 0.85 gestation) ventilated with a non-injurious strategy (VT at 5–7 ml/kg) had increased IL-8 and connective tissue growth factor (CTGF) mRNA levels and decreased vascular occludin protein density in the white matter after 2 h (110). When the length of ventilation was extended to 24 h, ventilated lambs had increased astrogliosis within cortical gray matter but otherwise no apparent neuropathology or changes in glial cell populations compared to unventilated control lambs (68).
Both the preterm baboon and lamb models discussed are neonatal ventilation models. However, as mentioned above, a disadvantage of the neonatal ventilation model is the intensive care requirements of maintaining a preterm animal for significant periods of time, making them more akin to human studies where individual parameters cannot be teased apart unless large numbers of animals are used, which is financially unviable. This is where the IUV model may be advantageous if used for extended periods beyond 24 h.
Influence of the Antenatal Environment on Respiratory Support and VIBI
Work explored in the previous sections have studied the pathology and mechanisms of VIBI in preterm but otherwise healthy animals. The ability to isolate effects of respiratory support with minimal confounding factors is vital and these findings provide a foundation to explore therapeutic options to minimize VIBI which will be discussed in section Bench to Bedside of this review. However, it is important to consider that the clinical situation is much more complex—many preterm infants will have been exposed to adverse uterine environments which may increase their risk of VIBI.
Adverse Antenatal Conditions Alter Responses to Postnatal Respiratory Support
Adverse antenatal conditions such as fetal growth restriction (FGR) and intrauterine inflammation have independently been associated with adverse neurodevelopmental outcomes in preterm infants (111, 112). Further, these infants often require respiratory support after birth, increasing the risk of brain injury. Yet, there is a paucity of information on how these antenatal conditions alter the response these infants have to ventilation and if this contributes to VIBI.
Fetal Growth Restriction and VIBI
FGR is a condition where the fetus fails to reach its projected growth potential, often due to placenta insufficiency (112). FGR fetuses are sometimes delivered preterm to prevent deterioration in an adverse in utero environment (112), thus many will require respiratory support due to prematurity. FGR fetuses have altered cardiovascular and vascular function, most notably the characteristic “brain-sparing” phenomenon by redirecting blood flow and oxygen delivery to important organs including the heart, adrenals, and brain. These adaptations persist to early postnatal life and may affect how a growth-restricted infant responds to ventilation. Preterm growth-restricted lambs ventilated with a gentle non-injurious strategy for 24 h had disrupted interaction of astrocyte end-feet with cerebral blood vessels, increased microgliosis, and increased oxidative stress compared to their unventilated counterparts and to ventilated preterm appropriately-grown lambs (68). Notably, differences between growth-restricted and appropriately grown lambs were evident after 2 h of ventilation (110). This suggests that growth restricted infants may be at increased risk of VIBI, perhaps in part due to differences in the neurovascular unit and blood-brain barrier properties (68, 110).
Intrauterine Inflammation and VIBI
Intrauterine inflammation, which most commonly presents as chorioamnionitis, is a major cause of preterm birth (113). Antenatal inflammation alters the vulnerability and response of the immature brain to ventilation (71, 91, 114). Lipopolysaccharide(LPS)-mediated inflammation in utero amplified cerebral hemodynamic instability during the initiation of ventilation in preterm lambs (71). Compared to saline controls, these lambs that had been exposed to LPS 2 or 4 days before preterm delivery had increased inflammation, vascular extravasation, and microhemorrhages in cerebral white matter regions after ventilation (71). Further, injurious ventilation increased the number of apoptotic cells (TUNEL+ cells) in the subcortical white matter of LPS-exposed lambs, compared to their unventilated counterparts (114). Injurious ventilation had no obvious acute detrimental effects on white matter (89) and gray matter (90) compared to injuriously ventilated healthy preterm lambs and to LPS-exposed lambs that received gentle ventilation. Brain macro- and microstructure as assessed by MRI and DTI were similarly not different (89, 91). However, a novel DTI color map threshold technique detected lower diffusivity indices in white matter regions of the brain, indicative of subtle brain injury in the ventilated lambs that were exposed to inflammation prior to delivery (91). Importantly, using a non-injurious ventilation strategy did not mitigate VIBI in the LPS-exposed lambs (114). Clinically, histologic chorioamnionitis is associated with a longer cumulative duration of mechanical ventilation in VLBW infants (35), thereby increasing the risk of VIBI. However, the combination of chorioamnionitis and prolonged ventilation has not been investigated in large animals and the potential cerebral effects are unknown.
Cerebral Effects of Antenatal Medical Interventions
Corticosteroid administration is a common antecedent to preterm birth, where antenatal glucocorticoids (betamethasone and dexamethasone) are given to accelerate fetal lung maturation before preterm labor (115). Clinically, antenatal glucocorticoid administration is suggested to reduce the incidence and severity of IVH (115) and does not affect subsequent development of subsequent childhood mental and behavioral disorders in preterm infants (116). However, information on its interaction with respiratory support is scant. A recent study found that antenatal betamethasone improved cerebral hemodynamic instability in preterm lambs that received 15 min of high VT injurious ventilation followed by 75 min non-injurious ventilation (88). However, there was an increase in the percentage of amoeboid microglia in the periventricular white matter, the number of vessel profiles with protein extravasation in the subcortical white matter, and malondialdehyde levels in cerebrospinal fluid, suggesting increased inflammation and oxidative stress in betamethasone-treated animals than their saline-treated counterparts ventilated with the same protocol (88). This potential increased risk of VIBI following antenatal betamethasone administration may lie in the increased lung compliance and hence susceptibility of the lungs to volutrauma rather than a direct cerebral effect (88).
It is crucial to consider that antenatal glucocorticoid administration may have additional interactions with the conditions mentioned above; for example, growth restricted fetuses have different hemodynamic responses to antenatal glucocorticoids compared to appropriately grown fetuses (117). Maternal betamethasone administration increased fetal cardiac output and blood flow to major organs whereas cardiac output was decreased and blood flow to major organs remained unchanged in control fetuses (118). Furthermore, there were transient decreases in carotid blood flow, an index for CBF, in both control and FGR fetuses. While CBF of control fetuses were stable after returning to baseline levels, FGR fetuses displayed a persistent rebound increase in carotid blood flow from 10 h after treatment (119). Whether these altered responses are beneficial or harmful in the context of VIBI needs to be ascertained. Indeed, little is known about the combined effects of adverse antenatal conditions, antenatal glucocorticoid administration, and postnatal respiratory support on brain injury in the preterm infant. Large animal models of VIBI may provide a means to address this.
Bench to Bedside
Establishing reliable animal models with reproducible neuropathology that is reflective of injury seen clinically expedites efforts to test novel potential interventions and/or therapeutic candidates. Potential treatments for VIBI and their mechanisms of actions have recently been discussed in detail by Barton et al. (120) and this remains an active area of research.
In the delivery room setting, physiological-based cord clamping (PBCC) can stabilize pulmonary, systemic, and cerebral circulation in preterm (121, 122) and near-term lambs (123), essentially mitigating the hemodynamic pathway of injury, but it is unlikely to prevent VIBI resultant from the inflammatory pathway. PBCC refers to delaying umbilical cord clamping until respiration has been initiated and established in the newborn or providing respiratory support prior to umbilical cord clamping (121–123). Thus, a therapy that targets both pathways of VIBI, with a focus on modulating inflammation, is required. To date, animal experiments have investigated short-term effects of erythropoietin (EPO) and human amnion epithelial cells (hAECs) as prophylactic postnatal treatments for VIBI resultant from acute volutrauma (73, 87, 124). These treatments have proposed mechanisms of action that make them ideal candidates for neuroprotection. EPO has anti-inflammatory, anti-apoptotic, and neurotrophic properties while hAECs are anti-inflammatory and reparative (120).
When administered to preterm lambs that received 15 min of injurious high VT ventilation, single early low doses of 300 IU/kg and 1,000 IU/kg human recombinant EPO did not reduce or exacerbate lung and brain injury (124, 125), suggesting that EPO doses presently used in clinical trials appear to be safe for preterm infants receiving respiratory support. However, they appear to not be efficacious as a therapy for VIBI given the lack of therapeutic potential observed. High doses of EPO of 3,000 IU/kg and 5,000 IU/kg increased cerebrospinal fluid EPO levels to “neuroprotective levels” [>100 mU/ml (126)] within 2 h of administration (73, 124). These high doses, respectively, had a protective effect on blood-brain barrier integrity (124) and differential regional effects on white matter (73) despite both doses amplifying lung inflammation and injury (125, 127). Together, these data highlight a complex dose response with distinct effects on the lungs and brain, indicating that further investigation is required to elucidate the efficacy of EPO in the context of a preterm infant requiring respiratory support.
In a similar study, preterm lambs that received high VT ventilation were administered an intratracheal infusion of 9 × 107 hAECs before ventilation onset and an additional intravenous dose of 9 × 107 hAECs within 5 min of delivery (total 1.8 × 108 hAECs) (87, 128). The cells were able to enter the brain within 2 h of administration, as detected by fluorescent cell labeling in the frontal and parietal periventricular and subcortical white matter of the brain (87). Cell administration reduced microgliosis and vascular protein extravasation (87), potentially as a downstream effect of reduced pulmonary inflammation (128). However, hAECs did not stabilize hemodynamic transition or modulate systemic inflammation within the brief period of the experiment, and conversely they induced an increase in pro-inflammatory cytokine mRNA levels within the brain (87, 128). Further long-term effects of hAECs on acute VIBI have not been investigated to accurately determine the interaction of hAECs and ventilation on the preterm brain.
Chronic ventilation studies in animals have so far focused on treatments to reduce lung rather than brain injury. Sheep fetuses ventilated in utero for 12 h and administered intratracheal infusion of 3 × 107 hAECs and an intravenous dose of 3 × 107 hAECs at 3 h and 6 h after ventilation onset (total 1.2 × 108 hAECs) demonstrated a reduction in ventilation-induced lung injury (81), and as such may have the potential to reduce VIBI but this remains a speculation.
While the above strategies have some promise, it is unlikely that a single strategy will prevent or reduce VIBI. The key might lie in a multipronged approach that involves reducing the requirement for or duration of respiratory support, optimizing how PPV is administered to avoid adverse effects, and reducing the sequelae of unpreventable adverse effects of PPV (Figure 2).
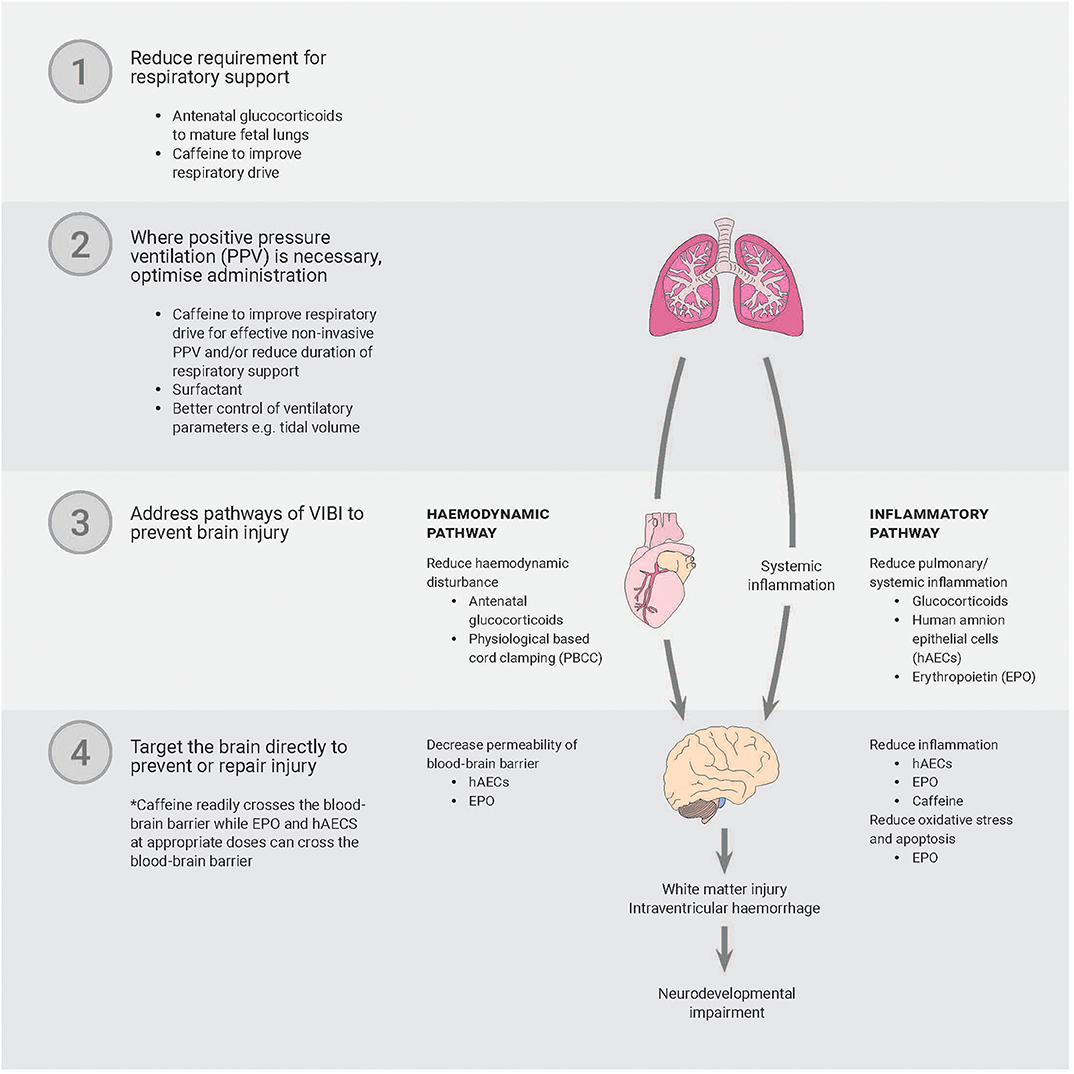
Figure 2. A multipronged approach is likely necessary to prevent or reduce ventilation-induced brain injury. Only strategies discussed in-text have been included in the diagram. PPV, positive pressure ventilation; PBCC, physiological-based cord clamping; hAECs, human amnion epithelial cells; EPO, erythropoietin.
In this regard, researchers in The Netherlands have investigated the use of caffeine in the delivery room, showing improved respiratory efforts of preterm infants (24–30 weeks GA), potentially reducing the need for invasive respiratory support in this setting (129). Further, spontaneous respiratory drive is a determinant of effective use of gentle non-invasive respiratory support (130). However, caffeine administration to mechanically ventilated preterm infants (23–30 weeks GA) in the first 5 days of life did not encourage early extubation or decrease ventilation duration in the NICU (130, 131). The trial was terminated due to safety concerns, making it difficult to interpret results and secondary outcome findings of morbidities including BPD and IVH (130, 131). In contrast, caffeine had neuroprotective effects in very preterm infants when assessed at 18 months' corrected age (132, 133), in part attributed to earlier discontinuation of PPV and decreased rates of bronchopulmonary dysplasia (134, 135). The treatment benefits of caffeine administered in the first 10 days of life on neurobehavioral and functional cognitive outcomes were less pronounced at 5- and 11-years follow-up, with only slight but statistically significant improvements to motor outcomes observed (136–138). Earlier administration of caffeine within the first 2 days of life has been associated with improved neurodevelopmental outcomes at 18 to 24 months' corrected age compared to late administration (133) but whether these benefits persist have not been reported. Certainly, these contradictory findings highlight the need to better understand the interaction of caffeine, respiratory support, and neurodevelopmental outcomes. Independently, caffeine has been postulated to have neuroprotective properties by reducing inflammation, reducing periventricular white matter injury, and stabilizing hemodynamics in preterm infants (133).
Correspondingly, protective ventilation strategies have reduced brain inflammation and vascular protein extravasation but do not completely mitigate injury in preterm lambs (15, 16, 72, 114). Together, these indicate that our current efforts to minimize the need for respiratory support and, where respiratory support is necessary, improve the way PPV is administered are inadequate to prevent VIBI. Large animal models will likely play a key role in studies focusing on stimulating respiratory function at birth and optimizing the delivery of non-invasive respiratory support to minimize lung and brain inflammation and injury. Additionally, there is an apparent need to devise treatments and large animal models of VIBI provide a means to address this.
Summary
This review highlights the necessity of large animal models when investigating the relationship between invasive respiratory support, the lungs, and the brain in the preterm infant. These models provide a powerful research tool; a combination of physiological, histological, molecular and imaging techniques provides an integrated picture of the interactions between respiratory support and the immature brain which is difficult to obtain in a clinical setting.
Recognizing the consequences of respiratory support on the immature brain will encourage development of effective therapies to prevent or treat VIBI in otherwise healthy preterm infants. VIBI should also be considered when investigating treatments for other conditions such as FGR, chorioamnionitis, and hypoxic injury where the compromised infant will often receive respiratory support.
Author Contributions
KC contributed to the conception of the review and created the table. KC and GS conceptualized and created the figures. KC, SM, GS, VS, and GP contributed to structuring, drafting, revising, and the final approval of the version to be published. All authors contributed to the article and approved the submitted version.
Funding
This work was supported by the National Health and Medical Research Council (NHMRC) and/or National Heart Foundation of Australia and/or NHMRC Fellowships (VS: 1165935 and GP: 1105526) and the Victorian Government's Operational Infrastructure Support Program.
Conflict of Interest
The authors declare that the research was conducted in the absence of any commercial or financial relationships that could be construed as a potential conflict of interest.
References
1. Blencowe H, Lee ACC, Cousens S, Bahalim A, Narwal R, Zhong N, et al. Preterm birth-associated neurodevelopmental impairment estimates at regional and global levels for 2010. Pediatr Res. (2013) 74(Suppl. 1):17–34. doi: 10.1038/pr.2013.204
2. Chow SSW, Creighton P, Chambers G, Lui K. Report of the Australian and New Zealand Neonatal Network 2017. Sydney, NSW: ANZNN (2019).
3. Blencowe H, Cousens S, Oestergaard MZ, Chou D, Moller AB, Narwal R, et al. National, regional, and worldwide estimates of preterm birth rates in the year 2010 with time trends since 1990 for selected countries: a systematic analysis and implications. Lancet. (2012) 379:2162–72. doi: 10.1016/S0140-6736(12)60820-4
4. Serenius F, Ewald U, Farooqi A, Holmgren PÅ, Håkansson S, Sedin G. Short-term outcome-after active perinatal management at 23-25 weeks of gestation. A study from two Swedish perinatal centres. Part 3: neonatal morbidity. Acta Paediatr Int J Paediatr. (2004) 93:1090–7. doi: 10.1111/j.1651-2227.2004.tb02722.x
5. Walsh MC, Morris BH, Wrage LA, Vohr BR, Poole WK, Tyson JE, et al. Extremely low birthweight neonates with protracted ventilation: mortality and 18-month neurodevelopmental outcomes. J Pediatr. (2005) 146:798–804. doi: 10.1016/j.jpeds.2005.01.047
6. Gagliardi L, Bellù R, Zanini R, Dammann O. Bronchopulmonary dysplasia and brain white matter damage in the preterm infant: a complex relationship. Paediatr PerinatEpidemiol. (2009) 23:582–90. doi: 10.1111/j.1365-3016.2009.01069.x
7. Soll RF, Edwards EM, Badger GJ, Kenny MJ, Morrow KA, Buzas JS, et al. Obstetric and neonatal care practices for infants 501 to 1500 g from 2000 to 2009. Pediatrics. (2013) 132:222–8. doi: 10.1542/peds.2013-0501
8. Serenius F, Ewald U, Farooqi A, Holmgren PÅ, Håkansson S, Sedin G. Short-term outcome after active perinatal management at 23-25 weeks of gestation. A study from two Swedish tertiary care centres. Part 2: infant survival. Acta Paediatr Int J Paediatr. (2004) 93:1081–9. doi: 10.1111/j.1651-2227.2004.tb02721.x
9. Cho SJ, Shin J, Namgung R. Initial resuscitation at delivery and short term neonatal outcomes in very-low-birth-weight infants. J Korean Med Sci. (2015) 30:S45–51. doi: 10.3346/jkms.2015.30.S1.S45
10. Stoll BJ, Hansen NI, Bell EF, Walsh MC, Carlo WA, Shankaran S, et al. Trends in care practices, morbidity, and mortality of extremely preterm Neonates, 1993-2012. J Am Med Assoc. (2015) 314:1039–51. doi: 10.1001/jama.2015.10244
11. Schmölzer GM, Kamlin OCOF, O'Donnell CPF, Dawson JA, Morley CJ, Davis PG. Assessment of tidal volume and gas leak during mask ventilation of preterm infants in the delivery room. Arch Dis Child Fetal Neonatal Ed. (2010) 95:1–4. doi: 10.1136/adc.2009.174003
12. van Vonderen JJ, Hooper SB, Krabbe VB, Siew ML, te Pas AB. Monitoring tidal volumes in preterm infants at birth: mask versus endotracheal ventilation. Arch Dis Child Fetal Neonatal Ed. (2015) 100:F43–6. doi: 10.1136/archdischild-2014-306614
13. Barton SK, Tolcos M, Miller SL, Roehr CC, Schmölzer GM, Davis PG, et al. Unraveling the links between the initiation of ventilation and brain injury in preterm infants. Front Pediatr. (2015) 3:97. doi: 10.3389/fped.2015.00097
14. Tsai W, Hwang Y, Hung T, Weng S, Lin S-J, Chang W-T. Association between mechanical ventilation and neurodevelopmental disorders in a nationwide cohort of extremely low birth weight infants. Res Dev Disabil. (2014) 35:1544–50. doi: 10.1016/j.ridd.2014.03.048
15. Polglase GR, Miller SL, Barton SK, Baburamani AA, Wong FY, Aridas JDS, et al. Initiation of resuscitation with high tidal volumes causes cerebral hemodynamic disturbance, brain inflammation and injury in preterm lambs. PLoS ONE. (2012) 7:e39535. doi: 10.1371/journal.pone.0039535
16. Polglase GR, Miller SL, Barton SK, Kluckow M, Gill AW, Hooper SB, et al. Respiratory support for premature neonates in the delivery room: effects on cardiovascular function and the development of brain injury. Pediatr Res. (2014) 75:682–8. doi: 10.1038/pr.2014.40
17. Hillman NH, Kallapur SG, Jobe AH. Physiology of transition from intrauterine to extrauterine life. Clin Perinatol. (2012) 39:769–83. doi: 10.1016/j.clp.2012.09.009
18. Rees S, Inder T. Fetal and neonatal origins of altered brain development. Early Hum Dev. (2005) 81:753–61. doi: 10.1016/j.earlhumdev.2005.07.004
19. Albertine KH. Brain injury in chronically ventilated preterm neonates. Clin Perinatol. (2012) 39:727–40. doi: 10.1016/j.clp.2012.06.017
20. Aly H, Hammad TA, Essers J, Wung JT. Is mechanical ventilation associated with intraventricular hemorrhage in preterm infants? Brain Dev. (2012) 34:201–5. doi: 10.1016/j.braindev.2011.04.006
21. Mian Q, Cheung P-Y, O'Reilly M, Barton SK, Polglase GR, Schmölzer GM. Impact of delivered tidal volume on the occurrence of intraventricular haemorrhage in preterm infants during positive pressure ventilation in the delivery room. Arch Dis Child Fetal Neonatal Ed. (2019) 104:F57–62. doi: 10.1136/archdischild-2017-313864
22. Aly H. Is it safer to intubate premature infants in the delivery room? Pediatrics. (2005) 115:1660–5. doi: 10.1542/peds.2004-2493
23. Australian and New Zealand Committee on Resuscitation. ANZCOR Guideline 13. 4 – Airway Management and Mask Ventilation of the Newborn Infant (2016).
24. Lindner W, Vossbeck S, Hummler H, Pohlandt F. Delivery room management of extremely low birth weight infants: spontaneous breathing or intubation? Pediatrics. (1999) 103:961–7. doi: 10.1542/peds.103.5.961
25. Bajaj M, Natarajan G, Shankaran S, Wyckoff M, Laptook AR, Bell EF, et al. Delivery room resuscitation and short-term outcomes in moderately preterm infants. J Pediatr. (2018) 195:33–38.e2. doi: 10.1016/j.jpeds.2017.11.039
26. Wallenstein MB, Birnie KL, Arain YH, Yang W, Yamada NK, Huffman LC, et al. Failed endotracheal intubation and adverse outcomes among extremely low birth weight infants. J Perinatol. (2016) 36:112–5. doi: 10.1038/jp.2015.158
27. Tracy M, Downe L, Holberton J. How safe is intermittent positive pressure ventilation in preterm babies ventilated from delivery to newborn intensive care unit? Arch Dis Child Fetal Neonatal Ed. (2004) 89:F84–7. doi: 10.1136/fn.89.1.F84
28. Poulton DA, Schmölzer GM, Morley CJ, Davis PG. Assessment of chest rise during mask ventilation of preterm infants in the delivery room. Resuscitation. (2011) 82:175–9. doi: 10.1016/j.resuscitation.2010.10.012
29. Parker JC, Hernandez LA, Peevy KJ. Mechanisms of ventilator-induced lung injury. Crit Care Med. (1993) 21:131–43. doi: 10.1097/00003246-199301000-00024
30. Jobe AH, Ikegami M. Mechanisms initiating lung injury in the preterm. Early Hum Dev. (1998) 53:81–94. doi: 10.1016/S0378-3782(98)00045-0
31. Attar MA, Donn SM. Mechanisms of ventilator-induced lung injury in premature infants. Semin Neonatol. (2002) 7:353–60. doi: 10.1053/siny.2002.0129
32. Schmölzer GM, Te Pas AB, Davis PG, Morley CJ. Reducing lung injury during neonatal resuscitation of preterm infants. J Pediatr. (2008) 153:741–5. doi: 10.1016/j.jpeds.2008.08.016
33. Mian QN, Pichler G, Binder C, O'Reilly M, Aziz K, Urlesberger B, et al. Tidal volumes in spontaneously breathing preterm infants supported with continuous positive airway pressure. J Pediatr. (2014) 165:702–706.e1. doi: 10.1016/j.jpeds.2014.06.047
34. Curley GF, Laffey JG, Zhang H, Slutsky AS. Biotrauma and ventilator-induced lung injury: clinical implications. Chest. (2016) 150:1109–17. doi: 10.1016/j.chest.2016.07.019
35. Choi Y-B, Lee J, Park J, Jun YH. Impact of prolonged mechanical ventilation in very low birth weight infants: results from a national cohort study. J Pediatr. (2018) 194:34–9.e3. doi: 10.1016/j.jpeds.2017.10.042
36. Lundqvist P, Källén K, Hallström I, Westas LH. Trends in outcomes for very preterm infants in the southern region of Sweden over a 10-year period. Acta Paediatr Int J Paediatr. (2009) 98:648–53. doi: 10.1111/j.1651-2227.2008.01155.x
37. Barnett ML, Tusor N, Ball G, Chew A, Falconer S, Aljabar P, et al. Exploring the multiple-hit hypothesis of preterm white matter damage using diffusion MRI. NeuroImage Clin. (2018) 17:596–606. doi: 10.1016/j.nicl.2017.11.017
38. Vento M, Aguar M, Leone TA, Finer NN, Gimeno A, Rich W, et al. Using intensive care technology in the delivery room: a new concept for the resuscitation of extremely preterm neonates. Pediatrics. (2008) 122:1113–6. doi: 10.1542/peds.2008-1422
39. Kumar P, Denson SE, Mancuso TJ. Premedication for nonemergency endotracheal intubation in the neonate. Pediatrics. (2010) 125:608–15. doi: 10.1542/peds.2009-2863
40. Andropoulos DB. Effect of anesthesia on the developing brain: infant and fetus. Fetal Diagn Ther. (2018) 43:1–11. doi: 10.1159/000475928
41. Lee J, Loepke AW. Does pediatric anesthesia cause brain damage? – Addressing parental and provider concerns in light of compelling animal studies and seemingly ambivalent human data. Korean J Anesthesiol. (2018) 71:255–73. doi: 10.4097/kja.d.18.00165
42. Vliegenthart RJS, van Kaam AH, Aarnoudse-Moens CSH, van Wassenaer AG, Onland W. Duration of mechanical ventilation and neurodevelopment in preterm infants. Arch Dis Child Fetal Neonatal Ed. (2019) 104:F631–5. doi: 10.1136/archdischild-2018-315993
43. Behnke J, Lemyre B, Czernik C, Zimmer K-P, Ehrhardt H, Waitz M. Non-invasive ventilation in neonatology. Dtsch Arztebl Int. (2019) 116:177–83. doi: 10.3238/arztebl.2019.0177
44. Yoder BA, Coalson JJ. Animal models of bronchopulmonary dysplasia. The preterm baboon models. Am J Physiol Lung Cell Mol Physiol. (2014) 307:L970–7. doi: 10.1152/ajplung.00171.2014
45. Albertine KH. Utility of large-animal models of BPD: chronically ventilated preterm lambs. Am J Physiol Lung Cell Mol Physiol. (2015) 308:L983–1001. doi: 10.1152/ajplung.00178.2014
46. Schittny JC. Development of the lung. Cell Tissue Res. (2017) 367:427–44. doi: 10.1007/s00441-016-2545-0
47. Chi JG, Dooling EC, Gilles FH. Gyral development of the human brain. Ann Neurol. (1977) 1:86–93. doi: 10.1002/ana.410010109
48. Hagberg H, Peebles D, Mallard C. Models of white matter injury: comparison of infectious, hypoxic-ischemic, and excitotoxic insults. Ment Retard Dev Disabil Res Rev. (2002) 8:30–8. doi: 10.1002/mrdd.10007
49. Counsell SJ, Rutherford MA, Cowan FM, Edwards AD. Magnetic resonance imaging of preterm brain injury. Arch Dis Child Fetal Neonatal Ed. (2003) 88:F269–74. doi: 10.1136/fn.88.4.F269
50. Workman AD, Charvet CJ, Clancy B, Darlington RB, Finlay BL. Modeling transformations of neurodevelopmental sequences across mammalian species. J Neurosci. (2013) 33:7368–83. doi: 10.1523/JNEUROSCI.5746-12.2013
51. Dieni S, Inder T, Yoder B, Briscoe T, Camm E, Egan G, et al. The pattern of cerebral injury in a primate model of preterm birth and neonatal intensive care. J Neuropathol Exp Neurol. (2004) 63:1297–309. doi: 10.1093/jnen/63.12.1297
52. Inder T, Neil J, Yoder B, Rees S. Patterns of cerebral injury in a primate model of preterm birth and neonatal intensive care. J Child Neurol. (2005) 20:965–7. doi: 10.1177/08830738050200120601
53. Rees SM, Loeliger MM, Munro KM, Shields A, Dalitz PA, Dieni S, et al. Cerebellar development in a baboon model of preterm delivery. J Neuropathol Exp Neurol. (2009) 68:605–15. doi: 10.1097/NEN.0b013e3181a39b3f
55. Åarströum K-E. on the early development of the isocortex in fetal sheep. Prog Brain Res. (1967) 26:1–59. doi: 10.1016/S0079-6123(08)61418-1
56. Patterson DSP, Sweasey D, Hebert CN. Changes occurring in the chemical composition of the central nervous system during foetal and post-natal development of the sheep. J Neurochem. (1971) 18:2027–40. doi: 10.1111/j.1471-4159.1971.tb05062.x
57. McIntosh GH, Baghurst KI, Potter BJ, Hetzel BS. Foetal brain development in the sheep. Neuropathol Appl Neurobiol. (1979) 5:103–14. doi: 10.1111/j.1365-2990.1979.tb00664.x
58. Back SA, Riddle A, Dean J, Hohimer AR. The instrumented fetal sheep as a model of cerebral white matter injury in the premature infant. Neurotherapeutics. (2012) 9:359–70. doi: 10.1007/s13311-012-0108-y
59. Riddle A. Spatial heterogeneity in oligodendrocyte lineage maturation and not cerebral blood flow predicts fetal ovine periventricular white matter injury. J Neurosci. (2006) 26:3045–55. doi: 10.1523/JNEUROSCI.5200-05.2006
60. Barlow RM. The foetal sheep: morphogenesis of the nervous system and histochemical aspects of myelination. J Comp Neurol. (1969) 135:249–62. doi: 10.1002/cne.901350302
61. Back SA, Riddle A, Hohimer AR. Role of instrumented fetal sheep preparations in defining the pathogenesis of human periventricular white-matter injury. J Child Neurol. (2006) 21:582–9. doi: 10.1177/08830738060210070101
62. Phillips KA, Bales KL, Capitanio JP, Conley A, Czoty PW, 't Hart BA, et al. Why primate models matter. Am J Primatol. (2014) 76:801–27. doi: 10.1002/ajp.22281
63. Conrad MS, Johnson RW. The domestic piglet: an important model for investigating the neurodevelopmental consequences of early life insults. Annu Rev Anim Biosci. (2015) 3:245–64. doi: 10.1146/annurev-animal-022114-111049
64. Eiby YA, Wright LL, Kalanjati VP, Miller SM, Bjorkman ST, Keates HL, et al. A pig model of the preterm neonate: anthropometric and physiological characteristics. PLoS ONE. (2013) 8:e68763. doi: 10.1371/journal.pone.0068763
65. Sweasey D, Patterson DSP, Glancy EM. Biphasic myelination and the fatty acid composition of cerebrosides and cholesterol esters in the developing central nervous system of the domestic pig. J Neurochem. (1976) 27:375–80. doi: 10.1111/j.1471-4159.1976.tb12256.x
66. Alcorn DG, Adamson TM, Maloney JE, Robinson PM. A morphologic and morphometric analysis of fetal lung development in the sheep. Anat Rec. (1981) 201:655–67. doi: 10.1002/ar.1092010410
67. Albertine KH, Jones GP, Starcher BC, Bohnsack JF, Davis PL, Cho SC, et al. Chronic lung injury in preterm lambs. Disordered respiratory tract development. Am J Respir Crit Care Med. (1999) 159:945–58. doi: 10.1164/ajrccm.159.3.9804027
68. Malhotra A, Castillo-Melendez M, Allison BJ, Sutherland AE, Nitsos I, Pham Y, et al. Neuropathology as a consequence of neonatal ventilation in premature growth-restricted lambs. Am J Physiol Integr Comp Physiol. (2018) 315:R1183–94. doi: 10.1152/ajpregu.00171.2018
69. Schilleman K, Van Der Pot CJM, Hooper SB, Lopriore E, Walther FJ, Te Pas AB. Evaluating manual inflations and breathing during mask ventilation in preterm infants at birth. J Pediatr. (2013) 162:457–63. doi: 10.1016/j.jpeds.2012.09.036
70. Björklund LJ, Ingimarsson J, Curstedt T, John J, Robertson B, Werner O, et al. Manual ventilation with a few large breaths at birth compromises the therapeutic effect of subsequent surfactant replacement in immature lambs. Pediatr Res. (1997) 42:348–55. doi: 10.1203/00006450-199709000-00016
71. Polglase GR, Nitsos I, Baburamani AA, Crossley KJ, Slater MK, Gill AW, et al. Inflammation in utero exacerbates ventilation-induced brain injury in preterm lambs. J Appl Physiol. (2012) 112:481–9. doi: 10.1152/japplphysiol.00995.2011
72. Skiöld B, Wu Q, Hooper SB, Davis PG, McIntyre R, Tolcos M, et al. Early detection of ventilation-induced brain injury using magnetic resonance spectroscopy and diffusion tensor imaging: an in vivo study in preterm lambs. PLoS ONE. (2014) 9:e95804. doi: 10.1371/journal.pone.0095804
73. Barton SK, McDougall ARA, Melville JM, Moss TJM, Zahra VA, Lim T, et al. Differential short-term regional effects of early high dose erythropoietin on white matter in preterm lambs after mechanical ventilation. J Physiol. (2016) 594:1437–49. doi: 10.1113/JP271376
74. Hillman NH, Moss TJM, Kallapur SG, Bachurski C, Pillow JJ, Polglase GR, et al. Brief, large tidal volume ventilation initiates lung injury and a systemic response in fetal sheep. Am J Respir Crit Care Med. (2007) 176:575–81. doi: 10.1164/rccm.200701-051OC
75. Hillman NH, Kallapur SG, Pillow JJ, Moss TJM, Polglase GR, Nitsos I, et al. Airway injury from initiating ventilation in preterm sheep. Pediatr Res. (2010) 67:60–5. doi: 10.1203/PDR.0b013e3181c1b09e
76. Hillman NH, Polglase GR, Pillow JJ, Saito M, Kallapur SG, Jobe AH. Inflammation and lung maturation from stretch injury in preterm fetal sheep. Am J Physiol Lung Cell Mol Physiol. (2011) 300:L232–41. doi: 10.1152/ajplung.00294.2010
77. Alahmari DM, Chan KYY, Stojanovska V, LaRosa D, Barton SK, Nitsos I, et al. Diffusion tensor imaging detects ventilation-induced brain injury in preterm lambs. PLoS ONE. (2017) 12:e0188737. doi: 10.1371/journal.pone.0188737
78. Kothe TB, Royse E, Kemp MW, Schmidt A, Salomone F, Saito M, et al. Effects of budesonide and surfactant in preterm fetal sheep. Am J Physiol Cell Mol Physiol. (2018) 315:L193–201. doi: 10.1152/ajplung.00528.2017
79. Allison BJ, Crossley KJ, Flecknoe SJ, Davis PG, Morley CJ, Harding R, et al. Ventilation of the very immature lung in utero induces injury and BPD-like changes in lung structure in fetal sheep. Pediatr Res. (2008) 64:387–92. doi: 10.1203/PDR.0b013e318181e05e
80. Allison BJ, Crossley KJ, Flecknoe SJ, Morley CJ, Polglase GR, Hooper SB. Pulmonary hemodynamic responses to in utero ventilation in very immature fetal sheep. Respir Res. (2010) 11:1–11. doi: 10.1186/1465-9921-11-111
81. Hodges RJ, Jenkin G, Hooper SB, Allison B, Lim R, Dickinson H, et al. Human amnion epithelial cells reduce ventilation-induced preterm lung injury in fetal sheep. Am J Obstet Gynecol. (2012) 206:448.e8–448.e15. doi: 10.1016/j.ajog.2012.02.038
82. Iwamoto HS, Teitel DF, Rudolph AM. Effects of lung distension and spontaneous fetal breathing on hemodynamics in sheep. Pediatr Res. (1993) 33:639–44. doi: 10.1203/00006450-199306000-00021
83. Giraud G, Morton M, Reid D, Reller M, Thornburg K. Effects of ductus arteriosus occlusion on pulmonary artery pressure during in utero ventilation in fetal sheep. Exp Physiol. (1995) 80:129–39. doi: 10.1113/expphysiol.1995.sp003828
84. Blanco CE, Martin CB, Hanson MA, McCooke HB. Breathing activity in fetal sheep during mechanical ventilation of the lungs in utero. Eur J Obstet Gynecol Reprod Biol. (1987) 26:175–82. doi: 10.1016/0028-2243(87)90054-2
85. O'Reilly M, Hooper SB, Allison BJ, Flecknoe SJ, Snibson K, Harding R, et al. Persistent bronchiolar remodeling following brief ventilation of the very immature ovine lung. Am J Physiol Lung Cell Mol Physiol. (2009) 297:992–1001. doi: 10.1152/ajplung.00099.2009
86. Gleason CA, Jones MD, Traystman RJ, Notter RH. Fetal cerebral responses to ventilation and oxygenation in utero. Am J Physiol Integr Comp Physiol. (1988) 255:R1049–54. doi: 10.1152/ajpregu.1988.255.6.R1049
87. Barton SK, Melville JM, Tolcos M, Polglase GR, McDougall AR, Azhan A, et al. Human amnion epithelial cells modulate ventilation-induced white matter pathology in preterm lambs. Dev Neurosci. (2015) 37:338–48. doi: 10.1159/000371415
88. Stojanovska V, Barton SK, Tolcos M, Gill AW, Kluckow M, Miller SL, et al. The effect of antenatal betamethasone on white matter inflammation and injury in fetal sheep and ventilated preterm lambs. Dev Neurosci. (2018) 40:497–507. doi: 10.1159/000496466
89. Alahmari DM, Barton SK, Galinsky R, Nitsos I, Atik A, Farrell M, et al. Correlation between diffusion tensor imaging and histological brain injury in ventilated preterm lambs. Imaging Med. (2017) 9:67–76. doi: 10.14303/Imaging-Medicine.1000061
90. Stojanovska V, Atik A, Nitsos I, Skiöld B, Barton SK, Zahra VA, et al. Effects of Intrauterine inflammation on cortical gray matter of near-term lambs. Front Pediatr. (2018) 6:145. doi: 10.3389/fped.2018.00145
91. Alahmari DM, Skiöld B, Barton SK, Nitsos I, McDonald C, Miller SL, et al. Diffusion tensor imaging colour mapping threshold for identification of ventilation-induced brain injury after intrauterine inflammation in preterm lambs. Front Pediatr. (2017) 5:70. doi: 10.3389/fped.2017.00070
92. Bapat R, Narayana PA, Zhou Y, Parikh NA. Magnetic resonance spectroscopy at term-equivalent age in extremely preterm infants: association with cognitive and language development. Pediatr Neurol. (2014) 51:53–9. doi: 10.1016/j.pediatrneurol.2014.03.011
93. Hyodo R, Sato Y, Ito M, Sugiyama Y, Ogawa C, Kawai H, et al. Magnetic resonance spectroscopy in preterm infants: association with neurodevelopmental outcomes. Arch Dis Child Fetal Neonatal Ed. (2018) 103:F238–44. doi: 10.1136/archdischild-2016-311403
94. Khwaja O, Volpe JJ. Pathogenesis of cerebral white matter injury of prematurity. Arch Dis Child Fetal Neonatal Ed. (2007) 93:F153–61. doi: 10.1136/adc.2006.108837
95. Back SA, Rosenberg PA. Pathophysiology of glia in perinatal white matter injury. Glia. (2014) 62:1790–815. doi: 10.1002/glia.22658
96. Greisen G. Autoregulation of cerebral blood flow in newborn babies. Early Hum Dev. (2005) 81:423–8. doi: 10.1016/j.earlhumdev.2005.03.005
97. Verma PK, Panerai RB, Rennie JM, Evans DH. Grading of cerebral autoregulation in preterm and term neonates. Pediatr Neurol. (2000) 23:236–42. doi: 10.1016/S0887-8994(00)00184-3
98. du Plessis AJ. The role of systemic hemodynamic disturbances in prematurity-related brain injury. J Child Neurol. (2009) 24:1127–40. doi: 10.1177/0883073809339361
99. Czynski AJ, Terry MH, Deming DD, Power GG, Buchholz JN, Blood AB. Cerebral autoregulation is minimally influenced by the superior cervical ganglion in two-week-old lambs, and absent in preterm lambs immediately following delivery. PLoS ONE. (2013) 8:e82326. doi: 10.1371/journal.pone.0082326
100. Gilmore MM, Stone BS, Shepard JA, Czosnyka M, Easley RB, Brady KM. Relationship between cerebrovascular dysautoregulation and arterial blood pressure in the premature infant. J Perinatol. (2011) 31:722–9. doi: 10.1038/jp.2011.17
101. Perlman JM, McMenamin JB, Volpe JJ. Fluctuating cerebral blood-flow velocity in respiratory-distress syndrome. N Engl J Med. (1983) 309:204–9. doi: 10.1056/NEJM198307283090402
102. Rees SM, Camm EJ, Loeliger M, Cain S, Dieni S, McCurnin D, et al. Inhaled nitric oxide: effects on cerebral growth and injury in a baboon model of premature delivery. Pediatr Res. (2007) 61:552–8. doi: 10.1203/pdr.0b013e318045be20
103. Loeliger M, Inder TE, Shields A, Dalitz P, Cain S, Yoder B, et al. High-frequency oscillatory ventilation is not associated with increased risk of neuropathology compared with positive pressure ventilation: a preterm primate model. Pediatr Res. (2009) 66:545–50. doi: 10.1203/PDR.0b013e3181bb0cc1
104. Loeliger M, Inder TE, Dalitz PA, Cain S, Camm EJ, Yoder B, et al. Developmental and neuropathological consequences of ductal ligation in the preterm baboon. Pediatr Res. (2009) 65:209–14. doi: 10.1203/PDR.0b013e31818d6d0b
105. Coalson JJ, Winter VT, Siler-Khodr T, Yoder BA. Neonatal chronic lung disease in extremely immature baboons. Am J Respir Crit Care Med. (1999) 160:1333–46. doi: 10.1164/ajrccm.160.4.9810071
106. Yoder BA, Siler-Khodr T, Winter VT, Coalson JJ. High-frequency oscillatory ventilation: effects on lung function, mechanics, and airway cytokines in the immature baboon model for neonatal chronic lung disease. Am J Respir Crit Care Med. (2000) 162:1867–76. doi: 10.1164/ajrccm.162.5.9912145
107. Loeliger M, Inder T, Cain S, Ramesh RC, Camm E, Thomson MA, et al. Cerebral outcomes in a preterm baboon model of early versus delayed nasal continuous positive airway pressure. Pediatrics. (2006) 118:1640–53. doi: 10.1542/peds.2006-0653
108. Verney C, Rees S, Biran V, Thompson M, Inder T, Gressens P. Neuronal damage in the preterm baboon: impact of the mode of ventilatory support. J Neuropathol Exp Neurol. (2010) 69:473–82. doi: 10.1097/NEN.0b013e3181dac07b
109. Griffith JL, Shimony JS, Cousins SA, Rees SE, McCurnin DC, Inder TE, et al. MR imaging correlates of white-matter pathology in a preterm baboon model. Pediatr Res. (2012) 71:185–91. doi: 10.1038/pr.2011.33
110. Allison BJ, Hooper SB, Coia E, Jenkin G, Malhotra A, Zahra V, et al. Does growth restriction increase the vulnerability to acute ventilation-induced brain injury in newborn lambs? Implications for future health and disease. J Dev Orig Health Dis. (2017) 8:556–65. doi: 10.1017/S204017441700037X
111. Miller SL, Huppi PS, Mallard C. The consequences of fetal growth restriction on brain structure and neurodevelopmental outcome. J Physiol. (2016) 594:807–23. doi: 10.1113/JP271402
112. Sharma D, Shastri S, Sharma P. Intrauterine growth restriction: antenatal and postnatal aspects. Clin Med Insights Pediatr. (2016) 10:67–83. doi: 10.4137/CMPed.S40070
113. Goldenberg RL, Culhane JF, Iams JD, Romero R. Epidemiology and causes of preterm birth. Lancet. (2008) 371:75–84. doi: 10.1016/S0140-6736(08)60074-4
114. Barton SK, Moss TJM, Hooper SB, Crossley KJ, Gill AW, Kluckow M, et al. Protective ventilation of preterm lambs exposed to acute chorioamnionitis does not reduce ventilation-induced lung or brain injury. PLoS ONE. (2014) 9:e112402. doi: 10.1371/journal.pone.0112402
115. Roberts D, Brown J, Medley N, Dalziel SR. Antenatal corticosteroids for accelerating fetal lung maturation for women at risk of preterm birth. Cochrane Database Syst Rev. (2017) 3:CD004454. doi: 10.1002/14651858.CD004454.pub3
116. Räikkönen K, Gissler M, Kajantie E. Associations between maternal antenatal corticosteroid treatment and mental and behavioral disorders in children supplemental content. JAMA. (2020) 323:1924–33. doi: 10.1001/jama.2020.3937
117. Miller SL, Wallace EM. Effect of antenatal steroids on haemodynamics in the normally grown and growth restricted fetus. Curr Pediatr Rev. (2013) 9:67–74. doi: 10.2174/1573396311309010014
118. Miller SL, Supramaniam VG, Jenkin G, Walker DW, Wallace EM. Cardiovascular responses to maternal betamethasone administration in the intrauterine growth-restricted ovine fetus. Am J Obstet Gynecol. (2009) 201:613.e1–613.e8. doi: 10.1016/j.ajog.2009.07.028
119. Miller SL, Chai M, Loose J, Castillo-Meléndez M, Walker DW, Jenkin G, et al. The effects of maternal betamethasone administration on the intrauterine growth-restricted fetus. Endocrinology. (2007) 148:1288–95. doi: 10.1210/en.2006-1058
120. Barton SK, Tolcos M, Miller SL, Christoph-Roehr C, Schmölzer GM, Moss TJM, et al. Ventilation-induced brain injury in preterm neonates: a review of potential therapies. Neonatology. (2016) 110:155–62. doi: 10.1159/000444918
121. Bhatt S, Alison BJ, Wallace EM, Crossley KJ, Gill AW, Kluckow M, et al. Delaying cord clamping until ventilation onset improves cardiovascular function at birth in preterm lambs. J Physiol. (2013) 591:2113–26. doi: 10.1113/jphysiol.2012.250084
122. Polglase GR, Dawson JA, Kluckow M, Gill AW, Davis PG, Te Pas AB, et al. Ventilation onset prior to umbilical cord clamping (physiological-based cord clamping) improves systemic and cerebral oxygenation in preterm lambs. PLoS ONE. (2015) 10:e0117504. doi: 10.1371/journal.pone.0117504
123. Polglase GR, Blank DA, Barton SK, Miller SL, Stojanovska V, Kluckow M, et al. Physiologically based cord clamping stabilises cardiac output and reduces cerebrovascular injury in asphyxiated near-term lambs. Arch Dis Child Fetal Neonatal Ed. (2018) 103:F530–8. doi: 10.1136/archdischild-2017-313657
124. Chan KYY, LaRosa DA, Tolcos M, Li A, Zahra VA, Polglase GR, et al. Optimizing the dose of erythropoietin required to prevent acute ventilation-induced cerebral white matter injury in preterm lambs. Dev Neurosci. (2017) 39:298–309. doi: 10.1159/000459620
125. Allison BJ, LaRosa DA, Barton SK, Hooper S, Zahra V, Tolcos M, et al. Dose-dependent exacerbation of ventilation-induced lung injury by erythropoietin in preterm newborn lambs. J Appl Physiol. (2019) 126:44–50. doi: 10.1152/japplphysiol.00800.2018
126. Juul SE, McPherson RJ, Farrell FX, Jolliffe L, Ness DJ, Gleason CA. Erythropoietin concentrations in cerebrospinal fluid of nonhuman primates and fetal sheep following high-dose recombinant erythropoietin. Biol Neonate. (2004) 85:138–44. doi: 10.1159/000074970
127. Polglase GR, Barton SK, Melville JM, Zahra V, Wallace MJ, Siew ML, et al. Prophylactic erythropoietin exacerbates ventilation-induced lung inflammation and injury in preterm lambs. J Physiol. (2014) 592:1993–2002. doi: 10.1113/jphysiol.2013.270348
128. Melville JM, McDonald CA, Bischof RJ, Polglase GR, Lim R, Wallace EM, et al. Human amnion epithelial cells modulate the inflammatory response to ventilation in preterm lambs. PLoS ONE. (2017) 12:e0173572. doi: 10.1371/journal.pone.0173572
129. Dekker J, Hooper SB, Van Vonderen JJ, Witlox RSGM, Lopriore E, Te Pas AB. Caffeine to improve breathing effort of preterm infants at birth: a randomized controlled trial. Pediatr Res. (2017) 82:290–6. doi: 10.1038/pr.2017.45
130. Amaro CM, Bello JA, Jain D, Ramnath A, D'Ugard C, Vanbuskirk S, et al. Early caffeine and weaning from mechanical ventilation in preterm infants: a randomized, placebo-controlled trial. J Pediatr. (2018) 196:52–7. doi: 10.1016/j.jpeds.2018.01.010
131. Jain VG, Saroha V, Patel RM, Jobe A. Is early caffeine therapy safe and effective for ventilated preterm infants? J Perinatol. (2019) 39:754–7. doi: 10.1038/s41372-019-0336-7
132. Schmidt B, Roberts RS, Davis P, Doyle LW, Barrington KJ, Ohlsson A, et al. Long-term effects of caffeine therapy for apnea of prematurity. N Engl J Med. (2007) 357:1893–902. doi: 10.1056/NEJMoa073679
133. Lodha A, Entz R, Synnes A, Creighton D, Yusuf K, Lapointe A, et al. Early caffeine administration and neurodevelopmental outcomes in preterm infants. Pediatrics. (2019) 143:e20181348. doi: 10.1542/peds.2018-1348
134. Schmidt B, Roberts RS, Davis P, Doyle LW, Barrington KJ, Ohlsson A, et al. Caffeine therapy for apnea of prematurity. N Engl J Med. (2006) 354:2112–21. doi: 10.1056/NEJMoa054065
135. Lodha A, Seshia M, McMillan DD, Barrington K, Yang J, Lee SK, et al. Association of early caffeine administration and neonatal outcomes in very preterm neonates. JAMA Pediatr. (2015) 169:33–8. doi: 10.1001/jamapediatrics.2014.2223
136. Schmidt B, Anderson PJ, Doyle LW, Dewey D, Grunau RE, Asztalos EV, et al. Survival without disability to age 5 years after neonatal caffeine therapy for apnea of prematurity. JAMA. (2012) 307:275–82. doi: 10.1001/jama.2011.2024
137. Schmidt B, Roberts RS, Anderson PJ, Asztalos EV, Costantini L, Davis PG, et al. academic performance, motor function, and behavior 11 years after neonatal caffeine citrate therapy for apnea of prematurity. JAMA Pediatr. (2017) 171:564. doi: 10.1001/jamapediatrics.2017.0238
Keywords: ventilation, respiratory support, ventilation-induced brain injury, neurodevelopment, preterm
Citation: Chan KYY, Miller SL, Schmölzer GM, Stojanovska V and Polglase GR (2020) Respiratory Support of the Preterm Neonate: Lessons About Ventilation-Induced Brain Injury From Large Animal Models. Front. Neurol. 11:862. doi: 10.3389/fneur.2020.00862
Received: 10 February 2020; Accepted: 07 July 2020;
Published: 14 August 2020.
Edited by:
Francisco J. Alvarez, Hospital de Cruces, SpainReviewed by:
Ting Guo, Hospital for Sick Children, CanadaDonna M. Ferriero, University of California, San Francisco, United States
Copyright © 2020 Chan, Miller, Schmölzer, Stojanovska and Polglase. This is an open-access article distributed under the terms of the Creative Commons Attribution License (CC BY). The use, distribution or reproduction in other forums is permitted, provided the original author(s) and the copyright owner(s) are credited and that the original publication in this journal is cited, in accordance with accepted academic practice. No use, distribution or reproduction is permitted which does not comply with these terms.
*Correspondence: Graeme R. Polglase, Z3JhZW1lLnBvbGdsYXNlQG1vbmFzaC5lZHU=
†These authors have contributed equally to this work