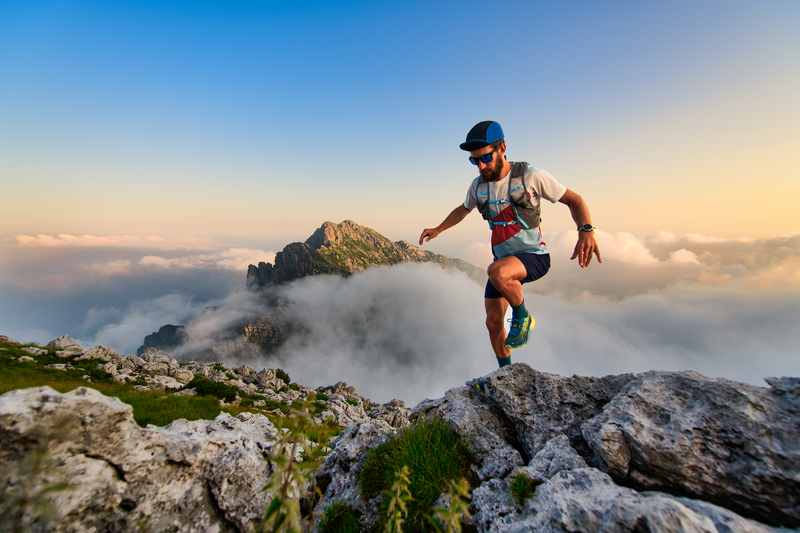
94% of researchers rate our articles as excellent or good
Learn more about the work of our research integrity team to safeguard the quality of each article we publish.
Find out more
REVIEW article
Front. Neurol. , 30 June 2020
Sec. Neurorehabilitation
Volume 11 - 2020 | https://doi.org/10.3389/fneur.2020.00607
This article is part of the Research Topic Brain Plasticity and Contribution of the Emotional Brain to Neural Remodelling after Injury View all 6 articles
There is increasing evidence that neuroplastic changes can occur even years after spinal cord injury, leading to reduced disability and better health which should reduce the cost of healthcare. In motor-incomplete spinal cord injury, recovery of leg function may occur if repetitive training causes afferent input to the lumbar spinal cord. The afferent input may be due to activity-based therapy without electrical stimulation but we present evidence that it is faster with electrical stimulation. This may be spinal cord stimulation or peripheral nerve stimulation. Recovery is faster if the stimulation is phasic and that the patient is trying to use their legs during the training. All the published studies are small, so all conclusions are provisional, but it appears that patients with more disability (AIS A and B) may need to continue using stimulation and for them, an implanted stimulator is likely to be convenient. Patients with less disability (AIS C and D) may make useful recovery and improve their quality of life from a course of therapy. This might be locomotion therapy but we argue that cycling with electrical stimulation, which uses biofeedback to encourage descending drive, causes rapid recovery and might be used with little supervision at home, making it much less expensive. Such an electrical therapy followed by conventional physiotherapy might be affordable for the many people living with chronic SCI. To put this in perspective, we present some information about what treatments are funded in the UK and the US.
Life expectancy after thoracic Spinal Cord Injury (SCI) is now close to normal in the western world (1). Around the time of the Second World War, the expectation was that there could be no recovery from SCI after the acute period: Cajal (2) had stated that neurons in the spinal cord were incapable of regeneration after injury. However, the Sygen study, carried out in the 1990s, showed that substantial spontaneous recovery was occurring in the months after injury (3): in people with incomplete injuries, motor scores reached a plateau 16 weeks after injury.
Sherwood et al. (4) showed, by neurophysiological tests, that 74/88 (84%) of patients diagnosed as having “complete” injuries, based on clinical tests, in fact retained some functional neurons passing the lesion; they proposed to describe these patients as discomplete. These spared neurons provide an opportunity for long-term recovery in people living with chronic incomplete and discomplete SCI. Indeed, Bareyre et al. (5) showed, in rats with a hemisection of the cord, that corticospinal neurons on the lesion side could sprout to make functional connections below the lesion. Friedli et al. (6) found, in 400 patients with tetraplegia, that asymmetry in the lesion allowed greater recovery, and also that, in monkeys, recovery correlated with corticospinal “detour circuits” below the injury. Many animal studies have demonstrated recovery after SCI following treadmill training, and this has reinforced the view that Activity-Based Therapy may enable patients with incomplete lesions to walk (7). Hubli and Dietz (8) described the knowledge gained as a “physiological basis for neurorehabilitation: a sufficient combination of sensory cues is required to generate and improve locomotor patterns.”
Many methods for rehabilitation have been tested, however, it is still far from clear what is most effective, or even what exactly the goal of the rehabilitation should be. This paper provides a historic review of rehabilitative interventions that incorporate electrical stimulation (ES), targeting lower limb peripheral nerves or nerve roots, combined with activity-based rehabilitation. Differences in approach are considered, in terms of electrode location, stimulation parameters (pattern and intensity), activity-based rehabilitation type and descending drive. We attempt to identify which factors might be most influential in achieving functional recovery; to that end, the review is focused on studies that incorporate International Standards for Neurological Classification of SCI (ISNCSCI) motor scores as an outcome measure. How, in the future, these interventions may be used more widely both for those with motor complete [ASIA Impairment Scale (AIS) A and B] and motor incomplete (AIS C and D) SCI, is then discussed.
Normal terminology used with ES is introduced in Table 1.
Table 1. Terms used in this field are often inexact, imprecise or even misleading. In this review, we are using the customary terms but offer this table in explanation.
Functional Electrical Stimulation (FES) describes stimulation through electrodes placed transcutaneously over peripheral nerves or muscle bellies, which activate mixed peripheral nerves as they branch into the muscle. FES may also describe stimulation through implanted electrodes placed on ventral nerve roots. FES after SCI has been applied in many clinical and experimental settings since the early 1970s. Primarily, lower-limb FES has been used to achieve walking and cycling; these activities form the focus of this review.
Liberson et al. (9) described a neuroprosthesis for correcting foot-drop in 1961. Patients with hemiplegia due to lesions in the brain or spinal cord caused by SCI, stroke, multiple sclerosis, and other conditions, had a device which stimulated the peroneal nerve during the swing phase of gait, lifting the foot clear of the ground. It took several decades before this treatment became established in clinical practice, but it is now broadly available. The device may use electrodes fixed on the skin over the nerve or implanted; the latter is helpful for people who are allergic to the self-adhesive electrodes, or who find it difficult to position the electrodes with sufficient accuracy.
When these neuroprostheses were developed, stimulation of the motor neurons was the aim, however, another important effect was inhibition of the plantarflexors (10); foot-drop often being caused by spasticity in these strong antagonists. Another discovery was a so-called carry-over effect (11). Patients with stroke who had been using the device for some time found that it was no longer necessary. Using it had caused sufficient recovery for them to be able to walk without the device. For them, a neuroprosthesis had acted as a therapy.
Between about 1975 and 2000, an active goal of research was to develop motor neuroprostheses to enable paraplegics with complete spinal cord lesions to walk during activities of daily living. Because most of the leg muscles in these patients will contract if stimulated, it seemed reasonable that if enough channels of stimulation were available, crude walking should be possible. Groups in Vienna (12, 13), London (14–17), and Cleveland (18) developed multi-channel stimulators and implanted them into volunteers. The results were disappointing; no really practical prosthesis was demonstrated. The three main reasons for lack of success were the following.
i. Rapid muscle fatigue. The force produced by electrically-stimulated muscle declines rapidly, limiting the endurance for activities of daily living. This decline is usually attributed to the inverse recruitment of the stimulated nerve: because the larger axons are stimulated first, as stimulus intensity increases, fast muscle fibers are activated before slow fibers, which is opposite to the normal order (19). However, this explanation is insufficient. When SCI people are trained enough, their muscles can be converted largely to more oxidative fiber-types, and yet they still fatigue rapidly (20, 21).
ii. There is no satisfactory site for electrodes. Electrodes could be: (a) self-adhesive electrodes on the skin over the target muscles; (b) the tips of percutaneous wires that are inserted into the muscles through the skin (22); (c) electrodes, usually in an insulating cuff, surgically-implanted on the nerve; or (d) electrodes on the anterior nerve roots in the spinal canal. The disadvantages of each are: (a) too tedious to don and doff the electrodes every day, and not selective enough; (b) too dangerous due to infections and broken wires; (c) requires very extensive surgery with associate risk and poor reliability; (d) not possible to get enough different combinations of muscles for several useful functions (16).
iii. Little progress was made with the user interface. An early concept was that motor signals would be taken from the motor cortex and used to drive the neuroprosthesis. For lower limb rehabilitation, this does not seem ethically justifiable and, in practice, when stepping was controlled by the patient, it was usually by manually-operated switches on whatever was held to provide balance (usually a walking frame) (23). For the very crude stepping that was achieved, switches were adequate, but perhaps they would not do outside the clinic when confronted with curbs, bends or rough ground. For outdoor walking, some method that gives greater range of control, would be necessary, and this should ideally be innate.
At the same time, locomotor treadmill training (LTT), incorporating body-weight-support and therapist- or robotic-assistive step training, had become popular. Supported LTT was thought to improve function through the responsiveness of central pattern generators (CPG) to afferent stimuli noted in mammalian quadrupeds (24, 25). In people with chronic SCI, improvements in walking function (26–30) and muscle properties (31, 32) were reported after LTT, however a systematic review conducted in 2013 concluded that evidence on the effectiveness of locomotor therapy was limited (33). Few LTT studies have reported changes in ISNCSCI motor scores. One recent study did report motor scores from 60 participants with motor incomplete SCI (0.1–45 years post-injury) after 120 sessions of body-weight supported LTT (34). The training time averaged 11 months (sessions every 3 days), and the mean improvement in ISNCSCI motor scores was 6 points. LTT has also been combined with FES, and improvements in gait parameters were found to be slightly better than LTT combined with manual assistance (33). Field-Fote and Roach (30) reported improvements in walking function and lower extremity motor scores following 12 weeks of LTT combined with FES, training 5 days/week, but the improvements were not superior to LTT with manual or robotic assistance, or overground walking training without FES. Possover (35) used an implanted neuroprosthesis (electrodes were placed on the pelvic, sacral and femoral nerves) to provide FES during locomotor training in 4 people with SCI (AIS A—C). Impressive improvements in ISNCSCI motor scores were noted following 12 months of training (mean 12.8 points), and three participants became capable of voluntary weight-bearing standing and walking a few meters with a walker without FES (35).
Enabling people to cycle on a recumbent tricycle or exercise ergometer is much simpler than FES-walking and supported-LTT. The feet are fixed to the pedals, constraining their motion, and the moving mass of the mobile tricycle or flywheel in the ergometer smoothens the movement. In the 1980s, Petrofsky et al. (36) first described the development of a “computerized, closed loop control stimulator, and cycle ergometer for people with SCI.” Each of the main muscle groups (e.g., knee extensors, hip flexors, etc.) should be stimulated for a range of crank angle over which the muscles contribute positive propulsion, which is easy to understand and implement. Another advantage is that, while seated with the machine, falling is not likely.
Many FES cycling studies were subsequently carried out, where the purpose was to use the large paralyzed muscles as healthy exercise: benefits to muscle properties are now well-established (20, 37–51), and cardiopulmonary benefits have also been reported (40, 44, 45, 52–57). Based on these findings FES has become standard of care after SCI in clinical settings; cycle ergometers are mostly used, which have a motor to establish a cadence and the FES reinforces that motion unless there is spasticity. These studies typically recruited people with complete injuries only, to avoid discomfort caused by mixed peripheral nerve stimulation in people with incomplete SCI. At the time it was believed that motor recovery was unattainable in people with complete injuries, therefore it was seldom measured or reported.
In 2000 we reported a case study of an individual with incomplete SCI, who had been neurologically stable for 10 years before he started FES-cycling, averaging 22 min per day (58). His tricycle was usually on rollers indoors but sometimes he would cycle outdoors, and he increased his rides up to a maximum of 12 km. We discovered that he had then recovered some control over a previously completely paralyzed knee. He said that this improvement enabled him to move round in his kitchen with only one stick, instead of two, and he became able to pick objects up from the floor while standing. Needless to say, these gains were not rehabilitation targets but they were significant improvements to his quality of life.
Soon after, McDonald et al. (59) reported a case study on an individual with motor complete SCI (AIS A) who initiated a program of “activity-based rehabilitation,” consisting of FES cycling supplemented with ES of abdominal and upper limb muscles, 5 years post-injury. Over 3 years of therapy, he recovered substantial motor function (ISNCSCI motor scores improved from 0/100 to 20/100), and his AIS Grade improved from A to C. The same group later reported motor recovery in a group of 25 people with chronic complete and incomplete SCI (17 were AIS A) who carried out FES cycling 3 times/week for an average of 30 months (60). Their ISNCSCI motor scores improved by an average of 8.0 points, compared with an average change of 0.6 points in the control group (n = 20) who only received range of motion and stretching exercises.
Following these discoveries, we developed an ergometer for FES-cycling with virtual reality (VR) to encourage volitional effort, the iCycle. Its unique feature is that there is biofeedback to the VR so that the speed of the avatar depends on the real crankshaft torque when there is no torque due to stimulation. In a small clinical trial, we noted an improvement in ISNCSCI motor scores in people with chronic, incomplete SCI (AIS C and D) after 1 month of training on the iCycle, training 3 times/week. The mean improvement was 1.5 points after training, and 4.7 points after follow up (1 month later) (61).
Our data can be compared with the data presented by Yaşar et al. (62) from a trial on 10 people, also with chronic incomplete SCI (AIS C and D). FES cycling was carried out 3 times/week for 3 months with follow-up 3 months later. In that study, the participants were instructed not to contribute to the cycling voluntarily, but just allow the stimulation to occur. The mean change in ISNCSCI motor score at the end of therapy and follow-up were nearly equal to our iCycle data, except the rate of improvement was three times greater with the iCycle (Figure 1). This supports our idea that voluntary drive, encouraged by the virtual reality and biofeedback, does promote recovery. However, our data is equivocal, we found no correlation between the change in motor score and dose (time spent cycling).
Figure 1. Comparison of the rate of change of ISNCSCI motor score for six therapies from Table 2. These have n > 1 and non-negligible improvement in motor score. We excluded Sadowsky et al. (60) because of the very wide range of training in that study. LT without electrical stimulation (34), FES-Cycling (62), FES-Cycling with virtual reality and biofeedback (61), SCS Neuroprosthesis (63), FES Neuroprosthesis (35) and SCS with drug and zero-gravity training (64). EOT, End of Therapy; FU, Follow-Up.
Passive cycling, without stimulation, has been investigated as a therapy and the literature recently reviewed by Phadke et al. (65). There are cardiovascular, musculoskeletal and neurological effects, the latter being changes to the H-reflex and spasticity, but none of the studies measured neurological outcome after multiple sessions to see whether there were changes in ISNCSCI motor score.
Table 2. Treatment of SCI patients with chronic injuries by electrical stimulation: change in ISNCSCI Motor Score.
Spinal Cord Stimulation (SCS) describes stimulation through electrodes implanted in the epidural space inside the spinal canal or placed transcutaneously over the spine. Epidural SCS was first tested in 1967 by Shealy et al. (66), who described it as dorsal column stimulation, as a way to alleviate chronic pain. It is now well-established as a clinical treatment for pain using chronic implanted stimulators (67). Its effects on the motor system were noticed in the 1970s, notably reduction in spasticity and partial recovery. Its history has been reviewed by Minassian et al. (68). Briefly, in 1998, Dimitrievic et al. (69) showed that SCS can excite the CPG in humans after SCI; the neural circuits within the lumbar cord which give coordinated contractions of the leg muscles appropriate for stepping. Low-intensity stimulation, below a level necessary to produce any such motor response in the paralyzed muscles, may enable a discomplete patient to produce EMG responses by volition. Dimitrievic proposed that this offered a therapeutic pathway for SCI in 1984 (70). The mechanisms of recovery have been reviewed more recently by Taccola et al. (71).
The terms dorsal column stimulation and spinal cord stimulation may actually be inaccurate. While, within the chronic pain literature, SCS is widely considered to activate dorsal column fibers both orthodromically and antidromically [for review see (72)], others have shown that, with electrodes on the dura or even on the back, the neurons that are first stimulated within the spine are the large diameter sensory fibers of the posterior roots (73–75). These include A-alpha fibers conducting proprioceptive feedback from the muscle spindle afferents. Most likely, the activation of multiple structures, such as dorsal column fibers, the dorsal horn and posterior/ventral roots, contribute to the overall effects of SCS. In the studies reviewed here, the peripheral nerves (posterior roots) are targeted, and the susceptibility of evoked potentials to homosynaptic depression is typically used to verify posterior root activation (76, 77).
The activation of CPGs by non-invasive tonic SCS has been demonstrated in able-bodied people (78, 79). In these experiments, the limbs were suspended to remove afferent input, and the participants are asked not to voluntarily contribute to the movement, to minimize supraspinal influence. Locomotor-like behavior in response to the tonic SCS occurred in only 7 out of 65 participants tested (79); supraspinally mediated inhibition of the spinal circuitry may have prevented activation of CPGs in the majority of able-bodied participants. In people with SCI, where descending input has been compromised, CPGs have been activated more robustly with both non-invasive (64) and invasive (69) tonic SCS. There are also several observations that tonic invasive and non-invasive SCS enables descending motor control; people with chronic SCI were able to generate voluntarily-driven movements and step-like activity in the presence of SCS, which were otherwise not possible (64, 80–88).
Carhart et al. (88) tested the use of an implanted stimulator for SCS in one volunteer with incomplete cervical injury who trained with tonic stimulation on a treadmill with partial body-weight support. He trained five times per week for 150 sessions and changed from being a wheelchair-user to being a community walker, however his ISNCSCI motor Score remained 15/50. Angeli et al. (86, 89) reported a similar method, stimulation being applied tonically to four patients with chronic injuries, two AIS A and two AIS B. The first of these patients had been described in an earlier case study paper (82). All four had 40–45 sessions lasting 2 h of treadmill training before implantation. The stimulation through the 16-electrode array was optimized and then used chronically. After implantation, they trained once or twice per day, walking on the treadmill, walking over ground, or standing. While the stimulator was on, the AIS A's became able to stand with support while the AIS B's could also walk over ground. The AIS B's took 278 and 81 training sessions, respectively to reach this outcome. In only one of the four did the motor score change as a result of this training: one of the AIS B's changed from 0/50 to 1/50, the other remained 0/50. This method may be described as neuromodulation because tonic stimulation is only exciting the spinal cord while control is from the patient's brain (at least when the therapists no longer intervened). This is an intriguing result because the functional improvements were substantial with the stimulator on, despite almost no change in motor score.
Wagner et al. (63) used a neuroprosthetic approach with SCS to rehabilitate three patients (AIS C or D) for walking. They used similar epidural electrode arrays over the lumbar cord but used “spatio-temporal stimulation.” For each leg, the gait cycle was divided into three phases in which different motor neuron pools were targeted. In each phase the combination of electrodes, stimulation currents and frequencies were optimized. All three patients could take steps as soon as this epidural stimulation was started. Their therapy lasted 5 months, five sessions per week after which 2/3 could do overground walking with handles for balance. They had all gained some ability to move their legs without the stimulation and their ISNCSCI motor scores had increased by 16, 11, and 4 points. When walking outside, they wore accelerometers on their shoes for phasing and a belt-worn controller that could be commanded by voice.
The studies discussed in this review, which incorporated INSCSCI motor scores as an outcome measure, have been summarized in Table 2. All the results are from chronically-injured people. The papers have been ranked by change in INSCSCI motor score. Figure 1 shows the rate of change of motor score for some of these studies.
In this review, we have described the rehabilitative interventions, incorporating electrical stimulation (ES) targeting lower limb peripheral nerves, that have evolved since the 1960s for people living with SCI. We have used the ISNCSCI motor scores as a primary outcome measure for neuroplastic effects because it is a clinically-relevant and a standardized measure of motor function after SCI. Changes in ISNCSCI motor scores represent therapeutic, neuroplastic effects of an ES-based intervention because ES is not used during the assessment. There is currently no consensus on a minimally important difference (MID) in ISNCSCI motor score, which could be considered as a clinically relevant improvement. Nevertheless, in Table 2, we have ranked the papers by change in INSCSCI motor score. Based on this table, the importance of the training dose, and the type of stimulation and training used, for optimal neuroplastic effects, will be discussed. A summary of the advantages and disadvantages of each technique is provided in Table 3. Finally we discuss costs to put the science in a healthcare context.
Table 3. A summary of the advantages and disadvantages of Functional Electrical Stimulation (FES) and Spinal Cord Stimulation (SCS) and the available methods.
As shown in Table 2, the number of training sessions per week, and total number of training sessions completed, varied widely between studies. It is notable that the studies reporting the largest improvement in motor scores incorporated the highest frequency of sessions (5–7 sessions per week) and aggregate number of training sessions, pointing toward a dose effect. However, this does not hold true for the studies by Angeli (89) and Carhart (88), which reported no motor recovery despite a high dose of training; intensive LTT was provided without and then with SCS. While these studies reported remarkable improvements in standing and walking function in the presence of SCS, these improvements did not translate to improvements in motor scores.
A study by Harness et al. (90) explored dose effects in two groups of SCI participants: one experimental group (n = 21) who underwent “intense exercise” (actually six different exercises), and a control group (n = 8) who underwent self-regulated exercise, for 6 months. The control group exercised nearly twice as often, but the time per week was similar. The subjects in the experimental group gained in motor scores (by 5 points), whereas the control group did not show any gains. A dose effect was found in the experimental group only (R = 0.53; p < 0.02). They concluded that unstructured exercise might have less value for return of motor function in people with chronic SCI (90).
Clearly there is not a simple relationship between the dose of an intervention (session duration, weekly session frequency and total number of training sessions), and the outcome in terms of recovery of motor function. The stimulation parameters, and type of training carried out may have important affects in this relationship.
The key difference between FES and SCS is the anatomical sites where the electrodes are placed: close to the cord or close to the muscle. Despite this difference, both techniques activate large-to-medium diameter afferent fibers: afferent input is crucial to induce long-term plasticity, thus it is probable that similar mechanisms contribute to neuroplastic recovery with FES and SCS. Because SCS electrodes are often arranged so that all the lumbar roots can be stimulated, whereas FES electrodes are generally not applied to most lower limb muscles, it should be easier to directly activate more large afferents by SCS (75, 91, 92) than FES. However, there is an opposing effect which has been investigated by Formento et al. (92). Proprioceptive feedback (including the activation of smaller sensory fibers that are not usually targeted by the direct electrical stimulus), which is crucial in the control of human movement, may be substantially blocked during SCS. When axons are stimulated, action potentials propagate in both directions; in the large afferent fibers, the orthodromic action potentials travel to the synapses in the spinal cord while the antidromic travel toward the receptors in the periphery. If the antidromic action potentials meet naturally-generated orthodromic action potentials, they will annihilate each other—an effect called collision blocking. Because the largest afferent fibers are from the muscle spindles, this blocking causes loss of proprioception. The amount of loss depends on the proportion of these afferent fibers being stimulated, the stimulation frequency, and the position on the stimulating electrode along the nerve; the closer the electrode is to the spinal cord, the greater the blocking effect. Experiments showing this effect have been described by Formento et al. (93).
Loss of proprioception is not only a disadvantage for the patients' immediate motor control but may be fundamental to neuroplastic recovery. Takeoka (94) showed mutant mice, lacking muscle spindle afferents, could not recover from SCI like wild mice because the rearranged descending circuits were defective. It is thought that the monosynaptic connection between the spindle afferents and the motor neurons may be critical. This may go some way to explain why no changes in ISNCSCI motor score were achieved in the studies by Carhart (88) and Angeli (89) (Table 2), despite impressive functional recovery while stimulation was on. Given this disadvantage to SCS, Formento et al. (93) proposed a method of stimulation that mitigates the effect of collision blocking (use high frequencies at low amplitudes) that allows some proprioceptive feedback. However, setting up this stimulation is complicated and this difficulty may be an obstacle to widespread use because it will deter clinicians (95). The application of SCS phasically may, at least in part, overcome collision blocking.
The difference between electrodes being implanted or transcutaneous should also be considered, due to the possibility of activating different spinal and supraspinal structures with each technique. It can be noted from Table 2 that, among the studies applying phasic stimulation, those that used implanted electrodes achieved superior neuroplastic recovery compared with those using surface electrodes, which may be due to greater specificity being achieved with implanted compared to transcutaneous electrodes. The spread of stimulation during non-invasive FES causes activation of nearby, possibly antagonistic, muscles; this may hinder the intended functional activity. In addition, deep muscles cannot be activated by non-invasive FES. Implanted cuff electrodes placed on specific anterior nerve roots can be used to activate both deep and surface muscles, however it is still not selective enough, and there is a possible disadvantage of afferent fibers not being directly activated by the stimulus. With SCS, it has been shown that activation of the same afferent target neurons can be obtained with epidural and transcutaneous applications (75, 96), however, presumably greater specificity can still be achieved with epidural applications. In addition, transcutaneous SCS causes simultaneous activation of trunk muscles, which can be uncomfortable for people with retained sensation, particularly during tonic SCS; this limits the stimulation intensity that can be applied.
FES is routinely applied phasically, at an intensity sufficient to induce a motor response (i.e., supra-threshold). A single session of repetitive (phasic) supra-threshold stimulation (at 10–200 Hz), applied over a peripheral nerve, has been shown to transiently increase net corticospinal excitability (increased Motor Evoked Potential (MEP) amplitude) in both able-bodied people (97–100) and in people with incomplete SCI (101) due, at least in part, to reducing the activity of inhibitory cortical neurons (102, 103) [at a spinal level, FES has been shown to have either minimal or mainly inhibitory effects (104–107)]. A single session of tonic, sub-threshold peripheral nerve stimulation (known as TENS) has been shown to have the opposite effect, transiently reducing corticospinal excitability (108), with similar inhibitory effects at a spinal level (107, 109–111). Both FES (112) and TENS (113) have been found to be effective in the treatment of spasticity after SCI.
SCS is typically applied tonically rather than phasically, and the effects of tonic SCS on corticospinal excitability in humans has not been widely explored. One case report in a person with motor incomplete SCI observed increased corticospinal excitability following 14 sessions of tonic, low frequency (0.2 Hz) SCS applied in 10-min bursts of sub- and supra-threshold intensity (114). Although tonic SCS, applied at a supra-threshold intensity (at 15–50 Hz) initiates rhythmic CPG activity (69), tonic SCS is more often provided at sub-threshold intensities: at an intensity that enables voluntarily-driven movements in people with motor complete and incomplete SCI (64, 80–88). It is believed that this form of tonic SCS raises excitability in spinal circuitry, such that it becomes more responsive to descending input. However, in the pain management field, extensive animal research indicates that tonic, sub-threshold SCS induces or modifies the release of neuroactive substances involving GABAergic and cholinergic mechanisms, inhibiting hyperexcitability at the dorsal horn. Activation of supraspinal circuitry is also thought to contribute substantially to the attenuation of neural hyperexcitability via descending inhibitory tracts [for review see (72)]. SCS has also been reported to attenuate neural hyperexcitability and recover spinal inhibitory control in people with SCI (115–117), and has been successfully used in the treatment of spasticity (118, 119). The effects of tonic, subthreshold SCS on spinal hyperexcitability may be an important mechanism enabling voluntarily-driven movements in people with chronic SCI. Further work is required to elucidate the effects of sub-threshold SCS and FES, applied tonically or phasically, on spinal and corticospinal excitability.
Table 2 shows that both FES and SCS cause improvements in motor score, but neurological recovery (change in ISNCSCI scores) tends to be greater with phasic stimulation. It is difficult to compare the two recent (2018) SCS studies that used implanted electrodes; one applied tonic (89) and the other phasic (63) stimulation, but both appeared to be sub-threshold: at a level that enabled volitional control. The subjects in the study by Angeli et al. (89) were AIS A and B; they used tonic stimulation, showed little neurological recovery, but, with ES, could perform useful functions that were otherwise impossible. In contrast, the subjects in the study by Wagner et al. (63) were AIS C or D, their neuroprosthesis stimulated phasically, and rapid neurological change occurred allowing some new possible function without ES although function was better with ES. To what degree the greater recovery in the latter study is due to the phasic stimulation, and what is due to lesser initial paralysis, cannot yet be known. We await further results for each method with patients of all AIS grades.
The neuroprostheses described by Wagner et al. (63), which stimulates through epidural electrodes, can be compared to the implantable FES (motor) neuroprostheses from the 1980s. This new device solves all the three problems that beset those FES implants: movement is volitional, the leg movements being natural but reinforced by the stimulation; all the flexor and extensor motor neuron pools can be excited from this centrally-implanted electrode array; and finally, muscle fatigue should be slower because the motor neurons will be recruited in the natural order. The third point is, as far as we know, still only expectation based on normal physiology (120); actual rates of fatigue in SCI people have not been measured. Herman et al. (81) claimed in his case study that the patient's metabolism changed away from glycolysis with SCS, implying normal recruitment. Thus, the neuroprosthesis from Courtine's group (63) is a significant advance in neuroprosthetics. Making the system more convenient by implanting the accelerometers would not be a technical problem. It will be of great interest to see how well these devices work in people with motor complete SCI (AIS A and B); also, how well they are accepted, not just for gait training, but in ADL: will they actually be used instead of a wheelchair? What these studies do suggest is that after this much training, using the implants, the devices remain useful; their therapeutic effect has not been so great that they are no longer necessary, as was found in some 30% of patients with foot-drop (11).
Locomotor Training has been popular for treating patients with SCI in recent years. One reason is probably that both patients and their specialist clinicians put functional walking high in a wish list after bowel and bladder control (121).
Another reason is the discoveries made from experiments with spinally-injured animals. The isolated spinal cord can generate stance and gait when animals are tested on treadmills, showing automatic adjustment to belt speed, belt direction and body weight. This motor activity may be entirely driven by afferent input. The function must be trained by repetition following the injury and this training may be functionally-specific so that animals trained to walk can walk but not stand while those trained to stand will stand well but walk badly (122). The argument for LTT has been that the patients should do walk-training and that if there is some supraspinal input, these neurons may be able to engage with the spinal circuits that generate the gait, converting it from entirely afferent-driven toward normal, with both peripheral afferent input and sensory-motor signals between the lumbar cord and the brain.
In their review, Dietz and Fouad (120) claim that LTT functional training enhances the residual plasticity. Morrison's study (34) supports this claim, showing the functional improvements that accompanied the improved motor score from LTT without electrical stimulation. Results of combining LTT with ES are equivocal. The marked improvement seen by Herman et al. (81) in one person was not seen by Field-Fote and Roach (30) in their study.
However, Table 2 shows that a positive neuroplastic effect can be produced by walking or cycling, cycling with ES being faster than LTT with ES. Studies are now needed to find out what functional recovery occurs after FES-cycling therapies, perhaps both SCS and FES. It may be that cycling therapy needs to be followed by walk-training, for the patients to experience the full benefit from the neuroplastic recovery, but this might still be quicker and less costly than LTT alone.
In clinical use, robotic LTT and cycling (passive or combined with ES) are done with little or no incentive for voluntary drive. The legs are moved by a motor, constrained by attachment to the device, and no effort is required from the patient. This is advantageous (cheaper) because it requires minimal supervision or assistance from a therapist. While the passive leg movement by the motor provides useful proprioceptive feedback, there may be little supraspinal input due to the patient's effort. Voluntary drive is however inherent in some rehabilitative interventions, such as overground walking or LTT without robotic assistance, or it can be encouraged using biofeedback. If a person with a neurologically-complete lesion becomes able to produce contractions in their otherwise paralyzed muscles in the presence of sub-threshold SCS, as has been observed in SCS studies (86, 123), the demonstration would provide a strong incentive for therapy. If FES is being applied, the muscle contractions that are the direct response to the stimulation would mask voluntarily-produced muscle activity.
It has been hypothesized that the combination of ES with descending motor commands is essential for beneficial neuroplastic change: both afferent and antidromic electrical impulses in lower motor neurons have been proposed to have a facilitatory effect on anterior horn cells, increasing their probability of firing during descending voluntary drive, resulting in an Hebbian-type learning effect (124). This is supported by observations with paired associative stimulation, which found that increases in corticomotor excitability depend on the phase during the gait cycle (125) and the reported plasticity of sensorimotor cortical structures after repetitive ES over the common peroneal nerve (97), which are dependent on concurrent voluntary drive present at the time of FES (99).
Motor training consisting of voluntary movements has been found to be more effective than passive motor training in eliciting performance improvements and cortical reorganization (126). This may be explained by voluntary effort facilitating central nervous system excitability (127) due to increased excitability of cortical motoneurons (128) and intracortical facilitation (129). Indeed, in patients with stroke, ES used with rehabilitation interventions has been reported to be more effective when combined with instructions to the patient to try to co-activate the stimulated muscles voluntarily (130, 131). The comparison between the Yaşar study (62) and our own (61) supports that idea that voluntary drive, encouraged by the virtual reality and biofeedback, improves recovery rate during FES-cycling (61).
It has also been proposed that voluntary drive does not directly facilitate cortical activity, but instead reduces inhibition at the cortical level (132). When combing FES cycling with VR, we observed an unexpected reduction in power output at the start of the VR game in some patients (i.e., when the participant was instructed to contribute voluntarily to the cycling) (61). This was principally due to co-contraction of lower limb muscles presumably due to lower limb spasms, which may have been caused by reduced levels of descending inhibition. While the addition of voluntary drive appears to be effective in achieving neuroplasticity, these observations should be taken into consideration when designing the most suitable therapy for individuals with spasticity, and the incorporation of sub-threshold tonic stimulation may be beneficial.
LTT has clearly been shown to cause significant functional recovery in patients with AIS C and D injuries and this is maintained after the therapy (34, 133). For example, in the latter study, 45 participants did the 6-min walk test before and after at least 120 training sessions, increasing on average from 13 to 115 m. There were many accompanying improvements in autonomic function.
But what will healthcare systems pay for? In the UK, decisions about funding are based on subjective evaluation by the patients. The total value of a treatment depends on the product of the improvement in the quality of life and the number of years that it will apply. The unit is the Quality-Adjusted Life Year (QALY) and the National Health Service will usually pay about £25,000/QALY (134). The preferred measurement of Quality of Life is the SF-6D Health Utility Scale.
Lee et al. (135) used the SF-6D on 305 Australian patients with SCI. In the general Australian population, the mean score is 0.80; for paraplegics it was found to be 0.72, and for tetraplegics, 0.66. The differences between these means give some idea how much subjective improvement might be expected from treatment: a change equal to the difference between tetraplegia and paraplegia would probably be regarded as a remarkably successful therapy, yet is only a 6% improvement in quality of life. If, for example, we propose to treat people of middle age, and assume a life expectancy of 20 years, then, ignoring a discount rate, which is currently 1.5%, the QALY value would be 20 × 0.06 = 1.2. This would justify an expenditure of ~£30,000. What the actual gain in quality of life would be, will not be known until it is included in a clinical investigation.
Some of the studies cited involve 100–200 locomotor training sessions. In a recent paper, Behrman recommended at least 60, lasting 1½ h per day for successful Activity Based Therapy (7). Morrison (34) provides useful cost data from the US. Based on her figure, the cost of 120 LTT sessions, her preferred number, would be over $22k. The normal allowance from the health insurance companies in the US is 20 sessions of physiotherapy. She argues that given the improvement in walking ability and autonomic function seen in her participants, a longer course of therapy would actually save costs because of the reduced incidence of SCI complications, such as urinary tract infections and pressure sores.
This argument in favor of preventative treatment can be made in other countries and might prevail over subjective (QALY) reasoning. However, there is no doubt that LTT would take significant resourcing; Behrman et al. (7) advises: “Expanding staffing resources [and] equipment … are advantageous to successful delivery of the intervention,” implying that there will be additional estates costs also.
An alternative to LTT would be to use FES-cycling at home with some supervision from therapists. The frequency of the exercise would depend on the patient but could be done when convenient for them, and they could continue as long as the improvements justify the time taken. Changes in the motor score due to the neuroplastic response would not necessarily translate to useful functions for activities of daily living (transfers, stairs, etc.) but when the therapist can see what muscles have been restored to voluntary control, they can discuss with the patient what rehabilitation goals are realistic and might be achieved by conventional physiotherapy.
A comparison is needed between LTT, done with electrical stimulation assisted by physiotherapists, and FES-cycling done at home. It will be more complicated when neuromodulation of the posterior roots is added to the LTT, either using transcutaneous electrodes or implanted stimulators and epidural electrodes. In their single case study, Herman et al. (81) show clearly how walking speed improved when SCS was added to the LTT.
Having an implanted neuromodulator or neuroprosthesis, beside the extra cost of the device and surgery, is much more of commitment for hospital and patient: it may need adjustment from time to time, and might be used for the remainder of the patient's life. Of course, like footdrop stimulators, some patients may find that the therapeutic effect is so great that use of the device becomes unnecessary, but that does not seem to have been true for the 4 AIS A and B participants described by Angeli et al. (89).
The purpose of the implant might be to deliver a therapy for a limited period of time, after which it would be removed or simply left in place and no longer used. For devices that enable function, which otherwise would be impossible for the patient, this function might be an exercise, for example anaerobic exercise or muscle bulk maintenance, or it might allow activities of daily living. We expect that if the implant is to be successful, it should easily allow ADL and that these activities will bring the benefits of exercising the otherwise paralyzed muscles. People with SCI generally have less time than the general population for extra exercise. Facilitating use for ADL should be the goal for the therapists and implant designers.
In people with chronic incomplete and discomplete SCI, recovery of leg function may be possible with afferent input to the lumbar spinal cord caused by electrical stimulation combined with repetitive training. The stimulation may be either FES or SCS, but this should be phasic, synchronized to the flexion and extension of the legs while walking or cycling, and should coincide with descending voluntary drive, to optimize neuroplastic recovery. Tonic, sub-threshold SCS appears to be effective as a long-term intervention to enable volitional movements in people with motor complete SCI (AIS A and B), at least in part by restoring spinal and descending inhibitory control, but there is currently little evidence for neuroplastic recovery.
If training is done by cycling on a stationary machine, neuroplastic change is faster if there is virtual reality and biofeedback to motivate the patient. We attribute this to the importance of supraspinal drive which may otherwise be lacking. Cycle training should be less expensive than locomotor training because it could be done at home, however, it is an established point of view that patients using a wheelchair should learn to walk, if possible, and do so by locomotor training. Therefore, future research should investigate to what extent cycle training enables patients to attain useful rehabilitation goals and improve their quality of life.
Based on the small amount of evidence available, it appears that neuroprostheses that stimulate the posterior roots will give better functional performance compared to therapy alone. These neuroprostheses solve three major problems with FES implants. However, the cost of such implants is likely to be high, at least because of the regulatory requirements (136) and establishing clinical centers with sufficient expertise and long-term commitment may be a challenge.
Both authors contributed to the article and approved the submitted version.
The authors declare that the research was conducted in the absence of any commercial or financial relationships that could be construed as a potential conflict of interest.
The authors gratefully acknowledge the financial support of the INSPIRE FOUNDATION to our research projects related to this review.
1. Savic G, DeVivo MJ, Frankel HL, Jamous MA, Soni BM, Charlifue S. Long-term survival after traumatic spinal cord injury: a 70-year British study. Spinal Cord. (2017) 55:651–8. doi: 10.1038/sc.2017.23
2. Cajal SR. In: May RM, editor. Degeneration and Regeneration of the Nervous System. London: Oxford University Press (1928).
3. Geisler FH, Coleman WP, Grieco G, Poonian D. Measurements and recovery patterns in a multicenter study of acute spinal cord injury. Spine. (2001) 26:S68–86. doi: 10.1097/00007632-200112151-00014
4. Sherwood AM, Dimitrijevic MR, McKay WB. Evidence of subclinical brain influence in clinically complete spinal cord injury: discomplete SCI. J Neurol Sci. (1992) 110:90–8. doi: 10.1016/0022-510X(92)90014-C
5. Bareyre FM, Kerschensteiner M, Raineteau O, Mettenleiter TC, Weinmann O, Schwab ME. The injured spinal cord spontaneously forms a new intraspinal circuit in adult rats. Nat Neurosci. (2004) 7:269–77. doi: 10.1038/nn1195
6. Friedli L, Rosenzweig ES, Barraud Q, Schubert M, Dominici N, Awai L, et al. Pronounced species divergence in corticospinal tract reorganization and functional recovery after lateralized spinal cord injury favors primates. Sci Transl Med. (2015) 7:302ra134. doi: 10.1126/scitranslmed.aac5811
7. Behrman AL, Ardolino EM, Harkema SJ. Activity-based therapy: from basic science to clinical application for recovery after spinal cord Injury. J Neurol Phys Ther. (2017) 41:S39–S45. doi: 10.1097/NPT.0000000000000184
8. Hubli M, Dietz V. The physiological basis of neurorehabilitation–locomotor training after spinal cord injury. J Neuroeng Rehabil. (2013) 10:5. doi: 10.1186/1743-0003-10-5
9. Liberson WT, Holmquest HJ, Scot D, Dow M. Functional electrotherapy: stimulation of the peroneal nerve synchronized with the swing phase of the gait of hemiplegic patients. Arch Phys Med Rehabil. (1961) 42:101–5.
10. Burridge JH, McLellan DL. Relation between abnormal patterns of muscle activation and response to common peroneal nerve stimulation in hemiplegia. J Neurol Neurosurg Psychiatry. (2000) 69:353–61. doi: 10.1136/jnnp.69.3.353
11. Taylor PN, Burridge JH, Dunkerley AL, Wood DE, Norton JA, Singleton C, et al. Clinical use of the Odstock dropped foot stimulator: its effect on the speed and effort of walking. Arch Phys Med Rehabil. (1999) 80:1577–83. doi: 10.1016/S0003-9993(99)90333-7
12. Thoma H, Benzer H, Holle J, Moritz E, Pauser G. Method and clinical use of functional electrostimulation. Biomed Tech (Berl). (1979) 24:4–10. doi: 10.1515/bmte.1979.24.1-2.4
13. Thoma H, Frey M, Gruber H, Holle J, Kern H, Reiner E, et al. First implantation of a 16-channel electric stimulation device in the human body. Trans Am Soc Artif Intern Organs. (1983) 29:301–6.
14. Brindley GS, Polkey CE, Rushton DN. Electrical splinting of the knee in paraplegia. Paraplegia. (1979) 16:428–37. doi: 10.1038/sc.1978.78
15. Rushton DN, Donaldson ND, Barr FM, Harper VJ, Perkins TA, Taylor PN, et al. Lumbar root stimulation for restoring leg function: results in paraplegia. Artif Organs. (1997) 21:180–2. doi: 10.1111/j.1525-1594.1997.tb04647.x
16. Donaldson N, Rushton D, Tromans T. Neuroprostheses for leg function after spinal-cord injury. Lancet. (1997) 350:711. doi: 10.1016/S0140-6736(97)24036-5
17. Perkins TA, Donaldson Nde N, Fitzwater R, Phillips GF, Wood DE. Abstracts from the second vienna international workshop on functional electrostimulation. September 21-24. (1986) Vienna, Austria. leg powered paraplegic cycling system using surface functional electrical stimulation. Artif Organs. (1988) 12:297. doi: 10.1111/j.1525-1594.1988.tb02769.x
18. Davis JA Jr, Triolo RJ, Uhlir J, Bieri C, Rohde L, Kukke S. Preliminary performance of a surgically implanted neuroprosthesis for standing and transfers–where do we stand? J Rehabil Res Dev. (2001) 38:609–17.
19. Henneman E, Somjen G, Carpenter DO. Functional significance of cell size in spinal motoneurons. J Neurophysiol. (1965) 28:560–80. doi: 10.1152/jn.1965.28.3.560
20. Duffell LD, Rowlerson AM, Donaldson N, Harridge SD, Newham DJ. Effects of endurance and strength-directed electrical stimulation training on the performance and histological properties of paralyzed human muscle: a pilot study. Muscle Nerve. (2010) 42:756–63. doi: 10.1002/mus.21746
21. Duffell LD, Donaldson N, Newham DJ. Power output during functional electrically stimulated cycling in trained spinal cord injured people. Neuromodulation. (2010) 13:50–7. doi: 10.1111/j.1525-1403.2009.00245.x
22. Marsolais EB, Kobetic R. Functional electrical stimulation for walking in paraplegia. J Bone Joint Surg Am. (1987) 69:728–33. doi: 10.2106/00004623-198769050-00014
23. Thoma H, Frey M, Gruber H, Holle J, Kern H, Schwanda G, et al. Preview of abstracts fifth annual conference of the ieee engineering in medicine and biology society. Paraplegics should learn to walk with fingers. IEEE Trans Biomed Eng. (1983) 30:546–47. doi: 10.1109/TBME.1983.325152
24. Grillner S. Neurobiological bases of rhythmic motor acts in vertebrates. Science. (1985) 228:143–9. doi: 10.1126/science.3975635
25. Barbeau H, Rossignol S. Recovery of locomotion after chronic spinalization in the adult cat. Brain Res. (1987) 412:84–95. doi: 10.1016/0006-8993(87)91442-9
26. Visintin M, Barbeau H. The effects of body weight support on the locomotor pattern of spastic paretic patients. Can J Neurol Sci. (1989) 16:315–25. doi: 10.1017/S0317167100029152
27. Wernig A, Muller S, Nanassy A, Cagol E. Laufband therapy based on 'rules of spinal locomotion' is effective in spinal cord injured persons. Eur J Neurosci. (1995) 7:823–9. doi: 10.1111/j.1460-9568.1995.tb00686.x
28. Wirz M, Zemon DH, Rupp R, Scheel A, Colombo G, Dietz V, et al. Effectiveness of automated locomotor training in patients with chronic incomplete spinal cord injury: a multicenter trial. Arch Phys Med Rehabil. (2005) 86:672–80. doi: 10.1016/j.apmr.2004.08.004
29. Alexeeva N, Sames C, Jacobs PL, Hobday L, Distasio MM, Mitchell SA, et al. Comparison of training methods to improve walking in persons with chronic spinal cord injury: a randomized clinical trial. J Spinal Cord Med. (2011) 34:362–79. doi: 10.1179/2045772311Y.0000000018
30. Field-Fote EC, Roach KE. Influence of a locomotor training approach on walking speed and distance in people with chronic spinal cord injury: a randomized clinical trial. Phys Ther. (2011) 91:48–60. doi: 10.2522/ptj.20090359
31. Thomas SL, Gorassini MA. Increases in corticospinal tract function by treadmill training after incomplete spinal cord injury. J Neurophysiol. (2005) 94:2844–55. doi: 10.1152/jn.00532.2005
32. Jayaraman A, Shah P, Gregory C, Bowden M, Stevens J, Bishop M, et al. Locomotor training and muscle function after incomplete spinal cord injury: case series. J Spinal Cord Med. (2008) 31:185–93. doi: 10.1080/10790268.2008.11760710
33. Morawietz C, Moffat F. Effects of locomotor training after incomplete spinal cord injury: a systematic review. Arch Phys Med Rehabil. (2013) 94:2297–308. doi: 10.1016/j.apmr.2013.06.023
34. Morrison SA, Lorenz D, Eskay CP, Forrest GF, Basso DM. Longitudinal recovery and reduced costs after 120 sessions of locomotor training for motor incomplete spinal cord injury. Arch Phys Med Rehabil. (2018) 99:555–62. doi: 10.1016/j.apmr.2017.10.003
35. Possover M. Recovery of sensory and supraspinal control of leg movement in people with chronic paraplegia: a case series. Arch Phys Med Rehabil. (2014) 95:610–4. doi: 10.1016/j.apmr.2013.10.030
36. Petrofsky JS, Heaton H 3rd, Phillips CA. Outdoor bicycle for exercise in paraplegics and quadriplegics. J Biomed Eng. (1983) 5:292–6. doi: 10.1016/0141-5425(83)90003-1
37. Petrofsky JS. Functional electrical stimulation and the rehabilitation of the spinal cord-injured patient. Adv Clin Rehabil. (1987) 1:115–36.
38. Pacy PJ, Hesp R, Halliday DA, Katz D, Cameron G, Reeve J. Muscle and bone in paraplegic patients, and the effect of functional electrical stimulation. Clin Sci (Lond). (1988) 75:481–7. doi: 10.1042/cs0750481
39. Ragnarsson KT, Pollack S, O'Daniel W Jr, Edgar R, Petrofsky J, et al. Clinical evaluation of computerized functional electrical stimulation after spinal cord injury: a multicenter pilot study. Arch Phys Med Rehabil. (1988) 69:672–7.
40. Arnold PB, McVey PP, Farrell WJ, Deurloo TM, Grasso AR. Functional electric stimulation: its efficacy and safety in improving pulmonary function and musculoskeletal fitness. Arch Phys Med Rehabil. (1992) 73:665–8.
41. Stein RB, Gordon T, Jefferson J, Sharfenberger A, Yang JF, de Zepetnek JT, et al. Optimal stimulation of paralyzed muscle after human spinal cord injury. J Appl Physiol. (1992) 72:1393–400. doi: 10.1152/jappl.1992.72.4.1393
42. Rochester L, Chandler CS, Johnson MA, Sutton RA, Miller S. Influence of electrical stimulation of the tibialis anterior muscle in paraplegic subjects. 1. Contractile properties. Paraplegia. (1995) 33:437–49. doi: 10.1038/sc.1995.97
43. Rochester L, Barron MJ, Chandler CS, Sutton RA, Miller S, Johnson MA. Influence of electrical stimulation of the tibialis anterior muscle in paraplegic subjects. 2. Morphological and histochemical properties. Paraplegia. (1995) 33:514–22. doi: 10.1038/sc.1995.112
44. Mohr T, Andersen JL, Biering-Sorensen F, Galbo H, Bangsbo J, Wagner A, et al. Long-term adaptation to electrically induced cycle training in severe spinal cord injured individuals. Spinal Cord. (1997) 35:1–16. doi: 10.1038/sj.sc.3100343
45. Hjeltnes N, Aksnes AK, Birkeland KI, Johansen J, Lannem A, Wallberg-Henriksson H. Improved body composition after 8 wk of electrically stimulated leg cycling in tetraplegic patients. Am J Physiol. (1997) 273:R1072–9. doi: 10.1152/ajpregu.1997.273.3.R1072
46. Scremin AM, Kurta L, Gentili A, Wiseman B, Perell K, Kunkel C, et al. Increasing muscle mass in spinal cord injured persons with a functional electrical stimulation exercise program. Arch Phys Med Rehabil. (1999) 80:1531–6. doi: 10.1016/S0003-9993(99)90326-X
47. Gerrits HL, de Haan A, Sargeant AJ, Dallmeijer A, Hopman MT. Altered contractile properties of the quadriceps muscle in people with spinal cord injury following functional electrical stimulated cycle training. Spinal Cord. (2000) 38:214–23. doi: 10.1038/sj.sc.3100974
48. Crameri RM, Weston A, Climstein M, Davis GM, Sutton JR. Effects of electrical stimulation-induced leg training on skeletal muscle adaptability in spinal cord injury. Scand J Med Sci Sports. (2002) 12:316–22. doi: 10.1034/j.1600-0838.2002.20106.x
49. Skold C, Lonn L, Harms-Ringdahl K, Hultling C, Levi R, Nash M, et al. Effects of functional electrical stimulation training for six months on body composition and spasticity in motor complete tetraplegic spinal cord-injured individuals. J Rehabil Med. (2002) 34:25–32. doi: 10.1080/165019702317242677
50. Gerrits HL, Hopman MT, Sargeant AJ, Jones DA, De Haan A. Effects of training on contractile properties of paralyzed quadriceps muscle. Muscle Nerve. (2002) 25:559–67. doi: 10.1002/mus.10071
51. Duffell LD, Donaldson N, Perkins TA, Rushton DN, Hunt KJ, Kakebeeke TH, et al. Long-term intensive electrically stimulated cycling by spinal cord-injured people: effect on muscle properties and their relation to power output. Muscle Nerve. (2008) 38:1304–11. doi: 10.1002/mus.21060
52. Pollack SF, Axen K, Spielholz N, Levin N, Haas F, Ragnarsson KT. Aerobic training effects of electrically induced lower extremity exercises in spinal cord injured people. Arch Phys Med Rehabil. (1989) 70:214–9.
53. Goss FL, McDermott A, Robertson RJ. Changes in peak oxygen uptake following computerized functional electrical stimulation in the spinal cord injured. Res Q Exerc Sport. (1992) 63:76–9. doi: 10.1080/02701367.1992.10607559
54. Hooker SP, Figoni SF, Rodgers MM, Glaser RM, Mathews T, Suryaprasad AG, et al. Physiologic effects of electrical stimulation leg cycle exercise training in spinal cord injured persons. Arch Phys Med Rehabil. (1992) 73:470–6.
55. Mutton DL, Scremin AM, Barstow TJ, Scott MD, Kunkel CF, Cagle TG. Physiologic responses during functional electrical stimulation leg cycling and hybrid exercise in spinal cord injured subjects. Arch Phys Med Rehabil. (1997) 78:712–8. doi: 10.1016/S0003-9993(97)90078-2
56. Kjaer M, Mohr T, Biering-Sorensen F, Bangsbo J. Muscle enzyme adaptation to training and tapering-off in spinal-cord-injured humans. Eur J Appl Physiol. (2001) 84:482–6. doi: 10.1007/s004210100386
57. Berry HR, Kakebeeke TH, Donaldson N, Perret C, Hunt KJ. Energetics of paraplegic cycling: adaptations to 12 months of high volume training. Technol Health Care. (2012) 20:73–84. doi: 10.3233/THC-2011-0656
58. Donaldson N, Perkins TA, Fitzwater R, Wood DE, Middleton F. FES cycling may promote recovery of leg function after incomplete spinal cord injury. Spinal Cord. (2000) 38:680–2. doi: 10.1038/sj.sc.3101072
59. McDonald JW, Becker D, Sadowsky CL, Jane JA Sr, Conturo TE, et al. Late recovery following spinal cord injury. Case report and review of the literature. J Neurosurg. (2002) 97:252–65. doi: 10.3171/spi.2002.97.2.0252
60. Sadowsky CL, Hammond ER, Strohl AB, Commean PK, Eby SA, Damiano DL, et al. Lower extremity functional electrical stimulation cycling promotes physical and functional recovery in chronic spinal cord injury. J Spinal Cord Med. (2013) 36:623–31. doi: 10.1179/2045772313Y.0000000101
61. Duffell LD, Paddison S, Alahmary AF, Donaldson N, Burridge J. The effects of FES cycling combined with virtual reality racing biofeedback on voluntary function after incomplete SCI: a pilot study. J Neuroeng Rehabil. (2019) 16:149. doi: 10.1186/s12984-019-0619-4
62. Yasar E, Yilmaz B, Goktepe S, Kesikburun S. The effect of functional electrical stimulation cycling on late functional improvement in patients with chronic incomplete spinal cord injury. Spinal Cord. (2015) 53:866–9. doi: 10.1038/sc.2015.19
63. Wagner FB, Mignardot JB, Le Goff-Mignardot CG, Demesmaeker R, Komi S, Capogrosso M, et al. Targeted neurotechnology restores walking in humans with spinal cord injury. Nature. (2018) 563:65–71. doi: 10.1038/s41586-018-0649-2
64. Gerasimenko YP, Lu DC, Modaber M, Zdunowski S, Gad P, Sayenko DG, et al. Noninvasive reactivation of motor descending control after paralysis. J Neurotrauma. (2015) 32:1968–80. doi: 10.1089/neu.2015.4008
65. Phadke CP, Vierira L, Mathur S, Cipriano G Jr, Ismail F, et al. Impact of passive leg cycling in persons with spinal cord injury: a systematic review. Top Spinal Cord Inj Rehabil. (2019) 25:83–96. doi: 10.1310/sci18-00020
66. Shealy CN, Mortimer JT, Reswick JB. Electrical inhibition of pain by stimulation of the dorsal columns: preliminary clinical report. Anesth Analg. (1967) 46:489–91. doi: 10.1213/00000539-196707000-00025
67. Simpson EL, Duenas A, Holmes MW, Papaioannou D, Chilcott J. Spinal cord stimulation for chronic pain of neuropathic or ischaemic origin: systematic review and economic evaluation. Health Technol Assess. (2009) 13:1–154. doi: 10.3310/hta13170
68. Minassian K, McKay WB, Binder H, Hofstoetter US. Targeting lumbar spinal neural circuitry by epidural stimulation to restore motor function after spinal cord injury. Neurotherapeutics. (2016) 13:284–94. doi: 10.1007/s13311-016-0421-y
69. Dimitrijevic MR, Gerasimenko Y, Pinter MM. Evidence for a spinal central pattern generator in humans. Ann N Y Acad Sci. (1998) 860:360–76. doi: 10.1111/j.1749-6632.1998.tb09062.x
70. Dimitrijevic MR, Dimitrijevic MM, Faganel J, Sherwood AM. Suprasegmentally induced motor unit activity in paralyzed muscles of patients with established spinal cord injury. Ann Neurol. (1984) 16:216–21. doi: 10.1002/ana.410160208
71. Taccola G, Sayenko D, Gad P, Gerasimenko Y, Edgerton VR. And yet it moves: recovery of volitional control after spinal cord injury. Prog Neurobiol. (2018) 160:64–81. doi: 10.1016/j.pneurobio.2017.10.004
72. Linderoth B, Foreman RD. Conventional and novel spinal stimulation algorithms: hypothetical mechanisms of action and comments on outcomes. Neuromodulation. (2017) 20:525–33. doi: 10.1111/ner.12624
73. Rattay F, Minassian K, Dimitrijevic MR. Epidural electrical stimulation of posterior structures of the human lumbosacral cord: 2. quantitative analysis by computer modeling. Spinal Cord. (2000) 38:473–89. doi: 10.1038/sj.sc.3101039
74. Capogrosso M, Wenger N, Raspopovic S, Musienko P, Beauparlant J, Bassi Luciani L, et al. A computational model for epidural electrical stimulation of spinal sensorimotor circuits. J Neurosci. (2013) 33:19326–40. doi: 10.1523/JNEUROSCI.1688-13.2013
75. Hofstoetter US, Freundl B, Binder H, Minassian K. Common neural structures activated by epidural and transcutaneous lumbar spinal cord stimulation: elicitation of posterior root-muscle reflexes. PLoS ONE. (2018) 13:e0192013. doi: 10.1371/journal.pone.0192013
76. Knikou M. Neurophysiological characterization of transpinal evoked potentials in human leg muscles. Bioelectromagnetics. (2013) 34:630–40. doi: 10.1002/bem.21808
77. Hofstoetter US, Freundl B, Binder H, Minassian K. Recovery cycles of posterior root-muscle reflexes evoked by transcutaneous spinal cord stimulation and of the H reflex in individuals with intact and injured spinal cord. PLoS ONE. (2019) 14:e0227057. doi: 10.1371/journal.pone.0227057
78. Gerasimenko Y, Gorodnichev R, Puhov A, Moshonkina T, Savochin A, Selionov V, et al. Initiation and modulation of locomotor circuitry output with multisite transcutaneous electrical stimulation of the spinal cord in noninjured humans. J Neurophysiol. (2015) 113:834–42. doi: 10.1152/jn.00609.2014
79. Gerasimenko Y, Gorodnichev R, Machueva E, Pivovarova E, Semyenov D, Savochin A, et al. Novel and direct access to the human locomotor spinal circuitry. J Neurosci. (2010) 30:3700–8. doi: 10.1523/JNEUROSCI.4751-09.2010
80. Barolat G, Myklebust JB, Wenninger W. Enhancement of voluntary motor function following spinal cord stimulation–case study. Appl Neurophysiol. (1986) 49:307–14. doi: 10.1159/000100160
81. Herman R, He J, D'Luzansky S, Willis W, Dilli S. Spinal cord stimulation facilitates functional walking in a chronic, incomplete spinal cord injured. Spinal Cord. (2002) 40:65–8. doi: 10.1038/sj.sc.3101263
82. Harkema S, Gerasimenko Y, Hodes J, Burdick J, Angeli C, Chen Y, et al. Effect of epidural stimulation of the lumbosacral spinal cord on voluntary movement, standing, and assisted stepping after motor complete paraplegia: a case study. Lancet. (2011) 377:1938–47. doi: 10.1016/S0140-6736(11)60547-3
83. Hofstoetter US, Krenn M, Danner SM, Hofer C, Kern H, McKay WB, et al. Augmentation of voluntary locomotor activity by transcutaneous spinal cord stimulation in motor-incomplete spinal cord-injured individuals. Artif Organs. (2015) 39:E176–86. doi: 10.1111/aor.12615
84. Calvert JS, Grahn PJ, Strommen JA, Lavrov IA, Beck LA, Gill ML, et al. Electrophysiological guidance of epidural electrode array implantation over the human lumbosacral spinal cord to enable motor function after chronic paralysis. J Neurotrauma. (2019) 36:1451–60. doi: 10.1089/neu.2018.5921
85. Grahn PJ, Lavrov IA, Sayenko DG, Van Straaten MG, Gill ML, Strommen JA, et al. Enabling task-specific volitional motor functions via spinal cord neuromodulation in a human with paraplegia. Mayo Clin Proc. (2017) 92:544–54. doi: 10.1016/j.mayocp.2017.02.014
86. Angeli CA, Edgerton VR, Gerasimenko YP, Harkema SJ. Altering spinal cord excitability enables voluntary movements after chronic complete paralysis in humans. Brain. (2014) 137:1394–409. doi: 10.1093/brain/awu038
87. Huang H, He J, Herman R, Carhart MR. Modulation effects of epidural spinal cord stimulation on muscle activities during walking. IEEE Trans Neural Syst Rehabil Eng. (2006) 14:14–23. doi: 10.1109/TNSRE.2005.862694
88. Carhart MR, He J, Herman R, D'Luzansky S, Willis WT. Epidural spinal-cord stimulation facilitates recovery of functional walking following incomplete spinal-cord injury. IEEE Trans Neural Syst Rehabil Eng. (2004) 12:32–42. doi: 10.1109/TNSRE.2003.822763
89. Angeli CA, Boakye M, Morton RA, Vogt J, Benton K, Chen Y, et al. Recovery of over-ground walking after chronic motor complete spinal cord injury. N Engl J Med. (2018) 379:1244–50. doi: 10.1056/NEJMoa1803588
90. Harness ET, Yozbatiran N, Cramer SC. Effects of intense exercise in chronic spinal cord injury. Spinal Cord. (2008) 46:733–7. doi: 10.1038/sc.2008.56
91. Minassian K, Jilge B, Rattay F, Pinter MM, Binder H, Gerstenbrand F, et al. Stepping-like movements in humans with complete spinal cord injury induced by epidural stimulation of the lumbar cord: electromyographic study of compound muscle action potentials. Spinal Cord. (2004) 42:401–16. doi: 10.1038/sj.sc.3101615
92. Minassian K, Persy I, Rattay F, Dimitrijevic MR, Hofer C, Kern H. Posterior root-muscle reflexes elicited by transcutaneous stimulation of the human lumbosacral cord. Muscle Nerve. (2007) 35:327–36. doi: 10.1002/mus.20700
93. Formento E, Minassian K, Wagner F, Mignardot JB, Le Goff-Mignardot CG, Rowald A, et al. Electrical spinal cord stimulation must preserve proprioception to enable locomotion in humans with spinal cord injury. Nat Neurosci. (2018) 21:1728–41. doi: 10.1038/s41593-018-0262-6
94. Takeoka A, Vollenweider I, Courtine G, Arber S. Muscle spindle feedback directs locomotor recovery and circuit reorganization after spinal cord injury. Cell. (2014) 159:1626–39. doi: 10.1016/j.cell.2014.11.019
95. Musselman KE, Shah M, Zariffa J. Rehabilitation technologies and interventions for individuals with spinal cord injury: translational potential of current trends. J Neuroeng Rehabil. (2018) 15:40. doi: 10.1186/s12984-018-0386-7
96. Ladenbauer J, Minassian K, Hofstoetter US, Dimitrijevic MR, Rattay F. Stimulation of the human lumbar spinal cord with implanted and surface electrodes: a computer simulation study. IEEE Trans Neural Syst Rehabil Eng. (2010) 18:637–45. doi: 10.1109/TNSRE.2010.2054112
97. Khaslavskaia S, Ladouceur M, Sinkjaer T. Increase in tibialis anterior motor cortex excitability following repetitive electrical stimulation of the common peroneal nerve. Exp Brain Res. (2002) 145:309–15. doi: 10.1007/s00221-002-1094-9
98. Knash ME, Kido A, Gorassini M, Chan KM, Stein RB. Electrical stimulation of the human common peroneal nerve elicits lasting facilitation of cortical motor-evoked potentials. Exp Brain Res. (2003) 153:366–77. doi: 10.1007/s00221-003-1628-9
99. Khaslavskaia S, Sinkjaer T. Motor cortex excitability following repetitive electrical stimulation of the common peroneal nerve depends on the voluntary drive. Exp Brain Res. (2005) 162:497–502. doi: 10.1007/s00221-004-2153-1
100. Ridding MC, Brouwer B, Miles TS, Pitcher JB, Thompson PD. Changes in muscle responses to stimulation of the motor cortex induced by peripheral nerve stimulation in human subjects. Exp Brain Res. (2000) 131:135–43. doi: 10.1007/s002219900269
101. Thompson AK, Lapallo B, Duffield M, Abel BM, Pomerantz F. Repetitive common peroneal nerve stimulation increases ankle dorsiflexor motor evoked potentials in incomplete spinal cord lesions. Exp Brain Res. (2011) 210:143–52. doi: 10.1007/s00221-011-2607-1
102. Kaelin-Lang A, Luft AR, Sawaki L, Burstein AH, Sohn YH, Cohen LG. Modulation of human corticomotor excitability by somatosensory input. J Physiol. (2002) 540:623–33. doi: 10.1113/jphysiol.2001.012801
103. Golaszewski SM, Bergmann J, Christova M, Kunz AB, Kronbichler M, Rafolt D, et al. Modulation of motor cortex excitability by different levels of whole-hand afferent electrical stimulation. Clin Neurophysiol. (2012) 123:193–9. doi: 10.1016/j.clinph.2011.06.010
104. Knikou M, Conway BA. Effects of electrically induced muscle contraction on flexion reflex in human spinal cord injury. Spinal Cord. (2005) 43:640–8. doi: 10.1038/sj.sc.3101772
105. Thompson AK, Doran B, Stein RB. Short-term effects of functional electrical stimulation on spinal excitatory and inhibitory reflexes in ankle extensor and flexor muscles. Exp Brain Res. (2006) 170:216–26. doi: 10.1007/s00221-005-0203-y
106. Yamaguchi T, Fujiwara T, Tsai YA, Tang SC, Kawakami M, Mizuno K, et al. The effects of anodal transcranial direct current stimulation and patterned electrical stimulation on spinal inhibitory interneurons and motor function in patients with spinal cord injury. Exp Brain Res. (2016) 234:1469–78. doi: 10.1007/s00221-016-4561-4
107. Hardy SG, Spalding TB, Liu H, Nick TG, Pearson RH, Hayes AV, et al. The effect of transcutaneous electrical stimulation on spinal motor neuron excitability in people without known neuromuscular diseases: the roles of stimulus intensity and location. Phys Ther. (2002) 82:354–63. doi: 10.1093/ptj/82.4.354
108. Mima T, Oga T, Rothwell J, Satow T, Yamamoto J, Toma K, et al. Short-term high-frequency transcutaneous electrical nerve stimulation decreases human motor cortex excitability. Neurosci Lett. (2004) 355:85–8. doi: 10.1016/j.neulet.2003.10.045
109. Aydin G, Tomruk S, Keleş I, Demir SO, Orkun S. Transcutaneous electrical nerve stimulation versus baclofen in spasticity: clinical and electrophysiologic comparison. Am J Phys Med Rehabil. (2005) 84:584–92. doi: 10.1097/01.phm.0000171173.86312.69
110. Joodaki MR, Olyaei GR, Bagheri H. The effects of electrical nerve stimulation of the lower extremity on H-reflex and F-wave parameters. Electromyogr Clin Neurophysiol. (2001) 41:23–8.
111. Serrano-Muñoz D, Gómez-Soriano J, Bravo-Esteban E, Ávila-Martín G, Galán-Arriero I, Taylor J, et al. Soleus H-reflex modulation following transcutaneous high- and low-frequency spinal stimulation in healthy volunteers. J Electromyogr Kinesiol. (2019) 46:1–7. doi: 10.1016/j.jelekin.2019.03.004
112. Bekhet AH, Bochkezanian V, Saab IM, Gorgey AS. The effects of electrical stimulation parameters in managing spasticity after spinal cord injury: a systematic review. Am J Phys Med Rehabil. (2019) 98:484–99. doi: 10.1097/PHM.0000000000001064
113. Mills PB, Dossa F. Transcutaneous electrical nerve stimulation for management of limb spasticity: a systematic review. Am J Phys Med Rehabil. (2016) 95:309–18. doi: 10.1097/PHM.0000000000000437
114. Murray LM, Knikou M. Remodeling brain activity by repetitive cervicothoracic transspinal stimulation after human spinal cord injury. Front Neurol. (2017) 8:50. doi: 10.3389/fneur.2017.00050
115. Knikou M, Murray LM. Repeated transspinal stimulation decreases soleus H-reflex excitability and restores spinal inhibition in human spinal cord injury. PLoS ONE. (2019) 14:e0223135. doi: 10.1371/journal.pone.0223135
116. Hofstoetter US, McKay WB, Tansey KE, Mayr W, Kern H, Minassian K. Modification of spasticity by transcutaneous spinal cord stimulation in individuals with incomplete spinal cord injury. J Spinal Cord Med. (2014) 37:202–11. doi: 10.1179/2045772313Y.0000000149
117. Hofstoetter US, Freundl B, Danner SM, Krenn MJ, Mayr W, Binder H, et al. Transcutaneous spinal cord stimulation induces temporary attenuation of spasticity in individuals with spinal cord injury. J Neurotrauma. (2019) 37:481–93. doi: 10.1089/neu.2019.6588
118. Barolat G, Singh-Sahni K, Staas WE Jr, Shatin D, Ketcik B, et al. Epidural spinal cord stimulation in the management of spasms in spinal cord injury: a prospective study. Stereotact Funct Neurosurg. (1995) 64:153–64. doi: 10.1159/000098744
119. Dimitrijevic MR, Illis LS, Nakajima K, Sharkey PC, Sherwood AM. Spinal cord stimulation for the control of spasticity in patients with chronic spinal cord injury: II. Neurophysiologic observations. Cent Nerv Syst Trauma. (1986) 3:145–52. doi: 10.1089/cns.1986.3.145
120. Dietz V, Fouad K. Restoration of sensorimotor functions after spinal cord injury. Brain. (2014) 137:654–67. doi: 10.1093/brain/awt262
121. Ditunno PL, Patrick M, Stineman M, Ditunno JF. Who wants to walk? Preferences for recovery after SCI: a longitudinal and cross-sectional study. Spinal Cord. (2008) 46:500–6. doi: 10.1038/sj.sc.3102172
122. Edgerton VR, Courtine G, Gerasimenko YP, Lavrov I, Ichiyama RM, Fong AJ, et al. Training locomotor networks. Brain Res Rev. (2008) 57:241–54. doi: 10.1016/j.brainresrev.2007.09.002
123. Darrow D, Balser D, Netoff TI, Krassioukov A, Phillips A, Parr A, et al. Epidural spinal cord stimulation facilitates immediate restoration of dormant motor and autonomic supraspinal pathways after chronic neurologically complete spinal cord injury. J Neurotrauma. (2019) 36:2325–36. doi: 10.1089/neu.2018.6006
124. Rushton DN. Functional electrical stimulation and rehabilitation–an hypothesis. Med Eng Phys. (2003) 25:75–8. doi: 10.1016/S1350-4533(02)00040-1
125. Prior MM, Stinear JW. Phasic spike-timing-dependent plasticity of human motor cortex during walking. Brain Res. (2006) 1110:150–8. doi: 10.1016/j.brainres.2006.06.057
126. Lotze M, Braun C, Birbaumer N, Anders S, Cohen LG. Motor learning elicited by voluntary drive. Brain. (2003) 126:866–72. doi: 10.1093/brain/awg079
127. Rossini PM, Caramia MD, Zarola F. Mechanisms of nervous propagation along central motor pathways: noninvasive evaluation in healthy subjects and in patients with neurological disease. Neurosurgery. (1987) 20:183–91. doi: 10.1097/00006123-198701000-00035
128. Devanne H, Lavoie BA, Capaday C. Input-output properties and gain changes in the human corticospinal pathway. Exp Brain Res. (1997) 114:329–38. doi: 10.1007/PL00005641
129. Ziemann U, Rothwell JC, Ridding MC. Interaction between intracortical inhibition and facilitation in human motor cortex. J Physiol. (1996) 496:873–81. doi: 10.1113/jphysiol.1996.sp021734
130. Popovic MB, Popovic DB, Sinkjaer T, Stefanovic A, Schwirtlich L. Clinical evaluation of functional electrical therapy in acute hemiplegic subjects. J Rehabil Res Dev. (2003) 40:443–53. doi: 10.1682/JRRD.2003.09.0443
131. Kimberley TJ, Lewis SM, Auerbach EJ, Dorsey LL, Lojovich JM, Carey JR. Electrical stimulation driving functional improvements and cortical changes in subjects with stroke. Exp Brain Res. (2004) 154:450–60. doi: 10.1007/s00221-003-1695-y
132. Ridding MC, Taylor JL, Rothwell JC. The effect of voluntary contraction on cortico-cortical inhibition in human motor cortex. J Physiol. (1995) 487:541–8. doi: 10.1113/jphysiol.1995.sp020898
133. Harkema SJ, Hillyer J, Schmidt-Read M, Ardolino E, Sisto SA, Behrman AL. Locomotor training: as a treatment of spinal cord injury and in the progression of neurologic rehabilitation. Arch Phys Med Rehabil. (2012) 93:1588–97. doi: 10.1016/j.apmr.2012.04.032
134. Devlin N, Parkin D. Does NICE have a cost-effectiveness threshold and what other factors influence its decisions? A binary choice analysis. Health Econ. (2004) 13:437–52. doi: 10.1002/hec.864
135. Lee BB, King MT, Simpson JM, Haran MJ, Stockler MR, Marial O, et al. Validity, responsiveness, and minimal important difference for the SF-6D health utility scale in a spinal cord injured population. Value Health. (2008) 11:680–8. doi: 10.1111/j.1524-4733.2007.00311.x
Keywords: FES, SCS, neuroplasticity, spinal cord injury, FES-cycling, locomotor training, neuroprosthesis, posterior nerve roots
Citation: Duffell LD and Donaldson NdN (2020) A Comparison of FES and SCS for Neuroplastic Recovery After SCI: Historical Perspectives and Future Directions. Front. Neurol. 11:607. doi: 10.3389/fneur.2020.00607
Received: 11 March 2020; Accepted: 25 May 2020;
Published: 30 June 2020.
Edited by:
Manuel F. Gaviria, Institut Equiphoria, FranceReviewed by:
Andrew C. Smith, Regis University, United StatesCopyright © 2020 Duffell and Donaldson. This is an open-access article distributed under the terms of the Creative Commons Attribution License (CC BY). The use, distribution or reproduction in other forums is permitted, provided the original author(s) and the copyright owner(s) are credited and that the original publication in this journal is cited, in accordance with accepted academic practice. No use, distribution or reproduction is permitted which does not comply with these terms.
*Correspondence: Lynsey D. Duffell, bC5kdWZmZWxsQHVjbC5hYy51aw==
†These authors have contributed equally to this work
Disclaimer: All claims expressed in this article are solely those of the authors and do not necessarily represent those of their affiliated organizations, or those of the publisher, the editors and the reviewers. Any product that may be evaluated in this article or claim that may be made by its manufacturer is not guaranteed or endorsed by the publisher.
Research integrity at Frontiers
Learn more about the work of our research integrity team to safeguard the quality of each article we publish.