- 1Department of Neurology, Dalian Municipal Central Hospital Affiliated to Dalian Medical University, Dalian, China
- 2Department of Pharmacy, Dalian Municipal Central Hospital Affiliated to Dalian Medical University, Dalian, China
- 3School of Life Sciences, University of Warwick, Coventry, United Kingdom
Ischemic stroke is a major cause of disability and mortality worldwide, but effective restorative treatments are very limited at present. Regenerative medicine research revealed that stem cells are promising therapeutic options. Dental pulp stem cells (DPSCs) are autologously applicable cells that origin from the neural crest and exhibit neuro-ectodermal features next to multilineage differentiation potentials. DPSCs are of increasing interest since they are relatively easy to obtain, exhibit a strong proliferation ability, and can be cryopreserved for a long time without losing their multi-directional differentiation capacity. Besides, use of DPSCs can avoid fundamental problems such as immune rejection, ethical controversy, and teratogenicity. Therefore, DPSCs provide a tempting prospect for stroke treatment.
The past decade has witnessed intense advancement and tremendous therapeutic achievements in the ability to diagnose and treat stroke, a cerebrovascular disease of which 87% is ischemic in nature. Nevertheless, stroke remains a major cause of disability, morbidity, and mortality worldwide, and constitutes a major socioeconomic problem (1, 2). Ischemic stroke, due to partially or completely blocked blood flow in a cerebral artery, causes ischemic necrosis of brain tissue seriously impairing the health of affected individuals. The main therapeutic strategy for ischemic stroke is timely recanalization. This can either be achieved by tissue-type plasminogen activator application or mechanical thrombectomy. Particularly the latter can be applied up to 24 h after stroke in patients exhibiting a penumbra, and has revolutionized acute stroke treatment. However, the absolute number of patients qualifying for recanalization remains very low (3, 4). Hence, additional treatment approaches being effective beyond the first hours after stroke onset are urgently required.
Stem cell transplantation is a promising strategy to restore neurological function after stroke (5). Experimental stem cell transplantation in animals showed that numerous cell populations can improve functional recovery by a broad spectrum of mechanisms (6–8). Several kinds of stem cells are currently considered for therapy. These include embryonic stem cells (ESCs), fetal stem/progenitor cells, induced pluripotent stem cells (iPSCs), and adult stem cells. While embryonic or induced pluripotent stem cells exhibit a tremendous differentiation potential, they may also inherit a risk for tumor formation (9). The use of embryonic stem cells or fetal stem/progenitor cells raises ethical concerns. Adult stem cells show a limited proliferation and differentiation potential, but can still be beneficial after stroke due numerous mechanisms beyond tissue restoration. They are further believed to be safer in clinical application and their use is ethically less challenging (9–13).
Recent systematic reviews and meta-analyses on the most prominent adult stem cell therapy candidates, mesenchymal stem cells (MSCs), presented evidence that MSCs improve the outcome after stroke in animals (14) and patients, and confirmed the safety and feasibility of the approach (15). Nevertheless, there is still a lack of adult (stem) cells that can be derived from an autologous source, and may exhibit therapeutic abilities beyond those of MSCs.
Dental Pulp Stem Cells (DPSCs): A New Source of Adult Stem Cells
The dental pulp is a soft tissue located in the center of teeth. It comprises blood vessels, neural fibers, and connective tissue. The dental pulp contains both mesenchymal and ectodermal tissue as well as neural crest cells (16). Limited dentinal repair in the postnatal organism relies on specialized precursor cell populations residing in the dental pulp tissue. Gronthos et al. first reported the isolation and characterization of stem cells from dental pulp tissue of the third molar in 2000 (17). DPSCs are ectoderm-derived stem cells, originating from migrating neural crest cells (Figure 1). They are a subpopulation among dental pulp cells (DPCs) which possess MSC properties, such as a fibroblast-like morphology, adherence to a plastic surface, as well as surface marker expression, proliferation and colony forming behavior similar to that of MSCs (18, 19). It is not clear whether or not DPSCs are a kind of MSC population. Given their differentiation abilities as reviewed below, it might be assumed that DPSCs are a more naïve stem cell population that also, but not exclusively, exhibits MSC properties. A major benefit of DPSCs is that they can be isolated during routine dental procedures such as the eruption of deciduous teeth or extraction of impacted wisdom teeth (20) in simple and autologous fashion without ethical concerns. Another primary advantage of DPSCs is their potential for cell banking. Several studies have demonstrated that DPSCs retain their stem cell properties after long cryopreservation (21, 22). This is essential as cryopreservation can impact therapeutic capacities of other adult stem cell-containing populations in stroke (23). In addition, DPSC cultures can be established from extracted human molars with high efficiency, even after the whole tooth has been cryopreserved for up to 1 month (24). DPSCs also exhibit a multilineage differentiation potential into chondrocytes, adipocytes, odontoblasts, and potentially even neural-like cells (25–28).
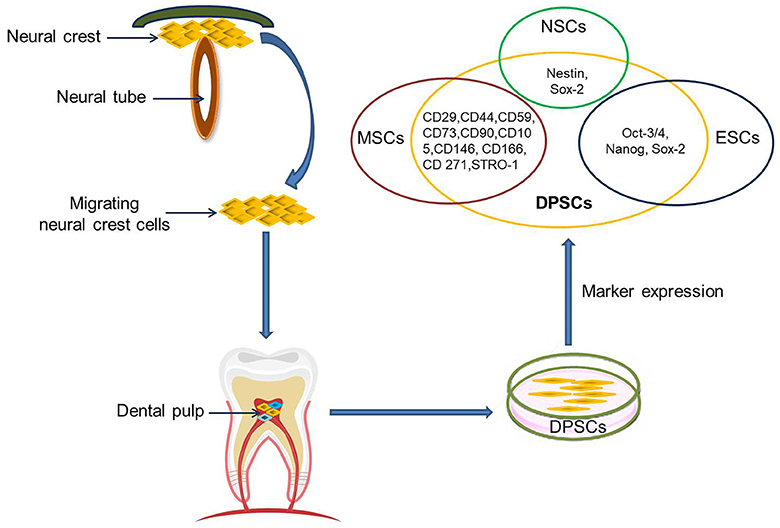
Figure 1. DPSCs origin, isolation, and marker expression. DPSCs originate from migrating neural crest cells, coming to rest in dental pulp, and express markers overlapping with MSCs, ESCs, and NCSs.
Currently, there are no specific markers that uniquely define DPSCs. In general, DPSCs, as a heterogeneous population, express a variety of markers similar to MSCs (Table 1) (Figure 1), and do not express hematopoietic markers such as CD14, CD19, CD34, and CD45 (18, 26, 29–32). DPSCs isolated by their high proliferative potential tend to include a large population of cells expressing CD44+, CD90+, and CD166+. However, DPSCs also express stemness-related markers similar to ESCs such as Oct-3/4, Nanog, and Sox-2, as well as the cytoskeleton-related markers nestin and vimentin (Figure 1) (33–35). They further express insulin-like growth factor 1 receptor (IGF1R) which is regarded as a pluripotency marker in ESCs. DPSC-secreted IGF1 interacts with IGF1R through an autocrine signaling pathway to maintain self-renewal and proliferation potential (36).
In addition, DPSCs (as neural crest-derived stem cells) not only express a number of neural stem cell (NSC) associated markers including nestin (26, 37) and Sox2 (38) (Figure 1), but also express low basal levels of markers associated with mature central nervous system cell types, including the neuronal markers βIII-tubulin, microtubule-associated protein 2 (MAP2), neurofilaments (NF) (33, 39), NeuN (40), the astrocytic marker glial fibrillary acidic protein (GFAP) (26, 33), and oligodendrocyte-associated CNPase (33). Taken together, this suggests that DPSCs can indeed differentiate into neuron-like cells under appropriate conditions, and differentiated cells even exhibit typical electrophysiological properties after neuronal differentiation (41, 42).
DPSCs as a Potential Candidate for Therapy of Neurological Diseases
Brain-derived NSCs are considered a promising population for stroke treatment due to their ability to self-renew and to differentiate into neural cells types (neurons, astrocytes, oligodendrocytes) (43). However, autologous harvest of adult human NSCs requires neurosurgical procedures due to their brain parenchymal residence (44), while allogeneic or even xenogenic NSCs grafting imposes the risk of graft rejection and additional immunological damage. Only a limited number of clinical trials currently explore the potential of NSCs for stroke treatment because of these limitations.
Adult stem cells or stem cell-containing populations are more frequently applied in translational research. As stated above, DPSCs share many biological characteristics with MSCs including bone marrow MSCs (BM-MSCs), adipose tissue-derived stem cells (ADSCs) and umbilical cord MSCs (UC-MSCs) but there are some variations in their proliferation potential (17, 27, 45), differentiation potential (17, 27, 46), immunomodulatory activity (27), secretome characteristics, and secretory capacity (47–49). Specifically, DPSCs have a higher proliferation rate and a greater clonogenic potential than MSCs (17, 45). Next to DPSCs, the DPC population also contains a higher number of stem/progenitor cells as compared to bone marrow (50). This may be attributed to the developmental state of the respective tissues. All teeth, even the permanent molars, are generated early in individual development and rest in the jar until they erupt. Abilities and capacities of stem cells may be much better preserved in tissue with a slow turnover such as the dental pulp when compared to BM, which exhibits a tremendous turn-over throughout life apart from some niches. DPSCs maintain their high rate of proliferation even after extensive subculturing.
Like MSCs, DPSCs can differentiate into cells of mesenchymal and non-mesenchymal tissues in vitro and in vivo. However, DPSCs exhibit stronger odontogenesis and neurogenesis capabilities, in turn being not as potent to produce adipogeneic, osteogeneic and chondrogeneic tissue than BM-MSCs (51) and ADSCs (46). Besides, DPSCs also have immunomodulatory capacities exceeding those of BM-MSCs, for example a higher suppression rate of T lymphocyte growth (17, 27).
DPSCs exhibit superior neuroprotective and neuro-supportive properties in neurological injuries and pathologies as compared with BM-MSCs and ADSCs (52). This might be related to a higher expression of trophic factors including brain derived neurotrophic factor (BDNF), glial cell-derived neurotrophic factor (GDNF), nerve growth factor (NGF), vascular endothelial growth factor (VEGF), and platelet derived growth factor (PDGF) in DPSCs as compared to BM-MSCs (47, 48), although the spectrum of growth and trophic factors secretion is similar (53). DPSCs also express higher quantities of CXCL14 and monocyte chemoattractant protein 1 (MCP-1) than ADSCs (49). Besides, the DPSC secretome contains higher concentrations of RANTES, FRACTALKINE, fms-related tyrosine kinase 3 (FLT-3), granulocyte-macrophage colony-stimulating factor (GM-CSF), and MCP-1 than the BM-MSCs secretome (54). DPSCs show higher angiogenic and neurogenic potentials in ectopic transplantation models compared to BM-MSCs and ADSCs, and exhibit the highest migration capacity. Transplantation of DPSCs in a mouse hindlimb ischemia model produced higher blood flow and capillary density than transplantation of BM-MSCs and ADSCs, which being associated with superior recovery of limb movement abilities and reduction of ischemic hindlimb damage (55). DPSCs also mediate stronger anti-apoptotic effects in a microenvironment challenged by oxidative and serum deprivation than BM-MSCs, ADSCs and UC-MSCs (45).
Cell size and diameter are important for safety after intravascular delivery as they are the major, but not the only, determinants of vascular obstruction and complications (56) (Table 2). Previously reported studies showed that the cell diameter of human DPSCs is around 15–16 μm (59), which is comparable to NSCs (57, 58) but slightly smaller than for most MSC populations (57, 60) including human BM-MSCs (61). Still this means that one has to expect a considerable pulmonary passage filtering effect after intravenous delivery, as well as a risk for microembolism after intraarterial administration (62). Hence, thorough investigations identifying the optimal route of DPSC administration by considering safety and efficacy aspects are recommended in DPSC translational research.
DPSCs for Ischemic Neuronal Damage: in vitro Effects
Treatment with immunosorted IGF1R+ DPSCs significantly modulates neurite regeneration and anti-inflammation in primary cortical cultures subject to oxygen/glucose deprivation (OGD) (36). DPSCs cultivated on adult mouse hippocampal slices were able to stimulate neurogenesis in both the CA1 zone and at the edges of the hippocampal slices through neurotrophic support in vitro (41). Besides, DPSCs can protect primary hippocampal, mesencephalic (63) and dopaminergic neurons (64) from β-amyloid peptide and 6-OHDA induced toxicity, respectively. Furthermore, DPSCs and conditioned medium from DPSCs show superior protective, migratory, and angiogenic effects in OGD-injured astrocytes as compared to BM-MSCs (52, 65). Reducing reactive gliosis, reactive oxygen species production and inflammatory mediators might contribute to this protective effect (52).
DPSCs Effects After Ischemic Stroke in vivo
Human DPSCs can differentiate toward functionally active neurons under appropriate culture conditions (66–68). This comes on top of their bystander effects, indicating that DPSCs might provide enhanced therapeutic capacities in neurological diseases including stroke, Parkinson's disease, Alzheimer's disease, and spinal cord injury (52, 63, 69). To date, there are several preclinical studies demonstrating that DPSCs exert neuroprotective effect resulting in improved functional outcome and reduced infract volumes in rodent stroke models (Table 3) (52, 66, 68, 70–76). No obvious deleterious effects were observed in these studies (66, 68, 71–73, 75), but have not been always explicitly looked for.
A number of remarkable improvements were seen in behavioral tests (Table 3), underpinning the considerable effect DPSCs may exert after ischemic stroke. However, many of the behavioral tests used are known for a tendency to overestimate true functional recovery in standard rodent models so future research may also include the use of highly specific behavioral readout systems (77).
During ischemia, neurons are unable to maintain normal transmembrane ion gradient and balance, resulting in cell death by apoptosis, excitatory toxicity, and oxidative stress. Inflammatory reactions contribute to cell death in subacute and even chronic stages what can be exacerbated in the presence of important stroke risk factors (78, 79). These pathophysiological processes are interrelated and can trigger each other, forming a vicious cycle (80, 81). Indeed, neuroinflammation and immune response after stroke have been recognized as key factors contributing to overall brain damage and the extent of neurological deficit (82). The administration of DPSCs during the acute phase of stroke dampens inflammation in vivo, and can promote recovery from in post-ischemia/reperfusion brain injury (70). Moreover, intracerebral transplantation of DPSCs or immunosorted IGF1R+ DPSCs into the ischemically injured neonatal murine brain significantly increases immunomodulation, enhances poststroke recovery, and promotes neuroplasticity (36, 67). Further, intravenous transplantation of DPSCs or DPSC-derived neurosphere cells significantly ameliorates the impact of global cerebral ischemia, decreases neuronal cell death in the hippocampal CA1 region, improves neuromotor and cognitive function as well as overall survival rates in stroke animals (73). Moreover, intracerebral transplantation of DPSCs also enhanced poststroke functional recovery after brain injury through increasing expression of the anti-apoptotic protein Bcl-2 (67).
Transplanted DPSCs can migrate into the boundary of ischemic areas, and express neural cell and NSC markers such as βIII tubulin, doublecortin (DCX), nestin, and NF (72). The cells' beneficial effects may even be exerted after xenogeneic transplantation as evidenced by a study showing that porcine DPSCs (CD31−/CD146− side population cells) injection promotes recovery form motor impairment and reduced infarct volume, promoted migration and differentiation of endogenous NSCs, and finally induced vasculogenesis after stroke in rats (66).
DPSC Exosomes—Great Opportunities for Cell Therapy Without Cells
The limited survival, differentiation and integration of DPSC-derived cells into the ischemically lesioned brain implies that the functional improvement is more likely mediated through bystander effects rather than cell replacement and differentiation (33). It has been well-documented that the MSC secretome contains a variety of cytokines, chemokines, and growth factors, along with extracellular vesicles (EVs). The most important EVs in MSC-conditioned medium are exosomes, which are of “nano” size (30–100 nm in diameter) (83, 84). EVs play an important role in intercellular communication because they can transfer RNA, micro-RNA, proteins, membrane receptors and even organelles (mitochondria) between cells (85, 86). MSC-derived EVs are of increasing interest since they may have a comparable therapeutic potential to MSCs themselves, but are relatively safer in application, and can pass through the BBB far more easily than cells (87). Studies demonstrated that administration of BM-MSC or ESC-derived exosomes could significantly increases neurogenesis and vasculogenesis, and promotes functional recovery in stroke animal (88, 89).
Likewise, accumulating evidence demonstrated the potent neuroprotective properties of DPSC-derived EVs. An in vitro study showed that DPSC-EVs which were grown on laminin-coated microcarriers display neuroprotective properties in 6-OHDA-exposed human dopaminergic neurons (90). DPSC-EVs also reduce cytotoxicity through anti-apoptotic mechanism by upregulating endogenous Bcl-2, and decrease the expression of the pro-apoptotic regulator Bax in Aβ peptide-exposed human neuroblastoma (SH-SY5Y) cells (54). An in vivo study showed that exosomes derived from DPSCs have beneficial effects after focal cerebral ischemia in the rat by stimulating angiogenesis and neurogenesis (91). In addition, the therapeutic potential of DPSC-derived conditioned medium (CM) was found to be similar to that of the injection of living cells in animal model of stroke, leading to motor function improvement and infarct volume reduction (76). Moreover, CM from human DPSCs also induced significant neuroprotection, enhanced neuronal sprouting, and reduced neuroinflammation in a mouse model of Alzheimer disease (92). DPSC-derived exosomes were further shown to exert strong anti-inflammatory effects at levels comparable to those of glucocorticoids. They also suppress cathepsin B and matrix metalloproteinase (MMP) activities at the site of inflammation in mice, likely mediated by the transport of annexin A1, phospholipases, and lipid mediators to the site of inflammation (93). Taken as a whole, these studies showed the potential of DPSC-derived exosomes for the treatment of central nervous system disorders.
Investigation of molecules within EVs provides new insight to EV-mediated beneficial mechanism, although determining the exact composition and content of the exosomal content (cargo) produced by different cell types is hard to establish due to inevitable differences regarding the conditions in which the cells are prepared and processed (83). High-throughput mass spectrometry-based analysis of proteins revealed some surface receptors (CD105, CD73, CD29, CD81, and CD44), signaling molecules (many of which are involved in controlling of TGF-β, BMP, MAPK, and PPAR recipient cell signaling pathways), adhesion molecules and MSC-associated markers which may account for the therapeutic potential of MSC-derived EVs (94, 95). Baglio et al. (96) reported a substantial similarity between the most represented miRNAs in ADSC and BM-MSC exosomes, but their relative proportions are different. The top 5 most abundant miRNAs (accounted for 50 % of the total miRNA reads) in ADSC exosomes were miR-486-5p, miR-10a-5p, miR-10b-5p, miR-191-5p, and miR-222-3p, while miR-143-3p, miR-10b-5p, miR-486-5p, miR-22-3p, and miR-21-5p were among the most abundant for BM-MSC exosomes. Besides, exosome libraries were highly enriched in the class of tRNAs, which represented >50 % of total small RNAs in ADSC exosomes and 23–35% in BM-MSC exosomes. However, since the studies of DPSC exosomes are just at the initial stage, there is no exactly content of these exosomes reported.
Possible Mechanisms of DPSC Therapy for Ischemic Stroke
Previous studies suggested that human DPSCs potentially differentiate into functional neural progenitors or neurons which may integrate into the brain (64, 97, 98). Similarly, studies showed that grafted DPSCs survive, migrated to infarct boundary zones, and differentiate into neurons and astrocytes in the rat. The cells also express neuron-specific markers including βIII tubulin and NF (52, 72). However, only a very small part of transplanted DPSCs (2.3 ± 0.7%) survived in the post-stroke brain, migrated to the peri-infarction areas, and differentiated into astrocytes (51.0 ± 8.6% GFAP+) in preference to neurons (8.7 ± 6.1% NeuN+) (68). Hence, the therapeutic potential of DPSCs is believed to be mainly exerted by their bystander effects (Figure 2) (76).
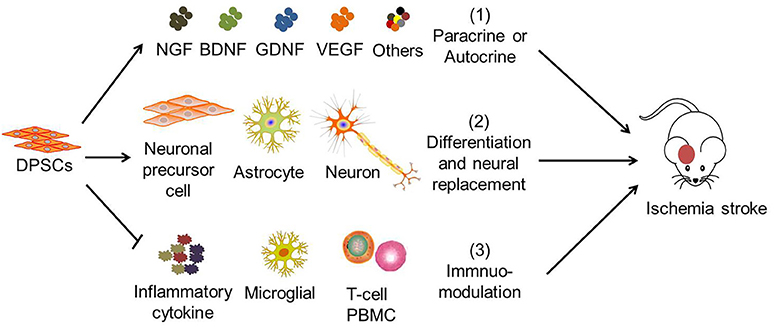
Figure 2. The mechanisms of DPSC therapy for ischemic stroke. The therapeutic effects of DPSCs in stroke are attributed to (1) paracrine or autocrine production of cytokines and growth factors, (2) neural replacement through differentiation into neuronal progenitor cells, astrocytes and neuron-like cells, (3) immuno-modulation with mitigation of pro-inflammatory cytokine expression, of microglial activation, inhibition of activated T-cell response, and peripheral blood mononuclear cell (PBMC) proliferation. (1) and (3) are believed to be the main therapeutic effects of DPSCs.
DPSCs have the potential to improve the microenvironment and enhancing neurogenesis (47, 64, 99, 100). DPSCs also have been shown to exert potent immune-modulatory properties via inhibition of activated T cell responses (27) and peripheral blood mononuclear cell proliferation (101), regulating the expression of inflammatory factors such as TGF-β and interleukin (IL)-6 (102), and the induction of Fas ligand-mediated T cell apoptosis (103). Although the suppression of T cell proliferation seen in in vitro studies is now established, this property of DPSCs may not be the sole mechanism of action in vivo, particularly since blood circulation increases the number of T cells perpetually. More studies are therefore warranted to further understand the interaction between DPSCs and the immune system because this cross talk has important therapeutic implications.
Limitations of DPSCs and Challenges Related to Their Use
Though DPSCs have a higher proliferation rate than MSCs, it still needs at least 1 to 2 months to acquire enough cells for therapy from primary isolation (104) (Table 4), which may limit their use at the acute stage of acute onset diseases. The long-term side effects associated with the use of DPSCs also have not been sufficiently studied so far. Further studies are warranted to clarify possible long-term risks associated with the use of these cells, as well as optimal cell preparation, storage and application procedures including routes and time points of application. Tailored potency assays for clinical trials are also lacking, and the optimal route of delivery awaits detailed investigation. Moreover, clinical investigation of the cells has just started (105) so appropriate double-blinded, randomized clinical trials have not yet been reported, currently preventing any conclusion on a potential clinical efficacy of these cells. In addition, further studies thoroughly assessing efficacy, safety and also the content of DPSC-derived EVs are required since EVs are highly promising therapeutic tools for regenerative medicine, but a thorough proof of concept is still missing. Additional research is also needed to capitalize on the DPSC differentiation potential. This might require specialized stroke models, for instance mimicking lacunar stroke, that may be more permissible for tissue restoration, and/or the use of biomaterials to support cell engraftment and survival (106).
Conclusions
This review summarizes the main DPSC characteristics including surface marker expression, proliferation and differentiation potential, cytokine and trophic factor secretion ability, as well as therapeutic effects in in vitro and in vivo stroke models. It also elucidates important underlying therapeutic mechanisms. DPSCs express a variety of markers that are found on MSCs, ESCs, and NSCs. Although they can differentiate into different types of neuronal cells, bystander effects are believed to be their predominant therapeutic mechanism. DPSCs are widely available, easily accessible, and can support well-established stroke therapies, thereby potentially extending the therapeutic time window and/or augmenting the therapeutic impact. DPSCs differ from the other adult stem cell populations due to their embryonic origin from the neural crest and are of special interest because of their neurotropic character, which makes DPSCs and their exosomes particularly attractive as a new therapeutic tool for the alleviation of symptoms of stroke and, potentially, other neurodegenerative diseases. Besides, DPSCs exhibit a higher proliferation rate, higher expression of trophic factors, stronger neuroprotective effects and neuro-supportive properties in vitro and in vivo than MSC populations (Table 4), which provide a tempting prospect for stroke treatment.
Author Contributions
XL, ZS, and CC wrote the manuscript. JB and SL designed the literature assessment strategy and edited the manuscript.
Funding
The work was fund by the National Natural Science Foundation of China (81300985), Natural Science Foundation of Liaoning Province (2015020549), LiaoNing Revitalization Talents Program (XLYC1807124) and Dalian Municipal Health and Family Planning Project (1711014, 1811014).
Conflict of Interest Statement
The authors declare that the research was conducted in the absence of any commercial or financial relationships that could be construed as a potential conflict of interest.
References
1. Rothwell PM. The high cost of not funding stroke research: a comparison with heart disease and cancer. Lancet. (2001) 357:1612–6. doi: 10.1016/s0140-6736(00)04730-9
2. Zhang Y, Zhang P, Shen X, Tian S, Wu Y, Zhu Y, et al. Early exercise protects the blood-brain barrier from ischemic brain injury via the regulation of MMP-9 and occludin in rats. Int J Mol Sci. (2013) 14:11096–112. doi: 10.3390/ijms140611096
3. Brandt T, von Kummer R, Muller-Kuppers M, Hacke W. Thrombolytic therapy of acute basilar artery occlusion. Variables affecting recanalization and outcome. Stroke. (1996) 27:875–81.
4. Goyal M, Demchuk AM, Menon BK, Eesa M, Rempel JL, Thornton J, et al. Randomized assessment of rapid endovascular treatment of ischemic stroke. N Engl J Med. (2015) 372:1019–30. doi: 10.56/NEJMoa1414905
5. Janowski M, Wagner DC, Boltze J. Stem cell-based tissue replacement after stroke: factual necessity or notorious fiction? Stroke. (2015) 46:2354–63. doi: 10.1161/STROKEAHA.114.007803
6. Scheibe F, Ladhoff J, Huck J, Grohmann M, Blazej K, Oersal A, et al. Immune effects of mesenchymal stromal cells in experimental stroke. J Cereb Blood Flow Metab. (2012) 32:1578–88. doi: 10.038/jcbfm.2012.55
7. Trounson A, McDonald C. Stem cell therapies in clinical trials: progress and challenges. Cell Stem Cell. (2015) 17:11–22. doi: 10.1016/j.stem.2015.06.007
8. Boese AC, Le QE, Pham D, Hamblin MH, Lee JP. Neural stem cell therapy for subacute and chronic ischemic stroke. Stem Cell Res Ther. (2018) 9:154. doi: 10.1186/s13287-018-0913-2
9. Leong WK, Lewis MD, Koblar SA. Concise review: preclinical studies on human cell-based therapy in rodent ischemic stroke models: where are we now after a decade? Stem Cells. (2013) 31:1040–3. doi: 10.02/stem.348
10. Prockop DJ, Brenner M, Fibbe WE, Horwitz E, Le Blanc K, Phinney DG, et al. Defining the risks of mesenchymal stromal cell therapy. Cytotherapy. (2010) 12:576–8. doi: 10.3109/14653249.2010.507330
11. Lees JS, Sena ES, Egan KJ, Antonic A, Koblar SA, Howells DW, et al. Stem cell-based therapy for experimental stroke: a systematic review and meta-analysis. Int J Stroke. (2012) 7:582–8. doi: 10.1111/j.747-4949.2012.00797.x
12. Savitz SI. Developing cellular therapies for stroke. Stroke. (2015) 46:2026–31. doi: 10.1161/STROKEAHA.115.007149
13. Kalladka D, Muir KW. Brain repair: cell therapy in stroke. Stem Cells Cloning. (2014) 7:31–44. doi: 10.2147/SCCAA.S38003
14. Sarmah D, Agrawal V, Rane P, Bhute S, Watanabe M, Kalia K, et al. Mesenchymal stem cell therapy in ischemic stroke: a meta-analysis of preclinical studies. Clin Pharmacol Ther. (2018) 103:990–8. doi: 10.1002/cpt.927
15. Nagpal A, Choy FC, Howell S, Hillier S, Chan F, Hamilton-Bruce MA, et al. Safety and effectiveness of stem cell therapies in early-phase clinical trials in stroke: a systematic review and meta-analysis. Stem Cell Res Ther. (2017) 8:191. doi: 10.1186/s13287-017-0643-x
16. Kerkis I, Kerkis A, Dozortsev D, Stukart-Parsons GC, Gomes Massironi SM, Pereira LV, et al. Isolation and characterization of a population of immature dental pulp stem cells expressing OCT-4 and other embryonic stem cell markers. Cells Tissues Organs. (2006) 184:105–16. doi: 10.1159/000099617
17. Gronthos S, Mankani M, Brahim J, Robey PG, Shi S. Postnatal human dental pulp stem cells (DPSCs) in vitro and in vivo. Proc Natl Acad Sci USA. (2000) 97:13625–30. doi: 10.1073/pnas.240309797
18. Martens W, Bronckaers A, Politis C, Jacobs R, Lambrichts I. Dental stem cells and their promising role in neural regeneration: an update. Clin Oral Investig. (2013) 17:1969–83. doi: 10.007/s00784-013-1030-3
19. Dominici M, Le Blanc K, Mueller I, Slaper-Cortenbach I, Marini F, Krause D, et al. Minimal criteria for defining multipotent mesenchymal stromal cells. The international society for cellular therapy position statement. Cytotherapy. (2006) 8:315–7. doi: 10.1080/14653240600855905
20. Egusa H, Sonoyama W, Nishimura M, Atsuta I, Akiyama K. Stem cells in dentistry–part I: stem cell sources. J Prosthodont Res. (2012) 56:151–65. doi: 10.1016/j.jpor.2012.06.001
21. Zhang W, Walboomers XF, Shi S, Fan M, Jansen JA. Multilineage differentiation potential of stem cells derived from human dental pulp after cryopreservation. Tissue Eng. (2006) 12:2813–23. doi: 10.1089/ten.2006.12.813
22. Papaccio G, Graziano A, d'Aquino R, Graziano MF, Pirozzi G, Menditti D, et al. Long-term cryopreservation of dental pulp stem cells (SBP-DPSCs) and their differentiated osteoblasts: a cell source for tissue repair. J Cell Physiol. (2006) 208:319–25. doi: 10.1002/jcp.20667
23. Weise G, Lorenz M, Posel C, Maria Riegelsberger U, Storbeck V, Kamprad M, et al. Transplantation of cryopreserved human umbilical cord blood mononuclear cells does not induce sustained recovery after experimental stroke in spontaneously hypertensive rats. J Cereb Blood Flow Metab. (2014) 34:e1–9. doi: 10.1038/jcbfm.2013.185
24. Perry BC, Zhou D, Wu X, Yang FC, Byers MA, Chu TM, et al. Collection, cryopreservation, and characterization of human dental pulp-derived mesenchymal stem cells for banking and clinical use. Tissue Eng Part C Methods. (2008) 14:149–56. doi: 10.1089/ten.tec.2008.0031
25. Fawzy El-Sayed KM, Dorfer C, Fandrich F, Gieseler F, Moustafa MH, Ungefroren H. Adult mesenchymal stem cells explored in the dental field. Adv Biochem Eng Biotechnol. (2013) 130:89–103. doi: 10.1007/10_2012_151
26. Gronthos S, Brahim J, Li W, Fisher LW, Cherman N, Boyde A, et al. Stem cell properties of human dental pulp stem cells. J Dent Res. (2002) 81:531–5. doi: 10.1177/154405910208100806
27. Pierdomenico L, Bonsi L, Calvitti M, Rondelli D, Arpinati M, Chirumbolo G, et al. Multipotent mesenchymal stem cells with immunosuppressive activity can be easily isolated from dental pulp. Transplantation. (2005) 80:836–42. doi: 10.1097/01.tp.0000173794.72151.88
28. Balic A, Aguila HL, Caimano MJ, Francone VP, Mina M. Characterization of stem and progenitor cells in the dental pulp of erupted and unerupted murine molars. Bone. (2010) 46:1639–51. doi: 10.016/j.bone.2010.02.019
29. Kawashima N. Characterisation of dental pulp stem cells: a new horizon for tissue regeneration? Arch Oral Biol. (2012) 57:1439–58. doi: 10.016/j.archoralbio.2012.08.010
30. Palmieri A, Pezzetti F, Graziano A, Riccardo D, Zollino I, Brunelli G, et al. Comparison between osteoblasts derived from human dental pulp stem cells and osteosarcoma cell lines. Cell Biol Int. (2008) 32:733–8. doi: 10.1016/j.cellbi.2008.02.003
31. Foudah D, Redondo J, Caldara C, Carini F, Tredici G, Miloso M. Human mesenchymal stem cells express neuronal markers after osteogenic and adipogenic differentiation. Cell Mol Biol Lett. (2013) 18:163–86. doi: 10.2478/s11658-013-0083-2
32. Blondheim NR, Levy YS, Ben-Zur T, Burshtein A, Cherlow T, Kan I, et al. Human mesenchymal stem cells express neural genes, suggesting a neural predisposition. Stem Cells Dev. (2006) 15:141–64. doi: 10.1089/scd.2006.15.141
33. Sakai K, Yamamoto A, Matsubara K, Nakamura S, Naruse M, Yamagata M, et al. Human dental pulp-derived stem cells promote locomotor recovery after complete transection of the rat spinal cord by multiple neuro-regenerative mechanisms. J Clin Invest. (2012) 122:80–90. doi: 10.1172/JCI59251
34. Cheng PH, Snyder B, Fillos D, Ibegbu CC, Huang AH, Chan AW. Postnatal stem/progenitor cells derived from the dental pulp of adult chimpanzee. BMC Cell Biol. (2008) 9:20. doi: 10.1186/471-2121-9-20
35. Kiraly M, Porcsalmy B, Pataki A, Kadar K, Jelitai M, Molnar B, et al. Simultaneous PKC and cAMP activation induces differentiation of human dental pulp stem cells into functionally active neurons. Neurochem Int. (2009) 55:323–32. doi: 10.1016/j.neuint.2009.03.017
36. Chiu HY, Lin CH, Hsu CY, Yu J, Hsieh CH, Shyu WC. IGF1R(+) dental pulp stem cells enhanced neuroplasticity in hypoxia-ischemia model. Mol Neurobiol. (2017) 54:8225–41. doi: 10.1007/s12035-016-0210-y
37. Patel M, Smith AJ, Sloan AJ, Smith G, Cooper PR. Phenotype and behaviour of dental pulp cells during expansion culture. Arch Oral Biol. (2009) 54:898–908. doi: 10.1016/j.archoralbio.2009.06.008
38. Karbanova J, Soukup T, Suchanek J, Pytlik R, Corbeil D, Mokry J. Characterization of dental pulp stem cells from impacted third molars cultured in low serum-containing medium. Cells Tissues Organs. (2011) 193:344–65. doi: 10.1159/000321160
39. Osathanon T, Nowwarote N, Pavasant P. Basic fibroblast growth factor inhibits mineralization but induces neuronal differentiation by human dental pulp stem cells through a FGFR and PLCgamma signaling pathway. J Cell Biochem. (2011) 112:1807–16. doi: 10.002/jcb.23097
40. Foudah D, Monfrini M, Donzelli E, Niada S, Brini AT, Orciani M, et al. Expression of neural markers by undifferentiated mesenchymal-like stem cells from different sources. J Immunol Res. (2014) 2014:987678. doi: 10.1155/2014/987678
41. Xiao L, Ide R, Saiki C, Kumazawa Y, Okamura H. Human dental pulp cells differentiate toward neuronal cells and promote neuroregeneration in adult organotypic hippocampal slices in vitro. Int J Mol Sci. (2017) 18:E1745. doi: 10.3390/ijms18081745
42. Li D, Zou XY, El-Ayachi I, Romero LO, Yu Z, Iglesias-Linares A, et al. Human dental pulp stem cells and gingival mesenchymal stem cells display action potential capacity in vitro after neuronogenic differentiation. Stem Cell Rev. (2018) 15:67–81. doi: 10.1007/s12015-018-9854-5
43. Ross JJ, Verfaillie CM. Evaluation of neural plasticity in adult stem cells. Philos Trans R Soc Lond B Biol Sci. (2008) 363:199–205. doi: 10.1098/rstb.2006.21
44. Palmer TD, Schwartz PH, Taupin P, Kaspar B, Stein SA, Gage FH. Cell culture. Progenitor cells from human brain after death. Nature. (2001) 411:42–3. doi: 10.1038/35075141
45. Zhang Y, Xing Y, Jia L, Ji Y, Zhao B, Wen Y, et al. An in vitro comparative study of multisource derived human mesenchymal stem cells for bone tissue engineering. Stem Cells Dev. (2018) 27:1634–45. doi: 10.089/scd.2018.0119
46. Jin Q, Yuan K, Lin W, Niu C, Ma R, Huang Z. Comparative characterization of mesenchymal stem cells from human dental pulp and adipose tissue for bone regeneration potential. Artif Cells Nanomed Biotechnol. (2019) 47:1577–84. doi: 10.080/21691401.2019.1594861
47. Mead B, Logan A, Berry M, Leadbeater W, Scheven BA. Paracrine-mediated neuroprotection and neuritogenesis of axotomised retinal ganglion cells by human dental pulp stem cells: comparison with human bone marrow and adipose-derived mesenchymal stem cells. PLoS ONE. (2014) 9:e109305. doi: 10.1371/journal.pone.0109305
48. Caseiro AR, Pereira T, Ivanova G, Luis AL, Mauricio AC. Neuromuscular regeneration: perspective on the application of mesenchymal stem cells and their secretion products. Stem Cells Int. (2016) 2016:9756973. doi: 10.1155/2016/9756973
49. Hayashi Y, Murakami M, Kawamura R, Ishizaka R, Fukuta O, Nakashima M. CXCL14 and MCP1 are potent trophic factors associated with cell migration and angiogenesis leading to higher regenerative potential of dental pulp side population cells. Stem Cell Res Ther. (2015) 6:111. doi: 10.1186/s13287-015-0088-z
50. Alge DL, Zhou D, Adams LL, Wyss BK, Shadday MD, Woods EJ, et al. Donor-matched comparison of dental pulp stem cells and bone marrow-derived mesenchymal stem cells in a rat model. J Tissue Eng Regen Med. (2010) 4:73–81. doi: 10.1002/term.220
51. Mayo V, Sawatari Y, Huang CY, Garcia-Godoy F. Neural crest-derived dental stem cells–where we are and where we are going. J Dent. (2014) 42:1043–51. doi: 10.16/j.jdent.2014.04.007
52. Song M, Lee JH, Bae J, Bu Y, Kim EC. Human dental pulp stem cells are more effective than human bone marrow-derived mesenchymal stem cells in cerebral ischemic injury. Cell Trans. (2017) 26:1001–16. doi: 10.3727/096368916X694391
53. Ye X, Hu J, Cui G. therapy effects of bone marrow stromal cells on ischemic stroke. Oxid Med Cell Longev. (2016) 2016:7682960. doi: 10.1155/2016/7682960
54. Ahmed Nel M, Murakami M, Hirose Y, Nakashima M. Therapeutic potential of dental pulp stem cell secretome for Alzheimer's disease treatment: an in vitro study. Stem Cells Int. (2016) 2016:8102478. doi: 10.1155/2016/8102478
55. Ishizaka R, Hayashi Y, Iohara K, Sugiyama M, Murakami M, Yamamoto T, et al. Stimulation of angiogenesis, neurogenesis and regeneration by side population cells from dental pulp. Biomaterials. (2013) 34:1888–97. doi: 10.016/j.biomaterials.2012.10.045
56. Ge J, Guo L, Wang S, Zhang Y, Cai T, Zhao RC, et al. The size of mesenchymal stem cells is a significant cause of vascular obstructions and stroke. Stem Cell Rev. (2014) 10:295–303. doi: 10.1007/s12015-013-9492-x
57. Boltze J, Arnold A, Walczak P, Jolkkonen J, Cui L, Wagner DC. The dark side of the force–constraints and complications of cell therapies for stroke. Front Neurol. (2015) 6:155. doi: 10.3389/fneur.2015.00155
58. Li T, Li C, Zhang CY, Zhao J. Effect of accutase or trypsin dissociation on the apoptosis of human striatum-derived neural stem cells. Zhongguo Yi Xue Ke Xue Yuan Xue Bao. (2015) 37:185–94. doi: 10.3881/j.issn.1000-503X.2015.02.009
59. Suchanek J, Soukup T, Visek B, Ivancakova R, Kucerova L, Mokry J. Dental pulp stem cells and their characterization. Biomed Pap Med Fac Univ Palacky Olomouc Czech Repub. (2009) 153:31–5. doi: 10.5507/bp.2009.005
60. Fischer UM, Harting MT, Jimenez F, Monzon-Posadas WO, Xue H, Savitz SI, et al. Pulmonary passage is a major obstacle for intravenous stem cell delivery: the pulmonary first-pass effect. Stem Cells Dev. (2009) 18:683–92. doi: 10.1089/scd.2008.0253
61. Nishimura M, Nguyen L, Watanabe N, Fujita Y, Sawamoto O, Matsumoto S. Development and characterization of novel clinical grade neonatal porcine bone marrow-derived mesenchymal stem cells. Xenotransplantation. (2019) 15:12501. doi: 10.1111/xen.12501
62. Cui LL, Kerkela E, Bakreen A, Nitzsche F, Andrzejewska A, Nowakowski A, et al. The cerebral embolism evoked by intra-arterial delivery of allogeneic bone marrow mesenchymal stem cells in rats is related to cell dose and infusion velocity. Stem Cell Res Ther. (2015) 6:11. doi: 10.1186/scrt544
63. Apel C, Forlenza OV, de Paula VJ, Talib LL, Denecke B, Eduardo CP, et al. The neuroprotective effect of dental pulp cells in models of Alzheimer's and Parkinson's disease. J Neural Transm (Vienna). (2009) 116:71–8. doi: 10.1007/s00702-008-0135-3
64. Nosrat IV, Smith CA, Mullally P, Olson L, Nosrat CA. Dental pulp cells provide neurotrophic support for dopaminergic neurons and differentiate into neurons in vitro; implications for tissue engineering and repair in the nervous system. Eur J Neurosci. (2004) 19:2388–98. doi: 10.1111/j.0953-816X.2004.03314.x
65. Song M, Jue SS, Cho YA, Kim EC. Comparison of the effects of human dental pulp stem cells and human bone marrow-derived mesenchymal stem cells on ischemic human astrocytes in vitro. J Neurosci Res. (2015) 93:973–83. doi: 10.1002/jnr.23569
66. Sugiyama M, Iohara K, Wakita H, Hattori H, Ueda M, Matsushita K, et al. Dental pulp-derived CD31(-)/CD146(-) side population stem/progenitor cells enhance recovery of focal cerebral ischemia in rats. Tissue Eng Part A. (2011) 17:1303–11. doi: 10.089/ten.TEA.2010.0306
67. Yamagata M, Yamamoto A, Kako E, Kaneko N, Matsubara K, Sakai K, et al. Human dental pulp-derived stem cells protect against hypoxic-ischemic brain injury in neonatal mice. Stroke. (2013) 44:551–4. doi: 10.1161/STROKEAHA.112.676759
68. Leong WK, Henshall TL, Arthur A, Kremer KL, Lewis MD, Helps SC, et al. Human adult dental pulp stem cells enhance poststroke functional recovery through non-neural replacement mechanisms. Stem Cells Transl Med. (2012) 1:177–87. doi: 10.5966/sctm.2011-0039
69. Yang C, Li X, Sun L, Guo W, Tian W. Potential of human dental stem cells in repairing the complete transection of rat spinal cord. J Neural Eng. (2017) 14:026005. doi: 10.1088/1741-2552/aa596b
70. Sowa K, Nito C, Nakajima M, Suda S, Nishiyama Y, Sakamoto Y, et al. Impact of dental pulp stem cells overexpressing hepatocyte growth factor after cerebral ischemia/reperfusion in rats. Mol Ther Methods Clin Dev. (2018) 10:281–90. doi: 10.1016/j.omtm.2018.07.009
71. Zhang X, Zhou Y, Li H, Wang R, Yang D, Li B, et al. Intravenous administration of DPSCs and BDNF improves neurological performance in rats with focal cerebral ischemia. Int J Mol Med. (2018) 41:3185–94. doi: 10.892/ijmm.2018.3517
72. Zhang X, Zhou Y, Li H, Wang R, Yang D, Li B, et al. Transplanted dental pulp stem cells migrate to injured area and express neural markers in a rat model of cerebral ischemia. Cell Physiol Biochem. (2018) 45:258–66. doi: 10.1159/000486772
73. Kumasaka A, Kanazawa K, Ohke H, Miura I, Miura Y. Post-ischemic intravenous administration of allogeneic dental pulp-derived neurosphere cells ameliorated outcomes of severe forebrain ischemia in rats. Neurocrit Care. (2017) 26:133–42. doi: 10.1007/s12028-016-0304-4
74. Nito C, Sowa K, Nakajima M, Sakamoto Y, Suda S, Nishiyama Y, et al. Transplantation of human dental pulp stem cells ameliorates brain damage following acute cerebral ischemia. Biomed Pharmacother. (2018) 108:1005–14. doi: 10.1016/j.biopha.2018.09.084
75. Yang KL, Chen MF, Liao CH, Pang CY, Lin PY. A simple and efficient method for generating Nurr1-positive neuronal stem cells from human wisdom teeth (tNSC) and the potential of tNSC for stroke therapy. Cytotherapy. (2009) 11:606–17. doi: 10.1080/14653240902806994
76. Inoue T, Sugiyama M, Hattori H, Wakita H, Wakabayashi T, Ueda M. Stem cells from human exfoliated deciduous tooth-derived conditioned medium enhance recovery of focal cerebral ischemia in rats. Tissue Eng Part A. (2013) 19:24–9. doi: 10.1089/ten.TEA.2011.0385
77. Boltze J, Lukomska B, Jolkkonen J. Mesenchymal stromal cells in stroke: improvement of motor recovery or functional compensation? J Cereb Blood Flow Metab. (2014) 34:1420–1. doi: 10.038/jcbfm.2014.94
78. Moller K, Boltze J, Posel C, Seeger J, Stahl T, Wagner DC. Sterile inflammation after permanent distal MCA occlusion in hypertensive rats. J Cereb Blood Flow Metab. (2014) 34:307–15. doi: 10.1038/jcbfm.2013.199
79. Moller K, Posel C, Kranz A, Schulz I, Scheibe J, Didwischus N, et al. Arterial hypertension aggravates innate immune responses after experimental stroke. Front Cell Neurosci. (2015) 9:461. doi: 10.3389/fncel.2015.00461
80. Siesjo BK. Pathophysiology and treatment of focal cerebral ischemia. Part II: mechanisms of damage and treatment. J Neurosurg. (1992) 77:337–54. doi: 10.3171/jns.1992.77.3.0337
81. Titomanlio L, Fernandez-Lopez D, Manganozzi L, Moretti R, Vexler ZS, Gressens P. Pathophysiology and neuroprotection of global and focal perinatal brain injury: lessons from animal models. Pediatr Neurol. (2015) 52:566–84. doi: 10.1016/j.pediatrneurol.2015.01.016
82. Offner H, Vandenbark AA, Hurn PD. Effect of experimental stroke on peripheral immunity: CNS ischemia induces profound immunosuppression. Neuroscience. (2009) 158:1098–111. doi: 10.16/j.neuroscience.2008.05.033
83. Raposo G, Stoorvogel W. Extracellular vesicles: exosomes, microvesicles, and friends. J Cell Biol. (2013) 200:373–83. doi: 10.1083/jcb.201211138
84. van der Pol E, Boing AN, Harrison P, Sturk A, Nieuwland R. Classification, functions, and clinical relevance of extracellular vesicles. Pharmacol Rev. (2012) 64:676–705. doi: 10.1124/pr.112.005983
85. Ratajczak J, Miekus K, Kucia M, Zhang J, Reca R, Dvorak P, et al. Embryonic stem cell-derived microvesicles reprogram hematopoietic progenitors: evidence for horizontal transfer of mRNA and protein delivery. Leukemia. (2006) 20:847–56. doi: 10.1038/sj.leu.2404132
86. Yu B, Zhang X, Li X. Exosomes derived from mesenchymal stem cells. Int J Mol Sci. (2014) 15:4142–57. doi: 10.3390/ijms15034142
87. Alvarez-Erviti L, Seow Y, Yin H, Betts C, Lakhal S, Wood MJ. Delivery of siRNA to the mouse brain by systemic injection of targeted exosomes. Nat Biotechnol. (2011) 29:341–5. doi: 10.1038/nbt.807
88. Xin H, Li Y, Cui Y, Yang JJ, Zhang ZG, Chopp M. Systemic administration of exosomes released from mesenchymal stromal cells promote functional recovery and neurovascular plasticity after stroke in rats. J Cereb Blood Flow Metab. (2013) 33:1711–5. doi: 10.038/jcbfm.2013.152
89. Kalani A, Chaturvedi P, Kamat PK, Maldonado C, Bauer P, Joshua IG, et al. Curcumin-loaded embryonic stem cell exosomes restored neurovascular unit following ischemia-reperfusion injury. Int J Biochem Cell Biol. (2016) 79:360–9. doi: 10.1016/j.biocel.2016.09.002
90. Jarmalaviciute A, Tunaitis V, Pivoraite U, Venalis A, Pivoriunas A. Exosomes from dental pulp stem cells rescue human dopaminergic neurons from 6-hydroxy-dopamine-induced apoptosis. Cytotherapy. (2015) 17:932–9. doi: 10.1016/j.jcyt.2014.07.013
91. Stanko P, Altanerova U, Jakubechova J, Repiska V, Altaner C. Dental mesenchymal stem/stromal cells and their exosomes. Stem Cells Int. (2018) 2018:8973613. doi: 10.1155/2018/8973613
92. Mita T, Furukawa-Hibi Y, Takeuchi H, Hattori H, Yamada K, Hibi H, et al. Conditioned medium from the stem cells of human dental pulp improves cognitive function in a mouse model of Alzheimer's disease. Behav Brain Res. (2015) 293:189–97. doi: 10.1016/j.bbr.2015.07.043
93. Pivoraite U, Jarmalaviciute A, Tunaitis V, Ramanauskaite G, Vaitkuviene A, Kaseta V, et al. Exosomes from human dental pulp stem cells suppress carrageenan-induced acute inflammation in mice. Inflammation. (2015) 38:1933–41. doi: 10.007/s10753-015-0173-6
94. Kim HS, Choi DY, Yun SJ, Choi SM, Kang JW, Jung JW, et al. Proteomic analysis of microvesicles derived from human mesenchymal stem cells. J Proteome Res. (2012) 11:839–49. doi: 10.1021/pr200682z
95. Vallabhaneni KC, Penfornis P, Dhule S, Guillonneau F, Adams KV, Mo YY, et al. Extracellular vesicles from bone marrow mesenchymal stem/stromal cells transport tumor regulatory microRNA, proteins, and metabolites. Oncotarget. (2015) 6:4953–67. doi: 10.18632/oncotarget.3211
96. Baglio SR, Rooijers K, Koppers-Lalic D, Verweij FJ, Perez Lanzon M, Zini N, et al. Human bone marrow- and adipose-mesenchymal stem cells secrete exosomes enriched in distinctive miRNA and tRNA species. Stem Cell Res Ther. (2015) 6:127. doi: 10.1186/s13287-015-0116-z
97. Kiraly M, Kadar K, Horvathy DB, Nardai P, Racz GZ, Lacza Z, et al. Integration of neuronally predifferentiated human dental pulp stem cells into rat brain in vivo. Neurochem Int. (2011) 59:371–81. doi: 10.1016/j.neuint.2011.01.006
98. Fang CZ, Yang YJ, Wang QH, Yao Y, Zhang XY, He XH. Intraventricular injection of human dental pulp stem cells improves hypoxic-ischemic brain damage in neonatal rats. PLoS ONE. (2013) 8:e66748. doi: 10.1371/journal.pone.0066748
99. Nosrat IV, Widenfalk J, Olson L, Nosrat CA. Dental pulp cells produce neurotrophic factors, interact with trigeminal neurons in vitro, and rescue motoneurons after spinal cord injury. Dev Biol. (2001) 238:120–32. doi: 10.1006/dbio.2001.0400
100. Matsushita K, Motani R, Sakuta T, Yamaguchi N, Koga T, Matsuo K, et al. The role of vascular endothelial growth factor in human dental pulp cells: induction of chemotaxis, proliferation, and differentiation and activation of the AP-1-dependent signaling pathway. J Dent Res. (2000) 79:1596–603. doi: 10.177/00220345000790081201
101. Wada N, Menicanin D, Shi S, Bartold PM, Gronthos S. Immunomodulatory properties of human periodontal ligament stem cells. J Cell Physiol. (2009) 219:667–76. doi: 10.1002/jcp.21710
102. Tomic S, Djokic J, Vasilijic S, Vucevic D, Todorovic V, Supic G, et al. Immunomodulatory properties of mesenchymal stem cells derived from dental pulp and dental follicle are susceptible to activation by toll-like receptor agonists. Stem Cells Dev. (2011) 20:695–708. doi: 10.1089/scd.2010.0145
103. Zhao Y, Wang L, Jin Y, Shi S. Fas ligand regulates the immunomodulatory properties of dental pulp stem cells. J Dent Res. (2012) 91:948–54. doi: 10.1177/0022034512458690
104. Dong Q, Wang Y, Mohabatpour F, Zheng L, Papagerakis S, Chen D, et al. Dental pulp stem cells: isolation, characterization, expansion, and odontoblast differentiation for tissue engineering. Methods Mol Biol. (2019) 1922:91–101. doi: 10.1007/978-1-4939-9012-2_9
105. Nagpal A, Kremer KL, Hamilton-Bruce MA, Kaidonis X, Milton AG, Levi C, et al. TOOTH (The Open study Of dental pulp stem cell Therapy in Humans): study protocol for evaluating safety and feasibility of autologous human adult dental pulp stem cell therapy in patients with chronic disability after stroke. Int J Stroke. (2016) 11:575–85. doi: 10.1177/1747493016641111
Keywords: stem cells, dental pulp stem cells, stroke, brain ischemia, cell therapy
Citation: Lan X, Sun Z, Chu C, Boltze J and Li S (2019) Dental Pulp Stem Cells: An Attractive Alternative for Cell Therapy in Ischemic Stroke. Front. Neurol. 10:824. doi: 10.3389/fneur.2019.00824
Received: 15 March 2019; Accepted: 17 July 2019;
Published: 02 August 2019.
Edited by:
Jean-Marc Olivot, Centre Hospitalier Universitaire de Toulouse, FranceReviewed by:
Yi-Je Chen, University of California, Davis, United StatesJukka Jolkkonen, University of Eastern Finland, Finland
Copyright © 2019 Lan, Sun, Chu, Boltze and Li. This is an open-access article distributed under the terms of the Creative Commons Attribution License (CC BY). The use, distribution or reproduction in other forums is permitted, provided the original author(s) and the copyright owner(s) are credited and that the original publication in this journal is cited, in accordance with accepted academic practice. No use, distribution or reproduction is permitted which does not comply with these terms.
*Correspondence: Shen Li, bGlzdGVubGlzaGVuQGhvdG1haWwuY29t
†These authors have contributed equally to this work